- 1NIBIO - Norwegian Institute of Bioeconomy Research, Ås, Norway
- 2Faculty of Chemistry, Biotechnology and Food Science, Norwegian University of Life Sciences (NMBU), Ås, Norway
- 3Institute of Plant Protection, Chinese Academy of Agricultural Sciences, Haidian, China
Giant panda could have bamboo as their exclusive diet for about 2 million years because of the contribution of numerous enzymes produced by their gut bacteria, for instance laccases. Laccases are blue multi-copper oxidases that catalyze the oxidation of a broad spectrum of phenolic and aromatic compounds with water as the only byproduct. As a “green enzyme,” laccases have potential in industrial applications, for example, when dealing with degradation of recalcitrant biopolymers, such as lignin. In the current study, a bacterial laccase, Lac51, originating from Pseudomonas putida and identified in the gut microbiome of the giant panda’s gut was transiently expressed in the non-food plant Nicotiana benthamiana and characterized. Our results show that recombinant Lac51 exhibits bacterial laccase properties, with optimal pH and temperature at 7–8 and 40°C, respectively, when using syringaldazine as substrate. Moreover, we demonstrate the functional capability of the plant expressed Lac51 to oxidize lignin using selected lignin monomers that serve as substrates of Lac51. In summary, our study demonstrates the potential of green and non-food plants as a viable enzyme production platform for bacterial laccases. This result enriches our understanding of plant-made enzymes, as, to our knowledge, Lac51 is the first functional recombinant laccase produced in plants.
Introduction
Laccases (EC 1.10.3.2, benzenediol:oxygen oxidoreductases) are a group of blue multi-copper oxidoreductases which are able to catalyze the oxidation of phenols and aromatic biopolymers (e.g., lignin) using molecular oxygen. Laccases, e.g., Lac51, a bacterial laccase originating from the gut microbe Pseudomonas putida, are also proposed to play important roles in helping giant panda (Ailuropoda melanoleuca) to subsist on bamboo as their almost exclusive diet, by facilitating the oxidation of lignin moieties, thereby aiding the digestion of bamboo (Zhu et al., 2011; Fang et al., 2012).
Being versatile and eco-friendly enzymes (i.e., not producing toxic peroxide intermediates), laccases have received much attention from scientists and industry. They have been widely applied in food, pulp and paper processing, textile industries, and bioremediation (Chauhan et al., 2017; Yang et al., 2017). Besides, there is a growing interest in their potential utilization for treatment of recalcitrant biomass, such as lignocellulose materials.
In nature, laccases are widely distributed among plants, fungi, bacteria, and insects (Ausec et al., 2011). The most intensively studied ligninolytic laccases and the most applied industrial laccases are from fungi, especially the white-rot basidiomycetes (Martinez et al., 2017). Fungal laccases have a high redox potential and have been used in varying industrial applications, such as food processing, beverage stabilization, and pulp bleaching (Martinez et al., 2017). Unfortunately, fungal laccases are sensitive to temperature and alkaline conditions, which limits their use in industrial applications, where harsh conditions are normally applied in processes such as biomass pretreatment and pulp and paper bleaching (Martinez et al., 2017). In such processes, bacterial laccases could become an alternative as most of them exhibit better tolerance to a broad pH range and high thermostability. For example, the well-known CotA laccase, a coat protein of Bacillus subtilis, displays high thermostability with a half-life of inactivation at 80°C of about 4 h (Martins et al., 2002). Furthermore, a laccase isolated from γ-proteobacterium JB works optimally at 55°C and is highly alkali-tolerant, being stable in the pH range 4–10 (Singh et al., 2007).
Owing to their advantageous physiochemical properties, bacterial laccases may become attractive biocatalysts for diverse industrial applications, including lignin processing. Lignin degradation is an important step to facilitate the ability of downstream oxidases to act on the lignin, thus creating access to plant carbohydrates. To achieve lignin degradation, low-molecular-weight small molecules (e.g., ABTS, HBT, and syringaldazine) could act as redox shuttles between the laccase and the lignin (Chen et al., 2012). By using low-molecular-weight redox mediators, such as 2, 2′-azino-bis (3-ethylbenzothazoline-6-sulfonate) (ABTS) and 1-hydroxybenzotriazole (HBT), the range of substrates a laccase can catalyze can be greatly expanded (Chauhan et al., 2017). Actinomycetes, particularly Streptomyces species which carry small two-domain laccases have been shown to have high lignin-degrading capacity when supplied with redox mediators (Majumdar et al., 2014). In addition, a Comamonas sp. B-9 isolated from eroded bamboo slips exhibits high lignin-degrading activity when grown on kraft lignin as carbon source, which has been attributed to secreted enzymes that include laccases (Chen et al., 2012).
Heterologous expression of bacterial laccases has mostly been done using Escherichia coli as the production host (Martins et al., 2002; Suzuki et al., 2003; Wu et al., 2010; Fang et al., 2012). This approach may suffer from low yields or formation of inclusion bodies, complicating downstream purification (Suzuki et al., 2003). Plants have been used for heterologous production of various industrial enzymes (Kwon et al., 2018) due to the low-cost and flexible production scalability. Indeed, plants have been exploited for expression of different laccases derived from plants and fungi (Hood et al., 2003; Wang et al., 2004; Sonoki et al., 2005; Ligaba-Osena et al., 2017; Preethi et al., 2020), but hardly for laccases of bacterial origin.
In this study, we aimed at transiently expressing a bacterial laccase, denoted as Lac51 and originating from a gut microbe of the giant panda (Fang et al., 2012), using the plant Nicotiana benthamiana, and to investigate the enzymatic properties of the plant-derived Lac51. Next to assessing substrate oxidation, an LC–MS–MS method was used to investigate the fate of substrates 2,6-dimethoxyphenol (2,6-DMP) and 3,4-dihydroxybenzaldehyde (3,4-DHBA) upon oxidation by plant-produced Lac51, thus generating insight into product formation by this laccase. The functional capability of the enzyme to modify lignin was assessed using selected lignin monomers.
Materials and Methods
Lac51 Gene Synthesis
The lac51 gene (GenBank accession number JN867369.1) from Pseudomonas putida (strain L, GenBank accession number AY450556.1; Fang et al., 2012) was chemically synthesized without its native signal peptide by GeneArt (New York, United States) after codon optimization for expression in Nicotiana benthamiana. The original TAT signal peptide at the N-terminus was replaced with the plant-derived signal peptide of Barley α-amylase (GenBank accession number CAX51374) linked with a His6-tag on the N-terminal end of lac51.
Construction of the Lac51 Plant Expression Vector
The lac51 coding region was inserted into the plant transient expression vector pEAQ-HT-DEST1 (Sainsbury et al., 2009), as shown in Figure 1, using a Gateway cloning system provided by Life Technologies (BP&LR Clonase II kit, donor vector pDONR™/Zeo, Invitrogen, United States). The Gateway cloning BP and LR reactions (Karimi et al., 2002) were conducted based on the manufacturer’s protocol, as described previously (Dobrica et al., 2017). Like the lac51 gene expression vector, a vector containing the red fluorescent protein (rfp) reporter gene was constructed.
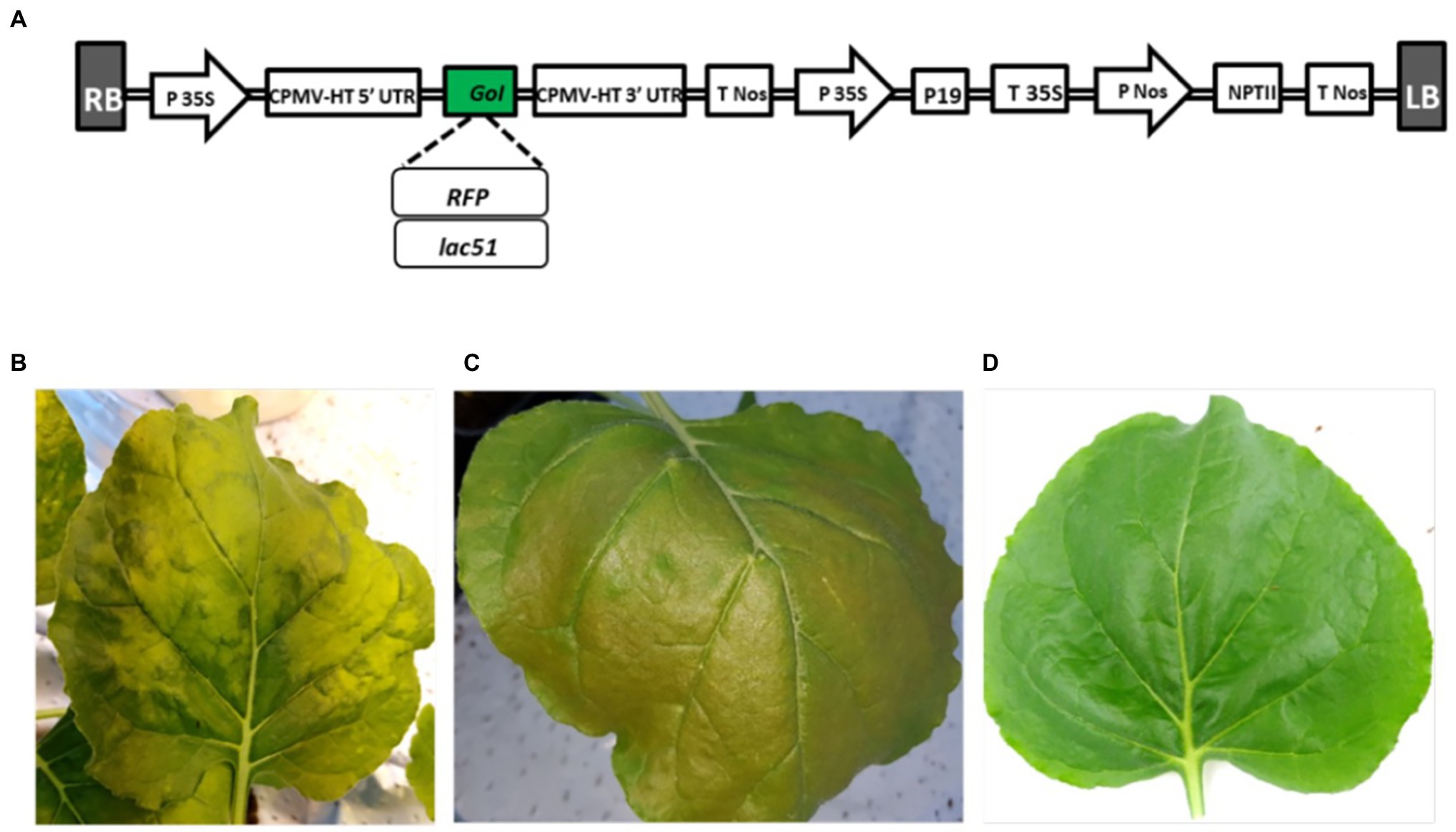
Figure 1. Expression of Lac51. (A) Schematic illustration of lac51 and red fluorescent protein (RFP) gene expression vectors. The Lac51-encoding gene was introduced into the pEAQ-HT-DEST1 vector using Gateway cloning technology. RB and LB, right and left borders; CPMV-HT 5′ UTR and 3′ UTR, 5′ and 3′ untranslated regions derived from Cowpea mosaic virus (CPMV); P 35S and T 35S, 35S promoter and terminator; P Nos and T Nos, nopaline synthase promoter and terminator; P19, suppressor of gene silencing; NPTII, neomycin phosphotransferase II gene conferring kanamycin resistance. (B–D) Phenotype of Nicotiana benthamiana leaves expressing lac51 (B) and the rfp reporter gene (C), where the latter gives a distinguishable red color, at 5 dpi, as compared to a leaf infiltrated only with buffer as control (D).
Agrobacterium-Mediated Transient Expression in Nicotiana benthamiana
The plant expression vectors pEAQ-HT-DEST1/RFP and pEAQ-HT-DEST1/Lac51 were introduced into ElectroMAX™ Agrobacterium tumefaciens LBA4404 cells (Invitrogen, United States) by electroporation using a BTX ECM 630 electroporator (BTX-Division of Harvard Apparatus, United States), with the parameters 2.0 kV, 200 Ω, and 25 μF, as described by Clarke et al. (2008). Agrobacterium LBA4404 harboring the expression vectors was cultured on LB medium supplemented with 50 mg L−1 kanamycin and incubated at 28°C for 72 h. The transformed clones were verified by colony PCR using the gene-specific-attB1/attB2 primer pair (attB1_lac51PSP 5′-GGGGACAAGTTTG TACAAAAAAGCAGGCTAT GGCTAACAAG CACCTGAG-3′; attB2_lac51PSP 5′-GGGGACC ACTTTGTACAAG AAAGCTGGGTCTACTTCA CCTCAATAGCAG-3′).
For transient expression of the lac51 and rfp genes, Agrobacterium cells harboring pEAQ-HT-DEST1/RFP or pEAQ-HT-DEST1/Lac51 were cultured and prepared as described earlier (Clarke et al., 2017; Dobrica et al., 2017) and infused into N. benthamiana, using an in-house vacuum-based agroinfiltration protocol as described previously (Sainsbury et al., 2009). Around 4–6--week-old tobacco plants grown in the growth chamber under a photoperiod of 16 h light and 8 h dark with a temperature of 22°C were subjected to agroinfiltration, using a 2 ml syringe without needle on the underside of the leaves, basically as described by Dobrica et al. (2017). After the agroinfiltration, the N. benthamiana plants were grown further in a greenhouse maintaining the growth conditions and harvested after n—days (representing dpi n—m).
Protein Extraction, Western Blot, and Purification
Protein extraction and Western blot analysis were done as before (Gottschamel and Lössl, 2016), with the following modifications. Briefly, for total protein analysis, frozen and grinded leaf samples were homogenized in pre-cold extraction buffer [0.7 M Sucrose, 0.5 M Tris–HCl pH 9.4, 50 mM EDTA, 0.1 M KCl, 2% β-mercaptoethanol (β-ME), 1x Complete protease inhibitor (CPI; Roche, Switzerland)]. After addition of an equal volume of phenol, mixing and incubation on a shaker for 10 min, and centrifugation at 5,500 g for 10 min at 4°C, the upper green phase was recovered and re-extracted with cold extraction buffer, mixed and incubated on a shaker for 10 min, and centrifuged at 5,500 g for 10 min at 4°C. The upper green phase was collected, and proteins were precipitated by overnight incubation with four volumes of 0.1 M NH4-acetate in methanol at −20°C. The pellet was recovered after centrifugation at 10,000 g for 10 min at 4°C, washed with 0.1 M NH4-acetate in methanol, air-dried, and dissolved in 1% SDS. The total soluble proteins were obtained by incubating the grinded leaf materials. After incubation for 15 min on ice, the supernatant was retained after centrifugation at 20,000 g for 30 min at 4°C. The protein concentration was determined with the Bradford assay (BioRad, United States) using bovine serum albumin (BSA) as standard.
For Western blot analysis, denatured protein samples were separated by electrophoresis in 4–12% SDS-polyacrylamide gels (Invitrogen, United States) and transferred to nitrocellulose membranes (Invitrogen, United States). The membranes were incubated with TBS-T solution (20 mM Tris–HCl pH 7.6, 137 mM NaCl, 0.1% Tween-20) containing 5% BSA as blocking buffer and subsequently treated with the primary and secondary antibodies diluted in TBS-T solution with 1% BSA. The recombinant proteins were detected with 1:5,000 diluted polyclonal anti-polyHistidine antibody produced in mouse, 1:20,000 diluted anti-AP-conjugated mouse IgG secondary antibody (Promega, United States) and a colorimetric reaction using the AP color development kit (Bio-Rad, United States). Protein samples prepared from tobacco leaves infiltrated with buffer were used as negative control.
For purification, powdered Lac51-containing leaf material was mixed with extraction buffer (0.15 M sodium phosphate buffer pH 8.0, 0.3 M KCl, 20 mM β-ME), filtered through Miracloth (Merck, Darmstadt, Germany) and centrifuged at 25,000 g, Lac51 was captured from the resulting supernatant by affinity chromatography using Ni2+-NTA beads (Qiagen, Hilden, Germany), washed with wash buffer (0.1 M sodium phosphate buffer pH 8.0, 0.3 M KCl, 10 mM imidazole), and eluted with elution buffer (0.1 M sodium phosphate buffer pH 8.0, 0.3 M KCl, 0.3 M imidazole). The eluted protein was concentrated using a 30 kDa MWCO Macrosep ultrafiltration device (Pall, Port Washington, United States) and buffer was exchanged to 0.05 M sodium phosphate buffer pH 8.0, 0.1 M KCl.
Enzyme Activity Determination Assays
Laccase activity was assessed with 2,2′-azino-bis (3-ethylbenzothiazoline-6-sulphonic acid; ABTS, Roche diagnostic, Mannheim, Germany) and syringaldazine (Thermo Scientific, Rockford, United States) as model substrates. As a reference, TvLac from Trametes versicolor (Sigma Aldrich, Saint Louis, MO, United States) was used. Enzymatic reactions were set up in 96-well plates with 200 μl total reaction volumes containing 0.1 mM substrate (ABTS or syringaldazine) in 50 mM Na-acetate buffer pH 5.0. The plate was incubated in an Eppendorf Thermomixer C (Eppendorf AG, Hamburg, Germany) at 37°C and 400 rpm and scanned with a Synergy H4 Hybrid plate reader (BioTek, Winooski, VT, United States) at 0.5, 5, 10, 20, and 30 min. Reactions were scanned at 350 and 740 nm for detecting ABTS and the ABTS radical, respectively, and at 530 nm for detecting the oxidized form of syringaldazine. In addition, the full spectrum of the final reactions, from 300 nm to 800 nm, was recorded after 31 min (see Supplementary Figure S1).
Determination of pH and Temperature Optima of the Plant Produced Lac51
To evaluate the biochemical activity of Lac51 in different conditions, syringaldazine was used as substrate. The assay system contained Cu2+-saturated Lac51, 50 mM Na-acetate pH 5.0, and 30 μM syringaldazine in a final volume of 0.1 ml. The absorbance was measured at 530 nm for over 30 min at 37°C. The effect of pH on laccase activity was determined using the following buffers: 50 mM Na-acetate buffer (pH 3.0–5.0), 50 mM K2HPO4-KH2PO4 buffer (pH 6.0–8.0), and 50 mM Tris–HCl buffer (pH 7.0–9.0). The effect of temperature was measured from 25 to 50°C in 50 mM Tris–HCl (pH 7.0).
Enzymatic Reaction With Lignin Monomers
Seven phenolic compounds were tested as potential substrates of Lac51: 2,6-DMP, 3,4-dimethoxybenzalcohol (3,4-DMBA), 3,4-dihydroxybenzaldehyde (3,4-DHBA), 4-methoxybenzylalcohol (4-MBA), gallic acid (GA), vanillin (V), and guaiacol (G), all purchased from Sigma Aldrich (Saint Louis, MO, United States). Enzymatic reactions were carried out in 2-ml Eppendorf tubes with 0.2 ml of total volume. The reactions contained 3 mM substrate and Lac51 or TvLac in 10 mM BisTris-HCl buffer pH 6.5. The reactions were incubated in an Eppendorf Thermomixer C (Eppendorf AG) at 37°C and 800 rpm for 24 h. After 24 h, the reactions were filtered through a 96-well filter plate equipped with 0.45-μm filter membrane (Merck Millipore Ltd., Tullagreen Carrigtwohill, Ireland) and analyzed with high performance liquid chromatography (HPLC) using a Dionex Ultimate 3000 (Dionex, Sunnyvale, United States) equipped with an Agilent Eclipse Plus C18 RRND 1.8 μm, 2.1 × 50 mm column equilibrated at 30°C and coupled to a Dionex Ultimate 3000 RS VWD UV detector and an LTQ Velos Pro Mass Spectrometer detector (Thermo Scientific, Rockford, United States). The equipment used was as follows: Column: Agilent Eclipse Plus C18 RRND 1.8 μm, 2.1 × 50 mm column; column temperature: 30°C; flow rate: 0.4 ml/min; detection: UV coupled to MS with direct flow; UV–Vis recorded at 315, 450, and 600 nm; MS scan in the m/z range 85–1,200. Initially, the column was conditioned with a mixture of 5% eluent B (100% ACN with 0.1% TFA) and 95% eluent A (100% H2O with 0.1% TFA) for 0.5 min, followed by a linear gradient increasing eluent B from 5 to 95% over 9.5 min. The concentration of eluent B was kept at 95% for 5 min, then increased linearly to 100% over 1 min. Next, the concentration of eluent B was set back to 5% with a linear gradient over 0.5 min. The chromatographic profile ended with reconditioning the column at 5% eluent B for 9 min before the next injection.
Results
Expression and Purification of Lac51 in Nicotiana benthamiana
The lac51- and rfp-expressing vectors (Figure 1A) were constructed and successfully introduced in N. benthamiana plants (leaves) using vacuum infiltration. Five days after infiltration (dpi), phenotypical alterations (Figures 1B,C) could be found when compared with the wild-type control (infiltrated with buffer; Figure 1D). To identify the optimal harvest time, material from lac51-expressing plant leaves was harvested at different days post infiltration (5, 7, 9, and 12) for analysis of Lac51 accumulation. Total proteins were isolated and subjected to Western blot analysis (Figure 2), which showed accumulation of Lac51, peaking at 7 dpi (Figure 2A). After 7 dpi, the amount of target protein declined rapidly, potentially indicating protein instability. Then, Lac51 was extracted and purified from leaves harvested at 7 dpi, using Ni-affinity chromatography and subsequently used for activity assays.
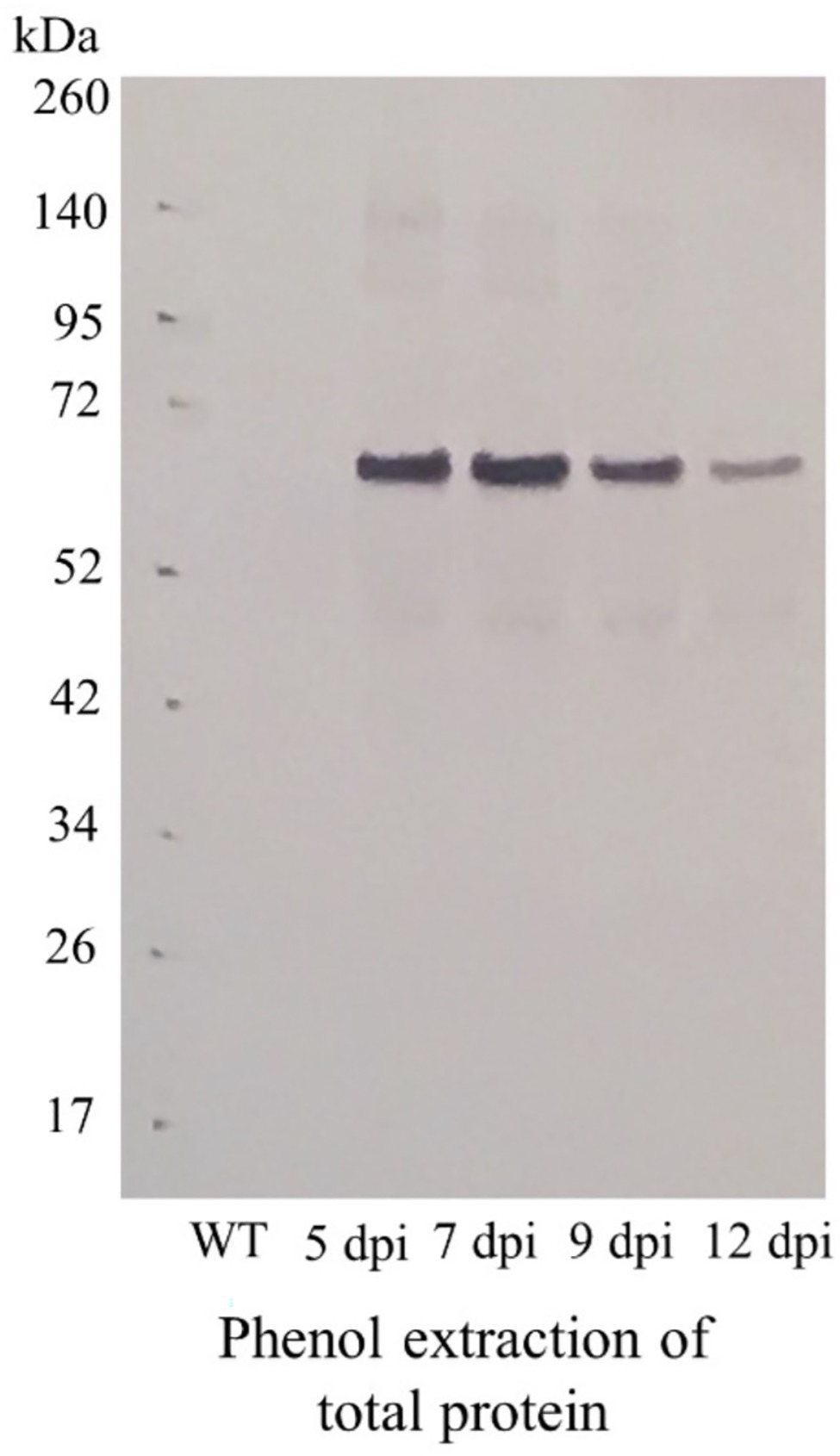
Figure 2. Accumulation of Lac51 protein in Nicotiana benthamiana leaves. Immunoblot analysis of total protein extracts from N. benthamiana leaves infiltrated with the lac51 construct, harvested 5, 7, 9, and 12 days after infiltration (dpi) or non-infiltrated leaves (WT).
Lac51 Enzyme Activity on Model Substrates
Expression of an active Lac51 protein in N. benthamiana was confirmed with the model substrate syringaldazine, using TvLac (Trametes versicolor laccase) as positive control. Lac51 was able to oxidize syringaldazine to its quinone form (indicated by an increase in absorbance at 530 nm), and in the first 10 min of the incubation, the activity of Lac51 was comparable with that of TvLac (Figure 3). However, while TvLac remained active up to 30 min (i.e., throughout the course of the reaction), the increase in the signal at 530 nm stopped after 10 min in reactions with Lac51, indicating inactivation of the enzyme.
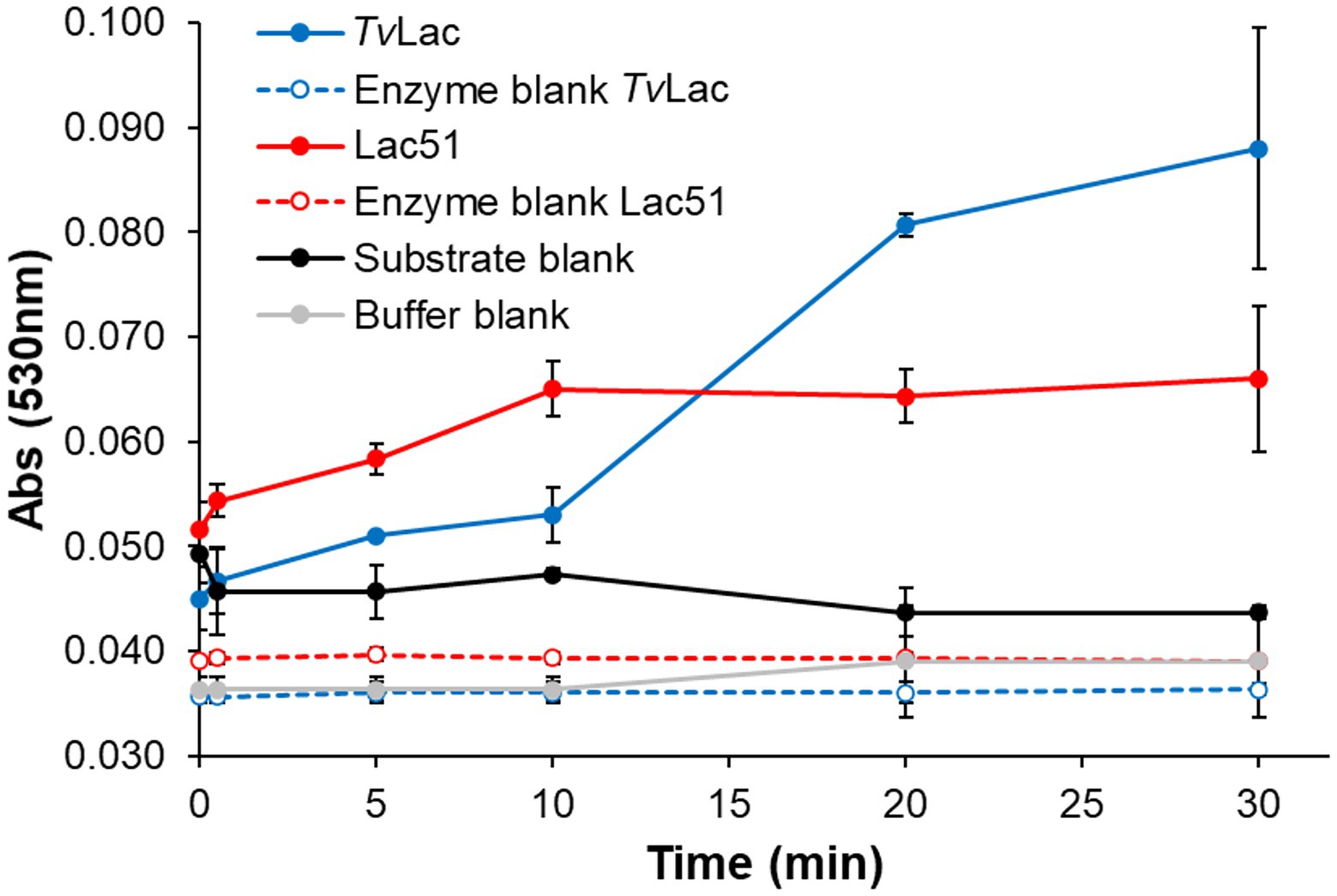
Figure 3. Laccase activity with syringaldazine. Syringaldazine (0.1 mM) was incubated with 0.4 μM Lac51 (red) or 0.2 μM TvLac (blue) in 50 mM Na-acetate buffer pH 5.0 at 37°C. The reaction was followed by detecting the formation of syringaldazine quinone at 530 nm. Substrate (black line) and enzyme blanks (dashed lines) were prepared by omitting enzymes and substrate, respectively; the buffer blank (gray line) lacked both enzyme and substrate. Error bars indicate SD of triplicates.
Lac51 was also incubated with ABTS (Figure 4), which is regarded as one of the most sensitive substrates for detecting laccase activity (Li et al., 2008). Accordingly, the control laccase TvLac generated ABTS radicals at a constant rate (Figure 4B), which coincided with a decrease in the ABTS concentration (Figure 4A; Supplementary Figure S1). On the other hand, incubating ABTS with Lac51 led to only a small decrease in the concentration of the substrate within the initial 5 min, after which the ABTS concentration remained constant throughout the reaction (Figure 4A). In addition, Lac51 generated no detectable amounts of ABTS radicals (Figure 4B). It is noteworthy that the ligninolytic activity of two enzymes is best compared based on substrate consumption because laccases catalyze radical reactions and generate a broad range of products, some of which may be polymeric and, hence, insoluble in the reaction medium.
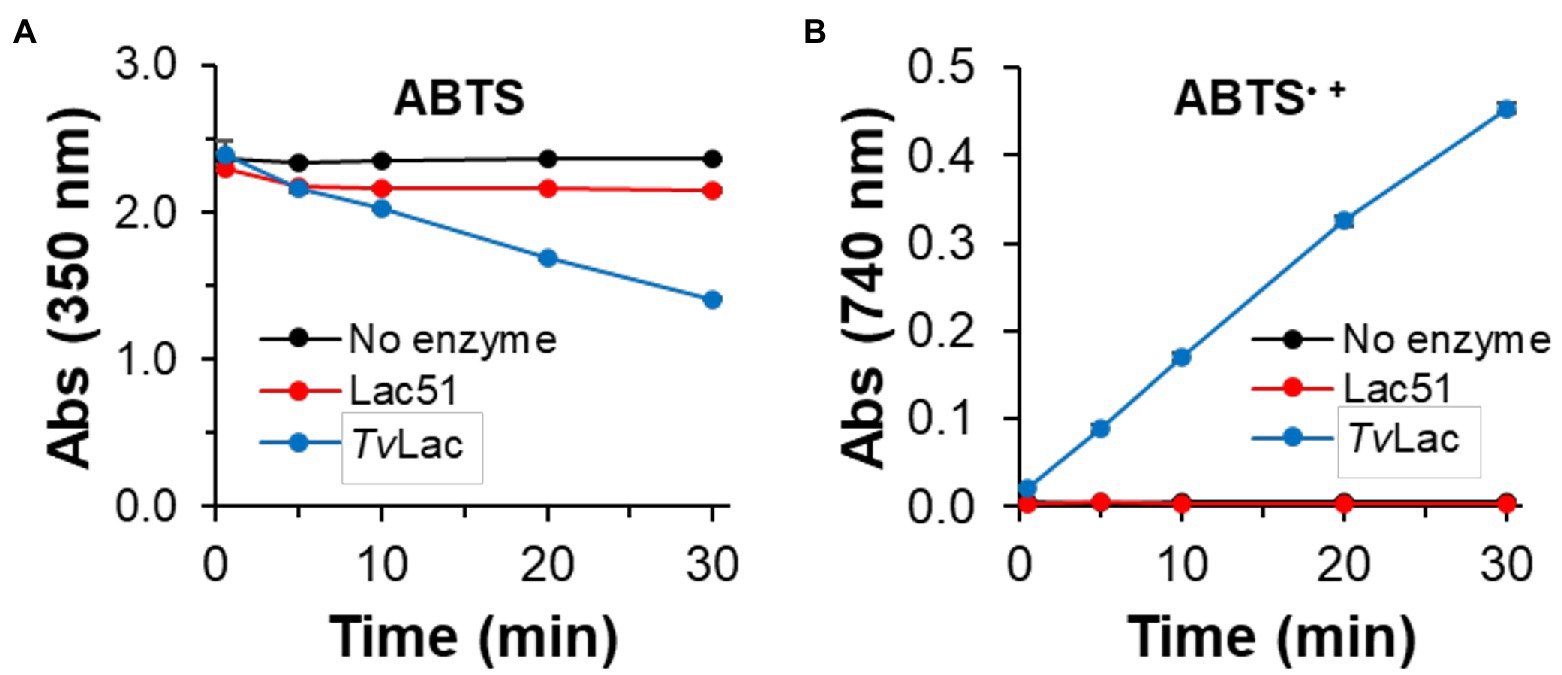
Figure 4. Laccase activity using ABTS. ABTS (0.1 mM) was incubated with 0.4 μM Lac51 (red) or 0.4 μM TvLac (blue) in 50 mM Na-acetate buffer pH 5.0 at 37°C. The reaction was followed by detecting (A) the consumption of ABTS at 350 nm and (B) the formation of ABTS•+ radical cations at 740 nm. Error bars indicate SD of triplicates.
Determination of pH and Temperature Optima of Lac51 Using Syringaldazine
As Lac51 showed measurable activity on syringaldazine, we used syringaldazine as a substrate and measured the rate of substrate consumption in the pH range of 3.0–9.0 and temperature range of 25–50°C. The optimal pH for the oxidation of syringaldazine was found to be 7.0, and the optimal temperature at pH 7.0 was found to be 40°C, as shown in Figure 5.
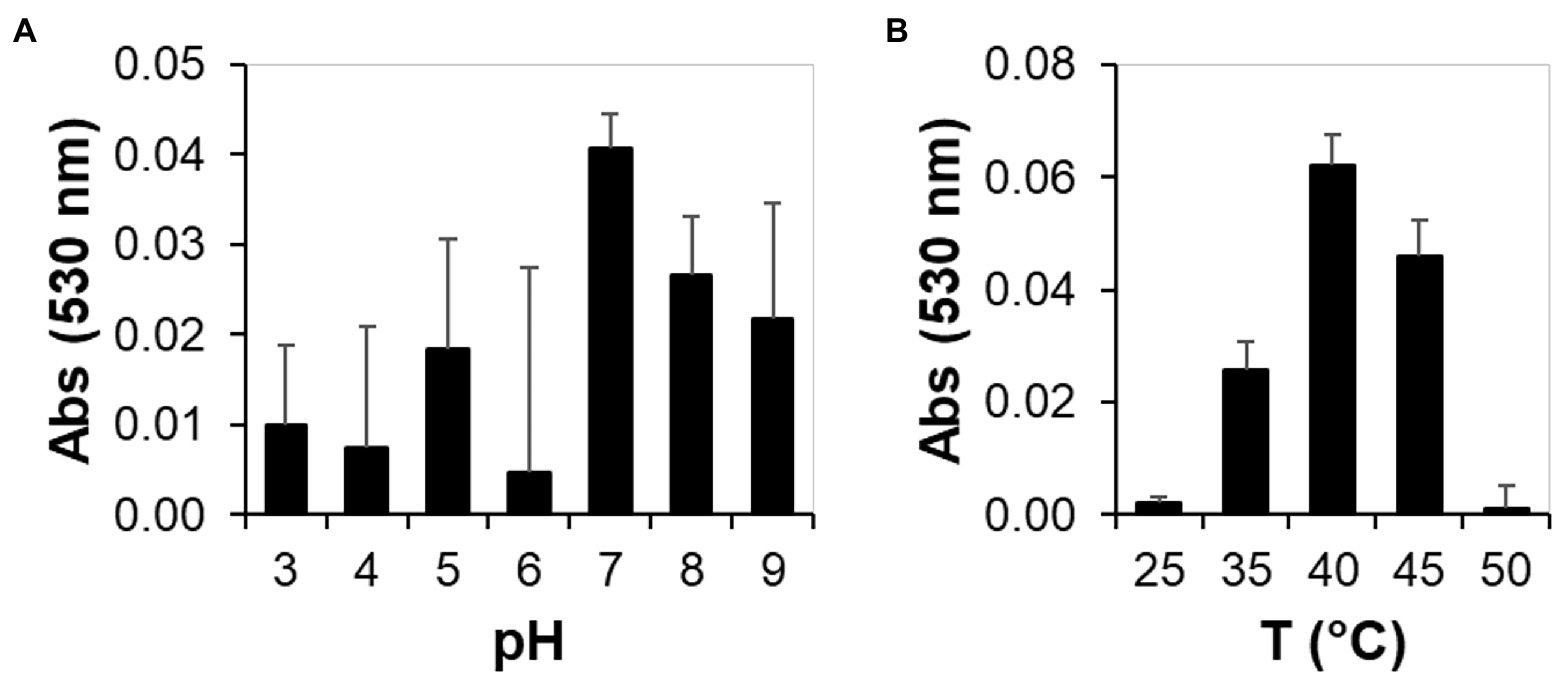
Figure 5. Biochemical activity of plant expressed Lac51 toward syringaldazine under different pH and temperature conditions. Syringaldazine assay was performed in different conditions containing 0.4 μM Cu2+-saturated Lac51, 50 mM Na-acetate, and 30 μM syringaldazine in a final volume of 0.1 ml with different pH (A) and temperature (B). The absorbance was measured at 530 nm for over 30 min at 37°C.
Enzyme Activity on Lignin Monomers
Lac51 and the positive control TvLac were tested for activity on seven lignin monomers. Spectrophotometric analysis revealed that Lac51 was active on three (2,6-DMP, 3,4-DHBA, and GA), while TvLac was active on five of these seven substrates [2,6-DMP, 3,4-DHBA, GA, vanillin (V), and guaiacol (G); Table 1]. After 24 h incubation, Lac51 oxidized 13% of 2,6-DMP, 41% of 3,4-DHBA and 19% of GA (Figure 6). TvLac, on the other hand, reacted more readily with these substrates, oxidizing completely 2,6-DMP and 3,4-DHBA, and leaving only 2% of GA behind in the reaction after 24 h. The action of both enzymes led to formation of insoluble residues in the reactions with 2,6-DMP and GA, presumably through polymerization of the lignin monomers.
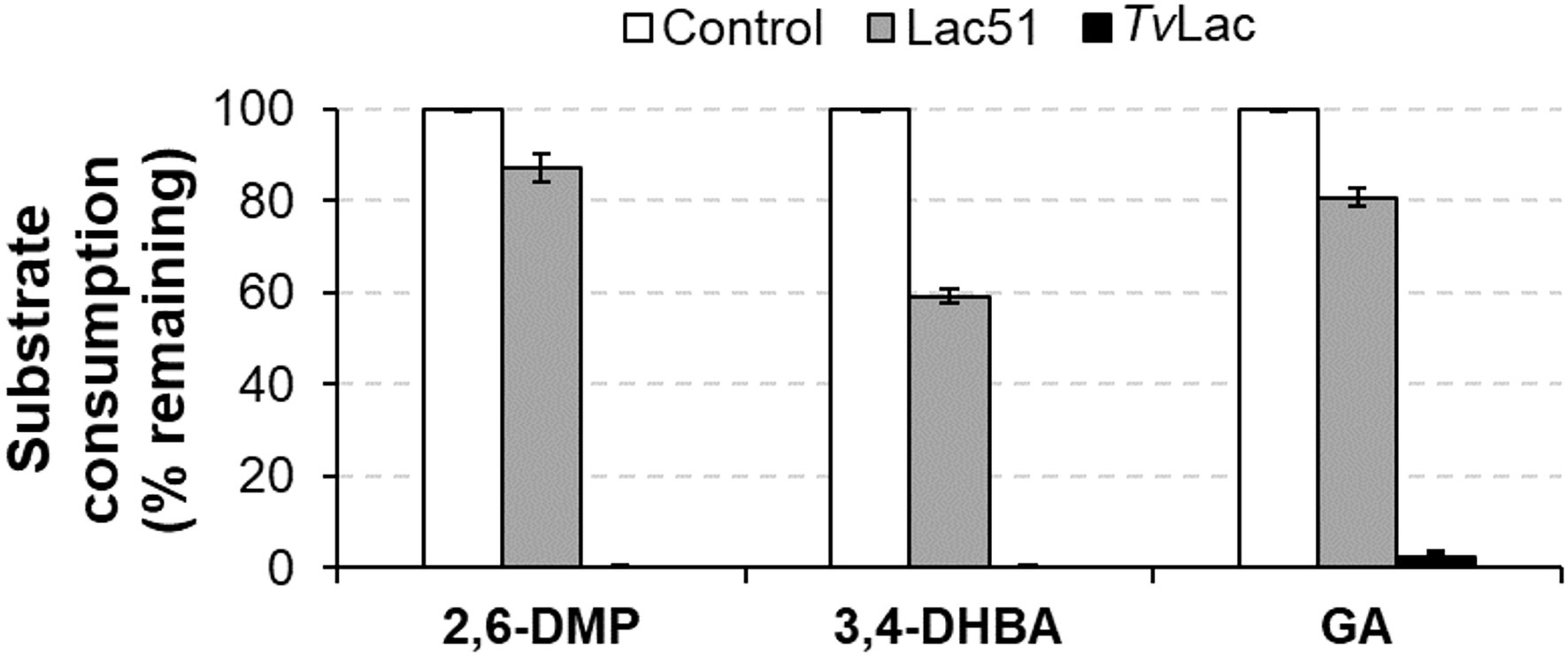
Figure 6. Activity of Lac51 and TvLac on 2,6-dimethoxyphenol (2,6-DMP), 3,4-dihydroxybenzaldehyde (3,4-DHBA), and gallic acid (GA). Lignin monomers (3 mM) were incubated with 2 μM Lac51 or TvLac in 10 mM BisTris-HCl buffer pH 6.5 at 37°C for 24 h. The reaction was followed by detecting the consumption of substrate at 315 nm for 2,6-DMP and GA and at 450 nm for 3,4-DHBA. The control reactions contained the substrates without enzyme. Reactions were run in triplicate; error bars represent SDs.
Formation of soluble laccase reaction products was confirmed with LC-UV/MS2 analysis (Supplementary Figure S2; Table 2; Supplementary Table S1). The product profiles of the two enzymes, judged from both UV (Supplementary Figure S2) and MS profiles (Table 2; Supplementary Table S1) differed on all three substrates, which in part may be due to the differences in the extent of substrate conversion and/or polymerization. When subjecting the compounds detected by MS to MS2 fragmentation (Supplementary Table S1), we could identify signals corresponding to the oxidized dimer of 2,6-DMP (with m/z value 305.00 in Table 2). In the reaction of 2,6-DMP with TvLac (which led to complete conversion of the substrate, Figure 6), products accumulated mostly in the insoluble fraction, leaving virtually no low-molecular-weight components in solution, apart from small amounts of the oxidized dimer (Supplementary Figure S2A; Table 2). As insoluble polymer was also observed when treating 2,6-DMP with Lac51, albeit in a smaller amount than with TvLac, it is likely that the peaks observed in the reaction with Lac51 are the intermediate oligomeric products that are polymerized further to form the insoluble residue. Notably, the concentration of the oxidized dimer of 2,6-DMP (at 6.67 min in Supplementary Figure S2A) corresponded to the extent of substrate conversion by the two enzymes on 2,6-DMP (Figure 6).
Discussion
In the current study, we assessed transient expression of a bacterial laccase, Lac51, originating from Pseudomonas putida and identified in the giant panda’s gut, in the non-food plant N. benthamiana using a vacuum infiltration-based system, providing a low-cost alternative to bacterial and eukaryotic expression hosts. Plants or plant cells/tissues have been used to express valuable recombinant proteins, including vaccines, enzymes, and biopharmaceuticals in molecular farming and have been established as an economically viable (and scalable) alternative to mainstream production systems (Clarke et al., 2013; Bock, 2015; Buyel et al., 2017). In general, nuclear or transient expression is preferred for expression of eukaryotic proteins, mostly when post-translational modification (e.g., glycosylation) of the target protein is a requirement for protein functionality or stability, whereas plastid expression is often the more common choice for expression of bacterial proteins (Xu et al., 2012). Plants have also been exploited for expression of different plant cell wall-active enzymes, including laccases derived from plants and fungi (Hood et al., 2003; Wang et al., 2004; Sonoki et al., 2005), but not laccases of bacterial origin (Preethi et al., 2020). Plant genetic engineering technologies have expanded the diversity of well-established plant-based bioproduction platforms (Paul and Ma, 2011; Xu et al., 2012) and also expressing systems, while stable transgenic lines display high yield of the final product and transient expressing plants exhibit fast processes (Gecchele et al., 2015).
In this work, the recombinant His6-tagged Lac51 was successfully cloned and transiently expressed in N. benthamiana, and the plant-derived Lac51 was shown to be produced in a catalytically active form. Notably, protein instability affected Lac51 accumulation over time, showing the impact of harvest time on the protein yield. Overexpression of plant cell wall-degrading enzymes especially in plant chloroplasts, which is often used for bacterial enzymes, can be a severe physiological burden for the host plant and limit plant growth (Oey et al., 2009). Apart from minor alterations in the leaf tissue (as compared to the RFP control), this was not the case during transient expression of Lac51 (Figure 1). The present results show the capability and potential of plant produced enzymes, that, however, still needs further research.
The catalytic activity of the plant produced Lac51 was compared to that of a commercially available fungal laccase TvLac from Trametes versicolor (this study) and to literature data for a recombinant Lac51 expressed in Escherichia coli [an earlier study by Fang et al. (2012)]. In general, the plant-expressed Lac51 was found to have similar substrate specificity as well as similar pH (7–8) and temperature (40–45°C) optima on syringaldazine compared to the E. coli-expressed variant (Fang et al., 2012). Differences in apparent operational stability may be attributed to differences in reaction conditions, the reaction time in particular [5 min vs. 30 min in Fang et al. (2012) and this study, respectively]. When comparing the (plant expressed) bacterial Lac51 to the fungal TvLac (this study), there were clear differences in substrate specificity and operational stability between the two enzymes under the tested conditions. In general, TvLac acted more readily with the tested substrates (Figures 4, 6) and retained its activity over a longer time period (Figure 3). The differences in operational stability (Figure 3) and possible mode of action (see Figure 4) indicate that laccases need to be selected carefully for targeted applications.
In summary, we transiently expressed a bacterial laccase originating from a giant panda gut microbe, denoted as Lac51, which is the first functional recombinant laccase produced in plants. The plant-expressed Lac51 showed enzymatic properties and lignin degradation potential similar to the E. coli-expressed variant reported earlier (Fang et al., 2012). Thus, our study demonstrates successful production of a catalytically active bacterial laccase and further shows the potential of green and non-food plants e.g., N. benthamiana as an economical enzyme production platform for the future production of bacterial enzymes.
Data Availability Statement
The original contributions presented in the study are included in the article/Supplementary Material, further inquiries can be directed to the corresponding author.
Author Contributions
AE, AV, HE, VE, and JC conceived and designed the study. LP, YW, and FQ carried out vector design, sequencing and participated in agroinfiltration. YW and AE performed protein purification and analysis, J-KJ analyzed enzyme properties. AE, YW, AV, HS, and JC drafted the manuscript. All authors contributed to the article and approved the submitted version.
Funding
This work was supported by the NIBIO core funding “ArcGene” project 11145 and the Research Council of Norway, through grants 243974 (Bioboost) and 270038 (NorBioLab).
Conflict of Interest
The authors declare that the research was conducted in the absence of any commercial or financial relationships that could be construed as a potential conflict of interest.
Publisher’s Note
All claims expressed in this article are solely those of the authors and do not necessarily represent those of their affiliated organizations, or those of the publisher, the editors and the reviewers. Any product that may be evaluated in this article, or claim that may be made by its manufacturer, is not guaranteed or endorsed by the publisher.
Acknowledgments
The authors thank Hege S. Steen, Sissel Haugslien, and Inger Heldal for their technical support to this study.
Supplementary Material
The Supplementary Material for this article can be found online at: https://www.frontiersin.org/articles/10.3389/fpls.2022.912293/full#supplementary-material
References
Ausec, L., Zakrzewski, M., Goesmann, A., Schluüter, A., and Mandic-Mulec, I. (2011). Bioinformatic analysis reveals high diversity of bacterial genes for laccase-like enzymes. PLoS One 6:e25724. doi: 10.1371/journal.pone.0025724
Bock, R. (2015). Engineering plastid genomes: methods, tools, and applications in basic research and biotechnology. Annu. Rev. Plant Biol. 66, 211–241. doi: 10.1146/annurev-arplant-050213-040212
Buyel, J. F., Twyman, R. M., and Fischer, R. (2017). Very-large-scale production of antibodies in plants: the biologization of manufacturing. Biotechnol. Adv. 35, 458–465. doi: 10.1016/j.biotechadv.2017.03.011
Chauhan, P. S., Goradia, B., and Saxena, A. (2017). Bacterial laccase: recent update on production, properties and industrial applications. 3 Biotech 7:323. doi: 10.1007/s13205-017-0955-7
Chen, Y. H., Chai, L. Y., Zhu, Y. H., Yang, Z. H., Zheng, Y., and Zhang, H. (2012). Biodegradation of kraft lignin by a bacterial strain Comamonas sp. B-9 isolated from eroded bamboo slips. J. Appl. Microbiol. 112, 900–906. doi: 10.1111/j.1365-2672.2012.05275.x
Clarke, J. L., Paruch, L., Dobrica, M.-O., Caras, I., Tucureanu, C., Onu, A., et al. (2017). Lettuce-produced hepatitis C virus E1E2 heterodimer triggers immune responses in mice and antibody production after oral vaccination. Plant Biotechnol. J. 15, 1611–1621. doi: 10.1111/pbi.12743
Clarke, J. L., Spetz, C., Haugslien, S., Xing, S., Dees, M. W., Moe, R., et al. (2008). Agrobacterium tumefaciens-mediated transformation of poinsettia, Euphorbia pulcherrima, with virus-derived hairpin RNA constructs confers resistance to Poinsettia mosaic virus. Plant Cell Rep. 27, 1027–1038. doi: 10.1007/s00299-008-0526-9
Clarke, J. L., Waheed, M. T., and Lössl, A. G. (2013). How can plant genetic engineering contribute to cost-effective fish vaccine development for promoting sustainable aquaculture? Plant Mol. Biol. 83, 33–40. doi: 10.1007/s11103-013-0081-9
Dobrica, M. O., Lazar, C., Paruch, L., Skomedal, H., Steen, H., Haugslien, S., et al. (2017). A novel chimeric hepatitis B virus S/preS1 antigen produced in mammalian and plant cells elicits stronger humoral and cellular immune response than the standard vaccine-constituent, S protein. Antivir. Res. 144, 256–265. doi: 10.1016/j.antiviral.2017.06.017
Fang, W., Fang, Z., Zhou, P., Chang, F., Hong, Y., Zhang, X., et al. (2012). Evidence for lignin oxidation by the giant panda fecal microbiome. PLoS One 7:e50312. doi: 10.1371/journal.pone.0050312
Gecchele, E., Merlin, M., Brozzetti, A., Falorni, A., Pezzotti, M., and Avesani, L. (2015). A comparative analysis of recombinant protein expression in different biofactories: bacteria, insect cells and plant systems. J. Vis. Exp. 97:e52459. doi: 10.3791/52459
Gottschamel, J., and Lössl, A. (2016). Erratum to: chloroplast-based expression of recombinant proteins by gateway cloning technology. Methods Mol. Biol. 1385:E1. doi: 10.1007/978-1-4939-3289-4_20
Hood, E. E., Bailey, M. R., Beifuss, K., Magallanes-Lundback, M., Horn, M. E., Callaway, E., et al. (2003). Criteria for high-level expression of a fungal laccase gene in transgenic maize. Plant Biotechnol. J. 1, 129–140. doi: 10.1046/j.1467-7652.2003.00014.x
Karimi, M., Inze, D., and Depicker, A. (2002). GATEWAYTM vectors for Agrobacterium-mediated plant transformation. Trends Plant Sci. 7, 193–195. doi: 10.1016/s1360-1385(02)02251-3
Kwon, K. C., Sherman, A., Chang, W. J., Kamesh, A., Biswas, M., Herzog, R. W., et al. (2018). Expression and assembly of largest foreign protein in chloroplasts: oral delivery of human FVIII made in lettuce chloroplasts robustly suppresses inhibitor formation in haemophilia A mice. Plant Biotechnol. J. 16, 1148–1160. doi: 10.1111/pbi.12859
Li, A., Zhu, Y., Xu, L., Zhu, W., and Tian, X. (2008). Comparative study on the determination of assay for laccase of Trametes sp. Afr. J. Biochem. Res. 2, 181–183. doi: 10.5897/AJBR.9000064
Ligaba-Osena, A., Hankoua, B., DiMarco, K., Pace, R., Crocker, M., McAtee, J., et al. (2017). Reducing biomass recalcitrance by heterologous expression of a bacterial peroxidase in tobacco (Nicotiana benthamiana). Sci. Rep. 7:17104. doi: 10.1038/s41598-017-16909-x
Majumdar, S., Lukk, T., Solbiati, J. O., Bauer, S., Nair, S. K., Cronan, J. E., et al. (2014). Roles of small laccases from Streptomyces in lignin degradation. Biochemistry 53, 4047–4058. doi: 10.1021/bi500285t
Martinez, A. T., Ruiz-Duenas, F. J., Camarero, S., Serrano, A., Linde, D., Lund, H., et al. (2017). Oxidoreductases on their way to industrial biotransformations. Biotechnol. Adv. 35, 815–831. doi: 10.1016/j.biotechadv.2017.06.003
Martins, L. O., Soares, C. M., Pereira, M. M., Teixeira, M., Costa, T., Jones, G. H., et al. (2002). Molecular and biochemical characterization of a highly stable bacterial laccase that occurs as a structural component of the Bacillus subtilis endospore coat. J. Biol. Chem. 277, 18849–18859. doi: 10.1074/jbc.M200827200
Oey, M., Lohse, M., Kreikemeyer, B., and Bock, R. (2009). Exhaustion of the chloroplast protein synthesis capacity by massive expression of a highly stable protein antibiotic. Plant J. 57, 436–445. doi: 10.1111/j.1365-313X.2008.03702.x
Paul, M., and Ma, J. K. C. (2011). Plant-made pharmaceuticals: leading products and production platforms. Biotechnol. Appl. Biochem. 58, 58–67. doi: 10.1002/bab.6
Preethi, P. S., Gomathi, A., Srinivasan, R., Kumar, J. G. S. P., Murugesan, K., and Kodiveri, M. G. (2020). “Laccase: recombinant expression, engineering and its promising applications,” in Microbial Enzymes: Roles and Applications in Industries. Microorganisms for Sustainability, Vol. 11 (Singapore: Springer).
Sainsbury, F., Thuenemann, E. C., and Lomonossoff, G. P. (2009). pEAQ: versatile expression vectors for easy and quick transient expression of heterologous proteins in plants. Plant Biotechnol. J. 7, 682–693. doi: 10.1111/j.1467-7652.2009.00434.x
Singh, G., Capalash, N., Goel, R., and Sharma, P. (2007). A pH-stable laccase from alkali-tolerant γ-proteobacterium JB: purification, characterization and indigo carmine degradation. Enzym. Microb. Technol. 41, 794–799. doi: 10.1016/j.enzmictec.2007.07.001
Sonoki, T., Kajita, S., Ikeda, S., Uesugi, M., Tatsumi, K., Katayama, Y., et al. (2005). Transgenic tobacco expressing fungal laccase promotes the detoxification of environmental pollutants. Appl. Microbiol. Biotechnol. 67, 138–142. doi: 10.1007/s00253-004-1770-8
Suzuki, T., Endo, K., Ito, M., Tsujibo, H., Miyamoto, K., and Inamori, Y. (2003). A thermostable laccase from Streptomyces lavendulae REN-7: purification, characterization, nucleotide sequence, and expression. Biosci. Biotechnol. Biochem. 67, 2167–2175. doi: 10.1271/bbb.67.2167
Wang, G., Li, Q., Luo, B., and Chen, X. (2004). Ex planta phytoremediation of trichlorophenol and phenolic allelo chemicals via an engineered secretory laccase. Nat. Biotechnol. 22, 893–897. doi: 10.1038/nbt982
Wu, J., Kim, K. S., Lee, J. H., and Lee, Y. C. (2010). Cloning, expression in Escherichia coli, and enzymatic properties of laccase from Aeromonas hydrophila WL-11. J. Environ. Sci. 22, 635–640. doi: 10.1016/S1001-0742(09)60156-X
Xu, J., Dolan, M. C., Medrano, G., Cramer, C. L., and Weathers, P. J. (2012). Green factory: plants as bioproduction platforms for recombinant proteins. Biotechnol. Adv. 30, 1171–1184. doi: 10.1016/j.biotechadv.2011.08.020
Yang, J., Li, W., Ng, T. B., Deng, X., Lin, J., and Ye, X. (2017). Laccases: production, expression regulation, and applications in pharmaceutical biodegradation. Front. Microbiol. 8:832. doi: 10.3389/fmicb.2017.00832
Keywords: bacterial laccase, lignin degrading enzymes, transient expression, tobacco, plant biotechnology
Citation: van Eerde A, Várnai A, Wang Y, Paruch L, Jameson J-K, Qiao F, Eiken HG, Su H, Eijsink VGH and Clarke JL (2022) Successful Production and Ligninolytic Activity of a Bacterial Laccase, Lac51, Made in Nicotiana benthamiana via Transient Expression. Front. Plant Sci. 13:912293. doi: 10.3389/fpls.2022.912293
Edited by:
Junhua Peng, Huazhi Bio-Tech Co., Ltd., ChinaReviewed by:
Valentina Perna, Technical University of Denmark, DenmarkJianguo Su, Huazhong Agricultural University, China
Chao Ran, Feed Research Institute (CAAS), China
Copyright © 2022 van Eerde, Várnai, Wang, Paruch, Jameson, Qiao, Eiken, Su, Eijsink and Clarke. This is an open-access article distributed under the terms of the Creative Commons Attribution License (CC BY). The use, distribution or reproduction in other forums is permitted, provided the original author(s) and the copyright owner(s) are credited and that the original publication in this journal is cited, in accordance with accepted academic practice. No use, distribution or reproduction is permitted which does not comply with these terms.
*Correspondence: Jihong Liu Clarke, amlob25nLmxpdS1jbGFya2VAbmliaW8ubm8=