- 1School of Agriculture, Jilin Agricultural Science and Technology College, Jilin, China
- 2College of Horticulture, Shenyang Agricultural University, Shenyang, China
- 3School of Agriculture, Yunnan University, Kunming, China
Orphan genes (OGs) are defined as genes having no sequence similarity with genes present in other lineages. OGs have been regarded to play a key role in the development of lineage-specific adaptations and can also serve as a constant source of evolutionary novelty. These genes have often been found related to various stress responses, species-specific traits, special expression regulation, and also participate in primary substance metabolism. The advancement in sequencing tools and genome analysis methods has made the identification and characterization of OGs comparatively easier. In the study of OG functions in plants, significant progress has been made. We review recent advances in the fast evolving characteristics, expression modulation, and functional analysis of OGs with a focus on their role in plant biology. We also emphasize current challenges, adoptable strategies and discuss possible future directions of functional study of OGs.
Introduction
Orphan genes (OGs) also known as taxonomically restricted genes (TRGs), more commonly referred to as ORFan genes are the sequences with no significant sequence’s similarity to any other ORF in a specified database. These are the genes that display no substantial sequence similarity outside their species and are unique to a particular taxonomic group (Siew and Fischer, 2003; Yin and Fischer, 2008; Ekstrom and Yin, 2016; Chen et al., 2020; Vakirlis et al., 2020; Weisman et al., 2020). Most OGs may evolve quickly enough that their similarity to other genes is lost after a certain evolutionary distance (Cai et al., 2006). The development of such genes may occur as a result of rearrangement and duplication processes followed by a rapid divergence event. However, the de novo model of evolution from non-coding genomic areas has also been considered as one of many possible mechanisms, appears more common than previously thought, and de novo gene birth provides an important mechanism for functional evolution (Khalturin et al., 2009; Toll-Riera et al., 2009; Tautz and Domazet-Lošo, 2011; Carvunis et al., 2012; Mahalak and Chamberlin, 2015; Schlötterer, 2015). Phylogenetically, the OGs have been found associated with lineage-specific characteristics, adaptations, and biological functions, and OGs have been reported to be involved in stress response, development of species-specific traits and primary substance metabolism (Wissler et al., 2013; Arendsee et al., 2014; Mukherjee et al., 2015; Jiang et al., 2018).
With the release of numerous plant genomes and transcriptomes data makes it easy to identify the OGs in a particular taxon. The BLAST (Basic Local Alignment Search Tool) algorithm is widely used in the screening and identification of OGs (Graham et al., 2004; Prabh and Rödelsperger, 2019). Studies have revealed that every species have been known to possess a considerable fraction of OGs (up to 30%) of the gene catalog in all sequenced genomes (Arendsee et al., 2014; Palmieri et al., 2014). It has been noted that the formation of OGs mostly occurs due to poorly assembled genomes that have been partially annotated by gene predictions (Prabh and Rödelsperger, 2016). Hence, the assembled genome’s quality and integrity are crucial for precise OG identification. Several studies have been conducted for the identification and characterization of the OGs in plants (including plants such as Brassica rapa, Arabidopsis thaliana, Oryza sativa, Populus trichocarpa, Vigna unguiculata, and Citrus sinensis) (Campbell et al., 2007; Yang et al., 2009; Lin et al., 2010; Donoghue et al., 2011; Xu et al., 2015; Jiang et al., 2018). Nonetheless, as compared to evolutionarily conserved genes, OGs are shorter, evolve faster, and have lower and more tissue-specific expression (Cai and Petrov, 2010; Palmieri et al., 2014; Li T. P. et al., 2021). In the absence of functional motifs, identifiable folds, and recognizable domains, the functional characterization of OGs becomes extremely mysterious.
This review mainly focuses on four segments, the first segment includes the identification and fast evolving characteristics of OGs in plants. The second segment covers the special expression regulatory mode of OGs, and the third segment covers the mysterious functions of OGs. Moreover, the study also entails a detailed description of current challenges, adoptable strategies and possible future directions of functional study of OGs.
Orphan Genes Identification and Its Fast Evolving Characteristics
Identification of Orphan Genes in Plants
Recent technological advancements have resulted in tremendous growth in genomics, with a vast number of genes being annotated. The rapid accumulation of genomic data on a large variety of plants will allow increasing integrity of identification of OGs. So far the presence of OGs has been reported in many plants or lineages [such as B. rapa (Jiang et al., 2018), A. thaliana (Yang et al., 2009; Lin et al., 2010; Donoghue et al., 2011; Cui et al., 2015), O. sativa (Guo et al., 2007; Yang et al., 2009; Cui et al., 2015; Jin et al., 2019), P. trichocarpa (Yang et al., 2009), V. unguiculata (Li G. et al., 2019), Poaceae (Campbell et al., 2007), Aegiceras corniculatum (Ma et al., 2021), C. sinensis (Xu et al., 2015), Amaranthus hypochondriacus (Cabrales-Orona and Délano-Frier, 2021), Camellia sinensis (Zhao and Ma, 2021), and eight Cucurbitaceae family members (Ma et al., 2022)]. Every sequenced genome contains OGs whose origins are obscure because of the absence of homologs (Blanco-Melo et al., 2016).
In various lineages or species, the percentage of genes that are OGs varies greatly, making up less than 1 to 17 percent of all genes in a genome, with 1–5% being typically normal (Table 1). The discrepancy in such a case can be attributed to the ongoing sequencing of multiple genomes, as well as the variable evolutionary distance among the species taken into consideration and their nearest sequenced relatives (Arendsee et al., 2014; Jiang M. et al., 2020). Similarly, multiple versions of the reference genome may lead to a distinct number of OGs, as in the case of the identification of Arabidopsis (Yang et al., 2009; Donoghue et al., 2011), and Populus (Yang et al., 2009; Lin et al., 2013).
In addition, the E-value cutoff is a major contributing factor to the determination of OGs. As in the case of the OGs discovered in the Arabidopsis genome, various numbers of OGs were present under distinct E-value cutoff (Table 1), with certain parameters (i.e., larger the E-value, the lesser are the number of genes obtained and vice-versa). Similarly, under the E-value cutoff of 1E-01 and 1E-04, respectively, 638 Oryza-specific genes and 1,926 OGs were identified in rice. However, the final numbers of OGs were different when the identification methods or programs were modified. Taking into consideration the rice genome, in which 37 OGs were obtained under BLAST and BLAT (BLAST-Like Alignment Tool) programs (Jin et al., 2019). Other effective modules or programs include the SMOTE-ENN-XGBoost model (Synthetic Minority Over-sampling TEchnique-Edited Nearest Neighbors-eXtreme Gradient Boosting) (Gao et al., 2020), BIND (BRAK-ER-Inferred Directly), and MIND (MAKER-Inferred Directly) platforms (Li J. et al., 2021), ORFanFinder (Ekstrom and Yin, 2016), combined BLAST and Microarray-based genome hybridization methods (Li G. et al., 2019).
By comparing the available ESTs (Expressed Sequence Tags) and ETs (Expressed Transcripts) from six Solanaceae species on a genomic scale, Solanaceae-specific transcripts in potato, tomato, pepper, tobacco, petunia, and Nicotiana benthamiana, were found representing 4,825, 3,151, 1,531, 8,076, 512, and 571 genes, respectively, using an E-value cutoff of 1E-10 and the TBLASTX (Rensink et al., 2005). TOGD, a database of OGs found in Triticum aestivum, was established, and 993 OGs were identified as a part of the wheat genome using homology searching against 94 representative plant species, contributing to a complete bioinformatics platform for functional and evolutionary investigations (Gao et al., 2019). Moreover, a comparative database of pangenomes was developed by the name of GreenPhylDB (version 5) for plant genomes that provides orphan gene clusters of 46 species across the plant kingdom using a phylogenetic-based approach, including plants such as Solanum tuberosum, Vitis vinifera, Solanum lycopersicum, and Helianthus annuus (Valentin et al., 2021). In addition, ATTED-II is a plant species co-expression database that provides comprehensive information on different co-expression data sets and network analysis tools, as well as a platform for new opportunities to study plant lineage-specific evolution (Aoki et al., 2016).
Furthermore, each gene has been allocated to a phylostratum through the BLAST-based phylostratigraphic approach, which represents the gene’s ancestral evolutionary node (Domazet-Lošo et al., 2007). Following the same approach, the origins of protein-coding genes in A. thaliana and rice have been traced, and several young genes have been discovered in both species (Cui et al., 2015). Based on protein sequence clustering, studies comparing non-family (NF) genes in 14 plant species have discovered over 94,000 such genes across these species, which have been classified into five main evolutionary groups (i.e., Viridiplantae wide, angiosperm specific, monocot specific, dicot specific, species-specific), highlighting the evolutionary and functional role of NF genes across species (Ye et al., 2013). However, phylostratigraphy underestimates gene age with a non-negligible probability and the false negative error is prevalent in phylostratigraphy, which can be controlled at least partially by the exclusion of error-prone genes identified via realistic simulations (Moyers and Zhang, 2018). Although the percentage of OGs in a given species is relatively low, the understanding of OGs continues to increase gradually (Wilson et al., 2005).
Fast Evolving Characteristics of Orphan Genes
On a phylogenetic level, OGs have been found to possess a faster rate of evolution in comparison with the evolutionary-conserved genes (ECGs). Considering the set of OGs present in a given species or lineages tends to share no sequence similarities with any other lineages. Hence, being a signature characteristic of a particular species. Likewise, on a molecular level OGs have been shown to possess a shorter gene or protein length, unusual GC content among coding genes (CDS) with a greater number of intron-less genes while a relatively lower percentage of multi-exon genes upon comparison with ECGs set (Campbell et al., 2007; Lin et al., 2010, 2013; Varshney et al., 2011; Xu et al., 2015; Plissonneau et al., 2016; Jiang et al., 2018). Furthermore, structural traits related to the process of origin and evolutionary time has revealed that OGs have a shorter time of origin than ECGs. Younger genes are shorter, higher in the non-synonymous substitution rate (Guo, 2013). Study showed that the elevated ratios of non-synonymous to synonymous SNPs (Single Nucleotide Polymorphisms) in the two sets of Arabidopsis OGs are mainly due to the elevated non-synonymous SNP density, indicating that a number of two sets of OGs evolve substantially faster than the ECGs at the protein sequence level (Lin et al., 2010). For example, due to their short evolutionary time, the genes generated in this time frame have shorter gene lengths (Ma et al., 2021). However, the GC content of CDS, genes, and introns are more variable in diverse species.
According to studies, most variations in GC content, are the consequence of a confluence of factors, including the organism’s living environment and behaviors (Ma et al., 2020). Genetic analysis of Triticeae-specific genes (TSGs) has shown that nearly one-third of the OGs are intron-less and over half of BSGs (Brassica-specific genes) are intron-less genes, respectively (Jiang et al., 2018; Ma et al., 2020). During evolution, the occurrence of intron-less genes can be associated with lineage-specific or species-specific characteristics within a given species, and OGs may be regarded as the prime factors for the existence of biodiversity (Zou et al., 2011). Similarly, ORFans restricted to the Phaseoleae family in pigeon pea tend to possess many of the same properties as ORFans found in other species, such as short length, few introns, and unique GC content (Varshney et al., 2011). Thus, all evidence indicates that OGs tend to possess more evolutionarily radical characteristics than ECGs. Several studies have revealed that the process of gene origin and extinction occurs dynamically when a given genome gets compromised, leading to the emergence of new genes which get fixated in the genome at various times in evolution (Arendsee et al., 2014; Bhandary et al., 2018). And it is considered nearly impossible to synthesize something as complex as functional protein from scratch (Singh and Wurtele, 2020). Thus, the formation of OGs may comprise a vast reservoir of functional proteins with such a tremendous rate of evolution, making them nearly impossible to trace any homology features. Recent study developed a simple method to estimate the probability that a homolog would be detected at a specified evolutionary distance if it was evolving at a constant rate under standard, which indicating that many OGs can be explained by homology detection failure (Weisman et al., 2020). And more sensitive synteny-based homology searches successfully find previously undetected homologs for many OGs.
Special Expression Regulatory Mode of Orphan Genes
Expression analysis is a practical and effective method for detecting the OGs’ probable function (Xu et al., 2015). The expression patterns of OGs are special and widely verified in plants. According to semi-qPCR results and RNA-seq data, BrOGs (B. rapa OGs) are expressed in diverse organs, tissues, and developmental stages in Brassica plants and several BrOGs have also demonstrated tissue-specific, organ-specific, or developmental stage-specific expression patterns (Jiang et al., 2018). Similarly, few of the BrOGs can be induced by Plasmodiophora brassicae, a soil-borne, obligatory, and biotrophic pathogen that infects Brassica crops, leading to the development of clubroot and hence reducing overall agricultural output (Chen et al., 2016; Jiang et al., 2018). Thus, the above evidence indicates that BrOGs play a certain role in developmental stages, different tissues, organs, and biotic stress responses. Camellia-specific genes (CSGs) had more tissue-specific expression compared to evolutionary conserved genes (Zhao and Ma, 2021). The Arabidopsis-specific genes tend to possess an upregulated expression in mature pollen and under heat stress (Yang et al., 2009). Upon comparison with the evolutionarily conserved set, the OGs found in Arabidopsis have been more highly methylated in floral tissue (Lin et al., 2010) and have a greater degree of tissue specificity with lower expression levels (Donoghue et al., 2011). OGs were transcribed at a lower rate on average.
In O. sativa, a larger proportion of these OGs have been found to get expressed after sexual maturation and under environmental pressure in comparison to the non-OGs (Guo et al., 2007), and have shown relatively high levels of expression in flower tissue, pistil tissue, and root tissue (Yang et al., 2009), while all reported 37 OGs have been supported with at least a single evidence of expression (Jin et al., 2019). Similarly, OGs have shown relatively high levels of expression in the female flowers, xylem tissue, cambium tissue, and leaf tissue of the P. trichocarpa plant (Yang et al., 2009). Other studies have revealed that the detectable expression of OGs in young or mature leaves, stem bark, and roots are associated with tissue specific development of that species (Lin et al., 2013).
Likewise, in the case of V. unguiculata, OGs have also been considered a major contributing factor in maintaining the balance of the agronomic and adaptive traits of domesticated crops in various climatic conditions under artificial selection (Li G. et al., 2019). Lately, it has also been reported that the evolution of nuclear OGs can be the result of the involvement of the mitochondrial genome in land plants (O’Conner and Li, 2020). In C. sinensis, the OGs have been found preferentially expressed in the callus of the plant, while nine of the OGs were expressed in response to abiotic treatments (Xu et al., 2015). Similarly, Aegiceras-specific genes (ASGs) have also been found to get highly expressed on a tissue-specific level whereas 86 ASGs co-expressed gene modules are predominantly involved in pathways associated with adversity stress, including plant hormone signal transduction, phenylpropanoid biosynthesis, photosynthesis, peroxisome, and pentose phosphate pathway, as according to weighted gene co-expression network analysis (WGCNA) (Ma et al., 2021). The expression of OGs in the case of A. hypochondriacus could be a significant element to sustain the extreme tolerance ability to diverse stress conditions among these plants (Cabrales-Orona and Délano-Frier, 2021). Functional annotation of 861 conserved Poaceae-specific genes (CPSGs) showed that 346 (40.2%) CPSGs are annotated as expressed genes (Campbell et al., 2007).
By this point, our knowledge of OGs has been based primarily on comparative genomic studies and expression analysis. However, functions analysis of OGs still faces plenty of challenges due to the absence of recognizable domains, functional motifs, and identifiable folds. Fortunately, numerous researchers have made many representative studies while trying to unravel the mystery of the function of OGs, which lays a solid foundation for the functional identification of the plant OGs.
Mysterious Functions of Orphan Genes
The vast majority of OGs in plants have still not been discovered. Although the function of OGs has not been well investigated, OGs have been known to play a significant role in the divergence of species (Ma et al., 2021). Analysis of the functionality of OGs provides some evidences for its authenticity (Kumar et al., 2015; Jin et al., 2019). To date, researchers have been facing challenges in understanding the encoded functional mechanisms of all the newly discovered OGs as well as their source of origination has been a topic of debate (Kell, 1998; Amiri et al., 2003; Rödelsperger et al., 2019; Zhang et al., 2019). Despite being unable to decipher the complete mechanism of action of OGs, the studies have provided keen evidence for their major involvement in the processes like primary substance metabolism, response to biotic and abiotic stresses in addition to playing a major role in species-specific traits’ formation (Table 2).
Role of Orphan Genes in Primary Substance Metabolism and Response to Species-Specific Traits
Plant genome sequencing studies with high-quality annotations provide an unprecedented opportunity to investigate the role of OGs in plants during environmental adaptation (Ma et al., 2021). The Arabidopsis QQS (Qua-Quine Starch, At3g30720) gene was the first report of OG among plants. Li et al. (2009) discovered that Arabidopsis QQS has a novel regulatory role. QQS has been reported upregulated in Arabidopsis Atss3 mutants that lack starch synthase III and possess higher leaf starch content. Further experiments have indicated that the accumulation of QQS transcript occurs in the response to developmental, environmental, and genetic stimuli.
Similarly, genetic and biochemical evidence has also presented the significant role of QQS in the control of starch content (Li et al., 2009). The gene has also been reported to have a significant role in the alteration of plant composition, whereas the gene knockouts tend to develop decreased levels of protein content in leaves and seeds. Despite these molecular modifications, no significant differences in the morphology and development of soybeans expressing QQS compared to wild type sibling controls have been identified (Li and Wurtele, 2015). A series of transgenic experiments has shown that the transgenic QQS expression increases the protein content of maize and rice, which are highly divergent from Arabidopsis (Li et al., 2015). Further research suggests that the QQS protein interacts with the evolutionarily conserved transcription factor AtNF-YC4 (Arabidopsis nuclear factor Y, subunit C4, At5g63470), as well as NF-YC4 homologs in maize, rice, and soybean, establishing a role of QQS in carbon and nitrogen allocation across species (Li et al., 2015; Jones et al., 2016). AtNF-YC4 is a key element of the NF-Y transcription factor and contains histone-fold domains, which is universal among plant species with a highly conserved peptide sequence (Li et al., 2015; Qi et al., 2019). AtQQS and AtNF-YC4 expression increased total protein content and reduced starch content in both leaf and seed of soybean, rice, and maize, except starch was not detected in the soybean seed (Tanvir et al., 2022). The interaction of an orphan gene from one species with the metabolic network of another species via conserved proteins point to novel possible avenues for understanding the complex traits modulation and phenotypic changes in crop species (O’Conner et al., 2018). Furthermore, QQS is proven to be under autonomous epigenetic control and exhibits epigenetic variation among natural accessions, as well as epigenetic variation as well as in wild populations from Central Asia, implying that de novo originated genes may be particularly susceptible to epigenetic variation in their early stages of formation (Silveira et al., 2013).
In B. rapa, BrOGs have been found to produce an extensive impact on soluble sugar metabolism, while BrOG1 can also probably influence the content of soluble sugar in a SUS-dependent manner (Jiang M. et al., 2020). In this context, a BrOG overexpression (BrOGOE) mutant library for A. thaliana has been developed, with the BrOG1AOE mutant producing much higher levels of glucose, fructose, and total sugar content with exceptionally lower levels of sucrose. BrOG1AOE mutant has also been shown to possess reduced expression activity of the Arabidopsis sucrose synthase (SUS) gene, while the activity of invertase (INV) remained unchanged. The overall decrement in the total sugar content (i.e., fructose, glucose) along with an increase in sucrose contents have been prominent in Chinese cabbage BrOG1 knockout lines. Similarly, upon comparison with the control plants, the BOrG1 mutant has a greater activity of BrSUS enzymes with no significant difference in BrINV activity, as well as increased expression of BrSUS1b, BrSUS3, and BrSUS5 genes have been reported. This pattern of expression suggests that the phenotype of the BrOG1 knockout mutants complements that of the BrOG1AOE mutants. These findings also indicate that BrOGs play a vital role in soluble sugar metabolism. Furthermore, the genomisotopic methodology was developed to give a potent new method for identifying and characterizing orphan biosynthetic gene cluster products (Gross et al., 2007).
To date, the functional characterization of OGs is considered a challenging task, although it has been demonstrated that OGs may play a role in fundamental agronomic variables (Dossa et al., 2021). Poaceae-specific Male Sterility 1 (Ms1) encoded a phospholipid-binding protein, which was essential for male fertility and metagenesis, and pollen exine development (Tucker et al., 2017; Wang et al., 2017). The grass species-specific Ms2 (Male Sterility 2) genes found dominant in wheat, barley, and Brachypodium have been found responsible for conferring male sterility (Ni et al., 2017). This Ms2 gene appears as an OG and is only found in wheat and its close relatives, expression of which has also been reported in anthers of a flower and has been associated with insertion of a retroelement into the promoter. Ms2 gene also plays a pivotal role in wheat breeding, providing higher yields of hybrid wheat. Similarly, 18 OGs were proven to be related to male sterility in watermelon (Citrullus lanatus), and the research also confirmed that 182 OGs were involved in flower development in cucumber (Cucumis sativus), 520 OGs may help with the large fruit size in wax gourd (Benincasa hispida) (Ma et al., 2022). Study showed that 18 CSGs were mainly associated with phenylalanine biosynthesis, biosynthesis of amino acids, pentose phosphate pathway, photosynthesis, and carbon metabolism (Zhao and Ma, 2021). TSGs were proven to be associated with reproductive organ development, and 25 flower-specific TSGs showed specific expression in developing anthers and had an even higher expression level (Ma et al., 2020). 67 Conserved Brassicaceae-Specific Genes (CBSGs) was proven to be the pollen determinant, which controlling allele specific pollen rejection in self-incompatible Brassicaceae species (Lin et al., 2010). Overexpression of rice genome unique gene GN2 (GRAINS NUMBER 2) showed less grain number, reduced plant height, and later heading date than control plants, which provided new insight into general phenotypic evolution and more information to elucidate the molecular mechanisms underlying rice grain number (Chen et al., 2017). Higher plants-specific regulator GS9 (Grain Shape Gene on Chromosome 9) was involved in regulating grain shape and appearance quality in rice (Zhao et al., 2018). The interaction of GS9 and OsOFP14 (LOC_Os04g33870) resulting in repression of GS9 transcription activation activity. GS9 together with OsOFP8 (LOC_Os01g64430) and OsOFP14 form a transcriptional complex, which indicating that GS9 might function in regulating brassinosteroids (BR) pathway, at least partially through its interaction with OsOFP8, thereby modulating rice spikelet development (Zhao et al., 2018).
Response to Stress
High stress-specificity is regarded as a significant characteristic related to OGs (Guo et al., 2007; Bosch et al., 2009; Donoghue et al., 2011). In B. rapa, expression patterns of 52 BrOGs were assessed upon infection with P. brassicae (Jiang et al., 2018). A total of 41 out of 52 BrOGs responded to P. brassicae treatment, while 39 out of 41 BrOGs were found upregulated. This immediate response strongly suggests that BrOGs have a significant role in P. brassicae stress responses. However, understanding the role of BrOGs in B. rapa-P. brassicae interactions at a molecular level necessitate further research to develop new tools for resistance breeding in Brassicaceae crops and Chinese cabbage in the future. Similarly, In Arabidopsis, the Brassicaceae-specific EWR1 (Enhancer of Vascular Wilt Resistance 1) gene and its Brassica oleracea (BoEWR1) homologs confer resistance to Vascular Wilt pathogens (Yadeta et al., 2014). In N. benthamiana, overexpression of AtEWR1 and BoEWR1 causes Verticillium dahliae resistance. Furthermore, the Arabidopsis drought stress tolerance trait has been found augmented by over-expression of AtEWR1 (Yadeta et al., 2014).
Two Arabidopsis-specific POFs (Proteins with obscure features) and a protein with an unknown function from Arabidopsis and Brassica-specific protein have also been found to elevate oxidative stress tolerance (Luhua et al., 2008). On a similar note, several studies have reported enhanced plant antiviral and antibacterial immune responses upon activation of AtQQS and AtNF-YC4 genes. Likewise, increased resistance/reduced susceptibility toward soybean cyst nematodes, viruses, aphids, fungi, and viruses can also be attributed to the over-expression of these genes in Arabidopsis and soybean. Furthermore, by mediating crosstalk between primary metabolism and environmental alterations, the QQS gene has also been involved in the optimization and tolerance to biotic/abiotic perturbations (Arendsee et al., 2014; Qi et al., 2019; Tanvir et al., 2022). Recently, 51 OGs were proven to be associated with environmental adaptation in watermelon (Ma et al., 2022).
Studies have also described a unique set of TRGs present in grain amaranths, a characteristic feature that can be crucial for maintaining a high level of stress tolerance in a variety of conditions (Cabrales-Orona and Délano-Frier, 2021). In parallel, BRB (Big Root Biomass) is a Lamiales-specific gene, the overexpression of BRB in Arabidopsis modulates root and shoot traits whereas the same gene has been found responsible to induce drought stress sensitivity, modulating the auxin pathway along with a major impact on root and shoot traits (Dossa et al., 2021). BRB is preferentially expressed in sesame root as compared to other organs such seed, leaf, flower and capsule. Similarly, the single motif AACACACAC facilitates the binding of an MYB transcription factor (SiMYB181, SIN_1023179) in the 5′-UTR of BRB. The presence of a single motif facilitates the binding of SiMYB181, repressing BRB expression while the duplicated motif prevents the binding of SiMYB181, leading to a normal transcription of BRB (Dossa et al., 2021).
In the same manner, other TRGs, such as TaSRTRG6 and TaSRTRG7, interact with tiny secreted fungal proteins while promoting resistance toward Septoria tritici blotch (STB) (Brennan et al., 2020). The occurrence of these two genes (i.e., TaSRTRG6 and TaSRTRG7) has been restricted to the Poaceae family, and yeast two hybrid (Y2H) and bimolecular fluorescence complementation (BiFC) assays have revealed that TaSRTRG6 can interact with three different Zymoseptoria tritici SSPs (Small secreted proteins), including Zt11, Zt19, and Zt24. Correspondingly, the interaction of TaSRTRG7 has also been observed with two Z. tritici SSPs (i.e., Zt16 and Zt18) which suggests the potential role of TaSRTRG6 and TaSRTRG7 in STB resistance. Resistance to Xanthomonas oryzae pv. oryzae infection is conferred by the rice orphan gene Xa7, and the Xa7 protein has been discovered to be a necessary factor for resistance response (Wang et al., 2021).
Furthermore, TaFROG (T. aestivum Fusarium Resistance Orphan Gene) has also been reported to induce leaf resistance in wheat toward Fusarium graminearum by interacting with TaSnRK1 (Sucrose Non-Fermenting1-Related Kinase1) (Perochon et al., 2015). TaFROG interacts with TaNACL-D1 (T. aestivum NAC-like D1), a wheat transcription factor that provides resistance to the Fusarium head blight (FHB) disease. The co-expression of TaNACL-D1 and TaFROG genes has been reported at the molecular level in response to F. graminearum, the pathogenic fungus agent of FHB, and its virulence factor deoxynivalenol (DON) (Perochon et al., 2019). This provides a new source of resistance genes for crop breeding programs. Another study has found that TaFROG modulates host immunity by regulating TaSnRK1 proteasomal degradation (Jiang C. et al., 2020). Further research has revealed that Osp24 (Orphan secreted protein 24) of F. graminearum acts as a cytoplasmic effector by competing with TaFROG for TaSnRK1 binding, implying that orphan proteins from both the host and the fungal pathogen play antagonistic roles during their interactions (Jiang C. et al., 2020).
Other OGs, like cowpea (V. unguiculata) UP12_8740, a drought-inducible OG, showing the largest expression difference (7.4-fold) in roots under drought stress, tend to confer enhanced drought tolerance in composite transgenic plants (Li G. et al., 2019). The UP12_8740 gene has been found to exhibit greater expression levels in UP12_8740-OE lines than in control lines, indicating that UP12_8740 is a novel gene providing drought resistance in V. unguiculata, according to quantitative RT-PCR analysis. OGs can be regarded as a valuable resource for the identification of new genes that are engaged in certain environmental adaptations. A set of OGs being part of the Monocot-specific functional hydroxycinnamoyl putrescine (HP) gene cluster (including OsODC, OsPHT3, and OsPHT4) are involved in HP biosynthesis. Further genetic and biochemical analyses of their respective gene cluster have revealed that OsPHT3 and OsPHT4 positively regulate rice immunity and cell death in rice (Fang et al., 2021). Rice tribe-specific gene OsDR10 (O. sativa defense-responsive gene 10) functions as a negative regulator in the SA-dependent pathway to balance rice defense response induced by pathogen infection (Xiao et al., 2009). A defense-related Oryza-specific orphan gene Xio1 (Xoo-induced orphan 1) specifically induced by the bacterial pathogen X. oryzae pv. oryzae (Xoo) in an immune receptor XA21-dependent manner (Moon et al., 2022). Overexpression of Xio1 showed enhanced resistance to Xoo and reactive oxygen species accumulation.
Similarly, transposon element (TE)-assisted formation of hydroxycinnamoyl tyramine (HT) gene cluster (including OsPDX3, OsTyDC1, OsTHT1, and OsTHT2) is specific to Oryza AA genome lineage, which contributes to enhanced disease resistance and often exhibits co-expression in a specific tissue or under certain stresses (Shen et al., 2021). In a conducted study, 1,007 genes of unknown functions were taken from Arabidopsis from which the ones containing OGs were selected and assessed for the response of their corresponding homozygous T-DNA insertional mutants to hypoxia, cold, heat, salinity, oxidative, and osmotic stresses (Luhua et al., 2013). Out of which 832 out of 1,007 mutants displayed tolerance or sensitivity to multiple abiotic stress treatments, implying that genes with unknown functions may play a role in abiotic stress response signaling or general acclimation mechanisms. Study showed that nearly one third of the TSGs were stress-responsive and inducible under abiotic and/or biotic stresses (Ma et al., 2020). These findings provide a concrete library of genes that can play a significant role in plant defensive strategies against various kinds of environmental conditions.
Although the functions of OGs are still difficult to predict by homologous comparison or other bioinformatics approaches, recent studies indicated that OGs implicated in various abiotic and biotic stresses response, plant growth and development regulation, primary substance metabolism, and species-specific adaptation. Functional analysis of these OGs indicated that they are of great importance for plant improvement by using mutants or altering their expression, and the significance of OGs characterization is just showing up. However, an enormous amount of work is needed to unravel the mysterious functions of OGs. OGs are prevalent in life forms and are important for the genome evolution and phenotypic changes (Ma et al., 2021). The generation of OGs can contribute to the morphological diversity of distinct species, and many OGs may be very good models for studying of the evolution of organisms.
Concluding Remarks and Future Perspectives
Orphan genes are defined as the genes with no observable homolog in other lineages, and hence are a particular characteristic of an individual species. The availability of a large collection of genomic databases has made it possible to identify and characterize the functional characteristics of OGs. The present study has revealed that the OGs play a specific role in primary substance metabolism, species-specific traits’ formation, and response to diverse stresses, making them important for lineage-specific adaptations (Figure 1). OGs have a shorter origination time and distinctive features relative to ECGs among all species (Ma et al., 2022). Gene structure analysis showed that OGs exhibit significantly shorter protein length, which mainly attributed to the fewer number of exons, and younger genes have fewer exons. OGs possess unusual GC content among CDS compared to ECGs set. Although OGs in different plants have no sequence similarities, they share similar genic features, implying their evolutionary mechanisms could be similar (Ma et al., 2020). The evolutionary origin of OGs is a microevolutionary process, and the study of how OGs arise and differentiate is essential to explain the generation and succession of novel phenotypes and eventually biodiversity (Ma et al., 2022). The relevance between the adaptive evolution and generation of new agronomic traits still needed to be determined. For example, the divergence of B. rapa from A. thaliana was under a relatively short evolutionary time about 12.4–13.4 million years (Yang et al., 2006; Liu et al., 2014), but some B. rapa plants displayed extreme morphological characteristics as a result of artificial selection during domestication and breeding, such as leafy heads of Chinese cabbage (Cheng et al., 2016), which is the completely different phenotype from Arabidopsis. Many OGs may appear and become fixed and functional in the B. rapa genome during this relatively short evolutionary time, therefore, the relationship between these OGs and leafy head formation of Chinese cabbage will become a very interesting research direction. Furthermore, Chinese cabbage is a long-day biennial plant and needs a vernalizing stimulus and subsequent long-day conditions for bolting (Song et al., 2015; Su et al., 2018). However, A. thaliana is a facultative long-day species and flowers most quickly in the long photoperiods during spring and early summer, and can display either a summer- or winter-annual flowering phenotype (Kinmonth-Schultz et al., 2021). Thus, whether these OGs are involved in the different flowering habits control is also the focus of future research, which may provide insights and gene resources into bolting resistance regulation, especially in solving the problem of premature bolting of spring Chinese cabbage cultivation.
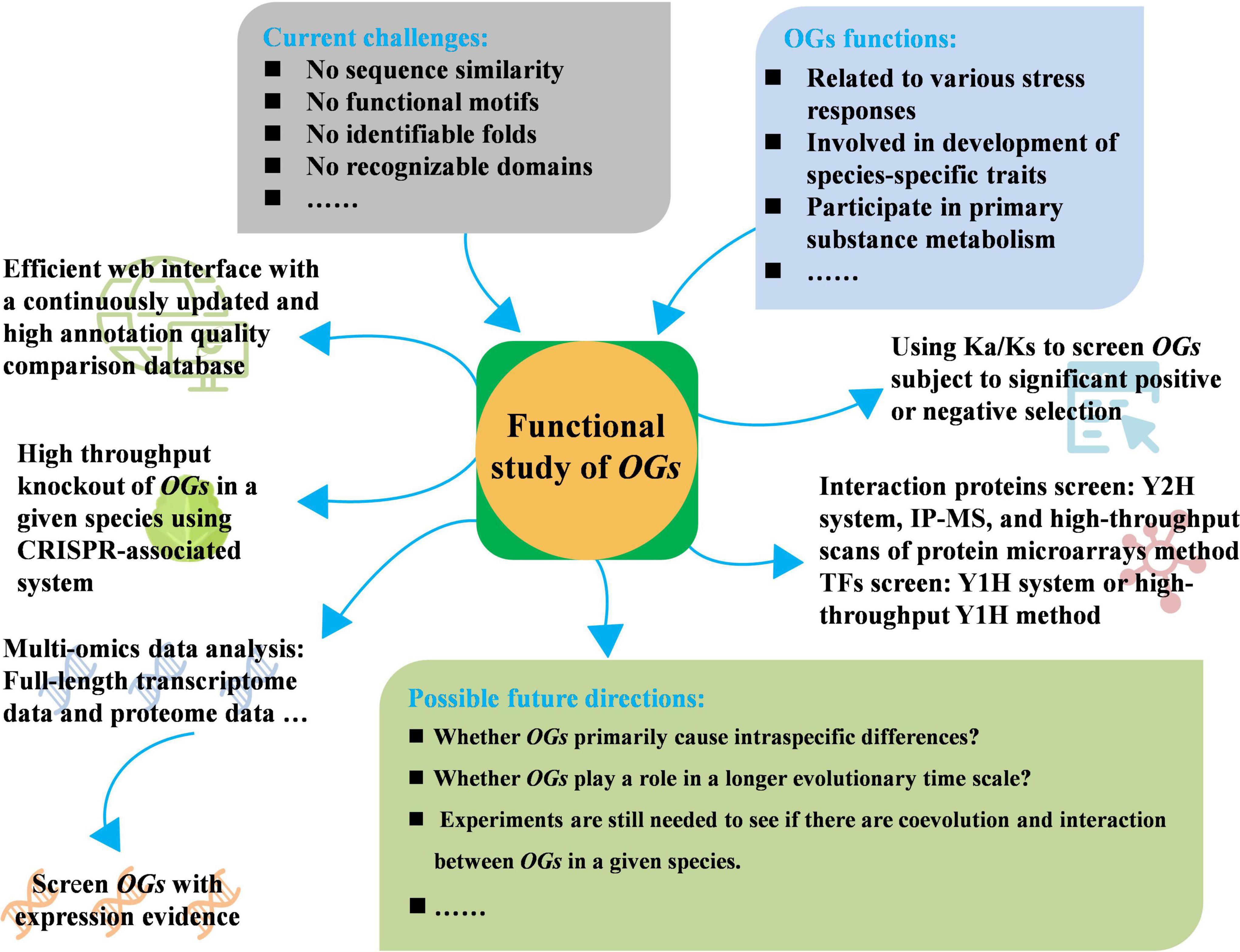
Figure 1. Current challenges, adoptable strategies, and possible future directions of functional study of orphan genes (OGs).
The current study also highlights the interactions made by OGs on a molecular level with more conserved cellular components, making them function across species, such as in the case of QQS. Thus, the predominant molecular genetic mechanisms controlling OGs emergence, turnover, and fixation remain to be elucidated. Although the mystery of OGs origins is gradually being unraveled, how some OGs can play a vital role in a very short evolutionary time and their specific functions need to be further studied, still, the mechanism of action and functional characteristics of OGs require further research in this arena. For OGs, we still need to continue to explore and discover, but we have now begun to trace the traces of their ancestors, and it seems that we can’t find the families of most OGs because they have no families. What is the relationship between the gene structures of OGs and its origin mechanism, origin motivation, and the influence of gene structure on biological function and phenotype? How does an OG participate in the complex biological network of an organism and perform biological functions? What drives the creation and retention of OG? These issues need to be further studied. At present, the research on the function of plant OGs started late, but the function of OGs can’t be underestimated. OGs are important for viability to the plants, and the discovery of many protein-protein interaction models has gradually uncovered the mysterious functions of OGs, such as the interaction of AtQQS and AtNF-YC4. OGs were not merely results of random evolutionary development, they may indeed be an essential part of organisms’ key trades (Tanvir et al., 2022), and the mysterious function of OGs still needs comprehensive exploration.
Although some progress has been made in the study of OGs, the research scope remains limited to a few species, the breadth of OGs function needs to be further explored, and some adoptable strategies may play a role in the study of the function of OGs. First, annotation quality of the database and search algorithms have a significant impact on the identification of OGs, one of the problems to be solved is to create an efficient web interface with a continuously updated and high annotation quality comparison database. Although comparative pangenomic database GreenPhylDB provided sequence information and protein domain signatures of OGs for several plant species, multiple expression data of OGs were not integrated, such as the transcriptome sequencing data under different conditions. Therefore, it is necessary to establish a new and comprehensive database for plant orphan gene comparison and annotation. Second, how do OGs participate in the complex biological network of organisms and play biological roles, as well as how do they participate in the life activities of important tissues of organisms, high-throughput knockout of OGs in a given species using CRISPR (Clustered Regularly Interspaced Short Palindromic Repeats) may significantly speed up the characterization of OGs function, clarifying the biological functions of OGs and their influence on phenotypes. CRISPR-associated system is a powerful genome-editing tool, combined with the development of next-generation sequencing and many other high-throughput techniques, which have thus been quickly developed into a high-throughput engineering strategy in plants (Huang et al., 2022). CRISPR-associated system has resulted in the development of powerful new screens to test gene functions at the genomic scale (Gaillochet et al., 2021). Mediated mutagenesis of CRISPR-associated system has increasingly been used to reveal or validate functions of candidate genes identified in genetic studies of various plant species (Puchta et al., 2022). Large-scale mutation have been mediated by using CRISPR-associated systems, which have been performed in tomato (Jacobs et al., 2017), rice (Meng et al., 2017; Ma et al., 2019), soya bean (Bai et al., 2020), maize (Liu et al., 2020), and cotton (Ramadan et al., 2021), which will provide theoretical basis and practical guidance for the functional study of OGs.
Due to the large number and proportion of OGs, it is very important to select suitable candidate OGs for their functional verification. Third, the use of multi-omics data, such as using full-length transcriptomes data to screen OGs with direct transcription evidence or using proteome data to screen OGs with expression evidence for subsequent verification. Multi-omics data will enable yet more powerful analyses and provide a powerful high-throughput approach to place them in a physiological context, and OGs are a wildly deviant group often with a highly variable pattern of expression, thus, even more than for ancient genes, it is important to use a wide variety of experimental conditions in any meta-analysis to evaluate OGs function (Bhandary et al., 2018). Recent study showed that 23 Holm oak (Quercus ilex) unique enzymes were related to the biosynthesis of hormones and secondary metabolites, which were identified through proteome analysis (López-Hidalgo et al., 2018). Fourth, using the genomic data of related species, Ka/Ks (the ratio of non-synonymous substitution and synonymous substitution) is used to determine whether there is selection pressure on candidate OGs, and the genes subject to significant selection may have important functions. Thus, the significance of choosing such OGs to study their functions may be greater. Recently, about 90% of the Carboxylesterase (CXE) genes of the four cotton species were found to have a Ka/Ks ratio of less than 1 after determination, which indicating that the family genes were affected strong purification options (Rui et al., 2022). The Brassica napus BnSDGs (SET domain genes) were proven to be under strong purifying selection during the evolution after Ka/Ks determination (Sehrish et al., 2022). Moreover, the interaction proteins of OGs of interest can be screened by different methods, such as Y2H system (Fields and Song, 1989), IP-MS (Immunoprecipitation-Mass Spectrometry) (Marcon et al., 2015), and high-throughput scans of protein microarrays method (Zhu et al., 2001; Struk et al., 2019). The functions of these OGs can be studied from the known functional interaction proteins. The construction of transcription factors (TFs) cDNA library can screen the potential interaction TFs of OGs with specific nuclear localization and no transcriptional activity by the yeast one-hybrid (Y1H) system, and clarify the function of target genes by analyzing the function of interaction TFs. Y1H system has become an important technique for detecting physical interactions between sequence-specific regulatory TFs and their DNA target sites (Reece-Hoyes and Marian Walhout, 2012). Recently, high-throughput Y1H screen has developed to provide a simple and effective strategy to identify TF-promoter interactions using a DNA fragment as bait (Li Z. et al., 2019), which will accelerate the identification of TFs that regulate the expression of OGs.
Although the evolutionary examples of OGs are described to determine whether they are preferentially included in the specific nodes of the regulatory network, future research is needed to determine whether they primarily cause intraspecific differences or play a role in a longer evolutionary time scale. Furthermore, experiments are still needed to see if there is coevolution and interaction between OGs in a given species. In brief, OGs have the potential to be utilized in the improvement of important agronomic traits and broaden gene resources for plant breeding.
Author Contributions
MJ and HL conceived the original idea. All authors contributed equally to writing and final editing, contributed to the article, and approved the submitted version.
Funding
This review was funded by the Fifth Batch of Jilin Province Youth Science and Technology Talent Promotion Project (QT202123).
Conflict of Interest
The authors declare that the research was conducted in the absence of any commercial or financial relationships that could be construed as a potential conflict of interest.
Publisher’s Note
All claims expressed in this article are solely those of the authors and do not necessarily represent those of their affiliated organizations, or those of the publisher, the editors and the reviewers. Any product that may be evaluated in this article, or claim that may be made by its manufacturer, is not guaranteed or endorsed by the publisher.
References
Amiri, H., Davids, W., and Andersson, S. G. (2003). Birth and death of orphan genes in Rickettsia. Mol. Biol. Evol. 20, 1575–1587. doi: 10.1093/molbev/msg175
Aoki, Y., Okamura, Y., Tadaka, S., Kinoshita, K., and Obayashi, T. (2016). ATTED-II in 2016: a plant coexpression database towards lineage-specific coexpression. Plant Cell Physiol. 57:e5. doi: 10.1093/pcp/pcv165
Arendsee, Z. W., Li, L., and Wurtele, E. S. (2014). Coming of age: orphan genes in plants. Trends Plant Sci. 19, 698–708. doi: 10.1016/j.tplants.2014.07.003
Bai, M., Yuan, J., Kuang, H., Gong, P., Li, S., Zhang, Z., et al. (2020). Generation of a multiplex mutagenesis population via pooled CRISPR-Cas9 in soya bean. Plant Biotechnol. J. 18, 721–731. doi: 10.1111/pbi.13239
Bhandary, P., Seetharam, A. S., Arendsee, Z. W., Hur, M., and Wurtele, E. S. (2018). Raising orphans from a metadata morass: a researcher’s guide to re-use of public’ omics data. Plant Sci. 267, 32–47. doi: 10.1016/j.plantsci.2017.10.014
Blanco-Melo, D., Venkatesh, S., and Bieniasz, P. D. (2016). Origins and evolution of tetherin, an orphan antiviral gene. Cell Host Microbe. 20, 189–201. doi: 10.1016/j.chom.2016.06.007
Bosch, T. C. G., Augustin, R., Anton-Erxleben, F., Fraune, S., Hemmrich, G., Zill, H., et al. (2009). Uncovering the evolutionary history of innate immunity: the simple metazoan Hydra uses epithelial cells for host defence. Dev. Comp. Immunol. 33, 559–569. doi: 10.1016/j.dci.2008.10.004
Brennan, C. J., Zhou, B., Benbow, H. R., Ajaz, S., Karki, S. J., Hehir, J. G., et al. (2020). Taxonomically restricted wheat genes interact with small secreted fungal proteins and enhance resistance to Septoria tritici blotch disease. Front. Plant Sci. 11:433. doi: 10.3389/fpls.2020.00433
Cabrales-Orona, G., and Délano-Frier, J. P. (2021). “Searching for an identity: functional characterization of taxonomically restricted genes in grain Amaranth,” in Compendium of Plant Genomes, ed. C. Kole (Switzerland, AG: Springer Cham), 97–124. doi: 10.1007/978-3-030-72365-1_7
Cai, J. J., and Petrov, D. A. (2010). Relaxed purifying selection and possibly high rate of adaptation in primate lineage-specific genes. Genome Biol. Evol. 2, 393–409. doi: 10.1093/gbe/evq019
Cai, J. J., Woo, P. C., Lau, S. K., Smith, D. K., and Yuen, K. Y. (2006). Accelerated evolutionary rate may be responsible for the emergence of lineage-specific genes in ascomycota. J. Mol. Evol. 63, 1–11. doi: 10.1007/s00239-004-0372-5
Campbell, M. A., Zhu, W., Jiang, N., Lin, H., Ouyang, S., Childs, K. L., et al. (2007). Identification and characterization of lineage-specific genes within the Poaceae. Plant Physiol. 145, 1311–1322. doi: 10.1104/pp.107.104513
Carvunis, A. R., Rolland, T., Wapinski, I., Calderwood, M. A., Yildirim, M. A., Simonis, N., et al. (2012). Proto-genes and de novo gene birth. Nature 487, 370–374. doi: 10.1038/nature11184
Chen, H., Tang, Y., Liu, J., Tan, L., Jiang, J., Wang, M., et al. (2017). Emergence of a novel chimeric gene underlying grain number in rice. Genetics 205, 993–1002. doi: 10.1534/genetics.116.188201
Chen, J., Pang, W., Chen, B., Zhang, C., and Piao, Z. (2016). Transcriptome analysis of Brassica rapa near-isogenic lines carrying clubroot-resistant and -susceptible alleles in response to Plasmodiophora brassicae during early infection. Front. Plant Sci. 6:1183. doi: 10.3389/fpls.2015.01183
Chen, K., Tian, Z., Chen, P., He, H., Jiang, F., and Long, C. A. (2020). Genome-wide identification, characterization and expression analysis of lineage-specific genes within Hanseniaspora yeasts. FEMS Microbiol. Lett. 367:fnaa077. doi: 10.1093/femsle/fnaa077
Cheng, F., Sun, R., Hou, X., Zheng, H., Zhang, F., Zhang, Y., et al. (2016). Subgenome parallel selection is associated with morphotype diversification and convergent crop domestication in Brassica rapa and Brassica oleracea. Nat. Genet. 48, 1218–1224. doi: 10.1038/ng.3634
Cui, X., Lv, Y., Chen, M., Nikoloski, Z., Twell, D., and Zhang, D. (2015). Young genes out of the male: an insight from evolutionary age analysis of the pollen transcriptome. Mol. Plant 8, 935–945. doi: 10.1016/j.molp.2014.12.008
Domazet-Lošo, T., Brajković, J., and Tautz, D. (2007). A phylostratigraphy approach to uncover the genomic history of major adaptations in metazoan lineages. Trends Genet. 23, 533–539. doi: 10.1016/j.tig.2007.08.014
Donoghue, M. T., Keshavaiah, C., Swamidatta, S. H., and Spillane, C. (2011). Evolutionary origins of Brassicaceae specific genes in Arabidopsis thaliana. BMC Evol. Biol. 11:47. doi: 10.1186/1471-2148-11-47
Dossa, K., Zhou, R., Li, D., Liu, A., Qin, L., Mmadi, M. A., et al. (2021). A novel motif in the 5’-UTR of an orphan gene ‘Big Root Biomass’ modulates root biomass in sesame. Plant Biotechnol. J. 19, 1065–1079. doi: 10.1111/pbi.13531
Ekstrom, A., and Yin, Y. (2016). ORFanFinder-automated identification of taxonomically restricted orphan genes. Bioinformatics 32, 2053–2055. doi: 10.1093/bioinformatics/btw122
Fang, H., Shen, S., Wang, D., Zhang, F., Zhang, C., Wang, Z., et al. (2021). A monocot-specific hydroxycinnamoylputrescine gene cluster contributes to immunity and cell death in rice. Sci. Bull. 66, 2381–2393. doi: 10.1016/j.scib.2021.06.014
Fields, S., and Song, O. (1989). A novel genetic system to detect protein-protein interactions. Nature 340, 245–246. doi: 10.1038/340245a0
Gaillochet, C., Develtere, W., and Jacobs, T. B. (2021). CRISPR screens in plants: approaches, guidelines, and future prospects. Plant Cell 33, 794–813. doi: 10.1093/plcell/koab099
Gao, Q., Jin, X., Xia, E., Wu, X., Gu, L., Yan, H., et al. (2020). Identification of orphan genes in unbalanced datasets based on ensemble learning. Front. Genet. 11:820. doi: 10.3389/fgene.2020.00820
Gao, Q. J., Yan, H. W., Xia, E. H., Zhang, S. H., and Li, S. W. (2019). TOGD: a database of orphan genes in Triticum aestivum. Int. J. Agric. Biol. 22, 961–966. doi: 10.17957/IJAB/15.1155
Graham, M. A., Silverstein, K. A., Cannon, S. B., and VandenBosch, K. A. (2004). Computational identification and characterization of novel genes from legumes. Plant Physiol. 135, 1179–1197. doi: 10.1104/pp.104.037531
Gross, H., Stockwell, V. O., Henkels, M. D., Nowak-Thompson, B., Loper, J. E., and Gerwick, W. H. (2007). The genomisotopic approach: a systematic method to isolate products of orphan biosynthetic gene clusters. Chem. Biol. 14, 53–63. doi: 10.1016/j.chembiol.2006.11.007
Guo, W. J., Li, P., Ling, J., and Ye, S. P. (2007). Significant comparative characteristics between orphan and nonorphan genes in the rice (Oryza sativa L.) genome. Comp. Funct. Genomics 2007:21676. doi: 10.1155/2007/21676
Guo, Y. L. (2013). Gene family evolution in green plants with emphasis on the origination and evolution of Arabidopsis thaliana genes. Plant J. 73, 941–951. doi: 10.1111/tpj.12089
Huang, Y., Shang, M., Liu, T., and Wang, K. (2022). High-throughput methods for genome editing: the more the better. Plant Physiol. 188, 1731–1745. doi: 10.1093/plphys/kiac017
Jacobs, T. B., Zhang, N., Patel, D., and Martin, G. B. (2017). Generation of a collection of mutant tomato lines using pooled CRISPR libraries. Plant Physiol. 174, 2023–2037. doi: 10.1104/pp.17.00489
Jiang, C., Hei, R., Yang, Y., Zhang, S., Wang, Q., Wang, W., et al. (2020). An orphan protein of Fusarium graminearum modulates host immunity by mediating proteasomal degradation of TaSnRK1α. Nat. Commun. 11:4382. doi: 10.1038/s41467-020-18240-y
Jiang, M., Zhan, Z., Li, H., Dong, X., Cheng, F., and Piao, Z. (2020). Brassica rapa orphan genes largely affect soluble sugar metabolism. Hortic. Res. 7:181. doi: 10.1038/s41438-020-00403-z
Jiang, M., Dong, X., Lang, H., Pang, W., Zhan, Z., Li, X., et al. (2018). Mining of Brassica-specific genes (BSGs) and their induction in different developmental stages and under Plasmodiophora brassicae stress in Brassica rapa. Int. J. Mol. Sci. 19:2064. doi: 10.3390/ijms19072064
Jin, G. H., Zhou, Y. L., Yang, H., Hu, Y. T., Shi, Y., Li, L., et al. (2019). Genetic innovations: transposable element recruitment and de novo formation lead to the birth of orphan genes in the rice genome. J. Syst. Evol. 59, 341–351. doi: 10.1111/jse.12548
Jones, D. C., Zheng, W., Huang, S., Du, C., Zhao, X., Yennamalli, R. M., et al. (2016). A clade-specific Arabidopsis gene connects primary metabolism and senescence. Front. Plant Sci. 7:983. doi: 10.3389/fpls.2016.00983
Kell, D. B. (1998). From code to mode for orphan genes. Trends Biotechnol. 16, 491–493. doi: 10.1016/S0167-7799(98)01254-2
Khalturin, K., Hemmrich, G., Fraune, S., Augustin, R., and Bosch, T. C. (2009). More than just orphans: are taxonomically-restricted genes important in evolution? Trends Genet. 25, 404–413. doi: 10.1016/j.tig.2009.07.006
Kinmonth-Schultz, H., Lewandowska-Sabat, A., Imaizumi, T., Ward, J. K., Rognli, O. A., and Fjellheim, S. (2021). Flowering times of wild Arabidopsis accessions from across norway correlate with expression levels of FT, CO, and FLC genes. Front. Plant Sci. 12:747740. doi: 10.3389/fpls.2021.747740
Kumar, A., Gates, P. B., Czarkwiani, A., and Brockes, J. P. (2015). An orphan gene is necessary for preaxial digit formation during salamander limb development. Nat. Commun. 6:8684. doi: 10.1038/ncomms9684
Li, G., Wu, X., Hu, Y., Munoz-Amatriain, M., Luo, J., Zhou, W., et al. (2019). Orphan genes are involved in drought adaptations and ecoclimatic-oriented selections in domesticated cowpea. J. Exp. Bot. 70, 3101–3110. doi: 10.1093/jxb/erz145
Li, Z., Bonaldi, K., Kang, S. E., and Pruneda-Paz, J. L. (2019). High-throughput yeast one-hybrid screens using a cell surface gLUC reporter. Curr. Protoc. Plant Biol. 4:e20086. doi: 10.1002/cppb.20086
Li, J., Singh, U., Bhandary, P., Campbell, J., Arendsee, Z., Seetharam, A. S., et al. (2021). Foster thy young: enhanced prediction of orphan genes in assembled genomes. Nucleic Acids Res. 50:e37. doi: 10.1093/nar/gkab1238
Li, T. P., Zhang, L. W., Li, Y. Q., You, M. S., and Zhao, Q. (2021). Functional analysis of the orphan genes Tssor-3 and Tssor-4 in male Plutella xylostella. J. Integr. Agric. 20, 1880–1888. doi: 10.1016/s2095-3119(21)63655-9
Li, L., Foster, C. M., Gan, Q., Nettleton, D., James, M. G., Myers, A. M., et al. (2009). Identification of the novel protein QQS as a component of the starch metabolic network in Arabidopsis leaves. Plant J. 58, 485–498. doi: 10.1111/j.1365-313X.2009.03793.x
Li, L., and Wurtele, E. S. (2015). The QQS orphan gene of Arabidopsis modulates carbon and nitrogen allocation in soybean. Plant Biotechnol. J. 13, 177–187. doi: 10.1111/pbi.12238
Li, L., Zheng, W., Zhu, Y., Ye, H., Tang, B., Arendsee, Z. W., et al. (2015). QQS orphan gene regulates carbon and nitrogen partitioning across species via NF-YC interactions. Proc. Natl. Acad. Sci. U. S. A. 112, 14734–14739. doi: 10.1073/pnas.1514670112
Lin, H., Moghe, G., Ouyang, S., Iezzoni, A., Shiu, S. H., Gu, X., et al. (2010). Comparative analyses reveal distinct sets of lineage-specific genes within Arabidopsis thaliana. BMC Evol. Biol. 10:41. doi: 10.1186/1471-2148-10-41
Lin, W. L., Cai, B., and Cheng, Z. M. (2013). Identification and characterization of lineage-specific genes in Populus trichocarpa. Plant Cell Tissue Organ Cult. 116, 217–225. doi: 10.1007/s11240-013-0397-9
Liu, H. J., Jian, L., Xu, J., Zhang, Q., Zhang, M., Jin, M., et al. (2020). High-throughput CRISPR/Cas9 mutagenesis streamlines trait gene identification in maize. Plant Cell 32, 1397–1413. doi: 10.1105/tpc.19.00934
Liu, S., Liu, Y., Yang, X., Tong, C., Edwards, D., Parkin, I. A., et al. (2014). The Brassica oleracea genome reveals the asymmetrical evolution of polyploid genomes. Nat. Commun. 5:4930. doi: 10.1038/ncomms4930
López-Hidalgo, C., Guerrero-Sánchez, V. M., Gómez-Gálvez, I., Sánchez-Lucas, R., Castillejo-Sánchez, M. A., Maldonado-Alconada, A. M., et al. (2018). A multi-omics analysis pipeline for the metabolic pathway reconstruction in the orphan species Quercus ilex. Front. Plant Sci. 9:935. doi: 10.3389/fpls.2018.00935
Luhua, S., Ciftci-Yilmaz, S., Harper, J., Cushman, J., and Mittler, R. (2008). Enhanced tolerance to oxidative stress in transgenic Arabidopsis plants expressing proteins of unknown function. Plant Physiol. 148, 280–292. doi: 10.1104/pp.108.124875
Luhua, S., Hegie, A., Suzuki, N., Shulaev, E., Luo, X., Cenariu, D., et al. (2013). Linking genes of unknown function with abiotic stress responses by high-throughput phenotype screening. Physiol. Plant. 148, 322–333. doi: 10.1111/ppl.12013
Ma, D., Ding, Q., Guo, Z., Zhao, Z., Wei, L., Li, Y., et al. (2021). Identification, characterization and expression analysis of lineage-specific genes within mangrove species Aegiceras corniculatum. Mol. Genet. Genomics 296, 1235–1247. doi: 10.1007/s00438-021-01810-0
Ma, D., Lai, Z., Ding, Q., Zhang, K., Chang, K., Li, S., et al. (2022). Identification, characterization and function of orphan genes among the current Cucurbitaceae genomes. Front. Plant Sci. 13:872137. doi: 10.3389/fpls.2022.872137
Ma, K., Han, J., Hao, Y., Yang, Z., Chen, J., Liu, Y. G., et al. (2019). An effective strategy to establish a male sterility mutant mini-library by CRISPR/Cas9-mediated knockout of anther-specific genes in rice. J. Genet. Genomics 46, 273–275. doi: 10.1016/j.jgg.2019.03.005
Ma, S., Yuan, Y., Tao, Y., Jia, H., and Ma, Z. (2020). Identification, characterization and expression analysis of lineage-specific genes within Triticeae. Genomics 112, 1343–1350. doi: 10.1016/j.ygeno.2019.08.003
Mahalak, K. K., and Chamberlin, H. M. (2015). Orphan genes find a home: interspecific competition and gene network evolution. PLoS Genet. 11:e1005254. doi: 10.1371/journal.pgen.1005254
Marcon, E., Jain, H., Bhattacharya, A., Guo, H., Phanse, S., Pu, S., et al. (2015). Assessment of a method to characterize antibody selectivity and specificity for use in immunoprecipitation. Nat. Methods 12, 725–731. doi: 10.1038/nmeth.3472
Meng, X., Yu, H., Zhang, Y., Zhuang, F., Song, X., Gao, S., et al. (2017). Construction of a genome-wide mutant library in rice using CRISPR/Cas9. Mol. Plant 10, 1238–1241. doi: 10.1016/j.molp.2017.06.006
Moon, H., Jeong, A. R., Kwon, O. K., and Park, C. J. (2022). Oryza-specific orphan protein triggers enhanced resistance to Xanthomonas oryzae pv. oryzae in rice. Front. Plant Sci. 13:859375. doi: 10.3389/fpls.2022.859375
Moyers, B. A., and Zhang, J. (2018). Toward reducing phylostratigraphic errors and biases. Genome Biol. Evol. 10, 2037–2048. doi: 10.1093/gbe/evy161
Mukherjee, S., Panda, A., and Ghosh, T. C. (2015). Elucidating evolutionary features and functional implications of orphan genes in Leishmania major. Infect. Genet. Evol. 32, 330–337. doi: 10.1016/j.meegid.2015.03.031
Ni, F., Qi, J., Hao, Q., Lyu, B., Luo, M. C., Wang, Y., et al. (2017). Wheat Ms2 encodes for an orphan protein that confers male sterility in grass species. Nat. Commun. 8:15121. doi: 10.1038/ncomms15121
O’Conner, S., and Li, L. (2020). Mitochondrial fostering: the mitochondrial genome may play a role in plant orphan gene evolution. Front. Plant Sci. 11:600117. doi: 10.3389/fpls.2020.600117
O’Conner, S., Neudorf, A., Zheng, W., Qi, M., Zhao, X., Du, C., et al. (2018). “From Arabidopsis to crops: the Arabidopsis QQS orphan gene modulates nitrogen allocation across species,” in Engineering Nitrogen Utilization in Crop Plants, eds A. Shrawat, A. Zayed, and D. A. Lightfoot (Switzerland, AG: Springer Cham), 95–117. doi: 10.1007/978-3-319-92958-3_6
Palmieri, N., Kosiol, C., and Schlötterer, C. (2014). The life cycle of Drosophila orphan genes. Elife 3:e01311. doi: 10.7554/elife.01311
Perochon, A., Jianguang, J., Kahla, A., Arunachalam, C., Scofield, S. R., Bowden, S., et al. (2015). TaFROG encodes a Pooideae orphan protein that interacts with SnRK1 and enhances resistance to the mycotoxigenic fungus Fusarium graminearum. Plant Physiol. 169, 2895–2906. doi: 10.1104/pp.15.01056
Perochon, A., Kahla, A., Vranić, M., Jia, J., Malla, K. B., Craze, M., et al. (2019). A wheat NAC interacts with an orphan protein and enhances resistance to Fusarium head blight disease. Plant Biotechnol. J. 17, 1892–1904. doi: 10.1111/pbi.13105
Plissonneau, C., Stürchler, A., and Croll, D. (2016). The evolution of orphan regions in genomes of a fungal pathogen of wheat. mBio 7:e01231-16. doi: 10.1128/mBio.01231-16
Prabh, N., and Rödelsperger, C. (2016). Are orphan genes protein-coding, prediction artifacts, or non-coding RNAs? BMC Bioinf. 17:226. doi: 10.1186/s12859-016-1102-x
Prabh, N., and Rödelsperger, C. (2019). De novo, divergence, and mixed origin contribute to the emergence of orphan genes in Pristionchus nematodes. G3 9, 2277–2286. doi: 10.1534/g3.119.400326
Puchta, H., Jiang, J., Wang, K., and Zhao, Y. (2022). Updates on gene editing and its applications. Plant Physiol. 188, 1725–1730. doi: 10.1093/plphys/kiac032
Qi, M., Zheng, W., Zhao, X., Hohenstein, J. D., Kandel, Y., O’Conner, S., et al. (2019). QQS orphan gene and its interactor NF-YC4 reduce susceptibility to pathogens and pests. Plant Biotechnol. J. 17, 252–263. doi: 10.1111/pbi.12961
Ramadan, M., Alariqi, M., Ma, Y., Li, Y., Liu, Z., Zhang, R., et al. (2021). Efficient CRISPR/Cas9 mediated pooled-sgRNAs assembly accelerates targeting multiple genes related to male sterility in cotton. Plant Methods 17:16. doi: 10.1186/s13007-021-00712-x
Reece-Hoyes, J. S., and Marian Walhout, A. J. (2012). Yeast one-hybrid assays: a historical and technical perspective. Methods 57, 441–447. doi: 10.1016/j.ymeth.2012.07.027
Rensink, W. A., Lee, Y., Liu, J., Iobst, S., Ouyang, S., and Buell, C. R. (2005). Comparative analyses of six solanaceous transcriptomes reveal a high degree of sequence conservation and species-specific transcripts. BMC Genomics 6:124. doi: 10.1186/1471-2164-6-124
Rödelsperger, C., Prabh, N., and Sommer, R. J. (2019). New gene origin and deep taxon phylogenomics: opportunities and challenges. Trends Genet. 35, 914–922. doi: 10.1016/j.tig.2019.08.007
Rui, C., Peng, F., Fan, Y., Zhang, Y., Zhang, Z., Xu, N., et al. (2022). Genome-wide expression analysis of carboxylesterase (CXE) gene family implies GBCXE49 functional responding to alkaline stress in cotton. BMC Plant Biol. 22:194. doi: 10.1186/s12870-022-03579-9
Schlötterer, C. (2015). Genes from scratch-the evolutionary fate of de novo genes. Trends Genet. 31, 215–219. doi: 10.1016/j.tig.2015.02.007
Sehrish, S., Sumbal, W., Xie, M., Zhao, C., Zuo, R., Gao, F., et al. (2022). Genome-wide identification and characterization of SET domain family genes in Brassica napus L. Int. J. Mol. Sci. 23:1936. doi: 10.3390/ijms23041936
Shen, S., Peng, M., Fang, H., Wang, Z., Zhou, S., Jing, X., et al. (2021). An Oryza-specific hydroxycinnamoyl tyramine gene cluster contributes to enhanced disease resistance. Sci. Bull. 66, 2369–2380. doi: 10.1016/j.scib.2021.03.015
Siew, N., and Fischer, D. (2003). Analysis of singleton ORFans in fully sequenced microbial genomes. Proteins 53, 241–251. doi: 10.1002/prot.10423
Silveira, A. B., Trontin, C., Cortijo, S., Barau, J., Del Bem, L. E., Loudet, O., et al. (2013). Extensive natural epigenetic variation at a de novo originated gene. PLoS Genet. 9:e1003437. doi: 10.1371/journal.pgen.1003437
Singh, U., and Wurtele, E. S. (2020). How new genes are born. Elife 9:e55136. doi: 10.7554/eLife.55136
Song, X., Duan, W., Huang, Z., Liu, G., Wu, P., Liu, T., et al. (2015). Comprehensive analysis of the flowering genes in Chinese cabbage and examination of evolutionary pattern of CO-like genes in plant kingdom. Sci. Rep. 5:14631. doi: 10.1038/srep14631
Struk, S., Jacobs, A., Sánchez Martín-Fontecha, E., Gevaert, K., Cubas, P., and Goormachtig, S. (2019). Exploring the protein-protein interaction landscape in plants. Plant Cell Environ. 42, 387–409. doi: 10.1111/pce.13433
Su, T., Wang, W., Li, P., Zhang, B., Li, P., Xin, X., et al. (2018). A genomic variation map provides insights into the genetic basis of spring Chinese cabbage (Brassica rapa ssp. pekinensis) selection. Mol. Plant 11, 1360–1376. doi: 10.1016/j.molp.2018.08.006
Tanvir, R., Ping, W., Sun, J., Cain, M., Li, X., and Li, L. (2022). AtQQS orphan gene and NtNF-YC4 boost protein accumulation and pest resistance in tobacco (Nicotiana tabacum). Plant Sci. 317, 111198. doi: 10.1016/j.plantsci.2022.111198
Tautz, D., and Domazet-Lošo, T. (2011). The evolutionary origin of orphan genes. Nat. Rev. Genet. 12, 692–702. doi: 10.1038/nrg3053
Toll-Riera, M., Bosch, N., Bellora, N., Castelo, R., Armengol, L., Estivill, X., et al. (2009). Origin of primate orphan genes: a comparative genomics approach. Mol. Biol. Evol. 26, 603–612. doi: 10.1093/molbev/msn281
Tucker, E. J., Baumann, U., Kouidri, A., Suchecki, R., Baes, M., Garcia, M., et al. (2017). Molecular identification of the wheat male fertility gene Ms1 and its prospects for hybrid breeding. Nat. Commun. 8, 869. doi: 10.1038/s41467-017-00945-2
Vakirlis, N., Carvunis, A. R., and McLysaght, A. (2020). Synteny-based analyses indicate that sequence divergence is not the main source of orphan genes. Elife 9:e53500. doi: 10.7554/eLife.53500
Valentin, G., Abdel, T., Gaetan, D., Jean-Francois, D., Matthieu, C., and Mathieu, R. (2021). GreenPhylDB v5: a comparative pangenomic database for plant genomes. Nucleic Acids Res. 49, D1464–D1471. doi: 10.1093/nar/gkaa1068
Varshney, R. K., Chen, W., Li, Y., Bharti, A. K., Saxena, R. K., Schlueter, J. A., et al. (2011). Draft genome sequence of pigeonpea (Cajanus cajan), an orphan legume crop of resource-poor farmers. Nat. Biotechnol. 30, 83–89. doi: 10.1038/nbt.2022
Wang, C., Chen, S., Feng, A., Su, J., Wang, W., Feng, J., et al. (2021). Xa7, a small orphan gene harboring promoter trap for AvrXa7, leads to the durable resistance to Xanthomonas oryzae pv. oryzae. Rice 14:48. doi: 10.1186/s12284-021-00490-z
Wang, Z., Li, J., Chen, S., Heng, Y., Chen, Z., Yang, J., et al. (2017). Poaceae-specific MS1 encodes a phospholipid-binding protein for male fertility in bread wheat. Proc. Natl. Acad. Sci. U. S. A. 114, 12614–12619. doi: 10.1073/pnas.1715570114
Weisman, C. M., Murray, A. W., and Eddy, S. R. (2020). Many, but not all, lineage-specific genes can be explained by homology detection failure. PLoS Biol. 18:e3000862. doi: 10.1371/journal.pbio.3000862
Wilson, G. A., Bertrand, N., Patel, Y., Hughes, J. B., Feil, E. J., and Field, D. (2005). Orphans as taxonomically restricted and ecologically important genes. Microbiology 151, 2499–2501. doi: 10.1099/mic.0.28146-0
Wissler, L., Gadau, J., Simola, D. F., Helmkampf, M., and Bornberg-Bauer, E. (2013). Mechanisms and dynamics of orphan gene emergence in insect genomes. Genome Biol. Evol. 5, 439–455. doi: 10.1093/gbe/evt009
Xiao, W., Liu, H., Li, Y., Li, X., Xu, C., Long, M., et al. (2009). A rice gene of de novo origin negatively regulates pathogen-induced defense response. PLoS One 4:e4603. doi: 10.1371/journal.pone.0004603
Xu, Y., Wu, G., Hao, B., Chen, L., Deng, X., and Xu, Q. (2015). Identification, characterization and expression analysis of lineage-specific genes within sweet orange (Citrus sinensis). BMC Genomics 16:995. doi: 10.1186/s12864-015-2211-z
Yadeta, K. A., Valkenburg, D. J., Hanemian, M., Marco, Y., and Thomma, B. P. (2014). The Brassicaceae-specific EWR1 gene provides resistance to vascular wilt pathogens. PLoS One 9:e88230. doi: 10.1371/journal.pone.0088230
Yang, T. J., Kim, J. S., Kwon, S. J., Lim, K. B., Choi, B. S., Kim, J. A., et al. (2006). Sequence-level analysis of the diploidization process in the triplicated FLOWERING LOCUS C region of Brassica rapa. Plant Cell 18, 1339–1347. doi: 10.1105/tpc.105.040535
Yang, X., Jawdy, S., Tschaplinski, T. J., and Tuskan, G. A. (2009). Genome-wide identification of lineage-specific genes in Arabidopsis, Oryza and Populus. Genomics 93, 473–480. doi: 10.1016/j.ygeno.2009.01.002
Ye, C. Y., Li, T., Yin, H., Weston, D. J., Tuskan, G. A., Tschaplinski, T. J., et al. (2013). Evolutionary analyses of non-family genes in plants. Plant J. 73, 788–797. doi: 10.1111/tpj.12073
Yin, Y., and Fischer, D. (2008). Identification and investigation of ORFans in the viral world. BMC Genomics 9:24. doi: 10.1186/1471-2164-9-24
Zhang, W., Gao, Y., Long, M., and Shen, B. (2019). Origination and evolution of orphan genes and de novo genes in the genome of Caenorhabditis elegans. Sci. China Life Sci. 62, 579–593. doi: 10.1007/s11427-019-9482-0
Zhao, D. S., Li, Q. F., Zhang, C. Q., Zhang, C., Yang, Q. Q., Pan, L. X., et al. (2018). GS9 acts as a transcriptional activator to regulate rice grain shape and appearance quality. Nat. Commun. 9:1240. doi: 10.1038/s41467-018-03616-y
Zhao, Z., and Ma, D. (2021). Genome-wide identification, characterization and function analysis of lineage-specific genes in the tea plant Camellia sinensis. Front. Genet. 12:770570. doi: 10.3389/fgene.2021.770570
Zhu, H., Bilgin, M., Bangham, R., Hall, D., Casamayor, A., Bertone, P., et al. (2001). Global analysis of protein activities using proteome chips. Science 293, 2101–2105. doi: 10.1126/science.1062191
Keywords: plant, orphan genes, identification, functions, research advances, prospects
Citation: Jiang M, Li X, Dong X, Zu Y, Zhan Z, Piao Z and Lang H (2022) Research Advances and Prospects of Orphan Genes in Plants. Front. Plant Sci. 13:947129. doi: 10.3389/fpls.2022.947129
Received: 18 May 2022; Accepted: 23 June 2022;
Published: 08 July 2022.
Edited by:
Honghao Lv, Institute of Vegetables and Flowers (CAAS), ChinaReviewed by:
Seth Jordan O’Conner, University of North Carolina at Chapel Hill, United StatesJelli Venkatesh, Seoul National University, South Korea
Copyright © 2022 Jiang, Li, Dong, Zu, Zhan, Piao and Lang. This is an open-access article distributed under the terms of the Creative Commons Attribution License (CC BY). The use, distribution or reproduction in other forums is permitted, provided the original author(s) and the copyright owner(s) are credited and that the original publication in this journal is cited, in accordance with accepted academic practice. No use, distribution or reproduction is permitted which does not comply with these terms.
*Correspondence: Zhongyun Piao, enlwaWFvQHN5YXUuZWR1LmNu; Hong Lang, bGFuZ2hvbmdAamxua3UuZWR1LmNu
†These authors have contributed equally to this work