- 1Department of Biological Sciences, International Islamic University, Islamabad, Pakistan
- 2Department of Biology, College of Sciences, United Arab Emirates University, Al Ain, United Arab Emirates
- 3Department of Agriculture, University of Swabi, Swabi, Pakistan
- 4Department of Biotechnology, University of Science and Technology, Bannu, Pakistan
- 5Department of Food Science and Technology, The University of Agriculture, Peshawar, Pakistan
- 6College of Life Science, Linyi University, Linyi, China
- 7Tasmanian Institute of Agriculture, University of Tasmania, Burnie, TAS, Australia
- 8Department of Agricultural Extension Education & Communication, The University of Agriculture, Peshawar, Pakistan
- 9Department of Biological Sciences, Faculty of Science, King Abdulaziz University, Jeddah, Saudi Arabia
- 10Department of Biology, College of Science, Taif University, Taif, Saudi Arabia
- 11Department of Biology,, Faculty of Science, University of Tabuk, Tabuk, Saudi Arabia
- 12Department of Biology, College of Science, Imam Abdulrahman Bin Faisal University, Dammam, Saudi Arabia
- 13Hainan Key Laboratory for Sustainable Utilization of Tropical Bioresource, College of Tropical Crops, Hainan University, Haikou, China
- 14Department of Agronomy, The University of Haripur, Haripur, Pakistan
The development of food and forage crops that flourish under saline conditions may be a prospective avenue for mitigating the impacts of climate change, both allowing biomass production under conditions of water-deficit and potentially expanding land-use to hitherto non-arable zones. Here, we examine responses of the native halophytic shrub Atriplex leucoclada to salt and drought stress using a factorial design, with four levels of salinity and four drought intensities under the arid conditions. A. leucoclada plants exhibited morphological and physiological adaptation to salt and water stress which had little effect on survival or growth. Under low salinity stress, water stress decreased the root length of A. leucoclada; in contrast, under highly saline conditions root length increased. Plant tissue total nitrogen, phosphorus and potassium content decreased with increasing water stress under low salinity. As salt stress increased, detrimental effects of water deficit diminished. We found that both salt and water stress had increased Na+ and Cl– uptake, with both stresses having an additive and beneficial role in increasing ABA and proline content. We conclude that A. leucoclada accumulates high salt concentrations in its cellular vacuoles as a salinity resistance mechanism; this salt accumulation then becomes conducive to mitigation of water stress. Application of these mechanisms to other crops may improve tolerance and producitivity under salt and water stress, potentially improving food security.
Introduction
Climate change has increased the global mean temperature and water scarcity throughout the world (Zamin et al., 2019a). Demand for water supply is continuously increasing and had increased threefold since the 1950s as the freshwater supply has been on the wane (Gleick, 2003; Wu and Tan, 2012). According to an estimate, an average of 90% of global fresh water is being used for agriculture (Shiklomanov, 2000). According to the (FAO, 2005) report salinity had affected 800 Mha of land globally. It is expected that salinity will annually destroy about 10 Mha of agricultural land (Khan et al., 2006; Zamin et al., 2019b). Thus, the landscaping sector will face serious challenges, to meet irrigation requirements (Shahin and Salem, 2014a).
To solve the problems of water shortage and groundwater salinity many irrigation systems and much equipment is being introduced (Shahin and Salem, 2014b; Zamin and Khattak, 2018). Two types of approaches had been taken on so far to overcome these problems. The first is modifying the environment by managing the irrigation and drainage and the second approach is genetically modifying the plants to enhance their stress tolerance (Läuchli and Lüttge, 2002; Mahmood et al., 2003). However, stress tolerance responses of plants are complex and the functions of many genes controlling these mechanisms are unknown (Chaves et al., 2003). Halophytes had been recommended as a solution for production with salt/brackish water (Khan and Weber, 2006). Native halophyte plants can grow in harsh environmental conditions and can be introduced into urban landscaping under drought and saline conditions (Franco et al., 2006; Zamin and Khattak, 2017).
Increasing water shortage and irrigation water salinity are the main abiotic stresses for the plants. These stresses disturb plant physiology and growth by disrupting their gene expression (Wu and Tan, 2012; Zamin et al., 2018). Plants have evolved numerous mechanisms to adapt to salt stress conditions (Munns and Tester, 2008). Water stress-avoiding refers to a range of morphological and physiological adaptations of plants to sustain suitable water status. Another approach to withstand water stress is water stress tolerance which includes physiological and biochemical mechanisms (Clarke and Durley, 1981).
Atriplex leucoclada (English name: Orache; Arabic name: Ragal, رغل), a halophytic plant, is a low perennial shrub that has developed various strategies to adapt to saline environments with excessively high salt content in the soil. Originating in the Mediterranean basin, A. leucoclada grows in many different habitats, but it usually occurs on sabkha, coastal and inland salt marshes with a high accumulation of salts, and occasionally on silty soils. A. leucoclada is an important species for agricultural use in arid regions. Atriplex species can be planted for soil desalination and CO2 sequestration. It is a useful plant for desert and extensive landscape schemes as a groundcover, occasionally requiring watering and maintenance to improve its appearance (Arriyadh, 2014).
Studying A. leucoclada under stress conditions will help us to better understand the salt and water stress resistance mechanisms. Moreover, introducing the identified species in landscaping will not only save a huge amount of water but also preserve the biodiversity, wildlife habitats, horticulture heritage, and national unique landscape of the country.
Materials and methods
Research site
The field experiment was conducted at AL-Foa Research Farm, United Arab Emirates University, Al Ain, Abu Dhabi, United Arab Emirates (24°12′ N and 55° 44′ E) during December 2015–July 2016. The experimental site is situated in the arid region, having a long, hot summer season of 4 months, i.e., from May to September with maximum temperatures above 45°C. The winter prevails from mid-November to the end of February followed by a short spring season from March to April. The mean annual temperature varies between 12 and 45°C during the winter and summer seasons, respectively (SCAD, 2015). Soil type was classified as Typic Torriorthent sandy-skeletal hyperthermic soil and is silty loam having pH (H2O) of 8.6, ECe of 8.2 dS m–1 with SAR of 22.4 and 0.6% organic matter (Abdelfattah et al., 2009).
Experimental design
Atriplex leucoclada seeds were sown in germinating trays with growing media of potting soil and sweet sand (Red desert sand with low salinity used for agriculture) 1:1 by volume. The soil used in potting mix was sandy in nature having 24.53 percent carbonate content with pH of 7.58 and Ec 9.49. The Ca content of soil sample was 25 mg/kg whereas Mg was 34.2 mg/kg and low K content, i.e., 7.53 mg/kg (Table 1). After 3 weeks of germination, seedlings were transplanted to 20 cm pots filled with red desert sand. Seedlings were thinned to one seedling per pot. The fertilization and weeding practices were equally applied to all treatments during the entire growing period of the plants. After 2 months of growth, four saltwater treatments, i.e., 5 dS m–1 (Control; S1), 10 dS m–1 (low salinity level; S2), 15 dS m–1 (moderate salinity level; S3), and 20 dS m–1 (high salinity level; S4) were prepared by dissolving NaCl to fresh water, supplied by Al-Ain municipality (Al-Dakheel et al., 2015; Zamin et al., 2019a). Salinity treatments were prepared in four different water tanks. These water tanks were connected to a drip irrigation line to supply water to each pot individually with four irrigation intestines. To estimate field capacity the fully water-saturated soil was weighed and then dried to constant weight at 105°C. The weight difference between water-saturated and oven-dried soil was taken as the weight of water needed to bring soil to field capacity, and lower FC was calculated accordingly. Four irrigation intensities were: 100% field capacity (Control; WL1), 80% field capacity (low stress; WL2), 60% field capacity (moderate stress; WL3), and 40% field capacity (severe stress; WL4). Irrigation water was quantified for each water stress treatment and was applied to the plants through a drip irrigation system. The experiment was conducted in an open field and plants were grown under natural environmental conditions. The experiment was conducted in a randomized complete block design with a split-plot arrangement replicated three times. The salinity levels were allotted to the main plot while the irrigation intensities were allotted to sub-plots.
Harvesting and sampling
Three plants from each treatment were harvested at the end of each month. Data was recorded for morphological parameters each month. For the quantitative chemical analysis, representative specimens of each plant were instantly ground in liquid nitrogen and stored at −80°C.
Morphological traits
After harvest, the plant samples were carefully cleaned from sands, washed with distilled water, and dried with the help of tissue paper. After harvesting each plant was divided into shoots and roots and oven-dried (60°C) and weighted (± 0.0001 g). For morphological traits, all the samples were put in Ziploc bags, placed in an ice bag at 4°C, and transferred to the laboratory. Shoot length was measured from the base of the stem to the apex end while root length was measured from the root base up to the end of the primary root.
Physiological traits
Photosynthetic rate (P) of upper, lower, and basal leaves was measured weekly using a Plant Photosynthesis Meter (EARS, Netherlands) (Samarah, 2005). Leaf water potential was recorded during midday using a WP4C Dewpoint psychrometer (Decagon Devices, Inc., United States) (Xiong et al., 2014). Leaf water potential was recorded after 1 and 5 months of treatment application. Phosphorus concentrations were estimated in plant leaves at the end of the experiment by the methodology laid out by Olsen (1954). K and Na+ content of plant extracts were determined by Flame Emission Spectroscopy at the end of the experiment. For Cl– content 50 mg of leaf and root samples were ground and heated in distilled water for 3 h (80°C). The Cl– content of the extract was then determined with the chloride analyzer at the end of the experiment.
Biochemical traits
ABA and Proline extraction was performed on 10 mg of freeze-dried leaf tissue as described by Forcat et al. (2008). The samples were analyzed for ABA and Proline using LCMS/MS and were filtered through a 0.45 μm cellulose acetate syringe. The phytohormones separation was done using a C18 column (ZORBAX Eclipse Plus). An injection of 2 μl was loaded onto the C18 column (1.8 μm particle size, 2.1 mm inner diameter and 50 mm long) at a flow rate of 0.2 mL/min and the column temperature was kept at 35°C. The liquid chromatography was connected to an Agilent Technologies Mass Spectrometry (6420 Triple Quad detector). For elution solvent A consist of formic acid (0.1%) with distilled water and solvent B consisted of an LCMS grade acetonitrile was used. The analytical procedure was as follows: Solvent A was used (5 min), then the gradient from 0 to 100% solvent B was used, (5–20 min) after the solvent B was kept constant (5 min) and at 25.1 min solvent A was 100% was used for (30-min). During the analysis with LC-MSMS only negative polarity mode was used for ABA and Proline analysis. For fragmentation nitrogen gas was used. The capillary voltage was 4,000 V, the gas flow was 8 L/min, the gas temperature was 300°C and the nebulizer pressure was 45 psi.
Statistical analysis
Two-way (ANOVA) was used to check the effect of salinity and drought, and their interaction on morphological, biochemical, and physiological traits. The normality was checked with the Shapiro–Wilk test. The post-hoc Tukey’s HSD was used to check the comparison between treatments. All the analysis was performed using the SPSS software.
Results and discussion
Percent survival (%), root and shoot length (cm), and weight (g)
Results concerning the morphological response of A. leucoclada to varying salt and drought stresses are given in Table 2. Analysis of variance revealed that all the morphological parameters (percent survival, root and shoot length, and weight) did not show any significant (P > 0.05) effect from salt stress. It is clear from the results of survival percentage that A. leucoclada can survive on all studied salt and water stress levels. Analysis of variance revealed that different water stress levels significantly (P ≤ 0.05) affected Shoot dry weight (SDW), RDW, and SL of A. leucoclada. Increasing the water stress level decreased the SDW. The highest SDW was recorded for WL1 (18.15 g) while the other three water stress levels have lower SDW. Maximum RDW was measured for WL1 (3.72 g) while the other three water levels of WL2, WL3, and WL4 were significantly lower and like each other. Maximum SL was recorded for 44.37 cm for WL1 while other three water levels of WL2, WL3, and WL4 were significantly lower and similar to each other. Different salt and water stress levels and interaction of salt and water stress levels had a non-significant effect (P > 0.05) on the RL of A. leucoclada.
Photosynthetic rate (Pr) (μmol/m2/s), leaf water potential (MPa), Na+1 uptake (μ mole g–1), Cl–1 uptake (μ mole g–1)
Different salt and water stress levels had a significant (P ≤ 0.05) effect on the Pr of A. leucoclada (Table 3). Pr increased with increasing water stress while decreasing with increasing salt stress.
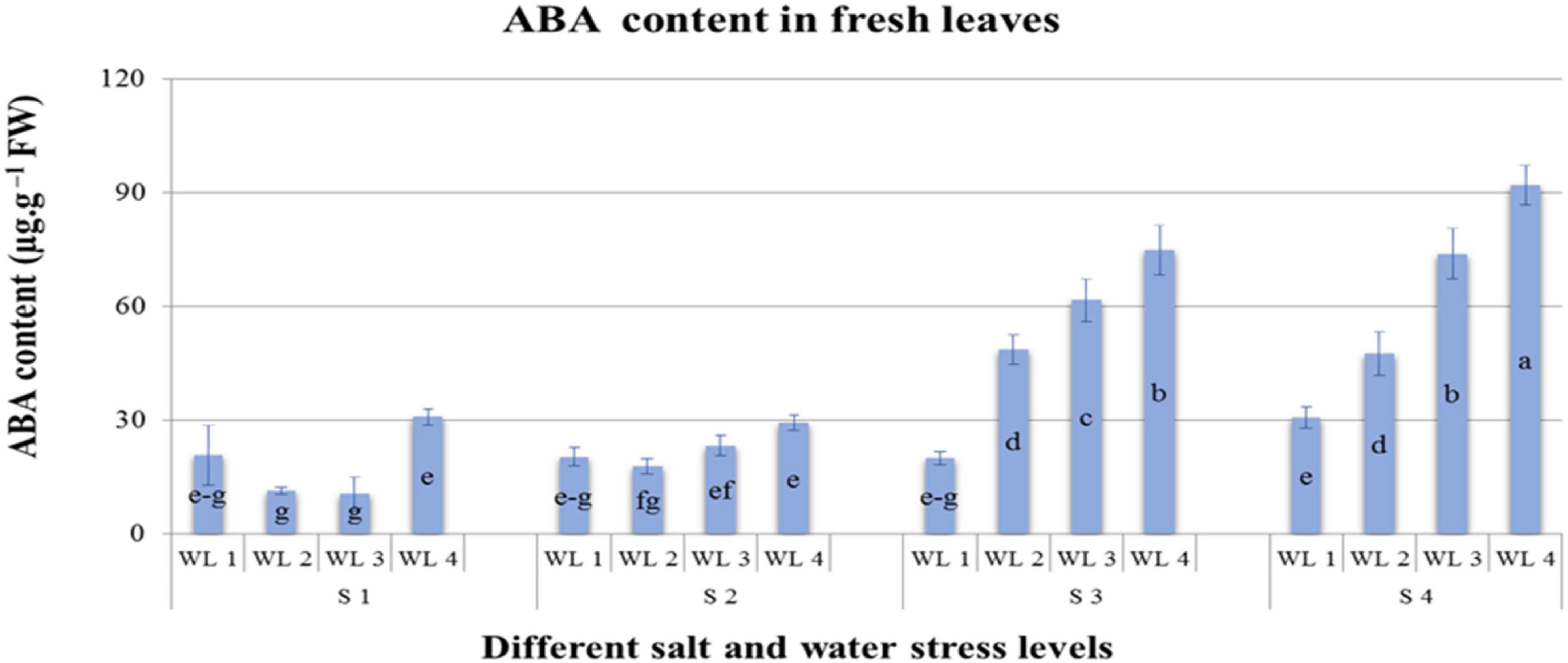
Figure 1. Interactive effect of salinity and drought stress on ABA content (μg. g–1 FW) content of Atriplex leucoclada. Error bars represent the standard error of the mean (n = 3). WL stands for water level (WL1 = 100% field capacity, WL2 = 80% field capacity, WL3 = 60% field capacity and WL4 = 40% field capacity) while S represents salinity (S1 = 5 dS m–1, S2 = 10 dS m–1, S3 = 15 dS m–1, and S4 = 20 dS m–1). Letters a–g indicate whether the bar graphs showing parameters are significant or nonsignificant. Bars having the same letters are nonsignificant.
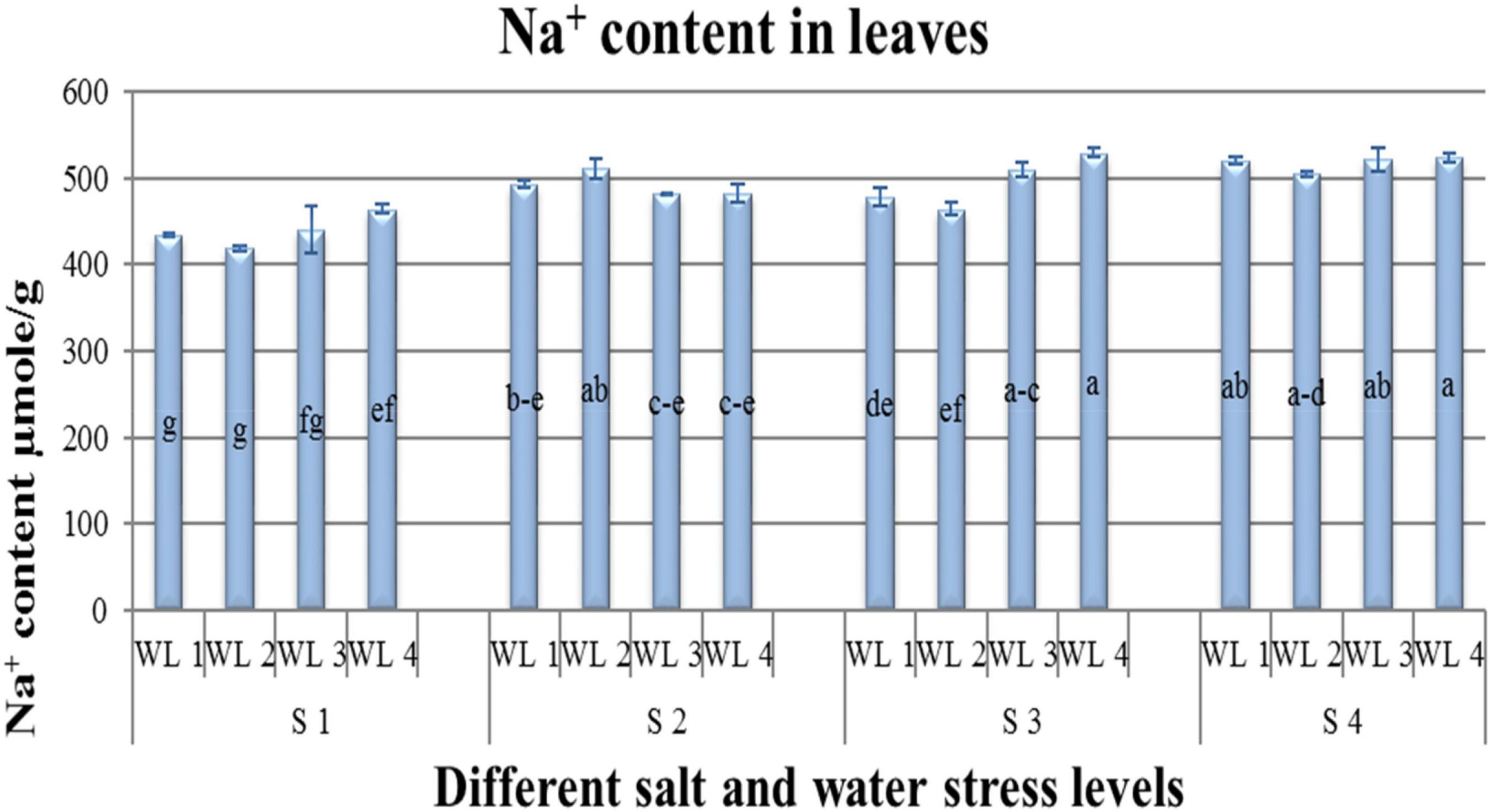
Figure 2. Interactive effect of salinity and drought stress on Na+ content of Atriplex leucoclada. The error bars represent the standard error of the mean (n = 3). WL stands for water level (WL1 = 100% field capacity, WL2 = 80% field capacity, WL3 = 60% field capacity and WL4 = 40% field capacity) while S represents salinity (S1 = 5 dS m–1, S2 = 10 dS m–1, S3 = 15 dS m–1, and S4 = 20 dS m–1). Letters a–g indicate whether the bar graphs showing parameters are significant or nonsignificant. Bars having the same letters are nonsignificant.
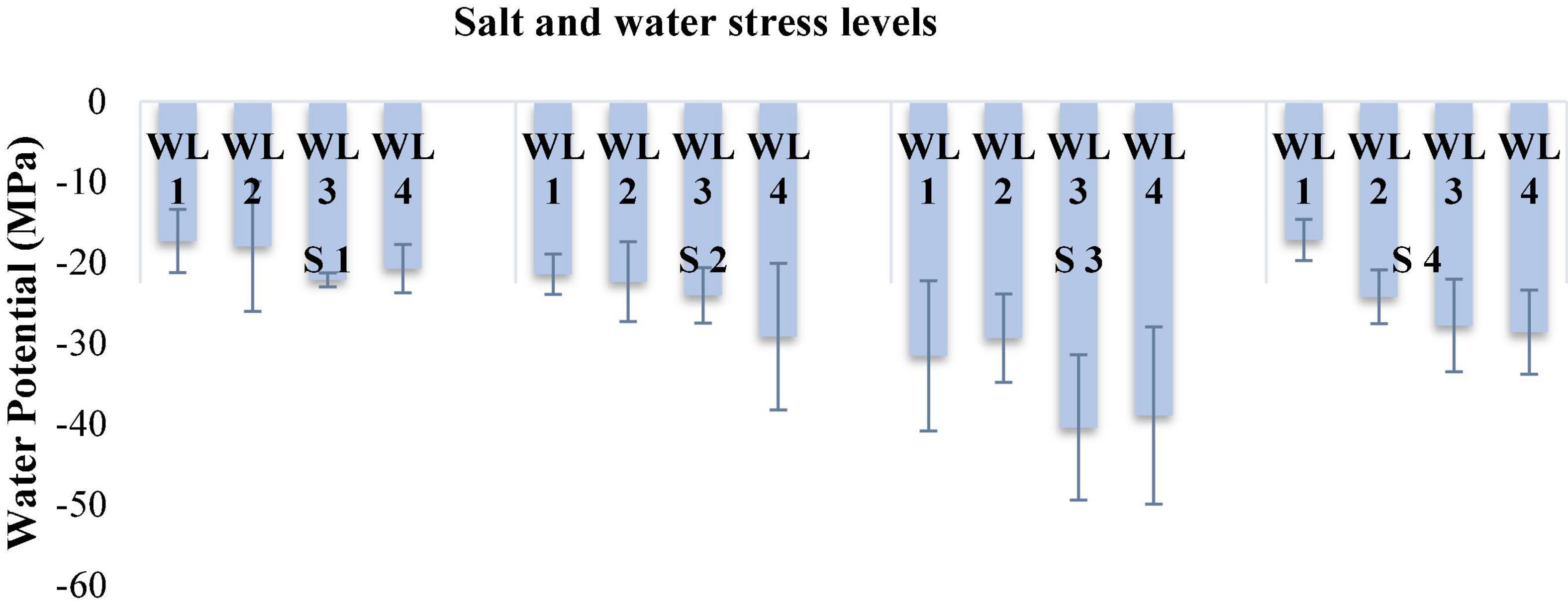
Figure 3. Interactive effect of salinity and drought stress on water potential (MPa) of Atriplex leucoclada. The error bars represent the standard error of the mean (n = 3). WL stands for water level (WL1 = 100% field capacity, WL2 = 80% field capacity, WL3 = 60% field capacity and WL4 = 40% field capacity) while S represents salinity (S1 = 5 dS m–1, S2 = 10 dS m–1, S3 = 15 dS m–1, and S4 = 20 dS m–1).
Salt × water stress had a significant effect (P ≤ 0.05) on the leaf water potential of A. leucoclada. After months of treatment application, S2WL1 had a maximum LWP of −46 MPa, and a minimum LWP (−64 MPa) was recorded for S1WL2 Table 3 and Figure 1.
Na+ and Cl– content had an interactive effect (P ≤ 0.05) on salt and water stress. Na+ and Cl– content increased not only with increasing salt stress but also with increasing water stress. Na+ was lower in S1WL2 and S1WL1 and increased with increasing salinity and water stress. Maximum Na+ content was reported for S3WL4 and S4WL4 (Figure 2). Similarly, the lowest Cl– content was recorded for S1WL1 and S1WL2. The highest Cl– content was recorded for S4WL1 (Table 3 and Figure 3).
Abscisic acid (μg. g–1 FW) and proline content (μg. g–1 FW)
Means of ABA quantified are represented in Table 4 and Figure 4. Salt and water stress levels had statistically significant (P ≤ 0.05) interaction for ABA production. ABA production showed an increasing trend with increasing both salt and water stress levels. Maximum ABA content was 92 μg. g–1 FW quantified at S4WL4. ANOVA revealed that different salt and water stress levels and their interaction had a significant effect on the proline content of A. leucoclada. Proline content increased with increasing the combined effect of salt and water stress and maximum proline content was 16,654 μg–1 FW recorded for S4WL4 (Table 4 and Figure 5).
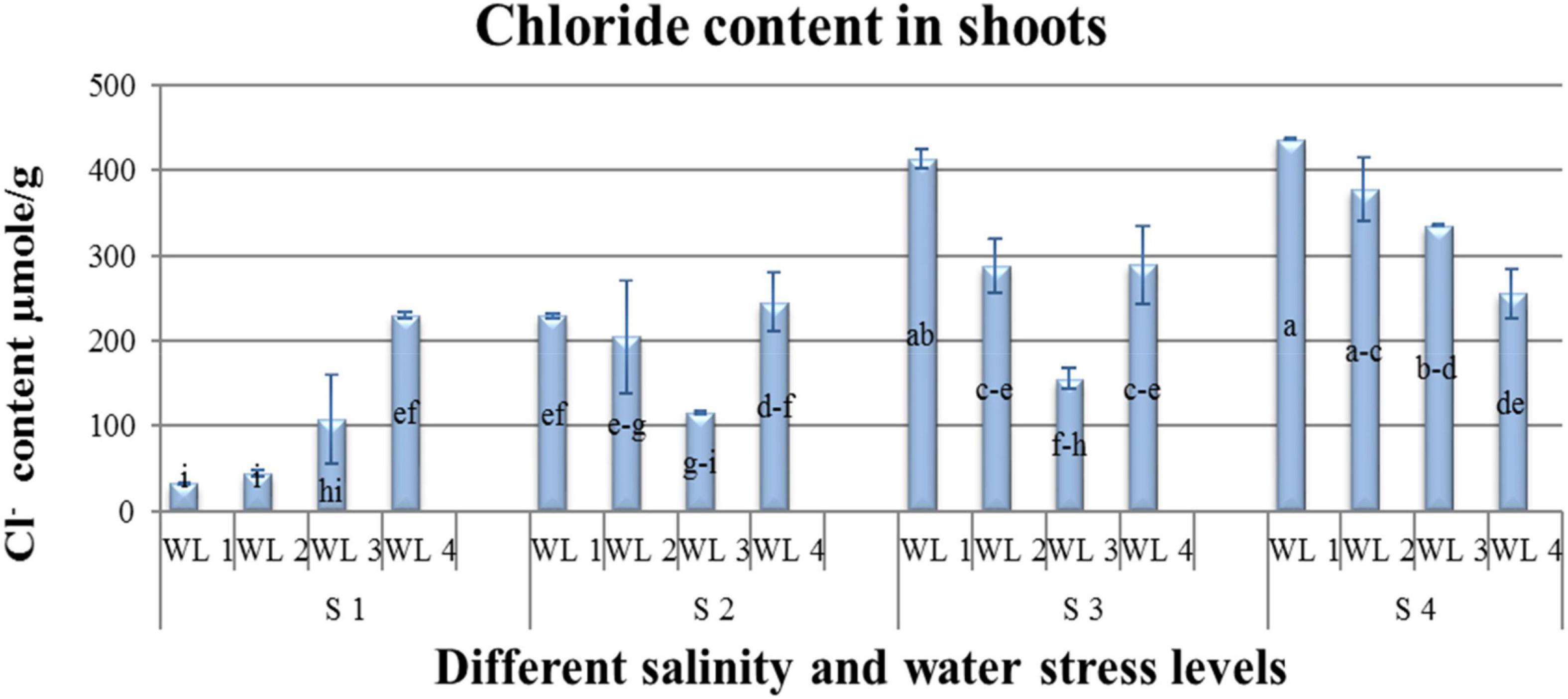
Figure 4. Interactive effect of salinity and drought stress on Cl– content of Atriplex leucoclada. Error bars represent the standard error of the mean (n = 3). WL stands for water level (WL1 = 100% field capacity, WL2 = 80% field capacity, WL3 = 60% field capacity and WL4 = 40% field capacity) while S represents salinity (S1 = 5 dS m–1, S2 = 10 dS m–1, S3 = 15 dS m–1, and S4 = 20 dS m–1). Letters a-i indicate whether the bar graphs showing parameters are significant or nonsignificant. Bars having the same letters are nonsignificant.
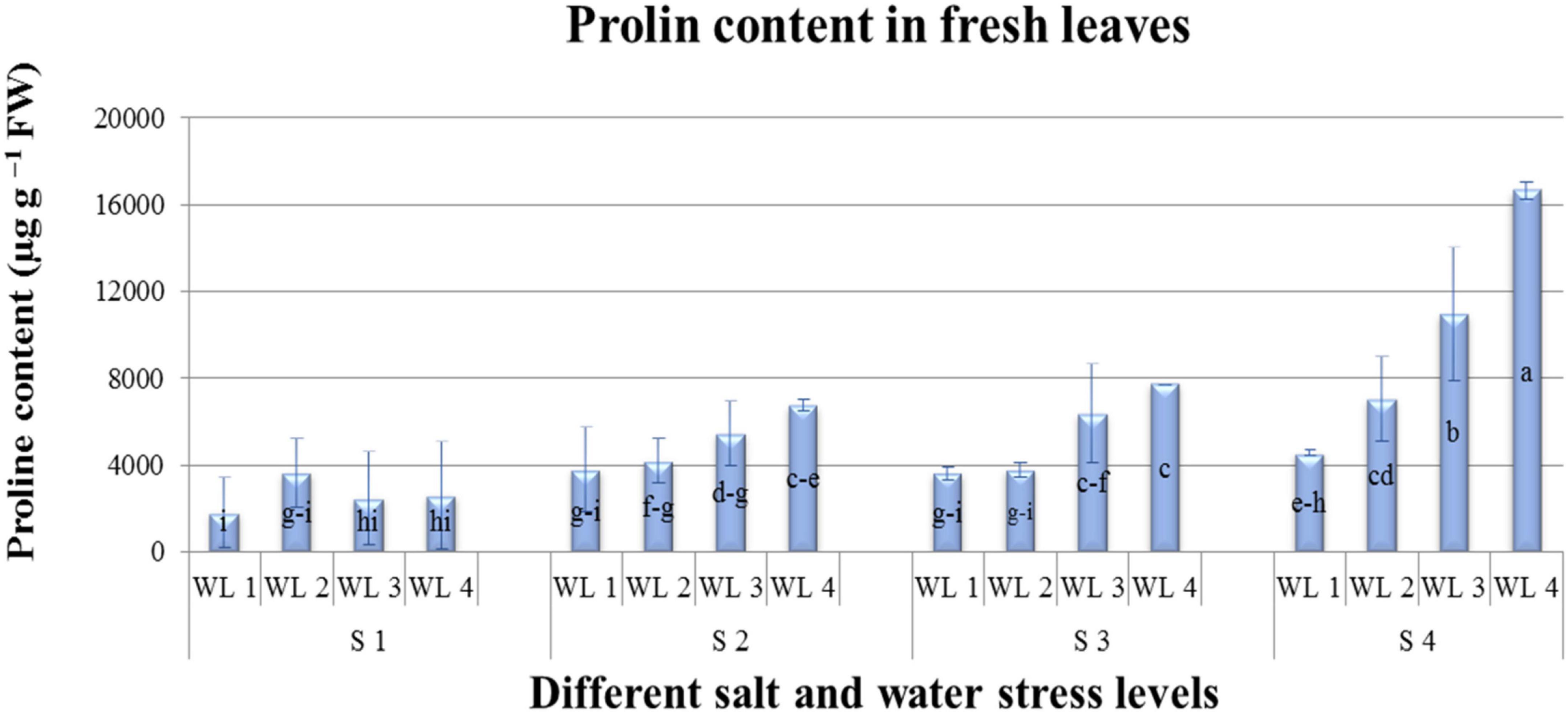
Figure 5. Interactive effect of salinity and drought stress on Proline content (μgg–1FW) content of Atriplex leucoclada. Error bars represent standard error of the mean (n = 3). WL stands for water level (WL1 = 100% field capacity, WL2 = 80% field capacity, WL3 = 60% field capacity and WL4 = 40% field capacity) while S represents salinity (S1 = 5 dS m–1, S2 = 10 dS m–1, S3 = 15 dS m–1, and S4 = 20 dS m–1. Letters a–i indicate whether the bar graphs showing parameters are significant or nonsignificant. Bars having the same letters are nonsignificant.
Discussion
Percent survival (%), root and shoot length (cm), and weight (g)
Salt and water stress were mostly studied separately for their effects on crop growth (Hamed et al., 2013). Hence only a few studies had examined their interactions (Hamed et al., 2013). A. leucoclada has been found to be resistant to salt and water stress and survived under all salt and water stresses applied. Salt stress had no significant effect on the growth of A. leucoclada. On the other hand, increasing water stress had shown a major decrease in growth parameters except for root length (Table 2). Similar results for reduced growth under salt stress as compared to water stress are also resorted by Hassine and Lutts (2010) for Atriplex halimus, Melo et al. (2016) for Atriplex nummularia and Almas et al. (2013) in Artemisia vulgaris and Álvarez et al. (2018) for Pistacia lentiscus. The same is reported for A. canescens (Glenn and Brown, 1998), A. lentiformis (Meinzer and Zhu, 1999), A. halimus (Alla et al., 2011), and Anethum graveolens (Tsamaidi et al., 2017). Result similar to our experiment for decreased RDW under water stress as compared to salt stress are reported by Wang et al. (2011) for tamarisk (Tamarix chinensis Lour), Yagmur and Kaydan (2008) for triticale (Triticosecale Witm., cv. Presto), Khan et al. (2017) for soybean and, Miranda-Apodaca et al. (2018) for Quinoa (Chenopodium quinoa).
Although soil water potential is decreased by saline water irrigation water flow to the roots remains the same. On the other hand, water stress decreases the soil matric potential and decreases water flow to the roots (Homaee et al., 2002). This can be the reason that the matric potential during water stress affected the shoot growth of studied species more than that did the osmotic potential (Shainberg and Shalhevet, 2012). These results corroborate with the results of Maggio et al. (2005) who reported 22% less aboveground dry weight in salt stress and 46% less dry weight in water stress than control.
Photosynthetic rate (μmol/m2/s), leaf water potential (MPa)
Both salt and water stress had an interactive effect (P ≤ 0.05) on Pr of A. leucoclada. Pr was maximum during the cooler month of March and start decreasing with increasing temperature in the months after that. Pr was significantly affected by salt x water stress. Salt stress decreased the Pr while water stress increased the Pr significantly. The current results are in line with Wang et al. (2011) for Tamarix chinensis. Water deficit led to earlier peaks of net photosynthetic rate (PN) during the day. In the case of quinoa, the highest salt concentration of (500 mM) decreased net Pr by 65% compared to controls. However, water stress resulted in 77% lower values for net Pr (Miranda-Apodaca et al., 2018).
Under water stress, leaf water potential and thus photosynthetic activity is decreased (Razzaghi et al., 2011). This reduction in photosynthesis can be caused by stomatal closure (Goldstein et al., 1996), disturbance of photosynthetic activity (Drew et al., 1990), or at both low and high salt concentrations (Yeo et al., 1991). Decreasing soil moisture content and water potential reduces the water potential of the plant tissue. In response to low water potential additional solutes are accumulated which is referred to as osmotic adjustment (OA) (Zhang et al., 1999; Verslues et al., 2006). In halophyte species, Na+ is involved in OA. It is supposed that Na+ largely exists in the vacuoles (Martínez et al., 2005).
Salt and water stress had an additive effect on LWP and leaf osmotic potential reduction of Zygophyllum xanthoxylum (Ma et al., 2012). Miranda-Apodaca et al. (2018) compared the effect of osmotic and ionic stress on quinoa. It was reported that plants subjected to saline treatment observed a greater capacity for osmotic adjustment. In contrast, plants subjected to water stress treatment showed more dehydration. The water potential diminished significantly due to salt and water stress (González et al., 2012). Álvarez et al. (2018) reported decreased water potential for all salt and water stress treatments in Pistacia lentiscus.
It was concluded that osmotic adjustment through the uptake of readily available inorganic ions (Na+ and Cl–) under salt stress is more efficient than adjustment through the production of organic solutes under water stress (Liu et al., 2008; Slama et al., 2008; Sucre and Suarez, 2011; Álvarez et al., 2012).
Herralde et al. (1998) submitted plants of Argyranthemum coronopifolium to salt and water stress independently. Water stress promoted significant differences in leaf water potential (−1.76 MPa for Ψw) in stressed plants vs. control.
Hassine et al. (2008) exposed Atriplex halimus plants to 40/160 mM NaCl or 15% polyethylene glycol. Shoot water potential in plants exposed to PEG remained lower than the plants under the highest salt stress. Duarte and Souza (2016) investigated water potentials in Capsicum annuum by irrigating with different levels of saline water. Salt stress resulted in a decrease in LWP. The decrease in the osmotic potential in plant leaves was a mean of saline stress adoption.
Our results are in agreement with the findings of Omami and Hammes (2006) in amaranth under salt and water stress, Mannan et al. (2013) for soybean, and Khan et al. (2015) for mung bean under salinity stress. It can be concluded that salt stress can help to reduce the negative effects of water stress by osmotic adjustment through Na+ and proline accumulation.
Na+1 uptake (μ mole g–1), Cl–1 uptake (μ mole g–1)
Under saline conditions, Na+ in the growth medium might compete with K absorption by the roots (Blumwald, 2000). It is assumed that K uptake and its deposition in tissues by the plant is reduced under salt stress (Lazof and Bernstein, 1999). This reduced potassium concentration in plant tissues grown under salt stress conditions is reported by many authors (Hu and Schmidhalter, 1997; Khan et al., 2000; García et al., 2004; Carter et al., 2005). This decrease is attributed to the antagonistic effects of Na+ and K ions (Suhayda et al., 1990).
The present study revealed a significant interactive effect of salt and water stress on Na+ and Cl– uptake. Increasing salt stress increased Na+ and Cl– uptake. Water stress also increased the Cl– uptake even at low salinity stress. Cl– uptake increased with increasing water stress at the lowest salinity level S1 only. Under the highest salinity level of S4 Cl– uptake decreased with increasing water stress levels.
An important “salt includer,” Jojoba also accumulated significant amounts of sodium under slat stress (Mills and Benzioni, 1992). Na+ content in shoots increased sharply across the salt levels in Atriplex canescens (Glenn and Brown, 1998) Salicornia rubra (Khan et al., 2001) Bruguiera cylindrica (Atreya et al., 2009) sea aster (Aster tripolium L) (Ueda et al., 2003), jojoba explants (Roussos et al., 2007), Atriplex halimus (Martínez et al., 2005), and S. portulacastrum (Slama et al., 2008).
Halophytic species Salicornia rubra was studied by Khan et al. (2001). Chloride concentration in shoots increased with increasing irrigation water salinity. Another halophyte Aster tripolium L was evaluated by Ueda et al. (2003) under water stress and NaCl (300 mM) stress. Cl– content increased three times and Na+ content increased up to five times in the NaCl-stressed leaves that of the control. Similarly, Sesuvium portulacastrum and Arthrocnemum macrostachyum also reported Na+ and Cl– compartmentalization (Messedi et al., 2004; Khan et al., 2005).
Under salt stress osmotic adjustment is achieved through increased Na+ and Cl– uptake. The production of organic osmotica is more energy-consuming (Greenway and Munns, 1980). Thus inorganic ion accumulation is an alternative mechanism to adjust osmotic potential and seems to save energy, which enables a plant to grow in less favorable conditions (Khalid and Cai, 2011). The shoot acts as a sink for Na+ ions when plants were grown under salt stress (Jefferies et al., 1979). Cells are able to avoid high levels of salts in the cytoplasm and achieve osmoregulation by increasing salt levels in the vacuoles by intracellular compartmentalization (Khan et al., 2000, 2005).
Abscisic acid (μg. g–1 FW) and proline content (μg. g–1 FW)
Kefu et al. (1991) stated saltbush (Atriplex spongiosa) plants did not increase ABA content on 75 mol m–3 NaCI salinity but increased at 150 mol m–3. Hassine and Lutts (2010) exposed Atriplex halimus plants to iso-osmotic stress of NaCl (160 mM) or PEG (15%). Hassine and Lutts (2010) reported that ABA accumulated in response to salt (160 mM NaCl). Alla et al. (2011) while studying A. halimus responses for salt (NaCl) or water stress (PEG) found that salt stress produced more metabolic disturbance than water stress. Razzaghi et al. (2011) observed an increase in ABA with increasing water stress.
These ABA-induced stress responses are important for plant survival during both salt and water stress but affect different physiological processes (Hassine et al., 2009). ABA stimulates Na+ and Cl– excretion by external salt bladders under salt stress and reduces water loss during water stress (Hassine and Lutts, 2010; Walker and Lutts, 2014). ABA acts as a major signal to regulate transpiration through stomatal pores (Schroeder et al., 2001; Bartels and Sunkar, 2005). ABA-regulated stomatal opening, root growth, and conductance (Schroeder et al., 2001; Sharp and LeNoble, 2002) are important in the avoidance of low water potential. ABA-induced increase of compatible solutes is important for drought avoidance (Ober and Sharp, 1994). The relative root and shoot growth is a response to water stress (Hsiao and Xu, 2000) and is the result of regulation of growth by ABA (Sharp and LeNoble, 2002).
This proline accumulation is involved in osmotic adjustment and protects cellular structures against salt stress and ROS (Hoque et al., 2007). Proline is one of the prominent organic solutes that are stored in the cytoplasm and organelles to balance the osmotic pressure of the ions in the vacuole under stress conditions (Hasegawa et al., 2000). Proline accumulation relates more to osmotic stress than any specific salt effect (Munns, 2002). Proline accumulation is a preventive metabolic adaptation that act as osmoprotectants and antioxidants and/or free radical scavengers (Larher et al., 2009).
Khalid and Cai (2011) reported M. officinalis response to proline accumulation by applying various levels of salt and water stress. The highest proline content resulted from combined application of salt and water stress. The same results were reported by Watanabe et al. (2000) and Wu et al. (2015). Teymouri et al. (2009) reported similar results studying three halophytic salsola species (S. rigida, S. dendroides, and S. richteri). The maximum increase in proline concentration under salt stress was recorded for S. richteri. Atriplex spongiosa had a similar trend of decreasing proline content in the range of 50–300 mol/m3 but increased rapidly at higher salinities (Storey and Jones, 1979). The same is the case for Suaeda monoica, low proline contents were recorded at 500 mol/m3 NaCl and below. However, a significant increase was detected at high salinities (Storey and Jones, 1979). Martínez et al. (2005) reported the same results as that of our experiment. He reported that 0 or 15% PEG had no impact on the proline concentration at low NaCl (50 mM) concentration. Atriplex halimus showed similar responses after treating seedlings with either NaCl (50, 300, and 550 mM NaCl) or drought (control and withholding water) (Alla et al., 2012). Similar responses of proline to salinity (Bajji et al., 1998) and to osmotic stress (Martinez et al., 2003) had been reported. This can be concluded that proline is efficiently only involved in stress tolerance within the first few hours of stress rather than in long-term stress tolerance (Hassine et al., 2008).
Conclusion
Atriplex leucoclada, showed morphological and physiological adaptations to both salt and water stress and had no negative effect of these stresses on survival percentage. A. leucoclada could be classified as obligatory halophyte. A. leucoclada resisted maximum salt stress with no significant effect on growth parameters. However, drought stress significantly decreased the growth of A. leucoclada. Na+ and Cl– content increased not only with increasing salt stress but also with increasing water stress. Cl– uptake in A. leucoclada increased with increasing water stress at lowest salinity level S1 only. Both salt and water stress had an additive role in increasing ABA and proline content. Higher salt stress levels increased proline content with increasing water stress levels.
In conclusion, A. leucoclada used salt-resistant mechanism to accumulate higher concentrations of salts in the cells. They use physiological adaptation using enzymatic and non-enzymatic mechanisms to cope with the negative impacts of higher salt stress and ROS (Reactive Oxygen Species) produced. A. leucoclada could be recommended for production as an alternate for landscape plants and as a fodder crop in areas with salt or brackish water. This will not only save limited available fresh water resources, but also bring more land under cultivation.
Data availability statement
The raw data supporting the conclusions of this article will be made available by the authors, without undue reservation.
Author contributions
HA and MZ: conceptualization. MA, NA, TN, AB, SS, and SH: methodology and formal analysis. SF, HFA, and YA: writing – original draft preparation. KL, MH, and SF: writing – review and editing. MZ: supervision. SA, BMA, AM, BA, and NMA: funding acquisition. All authors contributed to the article and approved the submitted version.
Conflict of Interest
The authors declare that the research was conducted in the absence of any commercial or financial relationships that could be construed as a potential conflict of interest.
Publisher’s Note
All claims expressed in this article are solely those of the authors and do not necessarily represent those of their affiliated organizations, or those of the publisher, the editors and the reviewers. Any product that may be evaluated in this article, or claim that may be made by its manufacturer, is not guaranteed or endorsed by the publisher.
References
Abdelfattah, M. A., Dawoud, M. A. H., and Shahid, S. A. (2009). Soil and Water Management for Combating Desertification–towards Implementation of the United Nations Convention to Combat Desertification from the UAE Perspectives. Riga: International conference Soil Degradation.
Al-Dakheel, A. J., Hussain, M. I., and Rahman, A. Q. M. A. (2015). Impact of irrigation water salinity on agronomical and quality attributes of Cenchrus ciliaris L. accessions. Agric. Water Manag. 159, 148–154. doi: 10.1016/j.agwat.2015.06.014
Alla, M. M. N., Khedr, A. H. A., Serag, M. M., Abu-Alnaga, A. Z., and Nada, R. M. (2012). Regulation of metabolomics in Atriplex halimus growth under salt and drought stress. Plant Growth Regul. 67, 281–304. doi: 10.1007/s10725-012-9687-1
Alla, M. M. N., Khedr, A. H. A., Serag, M. M., Abu-Alnaga, A. Z., and Nada, R. M. (2011). Physiological aspects of tolerance in Atriplexhalimus L. to NaCl and drought. Acta Physiol. Plant 33, 547–557. doi: 10.1007/s11738-010-0578-7
Almas, D. E., Bagherikia, S., and Mashaki, K. M. (2013). Effects of Salt and Water Stresses on Germination and Seedling Growth of Artemisia vulgaris L. Int. J. Agric. Crop Sci. 6:762.
Álvarez, S., Gómez-Bellot, M. J., Castillo, M., Bañón, S., and Sánchez-Blanco, M. J. (2012). Osmotic and saline effect on growth, water relations, and ion uptake and translocation in Phlomis purpurea plants. Environ. Exp. Bot. 78, 138–145. doi: 10.1016/j.envexpbot.2011.12.035
Álvarez, S., Rodríguez, P., Broetto, F., and Sánchez-Blanco, M. J. (2018). Long term responses and adaptive strategies of Pistacia lentiscus under moderate and severe deficit irrigation and salinity: osmotic and elastic adjustment, growth, ion uptake and photosynthetic activity. Agric. Water Manag. 202, 253–262. doi: 10.1016/j.agwat.2018.01.006
Arriyadh, H. C. F. T. D. O. (2014). Landscape Plants for Arriyadh Region: A Reference Manual. Riyadh: King Fahd Natnional Library Cataloging-in-Publication Data
Atreya, A., Vartak, V., and Bhargava, S. (2009). Salt priming improves tolerance to dessication stress and to extreme salt stress in Bruguiera cylindrica. Int. J. Integr. Biol. 6, 68–73.
Bajji, M., Kinet, J. M., and Lutts, S. (1998). Salt stress effects on roots and leaves of Atriplex halimus L. and their corresponding callus cultures. Plant Sci. 137, 131–142. doi: 10.1016/S0168-9452(98)00116-2
Bartels, D., and Sunkar, R. (2005). Drought and Salt Tolerance in Plants. Crit. Rev. Plant Sci. 24, 23–58. doi: 10.1080/07352680590910410
Blumwald, E. (2000). Sodium transport and salt tolerance in plants. Curr. Opin. Cell Biol. 12, 431–434. doi: 10.1016/s0955-0674(00)00112-5
Carter, C. T., Grieve, C. M., Poss, J. A., and Suarez, D. L. (2005). Production and ion uptake of Celosia argentea irrigated with saline wastewaters. Sci. Hort. 106, 381–394. doi: 10.1016/j.scienta.2005.04.007
Clarke, J. M., and Durley, R. C. (1981). “The responses of plants to drought stress,” in Water Stress on Plants, ed. G. M. Simpson (New York: Praeger), 89–139
Chaves, M.M., Maroco, J.P., and Pereira, J.S. (2003). Understanding plant responses to drought—From genes to the whole plant. Funct. Plant Biol. 30: 239–264.
Drew, M. C., Hold, P. S., and Picchioni, G. A. (1990). Inhibition by NaCl of net CO2 fixation and yield of cucumber. J. Am. Soc. Hortic. Sci. 115, 472–477. doi: 10.21273/jashs.115.3.472
Duarte, H. H. F., and Souza, E. R. de. (2016). Soil water potentials and Capsicum annuum L. under salinity. Rev. Bras. de Ciênc Do Solo 40, 1–11. doi: 10.1590/18069657rbcs20150220
FAO (2005). Global Metwork on Integrated Soil Management for Sustainable use of Salt-affected Soils. FAO Land and Plant Nutricion Management Services. Rome: Food and Agriculture Organization.
Forcat, S., Mark, H. B., John, W. M., and Murray, R. G. (2008). A rapid and robust method for simultaneously measuring changes in the phytohormones ABA, JA and SA in plants following biotic and abiotic stress. Plant Methods 4:16. doi: 10.1186/1746-4811-4-16
Franco, J. A., Martínez-Sánchez, J. J., Fernández, J. A., and Bañón, S. (2006). Selection and nursery production of ornamental plants for landscaping and xerogardening in semi-arid environments. J. Hortic. Sci. Biotechnol. 81, 3–17. doi: 10.1080/14620316.2006.11512022
García, N. F., Martínez, V., and Carvajal, M. (2004). Effect of salinity on growth, mineral composition, and water relations of grafted tomato plants. J. Plant Nutr. Soil Sci. 167, 616–622. doi: 10.1002/jpln.200420416
Gleick, P. H. (2003). Global freshwater resources: soft-path solutions for the 21st century. Science 302, 1524–1528. doi: 10.1126/science.1089967
Glenn, E. P., and Brown, J. J. (1998). Effects of soil salt levels on the growth and water use efficiency of Atriplex canescens (Chenopodiaceae) varieties in drying soil. Am. J. Bot. 85, 10–16. doi: 10.2307/2446548
Goldstein, G., Drake, D. R., Alpha, C., Melcher, P., Heraux, J., and Azocar, A. (1996). Growth and photosynthetic responses of Scaevola sericea, a Hawaiian coastal shrub, to substrate salinity and salt spray. Int. J Plant Sci. 157, 171–179. doi: 10.1086/297336
González, A., Tezara, W., Rengifo, E., and Herrera, A. (2012). Ecophysiological responses to drought and salinity in the cosmopolitan invader Nicotiana glauca. Braz. J. Plant Physiol. 24, 213–222. doi: 10.1590/s1677-04202012000300008
Greenway, H., and Munns, R. (1980). Mechanisms of salt tolerance in nonhalophytes. Annu. Rev. Plant Physiol. 31, 149–190. doi: 10.1146/annurev.pp.31.060180.001053
Hamed, K. B., Ellouzi, H., Talbi, O. Z., Hessini, K., Slama, I., Ghnaya, T., et al. (2013). Physiological response of halophytes to multiple stresses. Funct. Plant Biol. 40, 883–896. doi: 10.1071/fp13074
Hasegawa, P. M., Bressan, R. A., Zhu, J. K., and Bohnert, H. J. (2000). Plant cellular and molecular responses to high salinity. Annu. Rev. Plant Biol. 51, 463–499. doi: 10.1146/annurev.arplant.51.1.463
Hassine, A. B., and Lutts, S. (2010). Differential responses of saltbush Atriplex halimus L. exposed to salinity and water stress in relation to senescing hormones abscisic acid and ethylene. J. Plant Physiol. 167, 1448–1456. doi: 10.1016/j.jplph.2010.05.017
Hassine, A. B., Ghanem, M. E., Bouzid, S., and Lutts, S. (2008). An inland and a coastal population of the Mediterranean xero-halophyte species Atriplex halimus L. differ in their ability to accumulate proline and glycinebetaine in response to salinity and water stress. Annu. Rev. Plant Biol. 59, 1315–1326. doi: 10.1093/jxb/ern040
Hassine, A. B., Ghanem, M. E., Bouzid, S., and Lutts, S. (2009). Abscisic acid has contrasting effects on salt excretion and polyamine concentrations of an inland and a coastal population of the Mediterranean xero-halophyte species Atriplex halimus. Ann. Bot. 104, 925–936. doi: 10.1093/aob/mcp174
Herralde, F., Biel, C., Savé, R., Morales, M. A., Torrecillas, A., Alarcón, J. J., et al. (1998). Effect of water and salt stresses on the growth, gas exchange and water relations in Argyranthemum coronopifolium plants. Plant Sci. 139, 9–17. doi: 10.1016/S0168-9452(98)00174-5
Homaee, M., Feddes, R. A., and Dirksen, C. (2002). A Macroscopic Water Extraction Model for Nonuniform Transient Salinity and Water Stress. Soil Sci. Soc. Am. J. 66, 1764–1772. doi: 10.2136/sssaj2002.1764
Hoque, M. A., Okuma, E., Banu, M. N. A., Nakamura, Y., Shimoishi, Y., and Murata, Y. (2007). Exogenous proline mitigates the detrimental effects of salt stress more than exogenous betaine by increasing antioxidant enzyme activities. J. Plant Physiol. 164, 553–561. doi: 10.1016/j.jplph.2006.03.010
Hsiao, T. C., and Xu, L. K. (2000). Sensitivity of growth of roots versus leaves to water stress: biophysical analysis and relation to water transport. J. Exp. Bot. 51, 1595–1616. doi: 10.1093/jexbot/51.350.1595
Hu, Y., and Schmidhalter, U. (1997). Interactive effects of salinity and macronutrient level on wheat II Composition. J. Plant Nutr. 20, 1169–1182. doi: 10.1080/01904169709365325
Jefferies, R. L., Rudmik, T., and Dillon, E. M. (1979). Responses of halophytes to high salinities and low water potentials. Plant Physiol. 64, 989–994. doi: 10.1104/pp.64.6.989
Kefu, Z., Munns, R., and King, R. W. (1991). Abscisic acid levels in NaCl-treated barley, cotton and saltbush.Funct. Plant Biol. 18, 17–24. doi: 10.1071/pp9910017
Khalid, K. A., and Cai, W. (2011). The effects of mannitol and salinity stresses on growth and biochemical accumulations in lemon balm. Acta Ecol. Sin. 31, 112–120. doi: 10.1016/j.chnaes.2011.01.001
Khan, M. A., and Weber, D. J. (2006). Ecophysiology of High Salinity Tolerant Plants. Germany: Springer Science Business Media 40, 11–30. doi: 10.1007/1-4020-4018-0
Khan, M. A., Gul, B., and Weber, D. J. (2001). Effect of salinity on the growth and ion content of Salicornia rubra. Commun. Soil Sci. Plant Anal. 32, 2965–2977. doi: 10.1081/css-120000975
Khan, M. A., Ungar, I. A., and Showalter, A. M. (2000). Effects of salinity on growth, water relations and ion accumulation of the subtropical perennial halophyte Atriplex griffithii var. Stocksii. Ann. Bot. 85, 225–232. doi: 10.1006/anbo.1999.1022
Khan, M. A., Ungar, I. A., and Showalter, A. M. (2005). Salt stimulation and tolerance in an intertidal stem-succulent halophyte. J. Plant Nutr. 28, 1365–1374. doi: 10.1081/pln-200067462
Khan, M. S. A., Chowdhury, J. A., Razzaque, M. A., Ali, M. Z., Paul, S. K., and Aziz, M. A. (2017). Dry Matter Production and Seed Yield of Soybean as Affected by Post-Flowering Salinity and Water Stress. Bangladesh Agron. J. 19, 21–27. doi: 10.3329/baj.v19i2.31849
Khan, Md. S. A., Karim, M. A., Mahmud, A. A., Parveen, S., Bazzaz, Md. M., and Hossain, Md. A. (2015). Plant water relations and proline accumulations in soybean under salt and water stress environment. J. Plant Sci. 3, 272–278.
Khan, S., Tariq, R., Yuanlai, C., and Blackwell, J. (2006). Can irrigation be sustainable? Agric. Water Manag. 80, 87–99. doi: 10.1016/j.agwat.2005.07.006
Larher, F. R., Lugan, R., Gagneul, D., Guyot, S., Monnier, C., Lespinasse, Y., et al. (2009). A reassessment of the prevalent organic solutes constitutively accumulated and potentially involved in osmotic adjustment in pear leaves. Environ. Exp. Bot. 66, 230–241. doi: 10.1016/j.envexpbot.2009.02.005
Läuchli, A., and Lüttge, U. (2002). “Global Impact of Salinity and Agricultural Ecosystems,” in Salinity: Environment - Plants - Molecules, eds M. G. Pitman and A. Läuchli (New York, N Y: Springer).
Lazof, D. B., and Bernstein, N. (1999). Effects of salinization on nutrient transport to lettuce leaves: consideration of leaf developmental stage. New Phytol. 144, 85–94. doi: 10.1046/j.1469-8137.1999.00487.x
Liu, X., Huang, W., Niu, Z., Mori, S., and Tadano, T. (2008). Interactive effect of moisture levels and salinity levels of soil on the growth and ion relations of halophyte. Commun. Soil Sci. Plant Anal. 39, 741–752. doi: 10.1080/00103620701879448
Ma, Q., Yue, L. J., Zhang, J. L., Wu, G. Q., Bao, A. K., and Wang, S. M. (2012). Sodium chloride improves photosynthesis and water status in the succulent xerophyte Zygophyllum xanthoxylum. Tree Physiol. 32, 4–13. doi: 10.1093/treephys/tpr098
Maggio, A., De Pascale, S., Ruggiero, C., and Barbieri, G. (2005). Physiological response of field-grown cabbage to salinity and drought stress. Eur. J. Agron. 23, 57–67. doi: 10.1016/j.eja.2004.09.004
Mahmood, K., Marcar, N. E., Naqvi, M. H., Arnold, R. J., Crawford, D. F., Iqbal, S., et al. (2003). Genetic variation in Eucalyptus camaldulensis Dehnh. For growth and stem straightness in a provenance–family trial on saltland in Pakistan. For. Ecol. Manag. 176, 405–416. doi: 10.1016/s0378-1127(02)00230-x
Mannan, M. A., Karim, M. A., Haque, M. M., Khaliq, Q. A., Higuchi, H., and Nawata, E. (2013). Response of Soybean to Salinity: iII Water Status and Accumulation of Mineral Ions. Trop. Agri. Dev. 57, 41–48.
Martínez, J. P., Kinet, J. M., Bajji, M., and Lutts, S. (2005). NaCl alleviates polyethylene glycol-induced water stress in the halophyte species Atriplex halimus L. J. Exp. Bot. 56, 2421–2431. doi: 10.1093/jxb/eri235
Martinez, J. P., Ledent, J. F., Bajji, M., Kinet, J. M., and Lutts, S. (2003). Effect of water stress on growth, Na+ and K+ accumulation and water use efficiency in relation to osmotic adjustment in two populations of Atriplex halimus L. Plant Growth Regul. 41, 63–73. doi: 10.1023/a:1027359613325
Meinzer, F. C., and Zhu, J. (1999). Efficiency of C4 photosynthesis in Atriplex lentiformis under salinity stress. Funct. Plant Biol. 26, 79–86. doi: 10.1071/pp98143
Melo, H. F. de., Souza, E. R. de., Almeida, B. G. de., Freire, M. B. G., dos, S., and Maia, F. E. (2016). Growth, biomass production and ions accumulation in Atriplex nummularia Lindl grown under abiotic stress. Rev. Bras. de Eng. Agric. Ambient 20, 144–151. doi: 10.1590/1807-1929/agriambi.v20n2p144-151
Messedi, D., Labidi, N., Grignon, C., and Abdelly, C. (2004). Limits imposed by salt to the growth of the halophyte Sesuvium portulacastrum. J. Plant Nutr. Soil Sci. 167, 720–725. doi: 10.1002/jpln.200420410
Mills, D., and Benzioni, A. (1992). Effect of NaCl Salinity on Growth and Development of Jojoba Clones: 11. Nodal Segments Grown in Vitro. J. Plant Physiol. 139, 737–741. doi: 10.1016/s0176-1617(11)81720-7
Miranda-Apodaca, J., Yoldi-Achalandabaso, A., Aguirresarobe, A., del Canto, A., and Pérez-López, U. (2018). Similarities and differences between the responses to osmotic and ionic stress in quinoa from a water use perspective. Agric. Water Manag. 203, 344–352. doi: 10.1016/j.agwat.2018.03.026
Munns, R. (2002). Comparative physiology of salt and water stress. Plant Cell Environ. 25, 239–250. doi: 10.1046/j.0016-8025.2001.00808.x
Munns, R., and Tester, M. (2008). Mechanisms of salinity tolerance. Annu. Rev. Plant Biol. 59, 651–681. doi: 10.1146/annurev.arplant.59.032607.092911
Ober, E. S., and Sharp, R. E. (1994). Proline accumulation in maize (Zea mays L.) primary roots at low water potentials (I. Requirement for increased levels of abscisic acid). Plant Physiol. 105, 981–987. doi: 10.1104/pp.105.3.981
Olsen, S. R. (1954). Estimation of Available Phosphorus in Soils by Extraction with Sodium Bicarbonate. Washington, D.C: US Department of Agriculture
Omami, E. N., and Hammes, P. S. (2006). Interactive effects of salinity and water stress on growth, leaf water relations, and gas exchange in amaranth (Amaranthus spp.). N. Z. J. Crop Hortic. Sci. 34, 33–44. doi: 10.1080/01140671.2006.9514385
Razzaghi, F., Ahmadi, S. H., Adolf, V. I., Jensen, C. R., Jacobsen, S. E., and Andersen, M. N. (2011). Water Relations and Transpiration of Quinoa (Chenopodium quinoa Willd.) Under Salinity and Soil Drying. J. Agron. Crop Sci. 197, 348–360. doi: 10.1111/j.1439-037x.2011.00473.x
Roussos, P. A., Gasparatos, D., Tsantili, E., and Pontikis, C. A. (2007). Mineral nutrition of jojoba explants in vitro under sodium chloride salinity. Sci. Hortic. 114, 59–66. doi: 10.1016/j.scienta.2007.05.001
Samarah, N. H. (2005). Effects of drought stress on growth and yield of barley. Agron. Sustain. Dev. 25, 145–149. doi: 10.1051/agro:2004064
Schroeder, J. I., Kwak, J. M., and Allen, G. J. (2001). Guard cell abscisic acid signalling and engineering drought hardiness in plants. Nature 410, 327–330. doi: 10.1038/35066500
Shahin, S. M., and Salem, M. A. (2014a). “The Cost of Landscaping Beauty in the United Arab Emirates (UAE): Call for Quick Actions to Save the Irrigation Resources,” in Proceedings of ICMTSET. Dubai: Zelus International
Shahin, S. M., and Salem, M. A. (2014b). The five key solutions to rescue the landscaping sector in the United Arab Emirates (UAE). Discovery 25, 41–47.
Shainberg, I., and Shalhevet, J. (2012). Soil Salinity Under Irrigation: Processes and Management. Germany: Springer Science Business Media
Sharp, R. E., and LeNoble, M. E. (2002). ABA, ethylene and the control of shoot and root growth under water stress. J. Exp. Biol. 53, 33–37. doi: 10.1093/jexbot/53.366.33
Shiklomanov, I. A. (2000). Appraisal and assessment of world water resources. Water Int. 25, 11–32. doi: 10.1080/02508060008686794
Slama, I., Ghnaya, T., Savouré, A., and Abdelly, C. (2008). Combined effects of long-term salinity and soil drying on growth, water relations, nutrient status and proline accumulation of Sesuvium portulacastrum. C. R. Biol. 331, 442–451. doi: 10.1016/j.crvi.2008.03.006
Storey, R., and Jones, R. G. W. (1979). Responses of Atriplex spongiosa and Suaeda monoica to salinity. Plant Physiol. 63, 156–162. doi: 10.1104/pp.63.1.156
Sucre, B., and Suarez, N. (2011). Effect of salinity and PEG-induced water stress on water status, gas exchange, solute accumulation, and leaf growth in Ipomoea pes-caprae. Environ. Exp. Bot. 70, 192–203. doi: 10.1016/j.envexpbot.2010.09.004
Suhayda, C. G., Giannini, J. L., Briskin, D. P., and Shannon, M. C. (1990). Electrostatic changes in Lycopersicon esculentum root plasma membrane resulting from salt stress. Plant Physil. 93, 471–478. doi: 10.1104/pp.93.2.471
Teymouri, A., Jafari, M., and Azarnivand, H. (2009). Effect of proline, soluble carbohydrates and water potential on resistance to salinity of three Salsola species. Desert 14, 15–20.
Tsamaidi, D., Daferera, D., Karapanos, I. C., and Passam, H. C. (2017). The effect of water deficiency and salinity on the growth and quality of fresh dill (Anethum graveolens L.) during autumn and spring cultivation. Int. J. Plant Prod. 14, 33–46.
Ueda, A., Kanechi, M., Uno, Y., and Inagaki, N. (2003). Photosynthetic limitations of a halophyte sea aster (Aster tripolium L) under water stress and NaCl stress. J. Plant. Res. 116, 63–68. doi: 10.17660/actahortic.2015.1099.29
Verslues, P. E., Agarwal, M., Katiyar-Agarwal, S., Zhu, J., and Zhu, J. K. (2006). Methods and concepts in quantifying resistance to drought, salt and freezing, abiotic stresses that affect plant water status. Plant J. 45, 523–539. doi: 10.1111/j.1365-313x.2005.02593.x
Walker, D. J., and Lutts, S. (2014). The tolerance of Atriplex halimus L. to environmental stresses. Emir. J. Food Agric. 1081–1090. doi: 10.9755/ejfa.v26i12.19116
Wang, W., Wang, R., Yuan, Y., Du, N., and Guo, W. (2011). Effects of salt and water stress on plant biomass and photosynthetic characteristics of Tamarisk (Tamarix chinensis Lour.) seedlings. Afr. J. Biotechnol. 10, 17981–17989. doi: 10.5897/ajb11.1864
Watanabe, S., Kojima, K., Ide, Y., and Sasaki, S. (2000). Effects of saline and osmotic stress on proline and sugar accumulation in Populus euphratica in vitro. Plant Cell Tissue Organ. Cult. 63, 199–206. doi: 10.1023/a:1010619503680
Wu, G. Q., Feng, R. J., Liang, N., Yuan, H. J., and Sun, W. B. (2015). Sodium chloride stimulates growth and alleviates sorbitol-induced osmotic stress in sugar beet seedlings. Plant Growth Regul. 75, 307–316. doi: 10.1007/s10725-014-9954-4
Wu, P., and Tan, M. (2012). Challenges for sustainable urbanization: a case study of water shortage and water environment changes in Shandong. China. Procedia Environ. Sci. 13, 919–927. doi: 10.1016/j.proenv.2012.01.085
Xiong, D., Yu, T., Zhang, T., Li, Y., Peng, S., and Huang, J. (2014). Leaf hydraulic conductance is coordinated with leaf morpho-anatomical traits and nitrogen status in the genus Oryza. J. Exp. Biol. 66, 741–748. doi: 10.1093/jxb/eru434
Yagmur, M., and Kaydan, D. (2008). Alleviation of osmotic stress of water and salt in germination and seedling growth of triticale with seed priming treatments. Afr. J. Biotechnol. 7, 2156–2162.
Yeo, A. R., Lee, Λ. S., Izard, P., Boursier, P. J., and Flowers, T. J. (1991). Short-and long-term effects of salinity on leaf growth in rice (Oryza sativa L. J. Exp. Biol. 42, 881–889. doi: 10.1093/jxb/42.7.881
Zamin, M., and Khattak, A. M. (2017). Performance of Sporobolus spicatus ecotypes, UAE native grass, under various salinity levels. Pure Appl. Biol. 6, 595–604. doi: 10.19045/bspab.2017.60061
Zamin, M., and Khattak, A. M. (2018). Evaluating Sporobolus spicatus ecotypes under different mowing heights for turf use. Sarhad J. Agric. 34, 114–122. doi: 10.17582/journal.sja/2018/34.1.114.122
Zamin, M., Khattak, A. M., Salim, A. M., Marcum, K. B., Shakur, M., Jan, I., et al. (2019a). Performance of Aeluropus lagopoide s (mangrove grass) ecotypes, a potential turfgrass, under high saline conditions. Environ. Sci. Pollut. Res. 26, 13410–13421. doi: 10.1007/s11356-019-04838-3
Zamin, M., Fahad, S., Khattak, A. M., Adnan, M., Wahid, F., Raza, A., et al. (2019b). Developing the first halophytic turfgrasses for the urban landscape from native Arabian desert grass. Environ. Sci. Pollut. Res. 27, 39702–39716. doi: 10.1007/s11356-019-06218-3
Zamin, M., Khattak, A. M., Alyafei, M. A., Sajid, M., Shakur, M., Shah, S., et al. (2018). Sporobolus spicatus, a potential turf grass under the climatic conditions of United Arab Emirates. J. Sci. Agric. 2, 1–8. doi: 10.25081/jsa.2018.v2.868
Keywords: salt stress, water stress, A. leucoclada, alternate crops, saline agriculture
Citation: Alam H, Zamin M, Adnan M, Ahmad N, Nawaz T, Saud S, Basir A, Liu K, Harrison MT, Hassan S, Alharby HF, Alzahrani YM, Alghamdi SA, Majrashi A, Alharbi BM, Alabdallah NM and Fahad S (2022) Evaluating the resistance mechanism of Atriplex leucoclada (Orache) to salt and water stress; A potential crop for biosaline agriculture. Front. Plant Sci. 13:948736. doi: 10.3389/fpls.2022.948736
Received: 20 May 2022; Accepted: 01 June 2022;
Published: 01 August 2022.
Edited by:
Anis Ali Shah, University of Education Lahore, PakistanReviewed by:
Attaullah Khan, Key Laboratory of Forest Ecology and Management, Institute of Applied Ecology (CAS), ChinaYang Zhiyuan, Universitat Autònoma de Barcelona, Spain
Copyright © 2022 Alam, Zamin, Adnan, Ahmad, Nawaz, Saud, Basir, Liu, Harrison, Hassan, Alharby, Alzahrani, Alghamdi, Majrashi, Alharbi, Alabdallah and Fahad. This is an open-access article distributed under the terms of the Creative Commons Attribution License (CC BY). The use, distribution or reproduction in other forums is permitted, provided the original author(s) and the copyright owner(s) are credited and that the original publication in this journal is cited, in accordance with accepted academic practice. No use, distribution or reproduction is permitted which does not comply with these terms.
*Correspondence: Shah Fahad, c2hhaF9mYWhhZDgwQHlhaG9vLmNvbQ==; Muhammad Adnan, bWFkbmFuQHVvc3dhYmkuZWR1LnBr; Shah Saud, c2F1ZGhvcnRAZ21haWwuY29t