- 1CAS Key Laboratory of Forest Ecology and Management, Institute of Applied Ecology, Chinese Academy of Sciences, Shenyang, China
- 2School of Life and Environmental Sciences, Sydney Institute of Agriculture, The University of Sydney, Sydney, NSW, Australia
- 3National Engineering Laboratory for Efficient Utilization of Soil and Fertilizer Resources, College of Land and Environment, Shenyang Agricultural University, Shenyang, China
- 4Environmental Studies Department, University of California, Santa Cruz, Santa Cruz, CA, United States
Photosynthetically derived carbon (C) is allocated belowground, allowing plants to obtain nutrients. However, less is known about the amount of nutrients acquired relative to the C allocated belowground, which is referred to as C efficiency for nutrient acquisition (CENA). Here, we examined how C efficiency for nitrogen (N) and phosphorus (P) acquisition varied between ryegrass (Lolium perenne) and clover (Trifolium repens) with and without P fertilization. A continuous 13C-labeling method was applied to track belowground C allocation. Both species allocated nearly half of belowground C to rhizosphere respiration (49%), followed by root biomass (37%), and rhizodeposition (14%). With regard to N and P, CENA was higher for clover than for ryegrass, which remained higher after accounting for relatively low C costs associated with biological N2 fixation. Phosphorus fertilization increased the C efficiency for P acquisition but decreased the C efficiency for N acquisition. A higher CENA for N and P in clover may be attributed to the greater rhizosphere priming on soil organic matter decomposition. Increased P availability with P fertilization could induce lower C allocation for P uptake but exacerbate soil N limitation, thereby making N uptake less C efficient. Overall, our study revealed that species-specific belowground C allocation and nutrient uptake efficiency depend on which nutrient is limited.
Introduction
Belowground carbon (C) allocation by plants is an important driver for plant nutrient acquisition. In a global synthesis, Pausch and Kuzyakov (2018) reported that grassland species allocated on an average of 33% of gross primary productivity belowground to root biomass, rhizosphere respiration, and rhizodeposition. This belowground C is tightly associated with plant nutrient acquisition through various strategies, such as generating fine roots or root hairs, forming symbiotic associations with nitrogen (N)-fixing bacteria or mycorrhizal fungi, or stimulating microbial activity to mobilize nutrients from soil organic matter using root exudates (Lambers et al., 2008; Richardson et al., 2009; Zhu et al., 2014). Modeling studies have indicated that variation in C allocation for nutrient acquisition between N-fixing and non-fixing plants, or between arbuscular and ectomycorrhizal plants, is helpful for understanding their competitive advantages and how this relates to their abundance and productivity (Fisher et al., 2010; Brzostek et al., 2014). However, empirical research on how much total C plants allocate belowground to obtain nutrients is rare.
To assess the C efficiency associated with nutrient acquisition, we introduce a new parameter, i.e., C efficiency for nutrient acquisition (CENA), which we define as the amount of nutrients acquired relative to C allocated belowground (Wang et al., 2022). In most studies, belowground C allocation is primarily based on measures of root production, thus ignoring other C pathways such as allocation to root respiration, root exudates, and symbiotic relationships, which are extremely difficult to quantify (Vicca et al., 2012; Pausch and Kuzyakov, 2018; Keller et al., 2021). This assessment, without considering other C pathways, may underestimate total belowground C allocation, hindering an accurate understanding of CENA. 13C-labeling methods provide us with the opportunity to quantify root respiration, root exudates, and symbiotic microbial respiration, which have been successfully applied in previous studies (Schmitt et al., 2013; Ven et al., 2019). Compared to traditional methods, the isotope tracer method permits us to consider all these belowground C allocation pathways. As rhizosphere respiration and rhizodeposition may account for a large proportion of the total C allocated belowground (Pausch and Kuzyakov, 2018), accounting for all belowground C allocation pathways will be required to accurately estimate CENA.
Belowground C allocation and CENA can vary greatly among plant species due to differences in root architecture and morphological traits, root exudates, mycorrhizal association, and the capacity of biological N2 fixation (de Neergaard and Gorissen, 2004; Schmitt et al., 2013; Keller and Phillips, 2019). For example, legumes allocated more C to rhizosphere respiration compared to grasses, because of the extra energy and C demand for biological N2 fixation by symbiotic rhizobia (Warembourg et al., 2003), while grasses may spend more C on dense fine roots or high rates of rhizodeposition for enhancing nutrient mobilization and uptake from the soil (Schmitt et al., 2013). The interspecific difference in belowground C allocation patterns will trigger different responses in nutrient acquisition, thereby influencing CENA between legumes and grasses.
Soil nutrient availability may also be an important factor influencing belowground C allocation and CENA. Using economic principles, it can be expected that nutrients become more C expensive for plants when their availability is low (Bloom et al., 1985). Most plants are limited by N, phosphorus (P), or both (Harpole et al., 2011; Du et al., 2020), and the amount of C that plants allocate belowground may strongly depend on which nutrient is limiting their growth. Although plant demand for P is lower than for N, belowground C allocation for P uptake may be higher than for N given that soil P availability is usually much lower and less mobile compared to N (Vitousek et al., 2010). Plants secrete carboxylates to liberate inorganic P from mineral surfaces, or produce phosphatase extracellular enzymes to increase P mobilization through hydrolysis, when P availability to plants is limited (Lambers et al., 2008; Richardson et al., 2011; Wen et al., 2019). Furthermore, plants may increase belowground C allocation to support arbuscular mycorrhizal fungi to enhance P uptake under low P conditions (Smith et al., 2011; van der Heijden et al., 2015; Ven et al., 2019). Therefore, plants may allocate more belowground C to root exudates or rhizosphere respiration when plants are limited by P. Due to their capacity to fix N2 from the atmosphere, the growth of legumes is more likely to be P-limited (Png et al., 2017), and belowground C allocation and CENA in legumes may therefore be more sensitive to P availability in soil compared to grasses.
Here, we assessed belowground C allocation and C efficiency for N and P acquisition in ryegrass (Lolium perenne L., C3 grass) and clover (Trifolium repens L., legume) with and without P fertilization based on the same greenhouse experiment in Lu et al. (2020). By continuously labeling plants with CO2 depleted in 13C, we were able to quantify different components of belowground C allocation (root biomass, rhizosphere respiration, and rhizodeposition). We further used a 15N natural abundance method to estimate biological N2 fixation in clover and finally assessed CENA by comparing belowground C allocation to nutrient content in plant biomass after 58 days of growth. The objectives of this study were to (1) compare the difference in C efficiency for N (CENAN) and for P (CENAP) between ryegrass and clover and (2) assess how P fertilization affects CENAN and CENAP in ryegrass and clover. We hypothesized that (1) clover would have a higher C efficiency for N (CENAN) compared to ryegrass because plant N acquisition through biological N2 fixation is usually more C efficient compared to uptake from the soil (Fisher et al., 2010); (2) P fertilization would increase CENAN in clover because P fertilization would increase biological N2 fixation and reduce belowground C allocation associated with P uptake from the soil. However, P fertilization would increase N limitation in ryegrass, lowering CENAN; and (3) C efficiency for P (CENAP) would be lower in clover because biological N2 fixation would cause it to be more limited by P compared to ryegrass; for the same reason, P fertilization would increase CENAP more in clover than in ryegrass.
Materials and Methods
Experimental Design
Top soil (0–15 cm depth) was collected from a grassland at John Bruce Pye Farm in Camden, NSW, Australia (33°56′42″ S, 150°40′30″ E). The soil is a red-brown chromosol (Isbell, 2002) (or Alfisol based on USDA Soil Taxonomy), with a pH of 5.4, 34% sand, 31% silt, and 35% clay. The δ13C of soil organic C was −23.06‰, and the organic C, total N, and total P concentrations were 28.8, 2.5, and 0.15 mg g–1, respectively. The concentrations of soil mineral N (2 M KCl extraction) and available P (0.03 M NH4F and 0.025 M HCl) were 58.0 and 8.7 mg kg–1, respectively. Mesocosms consisted of bottom-capped polyvinyl chloride (PVC) pots (diameter 15 cm, height 20 cm) and sieved (4 mm) grassland soil (equivalent to 3.20 kg of oven-dried soil). After adjusting soil moisture content to 70% water-holding capacity (21% gravimetric soil moisture content), a modified Hoagland solution with macro- and micro-nutrients was added to all mesocosms [(NH4)2SO4 23.8, KNO3 25.7, Ca(NO3)2⋅4H2O 11.9, MgCl2⋅6H2O 16.4, H3BO3 0.08, ZnSO4⋅7H2O 0.2, CuSO4⋅5H2O 0.02, FeSO4⋅7H2O 0.25, and MnCl2⋅4H2O 0.3 g m–2]. The P was applied to the treatment with P as a KH2PO4 and K2HPO4 solution with an adjusted ratio to obtain a similar pH to the soil (4 g of P m–2), while for the treatment without P, a KCl solution was applied to eliminate the introduced K fertilization effect. These mesocosms (with and without P) were planted with either ryegrass (Lolium perenne L.) or clover (Trifolium repens L.) or were left unplanted (control). These two plant species are widely used to improve pastures in many temperate regions of the world. Besides the capacity of fixing N2 from the atmosphere, ryegrass and clover also differ greatly in root morphological and architectural traits (Table 1), mycorrhizal infection (Zhu et al., 2000), and quantity and quality of root exudates (Lu et al., 2020), thereby showing different strategies of carbon allocation and nutrient acquisition. Six treatments were replicated four times. After germinating, each planted mesocosm was thinned to 20 plants.
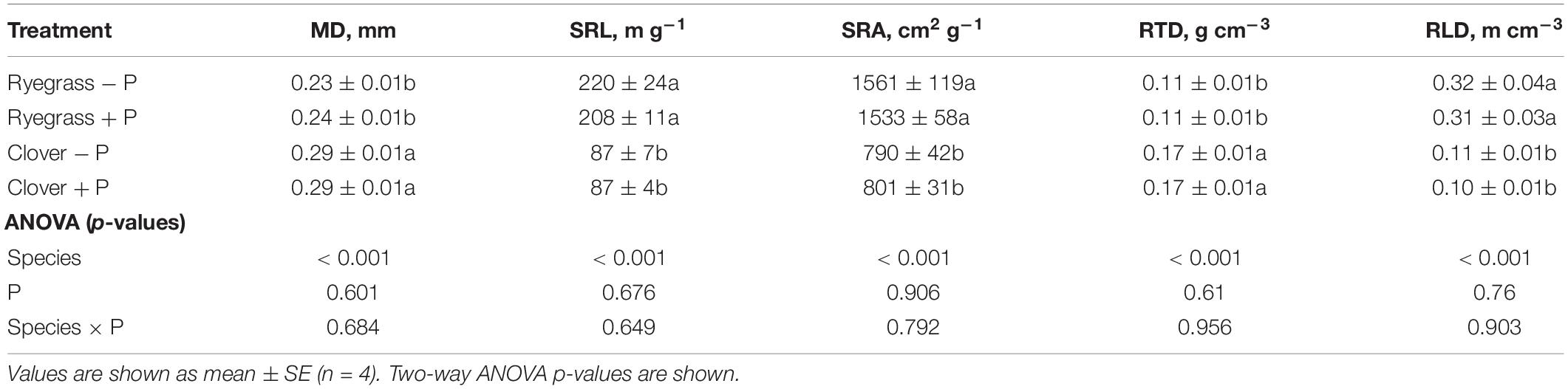
Table 1. Mean diameter (MD), specific root length (SRL), specific root surface area (SRA), root tissue density (RTD), and root length density (RLD) of ryegrass and clover with and without P addition.
This experiment lasted for 58 days in a controlled environmental facility at the Centre for Carbon, Water, and Food, University of Sydney, Camden (NSW). During the experimental period, the air temperature was kept at 25°C during the day and at 15°C during the night. The relative air humidity was kept at 60%, and artificial lights (Heliospectra, LX602C, 600 W, ∼1 mmol m–2 s–1) went on for 12 h every day. The concentration of CO2 was set at 800 ppm by injecting 13C-depleted CO2 into the chamber. This was needed to reduce the δ13C value of CO2 to a desired level and also to promote plants to grow faster, so that the labeled 13C signature could be detected in different pools or fluxes of belowground C allocation during a short cultivation period. Although a high level of CO2 concentration would affect plant photosynthesis and thereby nutrient uptake dynamics, we are not aware that it would cause significant bias when comparing the treatment effects (species and P) under the same CO2 concentration. Moreover, our study provides novel insights into a mechanistic understanding of plant–soil interactions and is relevant given that global atmospheric CO2 concentrations may reach 800 ppm or higher by the end of this century (Collins et al., 2013). We further note that we have used this method successfully in the past (e.g., Dijkstra and Cheng, 2007a; Canarini and Dijkstra, 2015). The δ13C value of CO2 was maintained at −20 ± 0.3‰ (mean ± standard deviation) throughout the experiment (measured on a G2131-i Analyzer, Picarro, Santa Clara, CA, United States). More details about continuous 13C-labeling method were reported in Lu et al. (2020). All mesocosms were randomly placed in the chamber and watered every 2 days to maintain soil moisture content at 70% water-holding capacity. The distribution of these mesocosms was randomly rotated every week to eliminate potential effects caused by spatial differences in light levels within the chamber.
Measurements
Total soil respiration was measured using a gas chamber method on 30, 44, and 58 days after planting (see full details in Lu et al., 2020). Briefly, at each gas sampling time, shoots were clipped at 1 cm above the soil surface and a non-transparent PVC chamber was sealed to each mesocosm. After removing initial CO2 inside the mesocosm and chamber by circulating air inside the mesocosm through a soda lime column, a 12 mL gas sample was taken from the septum of the chamber at 0 h (T0), 1 h (T1), and 2 h (T2), respectively. These gas samples (T0, T1, and T2) were measured for CO2 concentration and δ13C on a Delta V advantage isotope ratio mass spectrometer (IRMS) coupled to a Gasbench (Thermo Fisher Scientific, Bremen, Germany). As plants were continuously labeled with depleted 13C-CO2 (δ13C = −20‰), we were able to separate root-derived CO2 (root respiration and microbial respiration of rhizodeposits) from soil-derived CO2. At the end of the experiment, root samples were carefully picked from the mesocosm and soil samples were homogenized. The clipped shoots at each sampling time, hand-picked roots, and homogenized soil were measured for C%, N%, δ13C, and δ15N on a Delta V advantage IRMS coupled to a Conflo IV and Flash HT (Thermo Fisher Scientific, Bremen, Germany). The plant samples were also measured for P concentration on the UV–VIS spectrophotometer (UVmini-1240), following the protocol described by Jackson (1958).
Calculations
Belowground C allocation includes C for root growth (root biomass C), rhizosphere respiration (root-derived CO2), and rhizodeposition (root-derived SOC). For clover, rhizosphere respiration also includes a C cost for biological N2 fixation. We calculated rhizosphere respiration at each sampling date using a mass balance method based on δ13C signatures of CO2 in planted and unplanted mesocosms (Lu et al., 2020) as follows:
where Ctotal, Csoil, and Croot are total belowground CO2, soil-derived CO2, and root-derived CO2 in planted mesocosms, respectively. δ13Ctotal is the measured δ13C value of total belowground CO2 in planted treatments. δ13Csoil is the mean δ13C value of soil respiration in the unplanted control. δ13Croot is the δ13C value of root-derived CO2 in planted treatments, which was calculated based on the δ13C value of root tissue corrected by a fractionation factor of root-derived CO2 relative to root tissue (−1.74‰ for grass and −2.67‰ for legume; Werth and Kuzyakov, 2010). We calculated the rhizosphere priming effect as the difference in soil-derived CO2 between planted and unplanted control treatments (Lu et al., 2020).
Rhizosphere respiration during the whole 58-day experiment was then calculated using a linear extrapolation method based on the root-derived CO2 at three sampling dates (30, 44, and 58 days after planting). It is noted that clipping before trapping belowground CO2 may cause a decrease in root-derived CO2 (Shahzad et al., 2012). Root-derived CO2 was only measured during day time in this study. Root-derived CO2 at night in wheat was sometimes lower than during the day, but at other times, night-time root-derived CO2 was similar to day-time rhizosphere respiration (Kuzyakov and Cheng, 2001). Furthermore, we noted that the respiration of crowns and a negligible amount of shoot biomass were included in our measured rhizosphere respiration. Therefore, the calculated cumulative rhizosphere respiration during the entire experiment may have been somewhat overestimated, but unfortunately, we were unable to quantify this.
We calculated new root-derived SOC formed during the experiment (Cnew) based on δ13C signatures of soil organic C at the start and end of the experiment in planted mesocosms (Dijkstra and Cheng, 2007b) as follows:
where Cinitial and Cend are the total amount of soil organic C at the beginning and end of the experiment, respectively. δ13Cinitial is the δ13C value of Cinitial, δ13Cend is the δ13C value of Cend, and δ13Croot is the δ13C value of root biomass.
Carbon efficiency for nutrient acquisition was calculated as plant nutrient content divided by belowground C allocation. Plant N and P contents were calculated by multiplying tissue biomass with tissue N and P concentration. Considering the C cost for biological N2 fixation in clover, we calculated CENA associated with belowground C allocation for plant nutrient uptake from the soil only, by subtracting C used for biological N2 fixation from the total belowground C allocation (both for CENAN and CENAP) and subtracting biologically fixed N from the total plant N content (for CENAN) in clover treatments as follows:
where Nplant and Pplant are the N and P contents in the total plant biomass (shoots and roots) after day 58 plus the N and P contents in shoot biomass clipped on day 30 and 44, respectively. Nfix is the biologically fixed N in clover, Cbelow allocation is the total belowground C allocation, and Cbiological N fixation is the C used for biological N2 fixation. Carbon costs associated with biological N2 fixation in legumes are relatively constant, ranging between 8 and 12 g C g–1 fixed N, depending on soil temperature (Fisher et al., 2010). Here, we used a value of 8 g C g–1 fixed N as the C cost for biological N2 fixation in clover at 20°C. Biologically fixed N in clover was calculated using the 15N natural abundance method (Mia et al., 2018) as follows:
where Nclover is the N content in clover tissues, δ15Nryegrass and δ15Nclover are the δ15N values of ryegrass (used as a reference plant) and clover tissues, respectively, and δ15Nbnf is the δ15N value of N-fixing plants completely relying on biological N2 fixation (without N uptake from soil), which was estimated as −1.527‰ for clover (Mia et al., 2018).
Statistical Analyses
Two-way ANOVA was applied to test the main and interactive effects of plant species and P fertilization on root biomass C, rhizosphere respiration, rhizodeposition, belowground C allocation, plant tissue δ15N, biologically fixed N, and CENA. The post hoc Tukey’s honest significant difference (HSD) test was used to compare variables among ryegrass, ryegrass with P fertilization, clover, and clover with P fertilization treatments. Differences at p < 0.05 were considered significant, while differences between p > 0.05 and p < 0.1 were considered marginally significant. All statistical analyses were performed using the SPSS 20.0 (IBM SPSS Statistics 20, Armonk, United States).
Results
Belowground C Allocation
Ryegrass showed larger root biomass C than clover (Figure 1A); clover showed larger rhizosphere respiration than ryegrass (Figure 1B); and the two species did not differ in rhizodeposition (Figure 1C). Due to the contrasting patterns of root biomass and rhizosphere respiration, there was no significant difference in belowground C allocation between the two species (Figure 1D). Both ryegrass and clover allocated more belowground C to rhizosphere respiration (45 and 53%) and less to root biomass (40 and 34%) and rhizodeposition (15 and 13%) (Figure 1). For clover, the C cost for biological N fixation accounted on an average for 45% of rhizosphere respiration and 24% of total belowground C allocation, respectively. When excluding C cost for biological N2 fixation, clover showed lower rhizosphere respiration (Figure 1B) and less belowground C allocation than ryegrass (Figure 1D). P fertilization increased rhizosphere respiration (on an average by 6%; Figure 1B) but did not significantly influence the total belowground C allocation of both species (Figure 1D).
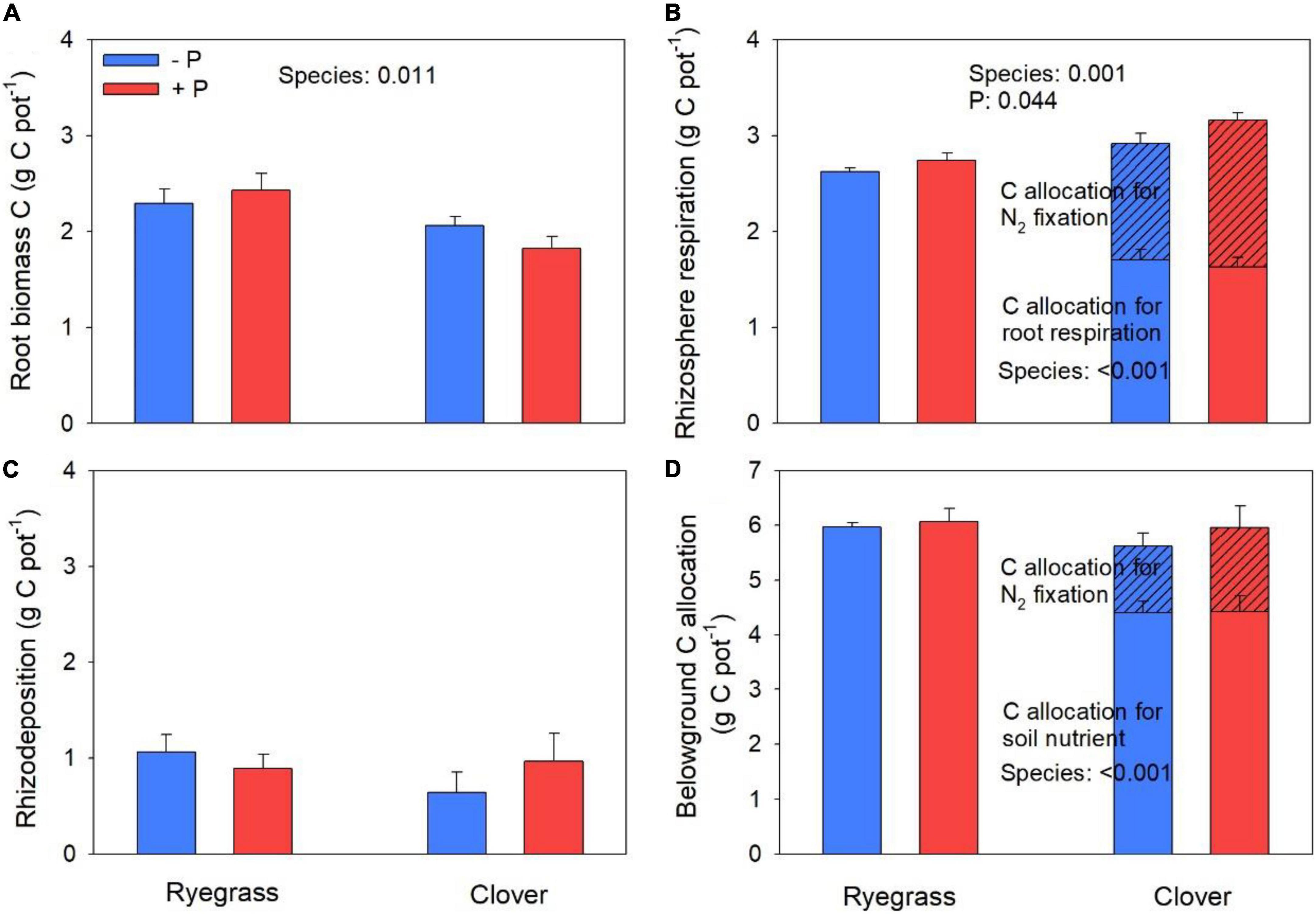
Figure 1. Root biomass C (A), rhizosphere respiration (B), rhizodeposition (C), and total belowground C allocation (D) of ryegrass and clover with and without P addition. For rhizosphere respiration and total belowground C allocation, allocation was separated into C allocation for biological N fixation (shaded bars) and for nutrient uptake from the soil (non-shaded bars). Sub-legend shows ANOVA p-values. Error bar indicates one standard error of the mean (n = 4).
Plant Nutrient Acquisition and Carbon Efficiency for Nutrient Acquisition
Plant N and P contents were reported in Lu et al. (2020). Briefly, clover had higher plant N and P contents than ryegrass (104 and 53% higher, respectively). On average, 37% of plant N in clover was biologically fixed by rhizobia from the atmosphere (Table 2). After subtracting biologically fixed N, non-fixed N in clover was still higher than in ryegrass (28%). Phosphorus fertilization significantly increased plant P content in both species (10%), marginally increased biologically fixed N in clover (27%, Table 2) and significantly decreased non-fixed N in both species (11%). CENAN (including C cost for biological N2 fixation) was higher for clover than for ryegrass (Figure 2A). When excluding C cost for biological N2 fixation, clover still showed a higher CENAN than ryegrass (Figure 2B), while CENAP was also higher for clover (Figure 2C), indicating that clover obtained more N and P from soil with less belowground C. Phosphorus addition increased CENAP (Figure 2C) but decreased CENAN in both species (Figure 2B), although the effects were marginally significant.
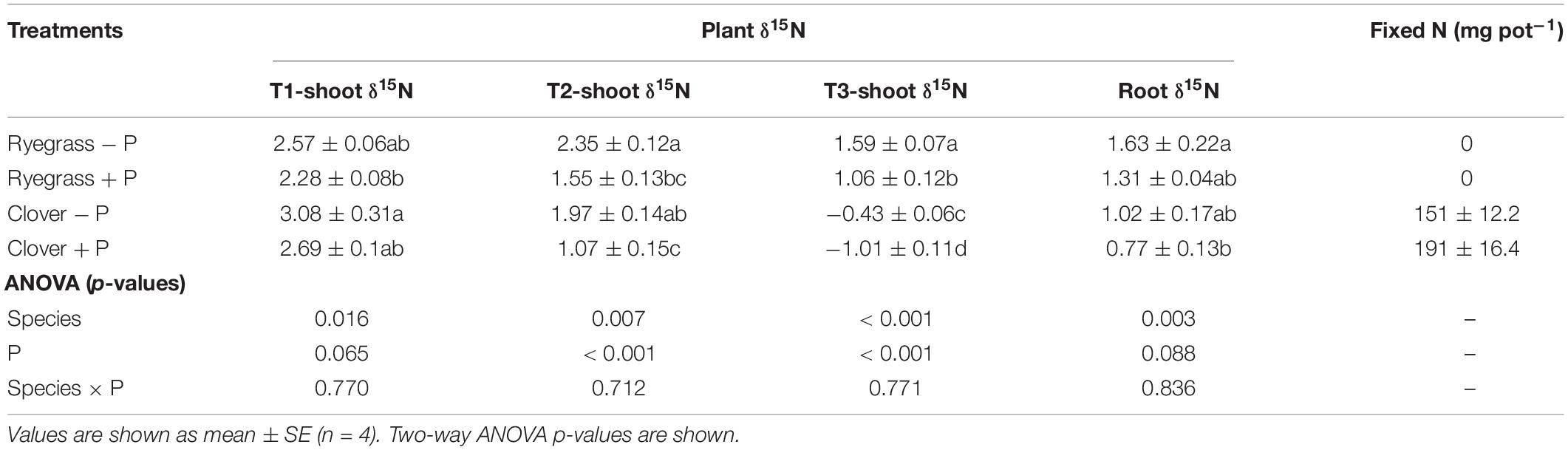
Table 2. Plant δ15N values in shoot and root biomass of ryegrass and clover, and biologically fixed N in clover with and without P fertilization (T1, Day 30; T2, Day 44; T3, Day 58).
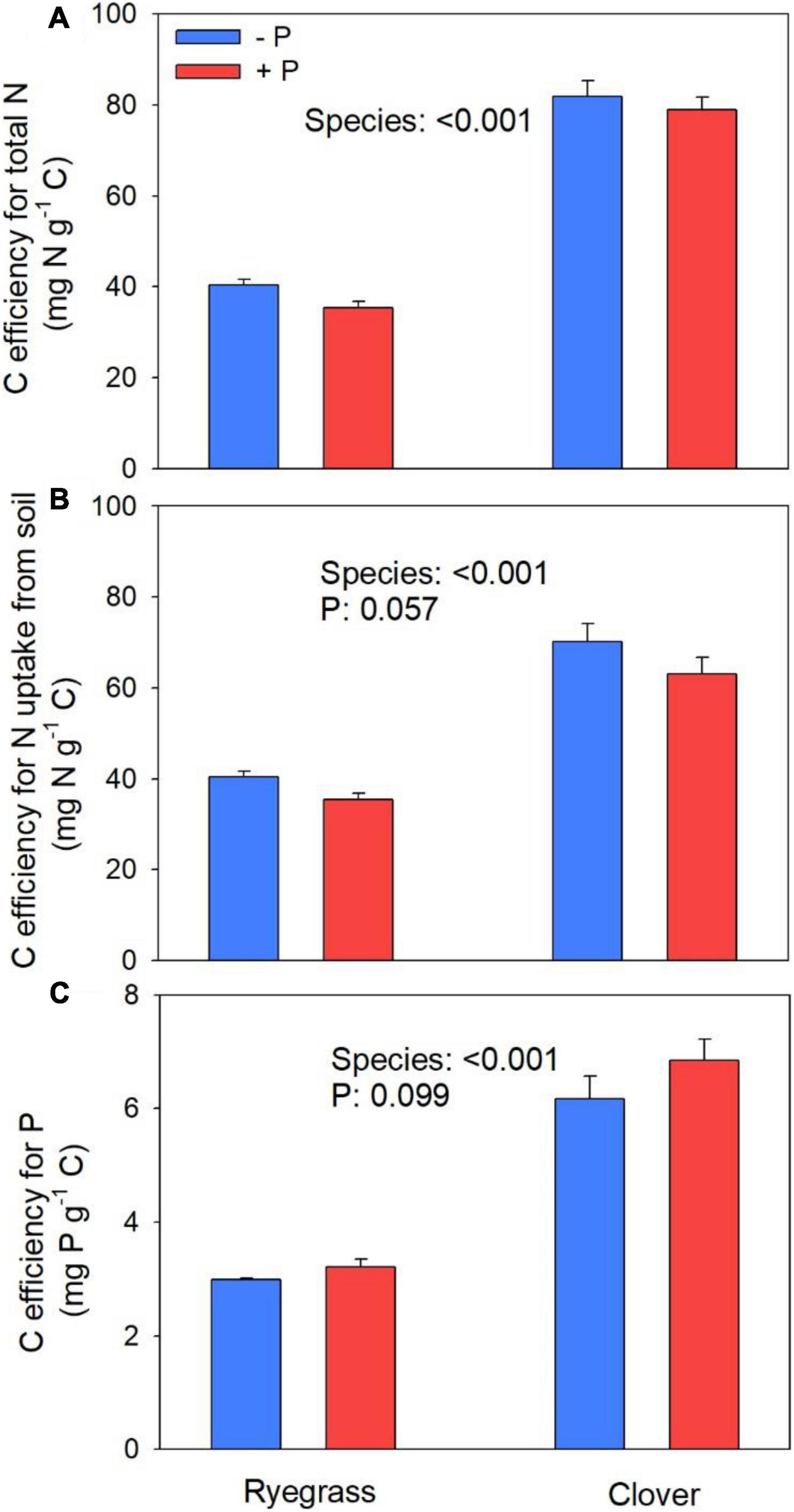
Figure 2. Belowground C efficiency for N acquisition (CENAN) including (A) and excluding (B) biological N2 fixation and associated C allocation, and belowground C efficiency for P acquisition (CENAP) excluding C used for biological N fixation (C) for ryegrass and clover with and without P addition. Sub-legend shows ANOVA p-values. Error bar indicates one standard error of the mean (n = 4).
Discussion
Half of the total belowground C allocation was allocated to rhizosphere respiration (including root respiration and rhizosphere microbial respiration of rhizodeposits), suggesting that autotrophic respiration plays an important role in plant belowground C allocation and the total soil CO2 efflux (Hopkins et al., 2013). Rhizodeposition remaining in soil accounted for the lowest fraction of belowground C allocation (an average of 14%), but actual rhizodeposition rates must be higher considering that most of the rhizodeposition is lost as CO2 via rapid microbial decomposition (Pausch et al., 2013; Pausch and Kuzyakov, 2018; Liu et al., 2019), which in our case was included in rhizosphere respiration. Root biomass accounted for 37% of total belowground C allocation, which was smaller than rhizosphere respiration and rhizodeposition. These results suggest that the assessment of belowground C allocation based only on root biomass is somewhat biased and that rhizosphere respiration and rhizodeposition cannot be ignored. We acknowledge that our estimates of rhizosphere respiration were based on only three flux measurements and that they were done during the day only, and as such have the greatest uncertainty that needs further investigation. Nevertheless, the distribution of belowground C among different pools or fluxes is to some degree consistent with the C allocation patterns for grassland species synthesized by Pausch and Kuzyakov (2018).
Although clover allocated less C to root biomass, it showed greater rhizosphere respiration than ryegrass, possibly because biological N2 fixation by clover requires C. Indeed, when accounting for C allocation toward biological N2 fixation and assuming that this C would eventually be respired as CO2, there was a larger rhizosphere respiration in ryegrass than in clover instead (Figure 1B). Thus, C allocation toward biological N2 fixation was an important component of rhizosphere respiration for clover. Previous studies also suggested that legumes had a higher demand for assimilated C as indicated by higher rhizosphere respiration than grasses (Warembourg et al., 2003; Schmitt et al., 2013). Furthermore, we estimated that the C cost for biological N2 fixation was about 8 g C g–1 N (C cost at 20°C) according to Fisher et al. (2010), while the C allocation for plant N uptake from soil in clover was then 14 g C g–1 N (inverse of CENAN, Figure 2B). This result suggests that biological N2 fixation by the legume is a relatively C efficient way to acquire N as compared to plant N uptake from soil. Even if the C cost for biological N2 fixation is higher than what we assumed (e.g., assuming 10 or 12 g C g–1 fixed N, respectively; Fisher et al., 2010), the C cost for biological N2 fixation would still be cheaper than or similar to that for N uptake from soil in clover (e.g., C allocation for plant N uptake would then be 13.3 or 12.3 g C g–1 N, respectively). Clearly, more work is needed to compare the C cost for biological N2 fixation vs. N uptake. Nevertheless, our results may explain why N2-fixing plants often compete with non-fixing plants, particularly under the condition of low N availability (Vitousek and Howarth, 1991; Crews, 1999; Menge et al., 2017; Wang et al., 2022).
Consistent with our hypothesis, clover had a higher CENAN than ryegrass because, as discussed above, less C was required for biological N2 fixation than for N uptake from soil. However, CENAN of clover was still higher than ryegrass after we accounted for the C cost associated with biological N2 fixation. In contrast to our hypothesis, CENAP was also higher for clover than for ryegrass. Possibly, the greater rhizosphere priming effect on soil organic matter decomposition that we observed for clover by the end of the experiment in another study may contribute to the higher CENAN and CENAP (Lu et al., 2020). By the end of the experiment, available forms of N in soil were extremely low (less than 7 mg N pot–1 or 2 mg N kg–1 soil), and likely very C expensive to take up by both ryegrass and clover. Therefore, stimulation of soil organic matter decomposition by root exudates and subsequent release of N (and P) for plant uptake may be a very C-efficient way for plants to acquire nutrients from the soil (Figure 3; Wang et al., 2022). Previous studies also suggested that legume species could produce larger rhizosphere priming effects than non-legume species (Cheng et al., 2003; Drake et al., 2013). Alternatively, the higher CENAP in clover than in ryegrass may also be attributed to the tendency of legumes to cause greater acidification in the rhizosphere and exude carboxylates to mobilize and increase concentrations of inorganic P through dissolution or desorption (Hinsinger, 2001; Nuruzzaman et al., 2006).
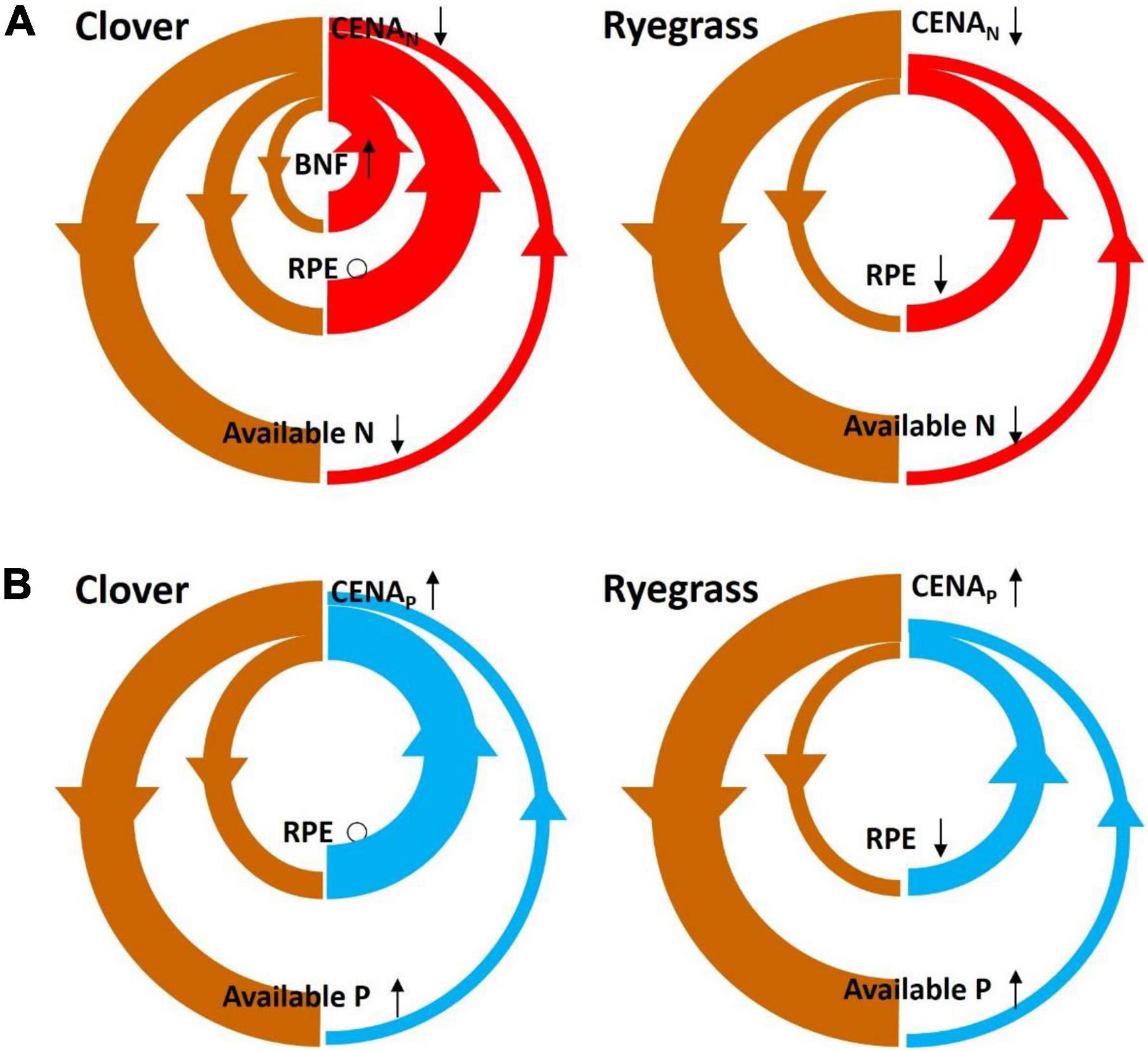
Figure 3. Schematic framework showing how belowground C efficiency for N acquisition (CENAN) (A) and for P acquisition (CENAP) (B) vary with soil P availability for clover and ryegrass. Brown arrows, carbon flows; red arrows, nitrogen flows; and blue arrows, phosphorus flows. Thickness of arrows indicates the relative importance of C, N, and P flows, while differences in thickness between carbon and nutrient flows indicate CENA (i.e., relatively thick carbon arrows compared to nutrient arrows indicate a low CENA and vice versa). BNF, biological N2 fixation; RPE, rhizosphere priming effect. Black arrows and circles next to CENAN, CENAP, BNF, RPE, available N, and available P indicate effect of P fertilization (upward arrow, positive; downward arrow, negative; circle, and no effect). For clover, CENAN decreases with P fertilization because the negative effect of P fertilization on N availability outweighs the positive effect on BNF.
In contrast to our hypothesis, P fertilization slightly decreased the CENAN in ryegrass and clover, suggesting that P fertilization caused these plants to allocate more belowground C to acquire N from soil. In this study, although biological N2 fixation in clover marginally increased with P fertilization (Table 2), the increase of this relatively cheap form of N acquisition was apparently not enough to counter the increase in belowground C allocation for soil N uptake. Possibly, P fertilization may have exacerbated soil N limitation by increasing microbial N immobilization (Blanes et al., 2012; Mehnaz et al., 2019), and thus did not improve belowground C allocation. Indeed, microbial biomass N measured at the end of the experiment was significantly higher with P fertilization (an average of 17%; Lu et al., 2020). In turn, increased microbial N immobilization with P fertilization may thus reduce soil available N, thereby making it more C expensive for plant uptake (Figure 3). It has also been suggested that C allocation could increase to maintain plant N uptake at low N conditions (Brzostek et al., 2014; Perkowski et al., 2021). Our results further imply that plant C allocation belowground for N uptake depends on P availability.
Phosphorous fertilization marginally increased the CENAP for both species, indicating that the P uptake may become somewhat less C expensive with increased soil P availability (Figure 3). This result is consistent with the resource optimization hypothesis that plants allocate more C to acquire limited resources (McMurtrie and Dewar, 2013). Previous studies also found that belowground C investment in P acquisition was more efficient with P fertilization (Ven et al., 2019). We expected that CENAP would increase more for clover than for ryegrass given that growth of the N-fixing clover would be more limited by P than ryegrass (Png et al., 2017). However, we found no support for this (no significant species × P interaction), but we should note that the increases in CENAP with P fertilization were relatively small and only marginally significant. Clearly, more work is needed for an in-depth understanding of the mechanisms underlying the CENAN and CENAP of grasses and legumes under different N and P availabilities.
While we did not investigate interspecific interactions on CENA, the CENA concept may have significant implications for plant community dynamics and competition for nutrients in legume-grass mixtures (Raven et al., 2018; Wang et al., 2022). When legumes have a higher CENA compared to grasses, they may temporarily outcompete grasses for soil nutrients until conditions arise that reduce CENA for legumes more than for grasses (e.g., under drought or high levels of soil N). Changes in CENA with time would also depend on how flexible plants are in switching C allocation toward different nutrient acquisition strategies (Fisher et al., 2010). Furthermore, interspecific competition for nutrients by itself, as well as the transfer of biologically fixed N from legume to grass, could potentially influence the CENA of individual species in grassland mixtures, which could help explain the often observed temporal dynamics in legume and grass abundance in mixed pastures (Ledgard and Steele, 1992). Further research is therefore needed to investigate the role of CENA in plant community dynamics in legume-grass mixtures.
Conclusion
In our study, we quantified the belowground C allocation and its efficiency for N and P acquisition (CENAN and CENAP, respectively). We showed that clover had higher CENAN and CENAP than ryegrass, even after accounting for the relatively low C costs associated with biological N2 fixation, possibly because of the distinct rhizosphere priming on soil organic matter decomposition. Furthermore, P fertilization decreased CENAN, possibly via exacerbating soil N limitation, while P fertilization increased CENAP because plant P acquisition was more efficient with increased P availability. Current modeling studies have indicated that net primary productivity and soil C storage are strongly associated with variation in the belowground C allocation for nutrient uptake (Fisher et al., 2010; Brzostek et al., 2014; Shi et al., 2016). Yet, estimates of CENAN and CENAP are lacking. To the best of our knowledge, this is one of the first studies to provide estimates for these parameters. We acknowledge that our study is based on two plant species only and in one soil type only, and that variation in CENA should be examined across a larger set of plant species, communities, and soil types during a relatively long period. Nevertheless, we believe that better understanding of CENAN and CENAP will help not only improve global C cycling model predictions but also identify management practices to increase yield and fertilizer use efficiency in agricultural systems.
Data Availability Statement
The original contributions presented in this study are included in the article/supplementary material, further inquiries can be directed to the corresponding author.
Author Contributions
JL and FD designed the experiment. JL, JY, and CK performed the experiment. JL analyzed the data. JL, LY, PW, WC, and FD wrote the manuscript. All authors contributed to the article and approved the submitted version.
Funding
This study was supported by the Australian Research Council (DP190102262), the National Natural Science Foundation of China (32001386), and a visiting scholarship to JL from the Joint Ph.D. Training Program by the University of Chinese Academy of Sciences.
Conflict of Interest
The authors declare that the research was conducted in the absence of any commercial or financial relationships that could be construed as a potential conflict of interest.
Publisher’s Note
All claims expressed in this article are solely those of the authors and do not necessarily represent those of their affiliated organizations, or those of the publisher, the editors and the reviewers. Any product that may be evaluated in this article, or claim that may be made by its manufacturer, is not guaranteed or endorsed by the publisher.
Acknowledgments
We thank Milad Bagheri Shirvan for laboratory assistance.
References
Blanes, M. C., Emmett, B. A., Viñegla, B., and Carreira, J. A. (2012). Alleviation of P limitation makes tree roots competitive for N against microbes in a N-saturated conifer forest: a test through P fertilization and 15N labelling. Soil Biol. Biochem. 48, 51–59. doi: 10.1016/j.soilbio.2012.01.012
Bloom, A. J., Chapin, F. S., and Mooney, H. A. (1985). Resource limitation in plants-An economic analogy. Annu. Rev. Ecol. Syst. 16, 363–392. doi: 10.1146/annurev.es.16.110185.002051
Brzostek, E. R., Fisher, J. B., and Phillips, R. P. (2014). Modeling the carbon cost of plant nitrogen acquisition: mycorrhizal trade-offs and multipath resistance uptake improve predictions of retranslocation. J. Geophys. Res. Biogeosci. 119, 1684–1697. doi: 10.1002/2014jg002660
Canarini, A., and Dijkstra, F. A. (2015). Dry-rewetting cycles regulate wheat carbon rhizodeposition, stabilization and nitrogen cycling. Soil Biol. Biochem. 81, 195–203. doi: 10.1016/j.soilbio.2014.11.014
Cheng, W., Johnson, D. W., and Fu, S. (2003). Rhizosphere effects on dcomposition: control of plant species, phenology, and fertilization. Soil Sci. Soc. Am. J. 67, 1418–1427. doi: 10.2136/sssaj2003.1418
Collins, M., Knutti, R., Arblaster, J., Dufresne, J.-L., Fichefet, T., Friedlingstein, P., et al. (2013). “Long-term Climate Change: Projections, Commitments and Irreversibility,” in Climate Change 2013: The Physical Science Basis. Contribution of Working Group I to the Fifth Assessment Report of the Intergovernmental Panel on Climate Change, eds T. F. Stocker, D. Qin, G.-K. Plattner, M. Tignor, S. K. Allen, J. Boschung, et al. (Cambridge: Cambridge University Press).
Crews, T. E. (1999). The presence of nitrogen fixing legumes in terrestrial communities: evolutionary vs ecological considerations. Biogeochemistry 46, 233–246. doi: 10.1007/BF01007581
de Neergaard, A., and Gorissen, A. (2004). Carbon allocation to roots, rhizodeposits and soil after pulse labelling: a comparison of white clover (Trifolium repens L.) and perennial ryegrass (Lolium perenne L.). Biol. Fertil. Soils 39, 228–234. doi: 10.1007/s00374-003-0699-x
Dijkstra, F. A., and Cheng, W. (2007a). Moisture modulates rhizosphere effects on C decomposition in two different soil types. Soil Biol. Biochem. 39, 2264–2274. doi: 10.1016/j.soilbio.2007.03.026
Dijkstra, F. A., and Cheng, W. (2007b). Interactions between soil and tree roots accelerate long-term soil carbon decomposition. Ecol. Lett. 10, 1046–1053. doi: 10.1111/j.1461-0248.2007.01095.x
Drake, J. E., Darby, B. A., Giasson, M. A., Kramer, M. A., Phillips, R. P., and Finzi, A. C. (2013). Stoichiometry constrains microbial response to root exudation-insights from a model and a field experiment in a temperate forest. Biogeosci 10, 821–838. doi: 10.5194/bg-10-821-2013
Du, E., Terrer, C., Pellegrini, A. F. A., Ahlstrom, A., van Lissa, C. J., Zhao, X., et al. (2020). Global patterns of terrestrial nitrogen and phosphorus limitation. Nat. Geosci. 13, 221–226. doi: 10.1038/s41561-019-0530-4
Fisher, J. B., Sitch, S., Malhi, Y., Fisher, R. A., Huntingford, C., and Tan, S. Y. (2010). Carbon cost of plant nitrogen acquisition: a mechanistic, globally applicable model of plant nitrogen uptake, retranslocation, and fixation. Glob. Biogeochem. Cycles 24:GB1014. doi: 10.1029/2009gb003621
Harpole, W. S., Ngai, J. T., Cleland, E. E., Seabloom, E. W., Borer, E. T., Bracken, M. E. S., et al. (2011). Nutrient co-limitation of primary producer communities. Ecol. Lett. 14, 852–862. doi: 10.1111/j.1461-0248.2011.01651.x
Hinsinger, P. (2001). Bioavailability of soil inorganic P in the rhizosphere as affected by root-induced chemical changes: a review. Plant Soil 237, 173–195.
Hopkins, F., Gonzalez-Meler, M. A., Flower, C. E., Lynch, D. J., Czimczik, C., Tang, J., et al. (2013). Ecosystem-level controls on root-rhizosphere respiration. N. Phytol. 199, 339–351. doi: 10.1111/nph.12271
Keller, A. B., Brzostek, E. R., Craig, M. E., Fisher, J. B., and Phillips, R. P. (2021). Root-derived inputs are major contributors to soil carbon in temperate forests, but vary by mycorrhizal type. Ecol. Lett. 24, 626–635. doi: 10.1111/ele.13651
Keller, A. B., and Phillips, R. P. (2019). Relationship between belowground carbon allocation and nitrogen uptake in saplings varies by plant mycorrhizal type. Front. Forests Glob. Chang. 2:81. doi: 10.3389/ffgc.2019.00081
Kuzyakov, Y., and Cheng, W. (2001). Photosynthesis controls of rhizosphere respiration and organic matter decomposition. Soil Biol. Biochem. 33, 1915–1925. doi: 10.1016/S0038-0717(01)00117-1
Lambers, H., Raven, J. A., Shaver, G. R., and Smith, S. E. (2008). Plant nutrient-acquisition strategies change with soil age. Trends Ecol. Evol. 23, 95–103. doi: 10.1016/j.tree.2007.10.008
Ledgard, S. F., and Steele, K. W. (1992). Biological nitrogen fixation in mixed legume/grass pastures. Plant Soil 141, 137–153. doi: 10.1007/BF00011314
Liu, Y., Ge, T., Zhu, Z., Liu, S., Luo, Y., Li, Y., et al. (2019). Carbon input and allocation by rice into paddy soils: a review. Soil Biol. Biochem. 133, 97–107. doi: 10.1016/j.soilbio.2019.02.019
Lu, J., Yang, J., Keitel, C., Yin, L., Wang, P., Cheng, W., et al. (2020). Rhizosphere priming effects of Lolium perenne and Trifolium repens depend on phosphorus fertilization and biological nitrogen fixation. Soil Biol. Biochem. 150:108005. doi: 10.1016/j.soilbio.2020.108005
McMurtrie, R. E., and Dewar, R. C. (2013). New insights into carbon allocation by trees from the hypothesis that annual wood production is maximized. N. Phytol. 199, 981–990. doi: 10.1111/nph.12344
Mehnaz, K. R., Corneo, P. E., Keitel, C., and Dijkstra, F. A. (2019). Carbon and phosphorus addition effects on microbial carbon use efficiency, soil organic matter priming, gross nitrogen mineralization and nitrous oxide emission from soil. Soil Biol. Biochem. 134, 175–186. doi: 10.1016/j.soilbio.2019.04.003
Menge, D. N. L., Batterman, S. A., Liao, W., Taylor, B. N., Lichstein, J. W., and Ángeles-Pérez, G. (2017). Nitrogen-fixing tree abundance in higher-latitude North America is not constrained by diversity. Ecol. Lett. 20, 842–851. doi: 10.1111/ele.12778
Mia, S., Dijkstra, F. A., and Singh, B. (2018). Enhanced biological nitrogen fixation and competitive advantage of legumes in mixed pastures diminish with biochar aging. Plant Soil 424, 639–651. doi: 10.1007/s11104-018-3562-4
Nuruzzaman, M., Lambers, H., Bolland, M. D. A., and Veneklaas, E. J. (2006). Distribution of carboxylates and acid phosphatase and depletion of different phosphorus fractions in the rhizosphere of a cereal and three grain legumes. Plant Soil 281, 109–120. doi: 10.1007/s11104-005-3936-2
Pausch, J., and Kuzyakov, Y. (2018). Carbon input by roots into the soil: quantification of rhizodeposition from root to ecosystem scale. Glob. Change Biol. 24, 1–12. doi: 10.1111/gcb.13850
Pausch, J., Tian, J., Riederer, M., and Kuzyakov, Y. (2013). Estimation of rhizodeposition at field scale: upscaling of a 14C labeling study. Plant Soil 364, 273–285. doi: 10.1007/s11104-012-1363-8
Perkowski, E. A., Waring, E. F., and Smith, N. G. (2021). Root mass carbon costs to acquire nitrogen are determined by nitrogen and light availability in two species with different nitrogen acquisition strategies. J. Exp. Bot. 72, 5766–5776. doi: 10.1093/jxb/erab253
Png, G. K., Turner, B. L., Albornoz, F. E., Hayes, P. E., Lambers, H., and Laliberte, E. (2017). Greater root phosphatase activity in nitrogen-fixing rhizobial but not actinorhizal plants with declining phosphorus availability. J. Ecol. 105, 1246–1255. doi: 10.1111/1365-2745.12758
Raven, J. A., Lambers, H., Smith, S. E., and Westoby, M. (2018). Costs of acquiring phosphorus by vascular land plants: patterns and implications for plant coexistence. N. Phytol. 217, 1420–1427. doi: 10.1111/nph.14967
Richardson, A. E., Barea, J. M., McNeill, A. M., and Prigent-Combaret, C. (2009). Acquisition of phosphorus and nitrogen in the rhizosphere and plant growth promotion by microorganisms. Plant Soil 321, 305–339. doi: 10.1007/s11104-009-9895-2
Richardson, A. E., Lynch, J. P., Ryan, P. R., Delhaize, E., Smith, F. A., Smith, S. E., et al. (2011). Plant and microbial strategies to improve the phosphorus efficiency of agriculture. Plant Soil 349, 121–156. doi: 10.1007/s11104-011-0950-4
Schmitt, A., Pausch, J., and Kuzyakov, Y. (2013). C and N allocation in soil under ryegrass and alfalfa estimated by 13C and 15N labelling. Plant Soil 368, 581–590. doi: 10.1007/s11104-012-1536-5
Shahzad, T., Chenu, C., Repinçay, C., Mougin, C., Ollier, J., and Fontaine, S. (2012). Plant clipping decelerates the mineralization of recalcitrant soil organic matter under multiple grassland species. Soil Biol. Biochem. 51, 73–80. doi: 10.1016/j.soilbio.2012.04.014
Shi, M., Fisher, J. B., Brzostek, E. R., and Phillips, R. P. (2016). Carbon cost of plant nitrogen acquisition: global carbon cycle impact from an improved plant nitrogen cycle in the Community Land Model. Glob. Change Biol. 22, 1299–1314. doi: 10.1111/gcb.13131
Smith, S. E., Jakobsen, I., Gronlund, M., and Smith, F. A. (2011). Roles of arbuscular mycorrhizas in plant phosphorus nutrition: interactions between pathways of phosphorus uptake in arbuscular mycorrhizal roots have important implications for understanding and manipulating plant phosphorus acquisition. Plant Physiol. 156, 1050–1057. doi: 10.1104/pp.111.174581
van der Heijden, M. G. A., Martin, F. M., Selosse, M. A., and Sanders, I. R. (2015). Mycorrhizal ecology and evolution: the past, the present, and the future. N. Phytol. 205, 1406–1423. doi: 10.1111/nph.13288
Ven, A., Verlinden, M. S., Verbruggen, E., and Vicca, S. (2019). Experimental evidence that phosphorus fertilization and arbuscular mycorrhizal symbiosis can reduce the carbon cost of phosphorus uptake. Funct. Ecol. 33, 2215–2225. doi: 10.1111/1365-2435.13452
Vicca, S., Luyssaert, S., Peñuelas, J., Campioli, M., Chapin, F. S., Ciais, P., et al. (2012). Fertile forests produce biomass more efficiently. Ecol. lett. 15, 520–526. doi: 10.1111/j.1461-0248.2012.01775.x
Vitousek, P. M., and Howarth, R. W. (1991). Nitrogen limitation on land and in the sea: how can it occur? Biogeochemistry 13, 87–115. doi: 10.1007/BF00002772
Vitousek, P. M., Porder, S., Houlton, B. Z., and Chadwick, O. A. (2010). Terrestrial phosphorus limitation: mechanisms, implications, and nitrogen-phosphorus interactions. Ecol. Appl. 20, 5–15. doi: 10.1890/08-0127.1
Wang, R., Lu, J., Jiang, Y., and Dijkstra, F. A. (2022). Carbon efficiency for nutrient acquisition (CENA) by plants: role of nutrient availability and microbial symbionts. Plant Soil doi: 10.1007/s11104-022-05347-y
Warembourg, F. R., Roumet, C., and Lafont, F. (2003). Differences in rhizosphere carbon-partitioning among plant species of different families. Plant Soil 256, 347–357. doi: 10.1023/a:1026147622800
Wen, Z., Li, H., Shen, Q., Tang, X., Xiong, C., Li, H., et al. (2019). Tradeoffs among root morphology, exudation and mycorrhizal symbioses for phosphorus-acquisition strategies of 16 crop species. N. Phytol. 223, 882–895. doi: 10.1111/nph.15833
Werth, M., and Kuzyakov, Y. (2010). 13C fractionation at the root-microorganisms-soil interface: a review and outlook for partitioning studies. Soil Biol. Biochem. 42, 1372–1384. doi: 10.1016/j.soilbio.2010.04.009
Zhu, B., Gutknecht, J. L. M., Herman, D. J., Keck, D. C., Firestone, M. K., and Cheng, W. (2014). Rhizosphere priming effects on soil carbon and nitrogen mineralization. Soil Biol. Biochem. 76, 183–192. doi: 10.1016/j.soilbio.2014.04033
Keywords: belowground carbon allocation, biological nitrogen fixation, carbon allocation for nutrient uptake, 13C-labeling, rhizosphere priming effect
Citation: Lu J, Yang J, Keitel C, Yin L, Wang P, Cheng W and Dijkstra FA (2022) Belowground Carbon Efficiency for Nitrogen and Phosphorus Acquisition Varies Between Lolium perenne and Trifolium repens and Depends on Phosphorus Fertilization. Front. Plant Sci. 13:927435. doi: 10.3389/fpls.2022.927435
Received: 24 April 2022; Accepted: 03 June 2022;
Published: 24 June 2022.
Edited by:
Shaojun Qiu, Institute of Agricultural Resources and Regional Planning (CAAS), ChinaReviewed by:
Honghui Wu, Institute of Agricultural Resources and Regional Planning (CAAS), ChinaJuanjuan Fu, Northwest A&F University, China
Copyright © 2022 Lu, Yang, Keitel, Yin, Wang, Cheng and Dijkstra. This is an open-access article distributed under the terms of the Creative Commons Attribution License (CC BY). The use, distribution or reproduction in other forums is permitted, provided the original author(s) and the copyright owner(s) are credited and that the original publication in this journal is cited, in accordance with accepted academic practice. No use, distribution or reproduction is permitted which does not comply with these terms.
*Correspondence: Peng Wang, d2FuZ3BlbmdAaWFlLmFjLmNu