- 1School of Agriculture, Ningxia University, Yinchuan, China
- 2Key Laboratory of Modern Molecular Breeding for Dominant and Special Crops in Ningxia, Yinchuan, China
- 3Ningxia Modern Facility Horticulture Engineering Technology Research Center, Yinchuan, China
- 4Ningxia Facility Horticulture Technology Innovation Center, Ningxia University, Yinchuan, China
- 5College of Horticulture and Landscape, Henan Institute of Science and Technology, Xinxiang, China
- 6Department of Horticulture, The University of Haripur, Haripur, Pakistan
One of the most significant environmental factors affecting plant growth, development and productivity is salt stress. The damage caused by salt to plants mainly includes ionic, osmotic and secondary stresses, while the plants adapt to salt stress through multiple biochemical and molecular pathways. Tomato (Solanum lycopersicum L.) is one of the most widely cultivated vegetable crops and a model dicot plant. It is moderately sensitive to salinity throughout the period of growth and development. Biotechnological efforts to improve tomato salt tolerance hinge on a synthesized understanding of the mechanisms underlying salinity tolerance. This review provides a comprehensive review of major advances on the mechanisms controlling salt tolerance of tomato in terms of sensing and signaling, adaptive responses, and epigenetic regulation. Additionally, we discussed the potential application of these mechanisms in improving salt tolerance of tomato, including genetic engineering, marker-assisted selection, and eco-sustainable approaches.
Introduction
Salinity has already affected more than one-third of irrigated areas, and it is expected that by 2050, more than half of the world’s cultivated land would be salinized (FAO, 2011; Zhao et al., 2020). Soil salinization severely limits the land use and affects crop yields significantly (van Zelm et al., 2020). Therefore, salt stress has become one of the major abiotic factors threatening food security worldwide. Although the saline-alkali land improvement technology helps in the expansion of arable land, the cost factor severely restricts its application. Cultivating salt-tolerant crops is a worth exploring direction to remediate this thorny problem. A clear understanding of the mechanisms mediating salt tolerance will accelerate the development of new varieties with enhanced salt tolerance.
Salt stress is commonly caused by high concentrations of sodium ion (Na+) and chloride ion (Cl–) in the soil solution, and its adverse effects on plants include primary stresses like osmotic stress and ion imbalance, as well as secondary stresses like oxidative stress and metabolic abnormalities (Yang and Guo, 2018a). The excessive accumulation of Na+ causes the soil’s osmotic pressure to rise, water potential to drop, and root water uptake to decrease, thereby reducing water availability (Julkowska and Testerink, 2015; van Zelm et al., 2020). Furthermore, the Na+/potassium ion (K+) ratio is disrupted, inhibiting the activities of numerous K+-dependent enzymes in the cells (Wu et al., 2018). Chloride harms the plants primarily by causing an ion imbalance via interfering with the uptake or metabolism of other necessary ions (Bazihizina et al., 2019; Zhao et al., 2020). Salt-induced osmotic stress and ion imbalance may not only impair the photosynthesis, thus affecting plant energy metabolism, but also trigger the production of reactive oxygen species (ROS), resulting in oxidative damage.
Tomato (Solanum lycopersicum L.), one of the most important vegetable crops, is grown all over the world. Its fruits are widely used as food in the fresh market, and it’s also a model plant for genetics, fruit development, and stress tolerance research (Rothan et al., 2019). Tomatoes are native to western South America, and their wild relatives have been adapted to severely saline coastal regions, however, cultivars have lost their salt resistance during domestication (Gálvez et al., 2012; Pailles et al., 2020). Salt tolerance is a complex trait, thus developing salt-tolerant cultivars necessitates a thorough study of physiological responses, metabolic changes, and gene expression patterns under salinity. To date, the vast majority of salt-tolerance research has been performed using model plants such as Arabidopsis, whose contributions to application-oriented research into salt-tolerance are limited by its inherently low levels of salt tolerance and lack of agronomically relevant yield-related traits (Morton et al., 2019). Tomato is a moderately salt-sensitive and yield-targeted crop that can overcome this limitation of Arabidopsis. In this review, we provide a critical review of the effects of salt stress, mechanisms of salt tolerance, and biotechniques to improve salt tolerance in tomato. Finally, we highlight research directions for accelerating the genetic improvement of salt tolerance in tomato. Understanding the response and tolerance of tomato to salt stress will enable translational applications to other yield-targeted crops, thus will contribute to the expansion of arable land, agricultural sustainability and global food security.
Impact of salinity on tomato
Morphological changes
Generally, the seed germination and early seedling growth stages are most susceptible to salinity, and roots are more vulnerable than other organs (Foolad, 2004). Salt stress delays germination and reduces the germination rate of tomato seeds through changing the activities of key enzymes and the levels of gibberellin (GA), respectively (Singh et al., 2012; Tanveer et al., 2020). Salinity induces the roots to absorb Na+, resulting a decrease in the osmotic potential and water intake, which in turn inhibits root growth (Siddiqui et al., 2017; Tanveer et al., 2020). Nevertheless, the root:shoot ratio increased in response to increasing salinity indicating that shoot growth is restricted while root growth is less hindered (Singh et al., 2012). In addition, salinity also represses the development of leaf, flower and fruit by inhibiting cell division or elongation, hindering sugar metabolism and decreasing water import, respectively (Ghanem et al., 2009; Siddiqui et al., 2017; Pinedo-Guerrero et al., 2020).
Physiological and biochemical changes
Under high salinity stress, tomato plants collect more Na+ and Cl–, while the levels of K+ and calcium ion (Ca2+) decrease, disrupting ion homeostasis, which can be restored by rescuing seedlings from salinity (Rahman et al., 2016; Parvin et al., 2019b). Sodium preferentially accumulate in old leaves, protecting young leaves from the toxic effects of saline stress (Khelil et al., 2007). The detrimental effects of Cl– result from interference in the uptake or metabolism of other essential ions, such as nitrate ion (NO3–) (Zhao et al., 2020).
Excessive ROS induced by high salinity can damage the structure of macromolecules (Waszczak et al., 2018). In roots, oxidative stress caused by salinity was earlier and more sensitive than that in leaves (Gapiñska et al., 2008). The increased anti-oxidase activity in salt-tolerant genotypes helps to protect against oxidative damage (Gharsallah et al., 2016b). Furthermore, the antioxidant defense system and the glyoxalase system work together to detoxify salt-induced ROS and improve salt tolerance in tomato (Parvin et al., 2019b).
Salinity also regulates the compatible solutes, such as proline (Pro) and glycine betaine (GB). Salinity significantly increases Pro, and its level in tomato leaves was higher than that in roots, which attributes in maintaining the chlorophyll content and cell turgidity to protect the photosynthetic activity (Gharsallah et al., 2016b; De la Torre-González et al., 2018). The GB content decreased both in two salt-stressed commercial genotypes, while the levels in the salt-tolerant genotype was lower than that in the sensitive genotype (De la Torre-González et al., 2018). However, exogenous application of GB or its accumulation by genetic engineering alleviated salt-induced K+ efflux, enhancing salt tolerance in tomato (Wei et al., 2017).
Short-term salt treatment reduces stomatal conductance, pore area and index, that strongly suppress the dynamic photosynthesis in leaves (Zhang et al., 2018). Under salt conditions, the phytohormones such as abscisic acid (ABA), ethylene (ET), and salicylic acid (SA) are also involved in tomato photosynthesis. ABA causes stomatal closure, reducing leaf gas exchange in salinized plants (Lovelli et al., 2012). ET controls net carbon dioxide (CO2) fixation through stomatal or non-stomatal factors, and regulates the activity of photosystems and efficiency of photoprotective processes (Borbély et al., 2020). SA increases photosynthetic activity by inducing higher maximal CO2 fixation rate, carboxylation efficiency of Rubisco, and photosynthetic quantum efficiency (Poór et al., 2011).
Unlike the severe damage caused by high salinity, moderate salt stress improves fruit quality by increasing soluble solid content, carotenoid levels, and the accumulation of glutamic acid (Glu), gamma-aminobutyric acid (GABA), glutamine, and α-tocopherol (Massaretto et al., 2018; Meza et al., 2020). The range of salinity to improve fruit quality without affecting tomato yield deserves special attention, which may depend on varieties and duration of stress.
Mechanisms for salt tolerance in tomato
Salt signaling pathways and ion transport
How salt enters the plants and salt perception remain unknown (Yang and Guo, 2018b; van Zelm et al., 2020). Ion uptake occurs mainly through the apoplastic and symplastic pathways, and water moves radially through the root via the apoplastic, symplastic and transcellular pathways (the latter two pathways are collectively referred to as cell-to-cell pathway) (Sánchez-Aguayo et al., 2004; Isayenkov and Maathuis, 2019). High salinity in the apoplast alters aqueous and ionic thermodynamic equilibria, which results in ionic and/or osmotic stresses. The percentage of water movement through the symplastic pathway in tomato plants treated with 75 mM NaCl was lower than that in untreated plants. The bulk flow of water and solutes along the apoplastic pathway under saline conditions would impart smaller selectivity and reduced ion uptake (Fernández-García et al., 2002; Sánchez-Aguayo et al., 2004). The contribution of the apoplastic pathway in tomato species that differ in salt tolerance needs to be further explored.
Various transporters are involved in Na+ uptake and movement across the plasma membrane (PM), of which non-selective cation channels (NSCCs) are a main route of Na+ influx into glycogenic plant roots (Isayenkov and Maathuis, 2019; Figure 1). NSCCs are regulated by different salt-induced early signals, such as Ca2+, 3′,5′-cyclic guanosine monophosphate (cGMP), and ROS (van Zelm et al., 2020). K+ transporters and transporters from the HKT family are also involved in primary sodium influx into roots (Kronzucker and Britto, 2011). When Na+ enters the cell through NSCCs, the membrane depolarizes. This change in membrane voltage prevents the hyperpolarization and tomato high-affinity K+ transporter 5 (HAK5) expression induced by K+ starvation (Nieves-Cordones et al., 2008). High Ca2+ reduces the Na+-induced depolarization in tomato root cells and mitigates the repression of HAK5 mediated high-affinity K+ uptake (Bacha et al., 2015).
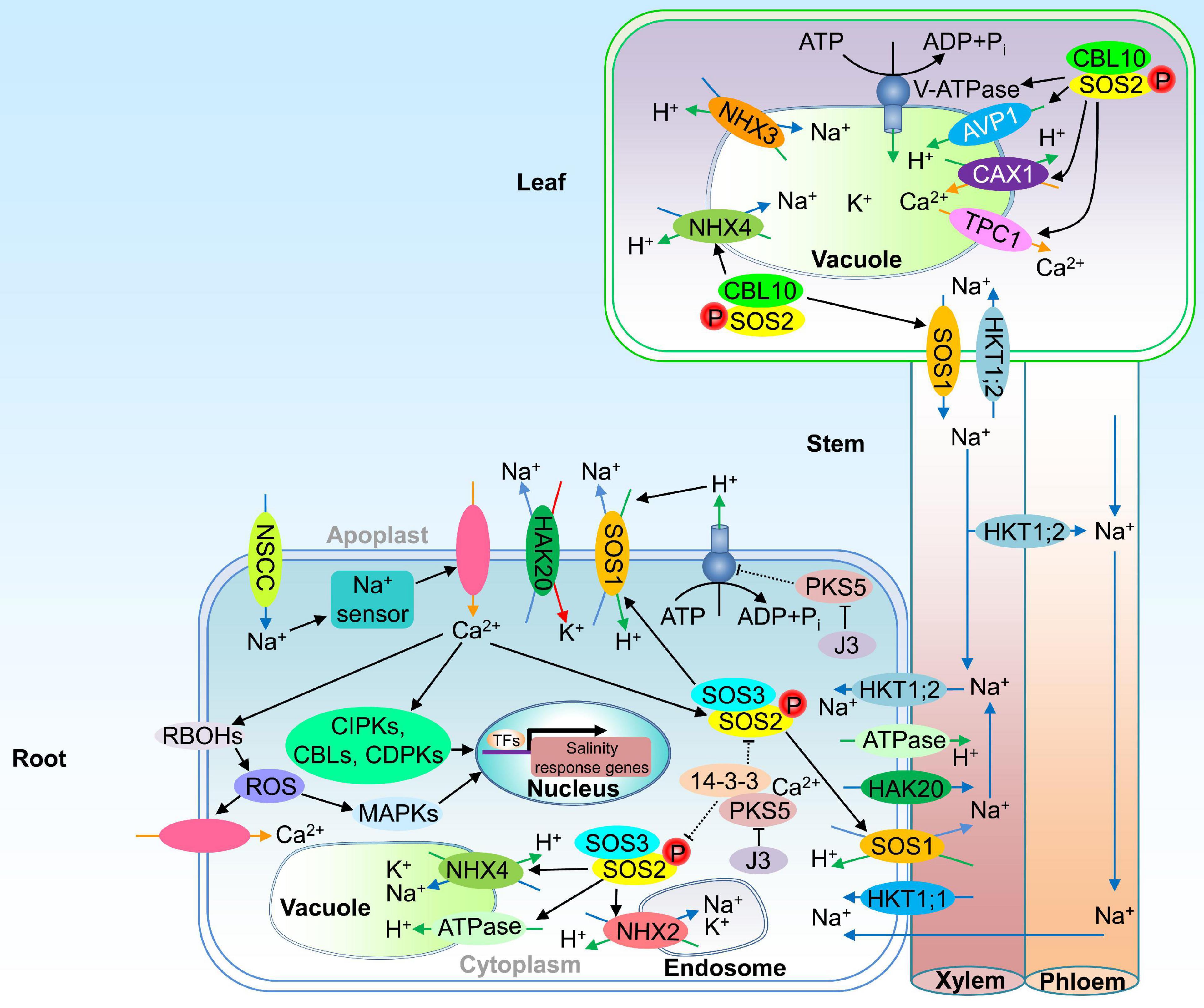
Figure 1. Schematic overview of sodium uptake into tomato roots and transport into leaves. Na+ ions enter tomato root cells primarily through NSCC pathway. Na+ entering the cell is sensed by a yet undetermined sensory mechanism. Subsequently, Ca2+, ROS, and hormone (not shown) signaling pathways are activated. Ca2+ induces ROS production by RBOHs and ROS induces Ca2+ import. As one part of the Ca2+-signaling pathway, CIPKs, CBLs, and CDPKs become active and alter the global transcriptional profile in the nucleus. MAPKs activated by Ca2+-ROS- signaling pathway also transduce downstream gene transcription in the nucleus. These early signaling pathways result in activation of detoxification mechanisms. Cytosolic Ca2+ ions activate SOS3. SOS2 regulates ATPase, NHX2 and NHX4, increasing Na+/H+ and K+/H+ anti-transport activity in root vacuole and endosome. The kinase activity of SOS2 is negatively regulated by 14-3-3 proteins, and the inhibition on SOS2 is released by the Ca2+-mediated binding of PKS5 with 14-3-3. SOS1 activated by SOS2-SOS3 heterologous kinase complex is responsible for extruding Na+ out the root and partitioning Na+ in organs. J3 inhibits PKS5 kinase activity, activating the activity of PM H+-ATPases and generating a proton gradient required for Na+ transport of SOS1. CBL10-SOS2 complex triggers the separation of Na+ into leaf vacuole and the transport of Na+ ions from leaves to xylem, activates the tonoplast targets TPC1 and AVP1, maintaining an appropriate Na+/Ca2+ ratio and V-ATPase, and promotes the proton gradient necessary to energize the Ca2+ transport toward the vacuole through CAX1. HKT1;2 is involved in xylem Na+ unloading and Na+ uploading into the phloem, thus promoting Na+ recirculation from shoots to roots, which can be additionally regulated by HKT1;1 in roots. HAK20 transports and regulates the homeostasis of Na+ and K+. ADP, adenosine diphosphate; ATP, adenosine triphosphate; AVP1, H+-pyrophosphatase; CAX1, CATION EXCHANGER 1; CBL, calcineurin B-like protein; CDPKs, calcium-dependent protein kinases; CIPKs, CBL-interacting protein kinases; RBOHs, respiratory burst oxidase homologs; HAK, high-affinity K+ transporter; HKT, high K+ affinity transporter; J3, DNAJ HOMOLOG3; MAPK, mitogen-activated protein kinase; NHX, Na+/H+ exchanger; NSCCs, non-selective cation channels; PKS5, PROTEIN KINASE5; ROS, reactive oxygen species; SOS, salt overly sensitive; TFs, transcription factors; TPC1, TWO-PORE CHANNEL 1. The dashed lines indicate that the negative regulatory roles are released under salt stress.
Plants sense and respond to salt stress within a short period of time. But so far, no specific salt sensor has been identified in cells. The glucuronosyltransferase encoded by monocation-induced [Ca2+]i increases 1 (MOCA1) is involved in the biosynthesis of glycosyl-inositol phosphorylceramide (GIPC) sphingolipids at the PM. GIPCs directly bind to Na+ and regulate the entry of Ca2+ into the cytosol, but the detailed signal transduction process remains unclear (Jiang et al., 2019; Zhao et al., 2020). The decreased pectin crosslinking caused by salt is sensed by a receptor-like kinase (RLK) FERONIA (FER), while the downstream signaling of this receptor is not part of the early signaling response (Feng et al., 2018). The response of plants to salt stress may be the result of sensing and integration of multiple signaling pathways (van Zelm et al., 2020; Figure 2).
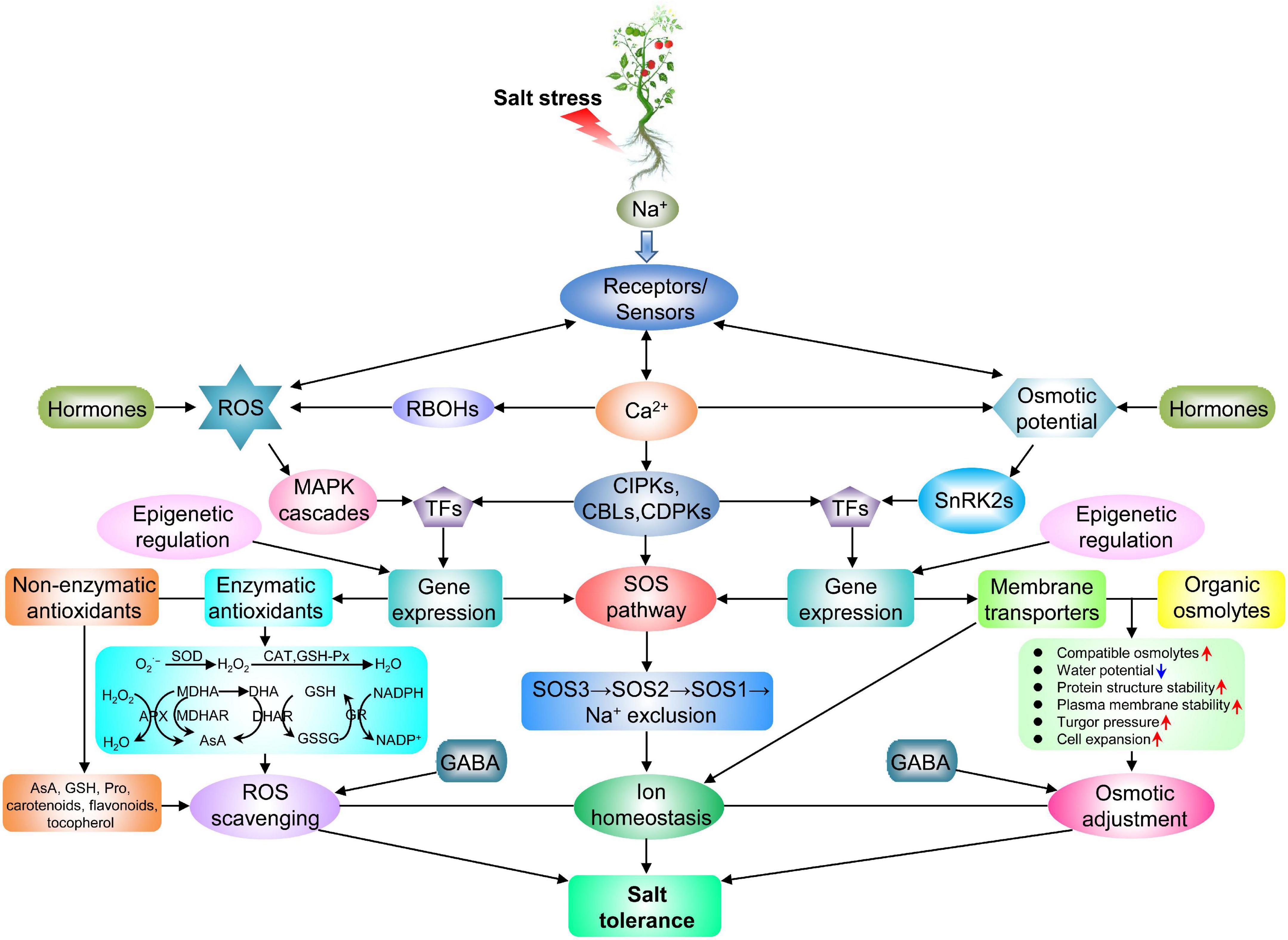
Figure 2. The potential mechanisms underlying salt tolerance in tomato. Salt stress is sensed by unknown receptors or sensors that act synergistically with Ca2+ waves, ROS production and osmotic potential. These changes activate antioxidant, ion transporter and osmoregulation pathways, leading to salt tolerance through ROS scavenging, ionic homeostasis and osmotic adaptation. Endogenous GABA participates in ROS scavenging and osmotic adaptation under salt conditions. The red and blue arrows in the box indicate the increase and decrease of the indicators, respectively. APX, ascorbate peroxidase; AsA, ascorbic acid; CAT, catalase; DHA, dehydroascorbate; DHAR, dehydroascorbate reductase; GABA, gamma-aminobutyric acid; GR, glutathione reductase; GSH, glutathione; GSH-Px, glutathione peroxidase; GSSG, oxidized glutathione; H2O2, hydrogen peroxide; MDHAR, monodehydroascorbate reductase; NADP+, oxidized form of NADPH; NADPH, nicotinamide adenine dinucleotide phosphate; O2⋅–, superoxide radical; Pro, proline; SnRK2s, sucrose non-fermenting-1-related protein kinase 2s; SOD, superoxide dismutase. The remaining abbreviations mentioned in this figure exist in Figure 1.
High salinity rapidly induces the accumulation of cytosolic Ca2+, which can form early signal components of salt sensing relays (Isayenkov and Maathuis, 2019). A series of Ca2+-dependent proteins, such as calcineurin B-like proteins (CBLs), calcium-dependent protein kinases (CDPKs) and CBL-interacting protein kinases (CIPKs) are involved in the signal decoding of Ca2+ influx into the cytoplasm (Zhao et al., 2020). As the best-characterized CBL- CIPK route, Ca2+-dependent salt overly sensitive (SOS) pathway governs ionic homeostasis and salt tolerance, and has been postulated as a molecular switch for salt stress responses (Huertas et al., 2012; Zhu, 2016). SOS3, a functional Ca2+-binding protein, senses Ca2+ and physically interacts with SOS2 at the PM. SOS2 is a serine/threonine protein kinase functioning as a key regulator of ion transporters (Belver et al., 2012). SOS3–SOS2 complex phosphorylates and activates SOS1, a PM Na+/H+ antiporter that extrudes Na+ out of the cell (Yang and Guo, 2018b). Tomato SOS3 is mainly expressed in roots (Cho et al., 2021), and SOS3–SOS2 complex can activate Na+/H+ antiport activity of Arabidopsis AtSOS1 (Huertas et al., 2012), indicating that the SOS pathway is conservative.
In tomato, decreased levels of SOS1 lead to a salt-sensitive phenotype (Olías et al., 2009a; Wang Z. et al., 2021). Unlike only the overexpression of activated forms of Arabidopsis AtSOS2 increases salinity tolerance in transgenic plants (Guo et al., 2004), overexpression of the both native and activated forms of tomato SOS2 increase salt tolerance (Huertas et al., 2012; Cho et al., 2021). Activation of SOS1 in tomato plants overexpressing SOS2 may contribute to the efflux of Na+ out the root epidermal cells as well as the active loading of Na+ into shoots (Olías et al., 2009a; Belver et al., 2012; Huertas et al., 2012). Overexpression of tomato enhancer of SOS3-1 (ENH1) excludes more Na+ from cytosol and transports into vacuoles, and maintains more K+ in cytosol to reestablish ion homeostasis (Li D. et al., 2013).
Tomato SOS2 regulates the activity of V-ATPase, energizing the Na+/H+ antiport at the endosomal-prevacuolar as well as vacuolar compartments. Additionally, SOS2 activates the Na+/H+ and K+/H+ antiport activity in root intracellular membranes and the Na+/H+ antiport activity in root tonoplast vesicles, which are regulated by the endosomal-vacuolar K+, Na+/H+ (NHX2 and NHX4) antiporters (Huertas et al., 2012). The K+/H+ antiporter NHX2 increases salt tolerance by improving K+ uptake and compartmentalization (Rodríguez-Rosales et al., 2008; Huertas et al., 2013). Tomato plants overexpressing both NHX2 and SOS2 grow better under salinity conditions than plants overexpressing only one of them (Baghour et al., 2019). How SOS2 activates NHX transporters in tomato roots remains to be clarified.
Under salt conditions, tomato CBL10 can form a complex with SOS2. SOS2–CBL10 complex maintains a proper Na+/Ca2+ ratio in the vacuole of leaf cells through the activation of tonoplast targets, therefore protecting young developing tissues from the damage caused by salt (Figure 1). The tonoplast targets include the cation channel TWO-PORE CHANNEL 1 (TPC1, mediating Ca2+ release from vacuoles) and two vacuolar H+-pumps, H+-pyrophosphatase AVP1 (AVP1) and V-ATPase (V-ATPase). The expression of tomato SOS2 is associated with CBL10. Loss function of CBL10 strongly inhibits the expression of CATION EXCHANGER 1 (CAX1) and impairs the proton gradient necessary to energize the Ca2+ transport toward the vacuole through CAX1 antiporter (Egea et al., 2018). The role of tomato SOS2-CBL10 complex in regulating vacuolar Na+ sequestration is not well characterized.
Plant 14-3-3 proteins are phosphoserine-binding proteins that regulate the activities of a wide array of targets, playing an important role in response to salt stress (Xu and Shi, 2006). 14-3-3 proteins inhibit the kinase activity of SOS2 under normal conditions. PROTEIN KINASE5 (PKS5) inhibits SOS2 activity by promoting the interaction between SOS2 and 14-3-3 proteins. Under salt conditions, chaperone DNAJ HOMOLOG3 (J3) interacts with PKS5 kinase and inhibits its activity, thereby releasing the inhibition of SOS2 activity by 14-3-3 protein, which is associated with salt-induced Ca2+ signal (Yang and Guo, 2018b; Yang et al., 2019; Zhao et al., 2020). At least 12 genes named TOMATO 14-3-3 PROTEIN1 (TFT1)-TFT12 are predicted to encode tomato 14-3-3 proteins, and the levels of TFT1, TFT4, TFT7, and TFT10 are significant up-regulated by salt (Xu and Shi, 2006). Enhanced salt tolerance in TFT7-overexpressing transgenic plants due to the reduction of oxidative stress injury rather than the maintenance and reestablishment of cellular ion homeostasis (Xu and Shi, 2007). In addition to salt stress, tomato TFT4 is also involved in response to alkali stress, but the functions of tomato TFT4 in the integration of H+ efflux, the basipetal indole-3-acetic acid (IAA) transport, and the PKS5-J3 pathway need to be further defined (Xu et al., 2013).
Salt stress induces the accumulation of ROS, including superoxide radical (O2⋅–), hydroxyl radical (OH⋅), singlet oxygen (1O2), and hydrogen peroxide (H2O2) (Yang and Guo, 2018a). ROS have toxic effects at high concentrations, while function as signal transduction molecules at low concentrations (Miller et al., 2010). Under salt conditions, increased cytosolic Ca2+ induces respiratory burst oxidase homologs (RBOHs) to generate ROS, and ROS induces Ca2+ entry into stomata (van Zelm et al., 2020). In tomato, most of eight RBOH genes were significantly upregulated by persistent salt stress, showing that RBOHs are essential regulators response to long-term salinity stress (Raziq et al., 2022). Tomato RBOH1-dependent apoplastic H2O2 mediates epigallocatechin-3-gallate-induced abiotic stress tolerance (Li et al., 2019b), high atmospheric CO2-dependent salt stress tolerance (Yi et al., 2015) and spermine-induced salinity-alkalinity stress tolerance (Xu et al., 2021). Furthermore, salt-induced ROS can activate downstream mitogen-activated protein kinase (MAPK) cascades. Activated MPKs transduce signals to downstream transcription factors (TFs) in the nucleus to induce the expression of stress-responsive genes (Isayenkov and Maathuis, 2019). MAPK cascade consists of three consecutively acting and phosphorylating protein kinases, MAP kinase kinase kinase (MAPKKK/MEKK), MAP kinase kinase (MAPKK/MKK), and MAP kinase (MAPK/MPK) (Mishra et al., 2006). In tomato genome, 16 MAPK, 5 MAPKK and 89 MAPKKK genes are identified (Kong et al., 2012; Wu et al., 2014). Tomato MAPKK2 and MAPKK5 were significantly upregulated by salt stress, and the transcript levels of 13 MAPKKK genes shown a more than 10-fold change under salt stress (Wu et al., 2014). Although there is no evidence that salt stress affects the expression levels of tomato MAPK family genes, MAPK3 improves salt tolerance by increasing the expression levels of ET synthesis genes and SOS pathway genes (Shu et al., 2022).
Membrane transporters play critical roles in maintaining Na+/K+ homeostasis (Figure 1). The Na+ transporter HKT1;2 gene is responsible for the major quantitative trait loci (QTL) involved in Na+ and K+ homeostasis in tomato. Silencing of HKT1;2 alters the Na+/K+ ratio, suppresses the response of SOS1 to salt, and increases salt hypersensitivity (Asins et al., 2013; Jaime-Pérez et al., 2017; Romero-Aranda et al., 2020). HKT1;2 of S. cheesmaniae is not only involved in xylem Na+ unloading but also involved in Na+ uploading into the phloem, thus promoting Na+ recirculation from aerial parts to the roots, which can be additionally favored by HKT1;1 silencing at the roots (Romero-Aranda et al., 2021). Decreasing Na+ content in young leaves is probably linked to the up-regulation of SOS1 contributing to Na+ exclusion from the cytosol toward the leaf apoplast. Nevertheless, preventing Na+ accumulation in the cytosol of mature/old leaves may be mainly attributed to the combined action of HKT1-like transporters (mediating Na+ unloading from the xylem) and NHX-type transporters (promoting Na+ and K+ accumulation in vacuoles and endosomes) (Olías et al., 2009b; Belver et al., 2012). CBL10 regulates the expression of HKT1;2 and SOS1. The lack of CBL10 severely inhibits Na+ compartmentalization into vacuoles as well as Na+ upload from xylem into cells, while promotes Na+ extrusion from leaf cells to xylem, dilapidating its functional role in regulating Na+ homeostasis and protecting shoot apex and developing tissues from salt damage (Egea et al., 2018). The HAK/KUP/KT (high-affinity K+/K+ uptake/K+ transporter) family transporters primarily mediate K+ fluxes. Tomato HAK20 is identified as the major player controlling root Na+/K+ ratio with genome-wide association studies (GWAS) (Wang et al., 2020). It functions in the loading of K+ and Na+ into the xylem in roots. The wild allele of HAK20 loads more Na+ into root xylem and enhances Na+ efflux, therefore lowering the Na+/K+ ratio in roots and leading to higher salt tolerance, but its function in shoot is still elusive (Wang et al., 2020; Xiang and Jiménez-Gómez, 2020).
Osmotic adjustment
High salinity causes ionic imbalance and water deficit in plant cells, leading to osmotic stress (Zhao et al., 2021). A hyperosmolality-gated calcium-permeable channel encoded by reduced hyperosmolality-induced [Ca2+]i increase1 (OSCA1) was identified as an osmotic stress sensor (Yuan et al., 2014), but the role of OSCA1 in the sense of high salinity-triggered osmotic stress is questioned (Zhao et al., 2020). Plants perform osmotic adjustment by increasing the concentration of solutes and decreasing water potential, which is vital for the alleviation of the osmotic imbalances and for maintaining cell turgor (Lv et al., 2019). Under osmotic stress, solutes can act as osmolytes or play a protective role by stabilizing the structure of biological macromolecules (Figure 2). Osmotic regulators mainly include inorganic ions and organic substances (Hao et al., 2021). The organic osmolytes includes sugar, complex sugars, Pro, GB, polyamines (PAs), polyols and late embryogenesis abundant (LEA) proteins (Yang and Guo, 2018b; Liu et al., 2022). Halophytes and salt-tolerant non-halophyte species synthesize organic solutes for osmotic adjustment in the cytoplasm only, while absorb Na+ and Cl– from the soil for the bulk of osmotic adjustment in the vacuole, a more energy-efficient strategy (Munns et al., 2016). Due to low tissue tolerance, a large portion of osmotic adjustment in salt-sensitive species occurs with organic solutes, but the high synthetic cost decreases the growth rate (Munns and Gilliham, 2015).
K+ is one of the macronutrients required by plants for growth, and plays an important role in preventing cell damage caused by salt. Exogenous K+ activates carbohydrate metabolism and Pro accumulation through endogenous hydrogen sulfide (H2S) signaling, thereby increasing osmotic tolerance and enhancing the hydration levels of the salt stressed tomato seedlings (Khan et al., 2021). Under salt conditions, K+, Na+, Cl–, and organic acids are the main osmolytes in tomato plants (Wang et al., 2011). The hydration ability of Pro helps the attached proteins bind more water, and prevents protein dehydration and denaturation under salt stress (Hao et al., 2021). The Pro increase in the tolerant tomato varieties supports the opinion that Pro counteracts the osmotic stress caused by salinity (De la Torre-González et al., 2018). On the other hand, the increased Pro in plants with salt treatment is generally not enough for beneficial effects, and the accumulation of Pro increases as the more stress effects. Thus, Pro concentration can be used as a negative indicator of tolerance. Foliar application of a mixture of Pro and Glu mitigated the negative effects of salt on tomato growth by accumulating total soluble sugars, but the concentration of Pro was significantly decreased (Alfosea-Simón et al., 2020). The beneficial effects of GB on reducing osmotic imbalance induced by salt stress have been demonstrated in various plants (Gupta and Huang, 2014; Lv et al., 2019). However, in some tomato genotypes, GB concentration is negatively correlated with salt tolerance, which may be due to the oxidative stress caused by H2O2 generated from the synthesis of GB via the choline pathway (De la Torre-González et al., 2018). The roles of Pro and GB in salt tolerance may depend on the species and cultivars. Foliar application of L-methionine (Met) and L-phenylalanine (Phe) induces salt tolerance of tomato by enhancing the PM stability, the contents of osmolytes, and the activity of antioxidative enzymes (Almas et al., 2021). Under salt stress, exogenous GABA inducing amino acid content increases osmotic adjustment capacity to resist water loss and neutralizes excessive Na+ in the vacuoles in tomato leaves (Wu et al., 2020). Trehalose alleviates the salt damage of tomato plants by promoting the accumulation of osmotic substances Pro, GB, and soluble proteins (Yang Y. et al., 2022).
Cell wall-associated protein kinases (WAKs) localized in PM induce the changes of solutes contents. Mutation of tomato WAK1 is tolerant to Na+ homeostasis but not to osmotic homeostasis, and it increases sucrose content in roots. The salt sensitivity of wak1 mutant is due to the altered osmotic and metabolic homeostasis (Meco et al., 2020). Under osmotic stress, the tomato cryptochrome 1a (cry1a) enhances the growth by reducing the MDA content and Pro accumulation, and specific blue light fluence rates are required for cry1a-mediated osmotic responses (D’Amico-Damião et al., 2021). The dehydrin tas14 gene, a member of the tomato LEA family, improves osmotic tolerance by reducing osmotic potential and accumulating solutes (such as sugars and K+). Plants overexpressed tas14 transfer Na+ into adult leaves while K+ and sugars in young leaves, achieving osmotic balance in older leaves at a minimal energy cost (Muñoz-Mayor et al., 2012). Overexpression of tomato ICE1a, a MYC-type ICE1-like TF, increases the levels of Pro, soluble sugars and LEA proteins, enhancing osmotic and salt tolerance (Feng et al., 2013). In tomato plants, the accumulation of several osmotic protectants (Pro, sucrose, glucose, and GB) under salt conditions, depends on the regulation of key enzymes in their synthetic pathway at both transcriptional and post-transcriptional levels (Rivero et al., 2014).
Endogenous ABA improves short-term osmotic stress resistance in tomato via osmotic and hydraulic adjustments (Li et al., 2022). Tomato ABSCISIC ACID STRESS RIPENING1 (ASR1) protects yeast from osmotic stress by inducing downstream components of the high-osmolarity glycerol pathway (Moretti et al., 2006). ASR1 also binds directly to a tomato cellulose synthase-like (CSL) protein gene that may play key roles in osmotic stress (Ricardi et al., 2014). All the tomato sucrose non-fermenting 1-related protein kinase 2 (SnRK2) genes are salt stress responsive and most of them are also induced by ABA (Yang et al., 2015; Chen et al., 2016). Overexpression of tomato SnRK2.1 and SnRK2.2 regulates the expression of stress-related genes and decreases osmotic tolerance, but the regulatory relationship between the activated SnRK2s and stress-related gene expression remains to be identified (Yang et al., 2015). In addition to ABA, ET and auxin also play important roles in osmotic stress response. Under osmotic stress, the ET-induced H2S is required for ET-induced tomato stomatal closure (Jia et al., 2018). Overexpression of tomato ET responsive factor 1 (TERF1) in tobacco induces not only the typical ET triple response but also salt tolerance by stimulating the expression of downstream genes. TERF1 may be a linker in ET and osmotic signaling pathways (Huang et al., 2004). Down-regulation of tomato Auxin Response Factor 4 (ARF4) improves salt and osmotic tolerance by reducing stomatal conductance along with increased leaf relative water content and ABA content (Bouzroud et al., 2020).
Reactive oxygen species generation and antioxidant defense
Reactive oxygen species accumulation
Salt stress rapidly induces the production of ROS in plant apoplast, chloroplasts, mitochondria, and peroxisomes (Chen et al., 2021). The apoplastic ROS are produced by the activation of PM-localized nicotinamide adenine dinucleotide phosphate (NADPH) oxidases (RBOHs), apoplastic peroxidases (PODs), diamine oxidases (DAOs), and PA oxidases (PAOs) (Qi et al., 2017). Salt induces the transcript of tomato RBOH1 and enhances the activity of NADPH oxidase, deriving H2O2 accumulation in the apoplast. Inhibition of RBOH1 expression impairs the ROS scavenging induced by RBOH1-dependent H2O2 signal, while the downstream molecular players that transduce ROS signaling to the transcriptional level remain elusive (Li et al., 2019b). Tomato MAPK3 may increase salt tolerance and decrease heat tolerance through the RBOH1-dependent antioxidant system, respectively (Yu et al., 2019; Shu et al., 2022). How tomato MAPK3 and RBOH1 function in the combined response to salt and heat stress remains to be elucidated. Salt stress improves the activities of DAO and PAO. The terminal oxidation of PAs by DAO and PAO contributes to the production of H2O2. PAO inhibitor reduced the activity of PAOs and inhibited H2O2 production, but did not increase salt tolerance due to the significantly increased electrolytic leakage (Takács et al., 2017). PAO induced by spermidine (Spd) mediates the elevation of H2O2 level, thereby activating the antioxidant system to eliminate excess ROS accumulation and relieve membrane lipid peroxidative damage and growth inhibition under saline-alkali stress (Yang J. et al., 2022). These reports suggest that ROS are primarily used for stress-sensing and signaling (Mittler et al., 2022).
Both salt stress-induced stomatal closure and accumulation of high levels of Na+ in the cytoplasm impair the photosynthetic machinery. As a result, the absorbed light exceeds the demand for photosynthesis, leading to the formation of ROS in chloroplasts, including O2⋅–, 1O2, and H2O2. O2⋅– is generated by the Mehler reaction in the photosystem I (PSI), 1O2 is produced by photosystem II (PSII) in the thylakoid membrane because of limitation of the electron transport between photosystems, and H2O2 is produced at the electron-donor side of PSII via the incomplete oxidation of water (Zhao et al., 2020). In tomato, salt stress induced the increased ROS levels and the decreased PSII activity by reducing the oxygen-evolving complex (OEC) activity on the donor side of PSII, damaging the donor and acceptor sides of the photosystem, and blocking the electron transfer on receptor side of PSII. Melatonin (MT) reduces the production of ROS by balancing the distribution of photosynthetic electron flux and enhances the scavenging ability of ROS by promoting the activities of enzymes involved in the ascorbate glutathione (AsA-GSH) cycle, increasing salt tolerance in tomato (Yin et al., 2019).
Another pathway of ROS production is mitochondrial respiration. Electrons leak from complexes I and III of the mitochondrial electron transport chain to molecular oxygen, resulting in O2⋅– generation and then be rapidly catalyzed into H2O2 (Liu et al., 2020). Peroxisomes are one of the major sites where plants produce intracellular H2O2 (Liu et al., 2021). SA induces mitochondrial ROS production by reducing mitochondrial hexokinase (mtHXK) activity in tomato leaves (Poór et al., 2019). Salt induces the increase of mitochondrial H2O2 in the cultivated tomato roots, attributing to the non-enzymatic reduction of superoxide by AsA and GSH, while the decreased H2O2 of wild salt-tolerant related species is due to the higher rate of H2O2 detoxification. The decreased H2O2 in peroxisomes is in part the result of the activities of ascorbate peroxidase (APX) and catalase (CAT) over that of superoxide dismutase (SOD) (Mittova et al., 2004). The gene encoding peroxisome-localized PAO4 is regulated by salt and oxidative stress, suggesting that tomato PAOs may also be involved in ROS metabolism in peroxisomes under salt conditions (Hao et al., 2018).
Enzymatic scavenging system
Salt-induced overaccumulation of ROS imposes oxidative stress on plants, causing ionic imbalance, DNA mutation, peroxidation of lipids and carbohydrates, protein denaturation, pigment breakdown and impaired enzymatic activity (Liu et al., 2020). Tomato plants have formed the antioxidant defense system to mitigate ROS stress caused by salt (Figure 2). The antioxidant defense system consists of enzymatic and non-enzymatic antioxidants (Hao et al., 2021). Enzymatic antioxidants include RBOHs, SOD, APX, CAT, glutathione reductase (GR), glutathione S-transferase (GST), glutathione peroxidase (GSH-Px), monodehydroascorbate reductase (MDHAR), dehydroascorbate reductase (DHAR), and guaiacol peroxidase (GPOX). Non-enzymatic antioxidants include AsA, GSH, Pro, carotenoids, flavonoids, and tocopherol (Yang and Guo, 2018b; Liu et al., 2022).
Superoxide dismutase is the most effective ROS scavenger and converts O2⋅– to H2O2, which is further detoxified to H2O by APX, CAT, and GSH-Px (Yang and Guo, 2018a). Based on the difference of metal cofactors and subcellular distribution, SODs are mainly categorized as copper/zinc SODs (Cu/Zn-SODs), iron SODs (Fe-SODs), and manganese SOD (Mn-SODs). Fe-SODs are mainly distributed in the chloroplast and cytoplasm, Mn-SODs in mitochondria, and Cu/Zn-SODs in chloroplasts, cytosol and peroxisomes (Czarnocka and Karpiñski, 2018). There are at least nine SOD genes in tomato, including four Cu/Zn-SODs, three Fe-SODs and one Mn-SOD, and most of them are regulated by salt stress (Feng et al., 2016). CAT rapidly catalyzes H2O2, producing H2O and O2. The expression of tomato CAT gene is fine-tuned under salt stress (Hernández-Hernández et al., 2018). Recently tomato microRNA398b (miR398b) is found to regulate antioxidant system under salt conditions. The inhibition of Cu/Zn-SOD 1 (CSD1) expression and SOD activity caused by miR398b promotes the accumulation of O2⋅–. Meanwhile, miR398b also decreases the activity of APX and CAT, and the contents of GSH as well, leading to H2O2 accumulation (He et al., 2021).
The AsA-GSH recycling pathway, also known as Asada-Halliwell pathway comprises of AsA, GSH, and four enzymes (including APX, MDHAR, DHAR, and GR) (Figure 2). AsA-GSH pathway is the heart of antioxidant defense, which mainly detoxify the H2O2 (Hasanuzzaman et al., 2019). The first step of this pathway is catalyzed by APX, which converts H2O2 to water and monodehydroascorbate radical (MDHA) using AsA as the electron donor. The combination of salinity (100 mM NaCl) and heat (42°C; 4 h/day) stress greatly enhanced the activity of APX to detoxify H2O2 and prevent oxidative damage in tomato plants (Sousa et al., 2022). MDHAR catalyzes the reduction of primary oxidation MDHA to AsA, thus maintaining the AsA pool. Overexpression of tomato chloroplast MDHAR elevates the AsA levels, sustains APX activity and accelerates the reductive detoxification of H2O2, therefore enhancing the tolerance to osmotic stress induced by salt and polyethylene glycol (PEG) (Li et al., 2012). The PA mediator transglutaminase (TGase) increases the PAs accumulation, that further promotes the activity of antioxidant enzymes (SOD, APX, MDHAR, and CAT), which reduces salt-induced oxidative damage in tomato (Zhong et al., 2020). The powerful antioxidant system of wild tomato mitigates salt-induced oxidative damage by the high expression of defense genes with the enhanced activities of antioxidant enzymes (Kashyap et al., 2020a). Through DHAR, GSH converts the oxidized form dehydroascorbate (DHA) to the reduced form AsA, yielding oxidized glutathione (GSSG). Finally, GSSG is reduced to GSH by GR using NADPH as the electron donor (Hao et al., 2021). Both GSH-Px and GST use GSH pool to detoxify H2O2 by catalyzing its conjugation with GSH. In tomato chloroplasts, exogenous GSH alleviates salt stress by improving the content of endogenous GSH, GSH/GSSH ratio and activities of H2O2-scavenging enzymes, but the detailed mechanism of GSH-induced salt stress amelioration and detoxification awaits further study (Zhou et al., 2018).
Non-enzymatic scavenging system
The already mentioned AsA and GSH, as well as Pro, carotenoids, flavonoids, and tocopherol, are non-enzymatic antioxidants that play a role in ROS detoxification and retrograde signaling (Czarnocka and Karpiñski, 2018). AsA provides electrons for various antioxidant defense reactions, and participates in redox signal transduction and enzymatic activity regulation. The AsA accumulation enhances oxidative stress tolerance in tomato (Li et al., 2019c), and the inhibition of AsA oxidase increases AsA content and salt tolerance in cherry tomato (Abdelgawad et al., 2019). Seed priming with AsA enhances salt tolerance by modulation of antioxidant mechanisms (Alves et al., 2021). GSH plays an important antioxidant role as the reductant of ROS and the substrate for some peroxidase. Exogenous GSH increases tomato resistance to salt-induced oxidative stress by maintaining the homeostasis of cellular redox, cellular ion, and PAs (Zhou et al., 2017, 2019). Tocopherol can effectively scavenge ROS and lipid free radicals, and is a protector of biological membranes. Tocopherol in chloroplasts of tomato functions as an antioxidant under the moderate stress (50 mM NaCl) similarly as in the early phase of severe stress (150 mM NaCl for 2 days). While in the late phase of severe stress (150 mM NaCl for 5 days), it may be involved in senescence signaling pathway (Skłodowska et al., 2009). Carotenoids are essential components of the photosynthetic antenna and reaction center complex, and exhibit antioxidant activity by protecting the photosynthetic machinery. The key carotenoid-related genes and carotenoid biosynthesis in mature-green fruits of tomato are enhanced by salinity (Leiva-Ampuero et al., 2020). Flavonoids are thought to be antioxidants in photoprotection. Chloroplast-located flavonoids scavenge 1O2 under stress and inhibit cellular lipid peroxidation (Czarnocka and Karpiñski, 2018). The total flavonoid content is positively correlated with the salt tolerance of tomato, denoting the underlying role of flavonoids for enhancing salt tolerance (Alam et al., 2021). The osmotic regulator Pro is also an antioxidant with the ability of scavenging free radicals and inhibiting lipid peroxidation. The foliar spray of low concentration of Pro significantly increases Pro and total soluble protein contents and the glutamine synthetase activity, and enhances the salinity tolerance of tomato under field conditions (Kahlaoui et al., 2018). The Pro and AsA pathways synergistically maintain cellular redox homeostasis in tomato plants under the combination of salinity and heat (Lopez-Delacalle et al., 2021).
Phytohormones play important roles in salt stress-induced ROS signaling and scavenging pathways. Exogenous ABA promotes the accumulation of Pro and soluble sugar, reduces the content of ROS, and improves the ability of the antioxidant enzyme system in tomato plants under saline-alkaline stress (Xu et al., 2022). SA decreases the tomato mitochondrial hexokinases (HXKs) transcription and activity, which contributes to mitochondrial ROS production (Poór et al., 2019). The ROS accumulation is directly controlled by ET signaling triggered by salt stress. However, ET cannot influence the ROS generated by SA in tomato cell suspension (Poór et al., 2013). Brassinolide (BR)-induced H2O2 generation can stimulate ET biosynthesis, and ET can also promote H2O2 generation. While ET signaling pathway participates in BR-induced salt tolerance in tomato by reducing oxidative damage and enhancing antioxidant enzyme capacity (Zhu et al., 2016). Defective jasmonic acid (JA) synthesis in tomato plants under salt stress is associated with the reduced activity of enzymatic and non-enzymatic antioxidants (Abouelsaad and Renault, 2018). The roles of cross-talk among phytohormones in salt stress-induced ROS signaling and scavenging pathways in tomato need to be further investigated.
Gamma-aminobutyric acid metabolic pathway, also known as GABA shunt, is probably involved in salt tolerance of tomato by modulating amino acid synthesis and ROS metabolism. Exogenous GABA has positive effects on alleviating salt stress, which is mainly due to induced osmotic regulation and antioxidant metabolism by the salt- and exogenous GABA-induced endogenous GABA (Wu et al., 2020; Figure 2). In GABA shunt, glutamate decarboxylase (GAD) catalyzes the decarboxylation of glutamate to GABA, GABA transaminase (GABA-T) converts GABA to succinic semialdehyde (SSA), and SSA dehydrogenase (SSADH) catalyzes the oxidation of SSA to succinate. The enhanced ROS accumulation and increased salt sensitivity in GADs- and GABA-Ts-silenced tomato plants are possibly attributed to the impaired function of mitochondrial electron transport chain caused by the decreased succinate. However, SSADH-silenced plants exhibited less sensitiveness to salt stress, that may possibly due to the similar levels of ROS between silenced and control plants under salt stress (Bao et al., 2015).
Epigenetic regulation
Epigenetic modifications, also known as chromatin modifications, contain DNA methylation, RNA-directed DNA methylation (RdDM), and histone modifications (Chinnusamy and Zhu, 2009; Deinlein et al., 2014). Epigenetic modifications regulate stress-responsive gene expression and plant development, conferring stress adaptation (Chinnusamy and Zhu, 2009; Figure 2).
DNA methylation is primarily catalyzed by the DNA methyltransferases (MTases) family. A total of 9 tomato MTases genes are identified, including 1 methyltransferase (MET) member (MET1), 3 chromomethylase (CMT) members (CMT2, CMT3, and CMT4), 4 domains rearranged methyltransferase (DRM) members (DRM5, DRM6, DRM7, and DRM8), and 1 DNA methyltransferase homolog 2 (DNMT2) member (METL). Except for MET1 and DRM8, all other MTase genes are significantly regulated by salt stress, suggesting that tomato MTase genes may be involved in salt stress response (Guo et al., 2020). PKE1 is a Pro-, lysine-, and glutamic-rich protein gene, and the lower expression level in tomato leaves is consistent with the hypermethylation of its coding sequence. Tomato PKE1 confers the salt tolerance involved in post-transcriptional regulation through binding to F-box proteins (Li et al., 2019a). The functions of heavy methylation in the promoter of PKE1 in tomato fruit and leaf salt responses remain unclear (Li et al., 2019a; Liu and He, 2020).
Small RNAs play the pivotal role in environmental stress responses of crop plants by regulating gene expression, and their generation mainly depends on proteins encoded by respective Dicer-like (DCL), Argonaute (AGO), and RNA-dependent RNA polymerases (RDR) gene families (Huang et al., 2016). Arabidopsis AtAGO4 is required for RdDM of the SUPERMAN (SUP) locus (Zilberman et al., 2003). In tomato, a total of 15 AGO proteins are identified, and 4 members are orthologs of AtAGO4, namely AGO4A-AGO4D (Xian et al., 2013). Tomato AGO4A is significantly induced by salt and drought stress (Bai et al., 2012). Down-regulation of AGO4A conferred enhanced salt and drought tolerance in transgenic tomato by reducing the transcript levels of DNA MTase genes (DRMs) and RNA silencing pathway genes, suggesting that tomato AGO4A as a core factor of RdDM pathway plays a negative role under salt and drought stress probably through regulating methylation process-associated genes (Huang et al., 2016).
In general, histone modifications are associated with changes in stress-induced gene regulation (Chinnusamy and Zhu, 2009). Histone post-translational modifications (HPTMs) include acetylation and methylation, acetylation is dynamically regulated by histone acetylases (HATs) and histone deacetylases (HDACs), and methylation is balanced by the activities of histone methylases (HMTs) and histone demethylases (HDMs). Plant HDACs are divided into three subfamilies: RDP3/HDA1 (Reduced Potassium Dependence 3/Histone Deacetylase 1, hereinafter named HDAs), plant-specific HD2s (Histone Deacetylase 2), and SIR2 (Silent Information Regulator 2) (Guo et al., 2017), and the members of HDA subfamily share sequence homology in the HDAC domain and require the Zn2+ cofactor for deacetylase activity (Yang and Seto, 2007; Zhao et al., 2015). There are 124 histone modifiers (HMs) in tomato, including 32 HATs, 14 HDACs (9 HDAs, 3 HD2s, and 2 SIR2s), 52 HMTs, and 26 HDMs (Aiese Cigliano et al., 2013). Among nine tomato HDA genes (named as HDA1-HDA9), the expression levels of HDA1, HDA4, and HDA9 in root and that of HDA3 in leaf were significantly stimulated by slat treatment, and the levels of HDA2, HDA5, and HDA6 were induced both in roots and leaves (Guo et al., 2017). The HDA5-silenced tomato plants exhibited reduced tolerance to salt stress (Yu et al., 2018), while the stress-related genes modified by HDA5 through histone deacetylation under salt conditions remains to be identified.
Not only HDACs but also HATs are implicated in regulation of salt tolerance. In Arabidopsis, HAT general control non-repressed protein 5 (GCN5)-mediated acetylation of lysine 9 (H3K9) and lysine 14 of histone H3 (H3K14) is associated with activation of cellulose synthesis genes CTL1 (chitinase-like gene), PGX3 (polygalacturonase involved in expansion-3) and MYB54 (MYB domain protein-54) under salinity. The severe growth inhibition and defects in cell wall integrity phenotypes of Arabidopsis gcn5 mutant under salt stress can be partially rescued by overexpression of chitinase-like protein CTL1 and can be almost fully recaptured by constitutive wheat TaGCN5 expression. GCN5 enhances salt tolerance through activating cellulose synthesis genes and GCN5-mediated salt tolerance may be conserved between monocot and dicot plants (Zheng et al., 2019). Tomato GCN5 can catalyze acetylation on histone H3 at H3K9 and H3K14 residues and constitutive GCN5 expression almost fully rescues growth defect phenotype of Arabidopsis null-mutant gcn5-7, indicating that tomato GCN5 functions similarly as AtGCN5 in developmental processes (Hawar et al., 2022). Nonetheless, the function of GCN5 in tomato salt tolerance requires further elucidation.
Gene expression changes
Plants generate salt tolerance mechanisms to minimize the adverse effects of salt stress by regulating the expression of salt response genes. Comprehensive analysis of gene expression profiles under salt stress by transcriptome technology can provide key clues for elucidating the molecular mechanism of salt stress in tomato. However, genes involved in salt tolerance may occur in wild tomato species but not in cultivated species (Foolad, 2007).
The wild tomato genotype S. pimpinellifolium ‘PI365967’ is more salt tolerant than the cultivar S. lycopersicum ‘Moneymaker’ (Sun et al., 2010). After treatment with 200 mM NaCl for 5 h, the number of differentially expressed genes (DEGs) in Moneymaker (1386) was higher than that in PI365967 (948). Eighty-six genes were specifically up-regulated in PI365966, including the genes encoding salicylic acid-binding protein 2 (SABP2), CIPKs, plasma membrane ATPase 1, peroxidase, lactoylglutathione lyase/glyoxalase I, and TINY-like protein and AP2/ERF domain protein (both belonging to DREB TF superfamily). Additionally, several genes encoding glutathione S-transferase showed significantly higher basal expression in PI365967 than in Moneymaker. This suggests that multiple strategies, such as SA signaling, SOS pathway, transcriptional regulation, ROS scavenging and detoxification synergistically confer salt tolerance in wild tomato. Among the 82 genes specifically down-regulated in PI365967, the gene encoding putative high-affinity nitrate transporter (a repressor of lateral root initiation) was most down-regulated (8-fold), which may promote root growth in PI365967 under salt stress conditions (Sun et al., 2010).
Similarly, another report using transcriptome analysis to reveal salt tolerance mechanisms found that most of the upregulated DEGs were involved in catalysis, transcriptional regulation and molecular transduction in wild tomato S. chilense under 500 mM NaCl conditions. However, the down-regulated DEGs were mainly involved in binding, molecular function regulator and catalysis (Kashyap et al., 2020b). Specifically, genes actively involved in Pro and arginine metabolism, oxidoreductase activity, hormone metabolism, ROS scavenging systems, signaling regulation, transporters, osmotic regulation, defense and stress responses, homeostasis and TFs are significantly up-regulated, and play an important role in salt tolerance of S. chilense. Genes encoding pentatricopeptide repeat-containing protein in S. chilense are significantly down-regulated, possibly defending against salt stress. Interestingly, although the existence of Wnt signaling (Wingless-related integration site) in plant systems is unknown, it plays a crucial role in conferring salt tolerance in S. chilense (Kashyap et al., 2020b). Transcriptome analysis of S. lycopersicum cv. MicroTom under salt (150 mM NaCl for 6 h) and oxidative stress (20 mM H2O2 for 6 h) revealed 6,643 significantly DEGs, including 3,950 DEGs identified under oxidative stress and 4,617 DEGs found under salt stress (Keshishian et al., 2018). Whereas up to 67.6% (2557) of induced and 75.6% (2162) of repressed DEGs show unique stress (salt or oxidative stress) regulation. Of the 33 cytokinin-related DEGs that were significantly regulated by stress, only 10 were regulated by both oxidative and salt stress, suggesting that although there is crosstalk between salt and oxidative stress, the transcriptional patterns of their gene regulation are not identical (Keshishian et al., 2018). Overall, the comparative transcriptomic analysis of tomato cultivars with different salt tolerance can help to improve our understanding on possible molecular mechanisms underlying salt tolerance in tomato.
Approaches for improving salt tolerance of tomato
Genetic engineering
Salt-tolerant transgenic lines have been developed for different metabolic properties, such as ion transport, osmoregulation, antioxidants, stress proteins, and universal stress proteins (TFs and signal transduction) (Ashraf and Munns, 2022). A series of tomato TFs are used to regulate salt tolerance via genetic engineering (Table 1). Overexpression of C2H2 zinc-finger protein ZFs improves salt tolerance by maintaining photosynthesis, increasing PA biosynthesis, and improving the ability of antioxidant AsA-mediated removal of ROS (Hichri et al., 2014; Li Y. et al., 2018). bZIP1, a member of the basic region/leucine zipper (bZIP) family, positively regulates salt tolerance by modulating ABA-mediated signaling pathways (Zhu et al., 2018). Overexpression of basic helix-loop-helix (bHLH) transcription factor bHLH22 enhances the resistance to salinity and drought by increasing osmotic potential, augmenting the accumulation of flavonoids and ABA, and improving the active oxygen scavenging system (Waseem et al., 2019). Tomato plants overexpressing WRKY8 improves salinity tolerance through enhancing the transcriptional levels of stress-responsive genes, Pro accumulating and activities of ROS-scavenging enzymes (Gao et al., 2020). Overexpressing MYB102 confers salt tolerance by regulating Na+/K+ homeostasis, ROS scavenging ability, and expression of salt stress-related genes (Zhang et al., 2020b). NAC transcription factor TAF1 increases the accumulation of Pro and Na+ ions in shoots, upregulates salt stress-responsive and ABA biosynthesis genes, and reduces stomatal conductance and stomatal pore area, thereby conferring salt tolerance (Devkar et al., 2020).
By genetic transformation, other genes involved in tomato oxidative stress and ion homeostasis have been shown to improve salt tolerance. RING finger E3 ligase Ring functions as a positive regulator of salt stress signaling through regulating the ion homeostasis of Na+ and K+, levels of H2O2 and lipid peroxidation, and expression of stress-related genes (Qi et al., 2016). Overexpression of the melatonin synthesis-related gene Caffeic Acid O-Methyltransferase 1 (COMT1) increases salt tolerance via maintaining the balance of Na+/K+, decreasing ion damage by activating SOS pathway, enhancing the antioxidant capability, and upregulating stress-related genes (Liu et al., 2019; Sun et al., 2020). Small SALT TOLERANCE ENHANCER1 (STE1) protein promotes ABA-dependent salt stress-responsive pathways by interacting with the ABA receptor PYLs and the positive ABA signaling regulator SnRK2s and by improving Na+ and K+ homeostasis and ROS scavenging (Meng et al., 2020).
Heterologous overexpression of salt stress-related genes can also improve salt tolerance in tomato. For example, overexpressing yeast trehalose-6-phosphate synthase (TPS1) in tomato increases salt tolerance, which is partly due to the promotion of trehalose biosynthesis (Cortina and Culiáñez-Macià, 2005), and the enhanced salt tolerance in tomato overexpressing Arthrobacter globiformis choline oxidase codA is partly attributed to the accumulating GB (Wei et al., 2017).
Although transgenic technology can improve the salt tolerance of plants through a single strategy, the degree of improvement is limited due to the polygenic trait of salt tolerance (Hao et al., 2021). Promising achievements in improving salt tolerance may be achieved by stacking/aggregating multiple genes (Ashraf and Munns, 2022). Precise editing of multi-target genes using clustered regularly interspaced short palindromic repeats (CRISPR)/CRISPR-associated (Cas) technology has emerged as an alternative to traditional plant breeding and transgenic methods (Hanin et al., 2016). As an ideal candidate for CRISPR/Cas9 based gene modulations, tomato has already achieved salt tolerance regulation through genome editing (Chandrasekaran et al., 2021). For instance, both mutant alleles slsos1-1 and slsos1-2 generated by the CRISPR/Cas9 system increase the Na+/K+ ratio in tomato roots and the sensitivity to salt stress (Wang Z. et al., 2021). As a negative regulator of ABA, hybrid proline-rich protein 1 (HyPRP1) plays a negative role in salt tolerance of tomato (Li et al., 2016). The precise elimination of HyPRP1 negative-response domain(s) by CRISPR/Cas protein-based targeted mutagenesis improves salinity tolerance in both germination and vegetative phases (Tran et al., 2021). The application of CRISPR/Cas in tomato also includes enhancing cultivation, promoting growth, alleviating biotic stress, and improving tolerance to abiotic stresses such as drought and temperature. This technology has a good prospect in the breeding and genetic research of tomato (Chandrasekaran et al., 2021). The in-depth understanding of the molecular mechanism of salt stress and the advancement of efficient and precise CRISPR/Cas technology are expected to accelerate the breeding of salt-tolerant tomato varieties with high yields.
Marker-assisted selection
Since salt tolerance is a polygenic trait, traditional breeding methods to improve salt tolerance of crops are time-consuming and labor-intensive, and may introduce undesirable traits while selecting traits. The combination of QTL analysis and marker-assisted selection (MAS) is predicted to be an effective method for simplifying this process (Hanin et al., 2016). MAS can be used in the selection of lines following a crossing program and without the need to evaluate performance of plants under stress (Munns et al., 2002; Flowers and Flowers, 2005). Through association analysis of molecular markers and salt stress phenotypes can not only assess the molecular basis of tomato salt tolerance, but it also provides guidance for the introgression of salt tolerance traits in the target varieties (Gharsallah et al., 2016a; Ezin et al., 2018).
Many QTLs associated with tomato salt response (including plant height, stem diameter, leaf number, leaf and root fresh and dry mass, ion concentration, antioxidant response, and survival rate) have been identified (Foolad, 2007; Villalta et al., 2008; Frary et al., 2010, 2011; Asins et al., 2013). These QTLs may be useful in breeding of the salt tolerant cultivars. Nevertheless, the accuracy and precision of QTL identification, the complexity of genetic and environmental interactions and the lack of evaluation reports under field conditions greatly limit progress in marker-assisted breeding of salt tolerance (Ashraf and Foolad, 2013). Molecular linkage map and identification of salt tolerance related QTLs are the primary requisite for improving the salt tolerance in tomato through MAS and pyramiding, while the next generation sequencing (NGS) helps to obtain high density genetic maps, which promotes MAS to play a substantial role in the molecular breeding of salt-tolerant tomato varieties (Hanin et al., 2016; Kashyap et al., 2021).
Genome-wide association studies can overcome the limitations of traditional QTL mapping, provide higher mapping resolution, and detect multiple alleles at the same locus (Ashraf and Munns, 2022). GWAS has become a more efficient technique for studying genetics underlying trait variation (Morton et al., 2019). Valid phenotypic data is a prerequisite for gene/QTL discovery, association mapping and GWAS (Qin et al., 2020). A GWAS of the root Na+/K+ ratio trait in a tomato population comprising materials from different genetic backgrounds reveals that HAK20 gene is effectively involved in the transport and maintenance of Na+ and K+ homeostasis under salt stress, thereby imparting salt tolerance to tomatoes (Wang et al., 2020). The model of high-throughput phenotype-genotype interaction will greatly facilitate the genetic dissection of salt tolerance-related traits in tomato, paving the way for the development of salt-tolerant lines/genotypes.
Grafting
Grafting is an economically justified and sustainable strategy to overcome saline stress, and offers an alternative to breeding of salt-tolerant tomato (Singh et al., 2020; Table 2). The effect of grafting depends on the characteristics of the scion and rootstock, their interaction and stress intensity (Giordano et al., 2021). Grafting salt-susceptible tomato cultivars onto salt-tolerant rootstock can effectively reduce the adverse effects of salt stress on growth, yield, and fruit quality. The root system of grafted plants is stronger and more efficient in uptake of water and nutrients. In addition, grafted tomato plants improve salt tolerance by reducing ionic stress, increasing the transfer of K+, Ca2+ and Mg2+ to shoots and leaves, and reducing ROS-induced oxidative damage (Koleška et al., 2018; Singh et al., 2020). The salt resistant tomato rootstock greatly reduces the yield loss of the sensitive genotypes and increases fruit quality under saline conditions. Salt-tolerant rootstock also controls the stomatal openness and closure of sensitive scions, improves the osmotic adjustment of leaves, and reduces the transport of accumulation of Na+ ions accumulation to young leaves (Coban et al., 2020).
Intergeneric grafting is a promising approach for enhancing the salinity tolerance of tomato. Grafting on salt tolerant eggplant (Solanum melongena) rootstock increases the average fruit yield and improves salt tolerance of tomato scion. Grafted plants maintain higher relative water content and antioxidant enzyme activities, and balance the salt damage through accumulating Pro. Meanwhile, the eggplant rootstock confers Na+ exclusion and K+ retention properties to the tomato scion (Sanwal et al., 2022). Grafting tomato on potato (Solanum tuberosum) rootstock increases water productivity by 56.8% under saline water-irrigations, significantly alters dry matter allocation, and induces mineral compartmentalization processes (Parthasarathi et al., 2021). Although grafting tomato on halophyte wolfberry (Lycium chinense) can enhance salt tolerance, it reduces the growth and fruit yield of the grafted plants due to the limitation of thinner woody stem (Feng et al., 2019). In conclusion, the selection of suitable rootstocks is a prerequisite for getting the maximum benefit from grafting under salt stress.
Pretreatments
Pretreatments increase the ability of tomato plants to adapt to salinity (Table 2). Seed priming and drought pretreatment have been reviewed elsewhere and will be not covered in this review (Cuartero et al., 2006).
Heat induces salinity tolerance of tomato through improving Na+ and K+ homeostasis, improving water balance, reducing oxidative stress, and increasing efficient photosynthetic performance (Rivero et al., 2014). Heat stress-induced salt tolerance belongs to a phenomenon known as cross-tolerance in plants. Thus, salinity can have a beneficial regulatory role in enhancing tomato tolerance to other stresses, such as protecting it against excessive sulfur toxicity (Jiang et al., 2017), significantly reducing the infection of biotrophic fungus Oidium neolycopersici (Achuo et al., 2006), and synergistically increasing the effect of DL-β-aminobutyric acid (BABA) on triggering systemic resistance in tomato plants against Pseudomonas syringae pv. tomato infection (Baysal et al., 2007). Salinity acclimation reduces the negative effects of salt stress (Kamanga et al., 2020), and moderate salt stress improves tomato fruit quality without decreasing yield (Meza et al., 2020). Elevated CO2 confers tomato tolerance to progressively higher soil salinity and secondary soil salinization by improving antioxidant capacity, ion homeostasis, and PA metabolism, decreasing ABA and ET levels, and suppressing transpiration (Yi et al., 2015; Brito et al., 2020; Zhang et al., 2020c). Furthermore, low red light (R) to far-red light (FR) ratio (R:FR) enhances salinity tolerance in tomato by regulating photosynthesis and ROS scavenging systems (Wang Y. et al., 2021).
Exogenous vanillic acid and quercetin improve salt tolerance by enhancing the action of glyoxalase system (Parvin et al., 2019a,2020). Spermidine and 24-epibrassinolide (EBR) enhance tomato tolerance to salinity and alkalinity stress by regulating PA metabolism (Hu et al., 2012; Zheng et al., 2016). Application of exogenous GSH reduces the level of PAs and promotes the transformation of PAs between different morphologies in tomato seedlings under salinity conditions, contributing to the improvement of salt tolerance (Zhou et al., 2019). Application of omeprazole (benzimidazole proton pump inhibitor) increases nutrient uptake and allocation, enhances photosynthesis and plant performance, thus improving resource use efficiency and salinity tolerance in tomato (Rouphael et al., 2018). Vermicompost leachate reduces the impact of salinity on leaf senescence and enhances salinity tolerance, which is related to the decreased ET synthesis, increased anthocyanin contents, and increased Pro and jasmonate accumulation (Benazzouk et al., 2020). The combined microalgae-cyanobacteria extract formulations (MEF) stimulates tomato plant growth and salt tolerance response through the enhanced antioxidant enzyme activities and the improved root growth and nutrient uptake (Mutale-joan et al., 2021).
Pretreatments may be simpler and more economical than other strategies to improve salt tolerance in tomato. The adaptive mechanism of tomato to salt stress can provide a theoretical basis for pretreatments to improve salt tolerance. The integration of metabolomics and other omics approaches will provide comprehensive insight into the response of tomato to salt stress.
Modulation of the rhizosphere
Interaction with beneficial soil microorganisms improves salt tolerance (Hanin et al., 2016). As a product of the co-evolution between plants and microorganisms, plant growth promoting bacteria (PGPB) represent a new biological pathway for sustainable agriculture to alleviate salt stress (Singh et al., 2018). Inoculating tomato seedlings with endophytic Pseudomonas spp. strain OFT5 decreases salt-induced ET levels, but promotes shoot uptake of the macronutrients and micronutrients, improving plant growth under moderate salt conditions (Win et al., 2018). Tomato plants inoculated with Pseudomonas 16S showed higher biomass accumulation than uninoculated plants. It is the result of the facilitation of Fe acquisition and an increase in the content of ROS-scavenging and antioxidant compounds (Zuluaga et al., 2021). Endophytic halotolerant Bacillus velezensis FMH2 alleviates salt stress on tomato plants by regulating ion accumulation (decreased endogenous Na+ accumulation, increased K+ and Ca2+ uptake) and enhancing antioxidant responses (Masmoudi et al., 2021). Root inoculation with Azotobacter chroococcum 76A not only enhances tomato adaptation to salt stress under low nitrogen conditions, but also promotes nutrient assimilation efficiency under moderate and severe salinity, showing its potential in improved nutrition and salt stress protection (Van Oosten et al., 2018). Comparative transcriptomics and metabolomics reveal that Pseudomonas oryzihabitans AXSa06 mediates salt tolerance in tomato by efficiently activating antioxidant metabolism, by dampening stress signals, by detoxifying Na+, as well as by effectively assimilating carbon and nitrogen (Mellidou et al., 2021).
Arbuscular mycorrhizal fungi (AMF) can alleviate salt stress by enhancing assimilation and uptake of key elements, activating antioxidant systems and photosynthesis, regulating key hormone accumulation, and activating nutrient transporters and enzymes (Giordano et al., 2021). For instance, AMF colonization reduces the expression of aquaporins genes in roots of salt-treated tomato plants, whereas significantly increases their transcript levels in leaves, and thereby presumably regulates water flow in tomato under salt stress (Ouziad et al., 2006). Moreover, AMF colonization increases P and K concentration, decreases Na content, enhances the activities of SOD, CAT, POD and APX, and increases tomato fruit yield by 33.3 and 106% at 50- and 100-mM salinity levels, respectively (Latef and Chaoxing, 2011; Table 2).
Plant growth promoting bacteria and AMF not only act as biofertilizers to improve plant growth in saline soils, but also promote bioremediation of contaminated soils (Mokrani et al., 2020). Co-inoculation of different beneficial microorganisms may further improve salt tolerance (Hanin et al., 2016), which requires more attempts.
Nanobiotechnology
Enhanced ROS scavenging improves salt tolerance in plants. Using nanoparticles (NPs) with ROS scavenging ability is an emerging approach for modulating ROS homeostasis in plants under stress conditions (Liu et al., 2021). Environmentally friendly metal-based nanomaterials can improve salt tolerance in tomato plants (Table 2). Under salinity conditions, foliar application of copper NPs (Cu-NPs) increases the content of phenolic substances in leaves and vitamin C, glutathione and phenolics in fruits, also improves the activities of phenylalanine ammonia lyase (PAL), APX, GSH-Px, SOD, and CAT. Cu-NPs induce salt tolerance of tomato by stimulating the antioxidant mechanism (Pérez-Labrada et al., 2019). Another study confirmed that the application of chitosan-polyvinyl alcohol hydrogels (Cs-PVA) and Cu-NPs activates the antioxidant defense mechanisms of tomato plants and are mediated by the octadecanoid pathway of the jasmonates (Hernández-Hernández et al., 2018). In addition to antioxidant enzyme activities, zinc oxide nanoparticles (ZnO-NPs) also enhance protein content and photosynthetic properties under salt stress, improving growth performance and alleviating the adverse effects of salinity on tomato (Faizan et al., 2021). Notably, combining NPs with other strategies may be more effective in improving salt tolerance in tomato. For example, nano-silicon application combined with grafting enhances shoot and root growth, fruit yield and quality of tomato under salt stress, and increases the contents of mineral, GA3, ABA, and Pro, indicating that this method holds promise as alternative techniques for alleviating salt stress in commercial tomato cultivars (Sayed et al., 2022). Overall, nanobiotechnology has a strong potential to modulate ROS homeostasis in plants and improve salt tolerance, contributing to sustainable agriculture (Liu et al., 2021).
The above-mentioned methods for improving salt tolerance in tomato should be combined with the cultivation approaches adopted by farmers, including crop rotation, selection of sowing and planting dates and harvest times, appropriate irrigation techniques, planting density and mulching films (Giordano et al., 2021). The evaluation of the effect of improving salt tolerance needs to comprehensively consider the yield and quality of tomato under field conditions and the cost-benefit ratio (Hanin et al., 2016; Ashraf and Munns, 2022).
Conclusion and future perspectives
A clear salt tolerance mechanism and the identification of key genes in tomato plants that respond to salt stress will contribute to the fast-track breeding of salt tolerant varieties. In this review, we described the effect of salt stress on tomato growth and development, the mechanisms of tomato response to salinity, and the methods to improve tomato salt tolerance. High salt induces osmotic stress and ionic imbalance, thus inhibiting the growth and photosynthesis of tomato plants. Coping these adverse effects tomato plants have adopted strategies including maintaining ion homeostasis, improving osmotic regulation ability and antioxidant enzyme activities. At present, several problems in the regulation mechanism of salt tolerance in plants need to be resolved, including perception of Na+, transport and detoxification mechanisms of Cl–, mapping of toxicity at cell and tissue levels, new QTLs that improve salt tolerance, and cross-effect of different hormones under salt stress (Ismail and Horie, 2017; Isayenkov and Maathuis, 2019; Zhao et al., 2020).
Tomato has a more stable genetic transformation system, that is conducive to improve its salt tolerance with the introduction of foreign genes via transformation (Cuartero et al., 2006). Although, overexpression of some genes in tomato has improved its salt tolerance, but the development of salt-tolerant varieties through genetic transformation has not been fully understood yet. The evaluation of salt tolerance in transgenic plants under laboratory or greenhouse conditions has little correlation with salt tolerance under field conditions, because the field environment is a compound stress, not just salt stress (Yamaguchi and Blumwald, 2005). Genetic transformation of the tomato transcription factors involved in salt stress may partially solve the problem as in field conditions the transgenic plants do not exhibits outstanding salt tolerance, because transcription factors are generally involved in a various stresses and initiate expression of multiple target genes (Cui et al., 2018; Waseem et al., 2019; Gao et al., 2020). Salt tolerance of tomatoes in the field can be evaluated through unmanned aerial vehicle-based phenotyping using morphometric and spectral analysis (Johansen et al., 2019).
Many genes involved in salt stress response are inducible expression type, while most transgenic plants are obtained by using constitutive promoters to drive the continuous expression of target genes, which may cause unexpected side effects. This could be alleviated by replacing constitutive promoters with stress-induced or tissue-specific promoters (Yamaguchi and Blumwald, 2005). For genetic transformation, special attention should be paid to the genetic background of the transformed plants, which may determine the effectiveness of salt-tolerant genes. For instance, tomato ZF2 enhances salt sensitivity in transgenic Arabidopsis, whereas improves tomato salt tolerance (Hichri et al., 2014), whether this effect is also present in tomatoes with different genetic backgrounds remains to be further studied. Moreover, the safety of transgenic crops is widely questioned worldwide, and traditional breeding costs considerable resources and is a lengthy process (Ismail and Horie, 2017). As the genome editing complex is degraded in the recipient cells, genome editing is defined as non-genetically modified (GM), which is more acceptable to the public, and it can offer products that will be difficult to produce using traditional breeding methods (Kanchiswamy et al., 2015). Although major breakthroughs have been made in tomato genome editing, that mainly focuses on development, metabolism, biotic stresses, and abiotic stresses such as drought, chilling and herbicides (Xu et al., 2019), while studies on tomato salt stress are few and far between. Genome editing can simultaneously edit multiple salt stress-related genes in the same tomato variety, helping to improve salt tolerance.
Many wild tomato species harboring salt-tolerant alleles are excellent natural resources for improving salt tolerance of cultivated tomatoes (Pailles et al., 2020; Wang et al., 2020). Genome editing enables de novo domestication of wild tomato species without causing an associated drag on salt tolerance, accelerating the genetic improvement of wild tomato species (Li T. et al., 2018). Crossing of salt-tolerant wild tomato with salt-sensitive varieties produces segregating populations for studying the genetic structure of salt tolerance (Bai et al., 2018). In addition, salt-tolerant wild tomato species can be used as rootstocks for grafting susceptible but high yielding commercial cultivars (Singh et al., 2020). Overall, in future, we can use GWAS, genome, transcriptome and proteomic analysis to identify the new QTLs and improve the regulatory network of tomato salt stress, optimize the combination of cultivation techniques to improve salt tolerance. We can develop the identification methods of high-throughput phenotyping for tomato salt tolerance, strengthen the utilization of wild salt-tolerant species, adopt efficient gene editing and genetic transformation techniques to carry out precise breeding, and use MAS breeding to improve breeding efficiency, ultimately achieving the goal of producing high-yield and high-quality tomatoes under salinity conditions.
Author contributions
MG, X-MW, Y-MG, and J-SL conceived and designed the manuscript. MG, X-SW, H-DG, and S-YB collected and analyzed the literatures. MG wrote the manuscript. AK, Y-MG, and J-SL reviewed the manuscript. All authors contributed to the article and approved the submitted version.
Funding
This work was supported by the Natural Science Foundation of Ningxia (Grant No. 2021AAC03099), the Horticulture Western First-class Discipline Construction Project of Ningxia University (Grant No. NXYLXK2017B03), and the Science and Technology Major Project of Ningxia (Grant Nos. NXNYYZ20200101 and 2021BBF02024).
Conflict of interest
The authors declare that the research was conducted in the absence of any commercial or financial relationships that could be construed as a potential conflict of interest.
Publisher’s note
All claims expressed in this article are solely those of the authors and do not necessarily represent those of their affiliated organizations, or those of the publisher, the editors and the reviewers. Any product that may be evaluated in this article, or claim that may be made by its manufacturer, is not guaranteed or endorsed by the publisher.
References
Abdelgawad, K. F., El-Mogy, M. M., Mohamed, M. I. A., Garchery, C., and Stevens, R. G. (2019). Increasing ascorbic acid content and salinity tolerance of cherry tomato plants by suppressed expression of the ascorbate oxidase gene. Agronomy 9:51. doi: 10.3390/agronomy9020051
Abouelsaad, I., and Renault, S. (2018). Enhanced oxidative stress in the jasmonic acid-deficient tomato mutant def-1 exposed to NaCl stress. J. Plant Physiol. 226, 136–144. doi: 10.1016/j.jplph.2018.04.009
Achuo, E. A., Prinsen, E., and Höfte, M. (2006). Influence of drought, salt stress and abscisic acid on the resistance of tomato to Botrytis cinerea and Oidium neolycopersici. Plant Pathol. 55, 178–186. doi: 10.1111/j.1365-3059.2005.01340.x
Aiese Cigliano, R., Sanseverino, W., Cremona, G., Ercolano, M. R., Conicella, C., and Consiglio, F. M. (2013). Genome-wide analysis of histone modifiers in tomato: Gaining an insight into their developmental roles. BMC Genomics 14:57. doi: 10.1186/1471-2164-14-57
Alam, M. S., Tester, M., Fiene, G., and Mousa, M. A. A. (2021). Early growth stage characterization and the biochemical responses for salinity stress in tomato. Plants 10:712. doi: 10.3390/plants10040712
Alfosea-Simón, M., Zavala-Gonzalez, E. A., Camara-Zapata, J. M., Martínez-Nicolás, J. J., Simón, I., Simón-Grao, S., et al. (2020). Effect of foliar application of amino acids on the salinity tolerance of tomato plants cultivated under hydroponic system. Sci. Hortic. 272:109509. doi: 10.1016/j.scienta.2020.109509
Almas, H. I., Anwar, S., Kausar, A., Farhat, F., Munawar, M., and Khalizadieh, R. (2021). Exogenous application of methionine and phenylalanine confers salinity tolerance in tomato by concerted regulation of metabolites and antioxidants. J. Soil Sci. Plant Nutr. 21, 3051–3064. doi: 10.1007/s42729-021-00588-9
Alves, R. D. C., Rossatto, D. R., da Silva, J. D. S., Checchio, M. V., de Oliveira, K. R., Oliveira, F. D. A., et al. (2021). Seed priming with ascorbic acid enhances salt tolerance in micro-tom tomato plants by modifying the antioxidant defense system components. Biocatal. Agric. Biotechnol. 31:101927. doi: 10.1016/j.bcab.2021.101927
Ashraf, M., and Foolad, M. R. (2013). Crop breeding for salt tolerance in the era of molecular markers and marker-assisted selection. Plant Breed. 132, 10–20. doi: 10.1111/pbr.12000
Ashraf, M., and Munns, R. (2022). Evolution of approaches to increase the salt tolerance of crops. Crit. Rev. Plant Sci. 41, 128–160. doi: 10.1080/07352689.2022.2065136
Asins, M. J., Villalta, I., Aly, M. M., Olias, R., Álvarez De Morales, P. A. Z., Huertas, R., et al. (2013). Two closely linked tomato HKT coding genes are positional candidates for the major tomato QTL involved in Na+/K+ homeostasis. Plant Cell Environ. 36, 1171–1191. doi: 10.1111/pce.12051
Bacha, H., Ródenas, R., López-Gómez, E., García-Legaz, M. F., Nieves-Cordones, M., Rivero, R. M., et al. (2015). High Ca2+ reverts the repression of high-affinity K+ uptake produced by Na+ in Solanum lycopersycum L. (var. microtom) plants. J. Plant Physiol. 180, 72–79. doi: 10.1016/j.jplph.2015.03.014
Baghour, M., Gálvez, F. J., Sánchez, M. E., Aranda, M. N., Venema, K., and Rodríguez-Rosales, M. P. (2019). Overexpression of LeNHX2 and SlSOS2 increases salt tolerance and fruit production in double transgenic tomato plants. Plant Physiol. Biochem. 135, 77–86. doi: 10.1016/j.plaphy.2018.11.028
Bai, M., Yang, G. S., Chen, W. T., Mao, Z. C., Kang, H. X., Chen, G. H., et al. (2012). Genome-wide identification of Dicer-like, Argonaute and RNA-dependent RNA polymerase gene families and their expression analyses in response to viral infection and abiotic stresses in Solanum lycopersicum. Gene 501, 52–62. doi: 10.1016/j.gene.2012.02.009
Bai, Y., Kissoudis, C., Yan, Z., Visser, R. G., and van der Linden, G. (2018). Plant behaviour under combined stress: Tomato responses to combined salinity and pathogen stress. Plant J. 93, 781–793. doi: 10.1111/tpj.13800
Bao, H., Chen, X., Lv, S., Jiang, P., Feng, J., Fan, P., et al. (2015). Virus-induced gene silencing reveals control of reactive oxygen species accumulation and salt tolerance in tomato by γ-aminobutyric acid metabolic pathway. Plant Cell Environ. 38, 600–613. doi: 10.1111/pce.12419
Baysal, Ö., Gürsoy, Y. Z., Örnek, H., Cetinel, B., and Teixeira da Silva, J. A. (2007). Enhanced systemic resistance to bacterial speck disease caused by Pseudomonas syringae pv. tomato by DL-β-aminobutyric acid under salt stress. Physiol. Plant. 129, 493–506. doi: 10.1111/j.1399-3054.2006.00818.x
Bazihizina, N., Colmer, T. D., Cuin, T. A., Mancuso, S., and Shabala, S. (2019). Friend or foe? Chloride patterning in halophytes. Trends Plant Sci. 24, 142–151. doi: 10.1016/j.tplants.2018.11.003
Belver, A., Olías, R., Huertas, R., and Rodríguez-Rosales, M. P. (2012). Involvement of SlSOS2 in tomato salt tolerance. Bioengineered 3, 298–302. doi: 10.4161/bioe.20796
Benazzouk, S., Dobrev, P. I., Djazouli, Z. E., Motyka, V., and Lutts, S. (2020). Positive impact of vermicompost leachate on salt stress resistance in tomato (Solanum lycopersicum L.) at the seedling stage: A phytohormonal approach. Plant Soil 446, 145–162. doi: 10.1007/s11104-019-04361-x
Borbély, P., Poór, P., and Tari, I. (2020). Changes in physiological and photosynthetic parameters in tomato of different ethylene status under salt stress: Effects of exogenous 1-aminocyclopropane-1-carboxylic acid treatment and the inhibition of ethylene signalling. Plant Physiol. Biochem. 156, 345–356. doi: 10.1016/j.plaphy.2020.09.019
Bouzroud, S., Gasparini, K., Hu, G., Barbosa, M. A. M., Rosa, B. L., Fahr, M., et al. (2020). Down regulation and loss of auxin response factor 4 function using CRISPR/Cas9 alters plant growth, stomatal function and improves tomato tolerance to salinity and osmotic stress. Genes 11:272. doi: 10.3390/genes11030272
Brito, F. A., Pimenta, T. M., Henschel, J. M., Martins, S. C., Zsögön, A., and Ribeiro, D. M. (2020). Elevated CO2 improves assimilation rate and growth of tomato plants under progressively higher soil salinity by decreasing abscisic acid and ethylene levels. Environ. Exp. Bot. 176:104050. doi: 10.1016/j.envexpbot.2020.104050
Cai, X., Zhang, C., Shu, W., Ye, Z., Li, H., and Zhang, Y. (2016). The transcription factor SlDof22 involved in ascorbate accumulation and salinity stress in tomato. Biochem. Biophys. Res. Commun. 474, 736–741. doi: 10.1016/j.bbrc.2016.04.148
Campos, J. F., Cara, B., Pérez-Martín, F., Pineda, B., Egea, I., Flores, F. B., et al. (2016). The tomato mutant ars1 (altered response to salt stress 1) identifies an R1-type MYB transcription factor involved in stomatal closure under salt acclimation. Plant Biotechnol. J. 14, 1345–1356. doi: 10.1111/pbi.12498
Chandrasekaran, M., Boopathi, T., and Paramasivan, M. (2021). A status-quo review on CRISPR-Cas9 gene editing applications in tomato. Int. J. Biol. Macromol. 190, 120–129. doi: 10.1016/j.ijbiomac.2021.08.169
Chen, P., Sun, Y. F., Kai, W. B., Liang, B., Zhang, Y. S., Zhai, X. W., et al. (2016). Interactions of ABA signaling core components (SlPYLs, SlPP2Cs, and SlSnRK2s) in tomato (Solanum lycopersicon). J. Plant Physiol. 205, 67–74. doi: 10.1016/j.jplph.2016.07.016
Chen, T., Shabala, S., Niu, Y., Chen, Z. H., Shabala, L., Meinke, H., et al. (2021). Molecular mechanisms of salinity tolerance in rice. Crop J. 9, 506–520. doi: 10.1016/j.cj.2021.03.005
Chinnusamy, V., and Zhu, J. K. (2009). Epigenetic regulation of stress responses in plants. Curr. Opin. Plant Biol. 12, 133–139. doi: 10.1016/j.pbi.2008.12.006
Cho, J. H., Sim, S. C., and Kim, K. N. (2021). Calcium sensor SlCBL4 associates with SlCIPK24 protein kinase and mediates salt tolerance in Solanum lycopersicum. Plants 10:2173. doi: 10.3390/plants10102173
Coban, A., Akhoundnejad, Y., Dere, S., and Dasgan, H. Y. (2020). Impact of salt-tolerant rootstock on the enhancement of sensitive tomato plant responses to salinity. HortScience 55, 35–39. doi: 10.21273/HORTSCI14476-19
Cortina, C., and Culiáñez-Macià, F. A. (2005). Tomato abiotic stress enhanced tolerance by trehalose biosynthesis. Plant Sci. 169, 75–82. doi: 10.1016/j.plantsci.2005.02.026
Cuartero, J., Bolarin, M. C., Asins, M. J., and Moreno, V. (2006). Increasing salt tolerance in the tomato. J. Exp. Bot. 57, 1045–1058. doi: 10.1093/jxb/erj102
Cui, J., Jiang, N., Zhou, X., Hou, X., Yang, G., Meng, J., et al. (2018). Tomato MYB49 enhances resistance to Phytophthora infestans and tolerance to water deficit and salt stress. Planta 248, 1487–1503. doi: 10.1007/s00425-018-2987-6
Czarnocka, W., and Karpiñski, S. (2018). Friend or foe? Reactive oxygen species production, scavenging and signaling in plant response to environmental stresses. Free Radic. Biol. Med. 122, 4–20. doi: 10.1016/j.freeradbiomed.2018.01.011
D’Amico-Damião, V., Lúcio, J. C. B., Oliveira, R., Gaion, L. A., Barreto, R. F., and Carvalho, R. F. (2021). Cryptochrome 1a depends on blue light fluence rate to mediate osmotic stress responses in tomato. J. Plant Physiol. 258:153374. doi: 10.1016/j.jplph.2021.153374
De la Torre-González, A., Montesinos-Pereira, D., Blasco, B., and Ruiz, J. M. (2018). Influence of the proline metabolism and glycine betaine on tolerance to salt stress in tomato (Solanum lycopersicum L.) commercial genotypes. J. Plant Physiol. 231, 329–336. doi: 10.1016/j.jplph.2018.10.013
Deinlein, U., Stephan, A. B., Horie, T., Luo, W., Xu, G., and Schroeder, J. I. (2014). Plant salt-tolerance mechanisms. Trends Plant Sci. 19, 371–379. doi: 10.1016/j.tplants.2014.02.001
Devkar, V., Thirumalaikumar, V. P., Xue, G. P., Vallarino, J. G., Tureèková, V., Strnad, M., et al. (2020). Multifaceted regulatory function of tomato SlTAF1 in the response to salinity stress. New Phytol. 225, 1681–1698. doi: 10.1111/nph.16247
Egea, I., Pineda, B., Ortíz-Atienza, A., Plasencia, F. A., Drevensek, S., García-Sogo, B., et al. (2018). The SlCBL10 calcineurin B-like protein ensures plant growth under salt stress by regulating Na+ and Ca2+ homeostasis. Plant Physiol. 176, 1676–1693. doi: 10.1104/pp.17.01605
Ezin, V., Dasenka, T., Agbobatinkpo, P. B., Ahanchede, A., and Handa, A. K. (2018). Molecular genetics of salt tolerance in tomato F2 segregating population with the aid of RAPD markers. Agric. Sci. 9, 1553–1568. doi: 10.4236/as.2018.912109
Faizan, M., Bhat, J. A., Chen, C., Alyemeni, M. N., Wijaya, L., Ahmad, P., et al. (2021). Zinc oxide nanoparticles (ZnO-NPs) induce salt tolerance by improving the antioxidant system and photosynthetic machinery in tomato. Plant Physiol. Biochem. 161, 122–130. doi: 10.1016/j.plaphy.2021.02.002
FAO (2011). The state of the world’s land and water resources for Food and Agriculture (SOLAW)—managing systems at risk. Abingdon: Food and Agriculture Organization of the United Nations and Earthscan.
Feng, H. L., Ma, N. N., Meng, X., Zhang, S., Wang, J. R., Chai, S., et al. (2013). A novel tomato MYC-type ICE1-like transcription factor, SlICE1a, confers cold, osmotic and salt tolerance in transgenic tobacco. Plant Physiol. Biochem. 73, 309–320. doi: 10.1016/j.plaphy.2013.09.014
Feng, K., Yu, J., Cheng, Y., Ruan, M., Wang, R., Ye, Q., et al. (2016). The SOD gene family in tomato: Identification, phylogenetic relationships, and expression patterns. Front. Plant Sci. 7:1279. doi: 10.3389/fpls.2016.01279
Feng, W., Kita, D., Peaucelle, A., Cartwright, H. N., Doan, V., Duan, Q., et al. (2018). The FERONIA receptor kinase maintains cell-wall integrity during salt stress through Ca2+ signaling. Curr. Biol. 28, 666–675. doi: 10.1016/j.cub.2018.01.023
Feng, X., Guo, K., Yang, C., Li, J., Chen, H., and Liu, X. (2019). Growth and fruit production of tomato grafted onto wolfberry (Lycium chinense) rootstock in saline soil. Sci. Hortic. 255, 298–305. doi: 10.1016/j.scienta.2019.05.028
Fernández-García, N., Martínez, V., Cerdá, A., and Carvajal, M. (2002). Water and nutrient uptake of grafted tomato plants grown under saline conditions. J. Plant Physiol. 159, 899–905. doi: 10.1078/0176-1617-00652
Flowers, T. J., and Flowers, S. A. (2005). Why does salinity pose such a difficult problem for plant breeders? Agric. Water Manage. 78, 15–24. doi: 10.1016/j.agwat.2005.04.015
Foolad, M. R. (2004). Recent advances in genetics of salt tolerance in tomato. Plant Cell Tiss. Organ Cult. 76, 101–119. doi: 10.1023/B:TICU.0000007308.47608.88
Foolad, M. R. (2007). Genome mapping and molecular breeding of tomato. Int. J. Plant Genomics 2007:64358. doi: 10.1155/2007/64358
Frary, A., Göl, D., Keleş, D., Okmen, B., Pinar, H., Siðva, H. O., et al. (2010). Salt tolerance in Solanum pennellii: Antioxidant response and related QTL. BMC Plant Biol. 10:58. doi: 10.1186/1471-2229-10-58
Frary, A., Keleş, D., Pinar, H., Göl, D., and Doðanlar, S. (2011). NaCl tolerance in Lycopersicon pennellii introgression lines: QTL related to physiological responses. Biol. Plant. 55, 461–468. doi: 10.1007/s10535-011-0111-x
Gálvez, F. J., Baghour, M., Hao, G., Cagnac, O., Rodríguez-Rosales, M. P., and Venema, K. (2012). Expression of LeNHX isoforms in response to salt stress in salt sensitive and salt tolerant tomato species. Plant Physiol. Biochem. 51, 109–115. doi: 10.1016/j.plaphy.2011.10.012
Gao, Y. F., Liu, J. K., Yang, F. M., Zhang, G. Y., Wang, D., Zhang, L., et al. (2020). The WRKY transcription factor WRKY8 promotes resistance to pathogen infection and mediates drought and salt stress tolerance in Solanum lycopersicum. Physiol. Plant. 168, 98–117. doi: 10.1111/ppl.12978
Gapiñska, M., Skłodowska, M., and Gabara, B. (2008). Effect of short-and long-term salinity on the activities of antioxidative enzymes and lipid peroxidation in tomato roots. Acta Physiol. Plant. 30, 11–18. doi: 10.1007/s11738-007-0072-z
Ghanem, M. E., van Elteren, J., Albacete, A., Quinet, M., Martínez-Andújar, C., Kinet, J. M., et al. (2009). Impact of salinity on early reproductive physiology of tomato (Solanum lycopersicum) in relation to a heterogeneous distribution of toxic ions in flower organs. Funct. Plant Biol. 36, 125–136. doi: 10.1071/FP08256
Gharsallah, C., Fakhfakh, H., Grubb, D., and Gorsane, F. (2016b). Effect of salt stress on ion concentration, proline content, antioxidant enzyme activities and gene expression in tomato cultivars. AoB Plants 8:plw055. doi: 10.1093/aobpla/plw055
Gharsallah, C., Abdelkrim, A. B., Fakhfakh, H., Salhi-Hannachi, A., and Gorsane, F. (2016a). SSR marker-assisted screening of commercial tomato genotypes under salt stress. Breed. Sci. 66, 823–830. doi: 10.1270/jsbbs.16112
Giordano, M., Petropoulos, S. A., and Rouphael, Y. (2021). Response and defence mechanisms of vegetable crops against drought, heat and salinity stress. Agriculture 11:463. doi: 10.3390/agriculture11050463
Guo, J. E., Hu, Z., Guo, X., Zhang, L., Yu, X., Zhou, S., et al. (2017). Molecular characterization of nine tissue-specific or stress-responsive genes of histone deacetylase in tomato (Solanum lycopersicum). J. Plant Growth Regul. 36, 566–577. doi: 10.1007/s00344-016-9660-8
Guo, X., Chen, G., Cui, B., Gao, Q., Guo, J. E., Li, A., et al. (2016). Solanum lycopersicum agamous-like MADS-box protein AGL15-like gene, SlMBP11, confers salt stress tolerance. Mol. Breed. 36:125. doi: 10.1007/s11032-016-0544-1
Guo, X., Xie, Q., Li, B., and Su, H. (2020). Molecular characterization and transcription analysis of DNA methyltransferase genes in tomato (Solanum lycopersicum). Genet. Mol. Biol. 43:e20180295. doi: 10.1590/1678-4685-GMB-2018-0295
Guo, Y., Qiu, Q. S., Quintero, F. J., Pardo, J. M., Ohta, M., Zhang, C., et al. (2004). Transgenic evaluation of activated mutant alleles of sos2 reveals a critical requirement for its kinase activity and C-terminal regulatory domain for salt tolerance in Arabidopsis thaliana. Plant Cell 16, 435–449. doi: 10.1105/tpc.019174
Gupta, B., and Huang, B. (2014). Mechanism of salinity tolerance in plants: Physiological, biochemical, and molecular characterization. Int. J. Genomics 2014:701596. doi: 10.1155/2014/701596
Hanin, M., Ebel, C., Ngom, M., Laplaze, L., and Masmoudi, K. (2016). New insights on plant salt tolerance mechanisms and their potential use for breeding. Front. Plant Sci. 7:1787. doi: 10.3389/fpls.2016.01787
Hao, S., Wang, Y., Yan, Y., Liu, Y., Wang, J., and Chen, S. (2021). A review on plant responses to salt stress and their mechanisms of salt resistance. Horticulturae 7:132. doi: 10.3390/horticulturae7060132
Hao, Y., Huang, B., Jia, D., Mann, T., Jiang, X., Qiu, Y., et al. (2018). Identification of seven polyamine oxidase genes in tomato (Solanum lycopersicum L.) and their expression profiles under physiological and various stress conditions. J. Plant Physiol. 228, 1–11. doi: 10.1016/j.jplph.2018.05.004
Hasanuzzaman, M., Bhuyan, M. B., Anee, T. I., Parvin, K., Nahar, K., Mahmud, J. A., et al. (2019). Regulation of ascorbate-glutathione pathway in mitigating oxidative damage in plants under abiotic stress. Antioxidants 8:384. doi: 10.3390/antiox8090384
Hawar, A., Xiong, S., Yang, Z., and Sun, B. (2022). Histone acetyltransferase SlGCN5 regulates shoot meristem and flower development in Solanum lycopersicum. Front. Plant Sci. 12:805879. doi: 10.3389/fpls.2021.805879
He, Y., Zhou, J., Hu, Y., Fang, C., Yu, Y., Yang, J., et al. (2021). Overexpression of sly-miR398b increased salt sensitivity likely via regulating antioxidant system and photosynthesis in tomato. Environ. Exp. Bot. 181:104273. doi: 10.1016/j.envexpbot.2020.104273
Hernández-Hernández, H., Juárez-Maldonado, A., Benavides-Mendoza, A., Ortega-Ortiz, H., Cadenas-Pliego, G., Sánchez-Aspeytia, D., et al. (2018). Chitosan-PVA and copper nanoparticles improve growth and overexpress the SOD and JA genes in tomato plants under salt stress. Agronomy 8:175. doi: 10.3390/agronomy8090175
Hichri, I., Muhovski, Y., Žižková, E., Dobrev, P. I., Franco-Zorrilla, J. M., Solano, R., et al. (2014). The Solanum lycopersicum zinc Finger2 cysteine-2/histidine-2 repressor-like transcription factor regulates development and tolerance to salinity in tomato and Arabidopsis. Plant Physiol. 164, 1967–1990. doi: 10.1104/pp.113.225920
Hichri, I., Muhovski, Y., Žižková, E., Dobrev, P. I., Gharbi, E., Franco-Zorrilla, J. M., et al. (2017). The Solanum lycopersicum WRKY3 transcription factor SlWRKY3 is involved in salt stress tolerance in tomato. Front. Plant Sci. 8:1343. doi: 10.3389/fpls.2017.01343
Hu, J., Chen, G., Yin, W., Cui, B., Yu, X., Lu, Y., et al. (2017). Silencing of SlHB2 improves drought, salt stress tolerance, and induces stress-related gene expression in tomato. J. Plant Growth Regul. 36, 578–589. doi: 10.1007/s00344-017-9664-z
Hu, X., Zhang, Y., Shi, Y., Zhang, Z., Zou, Z., Zhang, H., et al. (2012). Effect of exogenous spermidine on polyamine content and metabolism in tomato exposed to salinity–alkalinity mixed stress. Plant Physiol. Biochem. 57, 200–209. doi: 10.1016/j.plaphy.2012.05.015
Huang, W., Xian, Z., Hu, G., and Li, Z. (2016). SlAGO4A, a core factor of RNA-directed DNA methylation (RdDM) pathway, plays an important role under salt and drought stress in tomato. Mol. Breed. 36:28. doi: 10.1007/s11032-016-0439-1
Huang, Z., Zhang, Z., Zhang, X., Zhang, H., Huang, D., and Huang, R. (2004). Tomato TERF1 modulates ethylene response and enhances osmotic stress tolerance by activating expression of downstream genes. FEBS Lett. 573, 110–116. doi: 10.1016/j.febslet.2004.07.064
Huertas, R., Olias, R., Eljakaoui, Z., Gálvez, F. J., Li, J. U. N., De Morales, P. A., et al. (2012). Overexpression of SlSOS2 (SlCIPK24) confers salt tolerance to transgenic tomato. Plant Cell Environ. 35, 1467–1482. doi: 10.1111/j.1365-3040.2012.02504.x
Huertas, R., Rubio, L., Cagnac, O., García-Sánchez, M. J., Alché, J. D. D., Venema, K., et al. (2013). The K+/H+ antiporter LeNHX2 increases salt tolerance by improving K+ homeostasis in transgenic tomato. Plant Cell Environ. 36, 2135–2149. doi: 10.1111/pce.12109
Isayenkov, S. V., and Maathuis, F. J. (2019). Plant salinity stress: Many unanswered questions remain. Front. Plant Sci. 10:80. doi: 10.3389/fpls.2019.00080
Ismail, A. M., and Horie, T. (2017). Genomics, physiology, and molecular breeding approaches for improving salt tolerance. Annu. Rev. Plant Biol. 68, 405–434. doi: 10.1146/annurev-arplant-042916-040936
Jaime-Pérez, N., Pineda, B., García-Sogo, B., Atares, A., Athman, A., Byrt, C. S., et al. (2017). The sodium transporter encoded by the HKT1;2 gene modulates sodium/potassium homeostasis in tomato shoots under salinity. Plant Cell Environ. 40, 658–671. doi: 10.1111/pce.12883
Jia, C., Zhao, S., Bao, T., Zhao, P., Peng, K., Guo, Q., et al. (2021). Tomato BZR/BES transcription factor SlBZR1 positively regulates BR signaling and salt stress tolerance in tomato and Arabidopsis. Plant Sci. 302:110719. doi: 10.1016/j.plantsci.2020.110719
Jia, H., Chen, S., Liu, D., Liesche, J., Shi, C., Wang, J., et al. (2018). Ethylene-induced hydrogen sulfide negatively regulates ethylene biosynthesis by persulfidation of ACO in tomato under osmotic stress. Front. Plant Sci. 9:1517. doi: 10.3389/fpls.2018.01517
Jiang, Y., Ding, X., Zhang, D., Deng, Q., Yu, C. L., Zhou, S., et al. (2017). Soil salinity increases the tolerance of excessive sulfur fumigation stress in tomato plants. Environ. Exp. Bot. 133, 70–77. doi: 10.1016/j.envexpbot.2016.10.002
Jiang, Z., Zhou, X., Tao, M., Yuan, F., Liu, L., Wu, F., et al. (2019). Plant cell-surface GIPC sphingolipids sense salt to trigger Ca2+ influx. Nature 572, 341–346. doi: 10.1038/s41586-019-1449-z
Johansen, K., Morton, M. J., Malbeteau, Y. M., Aragon, B., Al-Mashharawi, S. K., Ziliani, M. G., et al. (2019). Unmanned aerial vehicle-based phenotyping using morphometric and spectral analysis can quantify responses of wild tomato plants to salinity stress. Front. Plant Sci. 10:370. doi: 10.3389/fpls.2019.00370
Julkowska, M. M., and Testerink, C. (2015). Tuning plant signaling and growth to survive salt. Trends Plant Sci. 20, 586–594. doi: 10.1016/j.tplants.2015.06.008
Kahlaoui, B., Hachicha, M., Misle, E., Fidalgo, F., and Teixeira, J. (2018). Physiological and biochemical responses to the exogenous application of proline of tomato plants irrigated with saline water. J. Saudi Soc. Agric. Sci. 17, 17–23. doi: 10.1016/j.jssas.2015.12.002
Kamanga, R. M., Echigo, K., Yodoya, K., Mekawy, A. M. M., and Ueda, A. (2020). Salinity acclimation ameliorates salt stress in tomato (Solanum lycopersicum L.) seedlings by triggering a cascade of physiological processes in the leaves. Sci. Hortic. 270:109434. doi: 10.1016/j.scienta.2020.109434
Kanchiswamy, C. N., Sargent, D. J., Velasco, R., Maffei, M. E., and Malnoy, M. (2015). Looking forward to genetically edited fruit crops. Trends Biotechnol. 33, 62–64. doi: 10.1016/j.tibtech.2014.07.003
Kashyap, S. P., Kumari, N., Mishra, P., Moharana, D. P., and Aamir, M. (2021). Tapping the potential of Solanum lycopersicum L. pertaining to salinity tolerance: Perspectives and challenges. Genet. Resour. Crop Evol. 68, 2207–2233. doi: 10.1007/s10722-021-01174-9
Kashyap, S. P., Kumari, N., Mishra, P., Moharana, D. P., Aamir, M., Singh, B., et al. (2020a). Transcriptional regulation-mediating ROS homeostasis and physio-biochemical changes in wild tomato (Solanum chilense) and cultivated tomato (Solanum lycopersicum) under high salinity. Saudi J. Biol. Sci. 27, 1999–2009. doi: 10.1016/j.sjbs.2020.06.032
Kashyap, S. P., Prasanna, H. C., Kumari, N., Mishra, P., and Singh, B. (2020b). Understanding salt tolerance mechanism using transcriptome profiling and de novo assembly of wild tomato Solanum chilense. Sci. Rep. 10:15835. doi: 10.1038/s41598-020-72474-w
Keshishian, E. A., Hallmark, H. T., Ramaraj, T., Plaèková, L., Sundararajan, A., Schilkey, F., et al. (2018). Salt and oxidative stresses uniquely regulate tomato cytokinin levels and transcriptomic response. Plant Direct 2:e00071. doi: 10.1002/pld3.71
Khan, M. N., Mukherjee, S., Al-Huqail, A. A., Basahi, R. A., Ali, H. M., Al-Munqedhi, B. M., et al. (2021). Exogenous potassium (K+) positively regulates Na+/H+ antiport system, carbohydrate metabolism, and ascorbate–glutathione cycle in H2S-dependent manner in NaCl-stressed tomato seedling roots. Plants 10:948. doi: 10.3390/plants10050948
Khelil, A., Menu, T., and Ricard, B. (2007). Adaptive response to salt involving carbohydrate metabolism in leaves of a salt-sensitive tomato cultivar. Plant Physiol. Biochem. 45, 551–559. doi: 10.1016/j.plaphy.2007.05.003
Koleška, I., Hasanagiæ, D., Todoroviæ, V., Murtiæ, S., and Maksimoviæ, I. (2018). Grafting influence on the weight and quality of tomato fruit under salt stress. Ann. Appl. Biol. 172, 187–196. doi: 10.1111/aab.12411
Kong, F., Wang, J., Cheng, L., Liu, S., Wu, J., Peng, Z., et al. (2012). Genome-wide analysis of the mitogen-activated protein kinase gene family in Solanum lycopersicum. Gene 499, 108–120. doi: 10.1016/j.gene.2012.01.048
Kronzucker, H. J., and Britto, D. T. (2011). Sodium transport in plants: A critical review. New Phytol. 189, 54–81. doi: 10.1111/j.1469-8137.2010.03540.x
Latef, A., and Chaoxing, H. (2011). Effect of arbuscular mycorrhizal fungi on growth, mineral nutrition, antioxidant enzymes activity and fruit yield of tomato grown under salinity stress. Sci. Hortic. 127, 228–233. doi: 10.1016/j.scienta.2010.09.020
Leiva-Ampuero, A., Agurto, M., Matus, J. T., Hoppe, G., Huidobro, C., Inostroza-Blancheteau, C., et al. (2020). Salinity impairs photosynthetic capacity and enhances carotenoid-related gene expression and biosynthesis in tomato (Solanum lycopersicum L. cv. Micro-Tom). PeerJ 8:e9742. doi: 10.7717/peerj.9742
Li, D., Ma, N. N., Wang, J. R., Yang, D. Y., Zhao, S. J., and Meng, Q. W. (2013). Overexpression of tomato enhancer of SOS3-1 (LeENH1) in tobacco enhanced salinity tolerance by excluding Na+ from the cytosol. Plant Physiol. Biochem. 70, 150–158. doi: 10.1016/j.plaphy.2013.05.014
Li, F., Wu, Q. Y., Duan, M., Dong, X. C., Li, B., and Meng, Q. W. (2012). Transgenic tomato plants overexpressing chloroplastic monodehydroascorbate reductase are resistant to salt-and PEG-induced osmotic stress. Photosynthetica 50, 120–128. doi: 10.1007/s11099-012-0021-y
Li, J., Ouyang, B., Wang, T., Luo, Z., Yang, C., Li, H., et al. (2016). HyPRP1 gene suppressed by multiple stresses plays a negative role in abiotic stress tolerance in tomato. Front. Plant Sci. 7:967. doi: 10.3389/fpls.2016.00967
Li, S., Wang, X., Liu, X., Thompson, A. J., and Liu, F. (2022). Elevated CO2 and high endogenous ABA level alleviate PEG-induced short-term osmotic stress in tomato plants. Environ. Exp. Bot. 194:104763. doi: 10.1016/j.envexpbot.2021.104763
Li, T., Yang, X., Yu, Y., Si, X., Zhai, X., Zhang, H., et al. (2018). Domestication of wild tomato is accelerated by genome editing. Nat. Biotechnol. 36, 1160–1163. doi: 10.1038/nbt.4273
Li, X., Li, Y., Ahammed, G. J., Zhang, X. N., Ying, L., Zhang, L., et al. (2019b). RBOH1-dependent apoplastic H2O2 mediates epigallocatechin-3-gallate-induced abiotic stress tolerance in Solanum lycopersicum L. Environ. Exp. Bot. 161, 357–366. doi: 10.1016/j.envexpbot.2018.11.013
Li, X., Ye, J., Munir, S., Yang, T., Chen, W., Liu, G., et al. (2019c). Biosynthetic gene pyramiding leads to ascorbate accumulation with enhanced oxidative stress tolerance in tomato. Int. J. Mol. Sci. 20:1558. doi: 10.3390/ijms20071558
Li, J., Chen, C., Wei, J., Pan, Y., Su, C., and Zhang, X. (2019a). SpPKE1, a multiple stress-responsive gene confers salt tolerance in tomato and tobacco. Int. J. Mol. Sci. 20:2478. doi: 10.3390/ijms20102478
Li, Y., Chu, Z., Luo, J., Zhou, Y., Cai, Y., Lu, Y., et al. (2018). The C2H2 zinc-finger protein SlZF3 regulates AsA synthesis and salt tolerance by interacting with CSN5B. Plant Biotechnol. J. 16, 1201–1213. doi: 10.1111/pbi.12863
Li, Z., Zhang, L., Wang, A., Xu, X., and Li, J. (2013). Ectopic overexpression of SlHsfA3, a heat stress transcription factor from tomato, confers increased thermotolerance and salt hypersensitivity in germination in transgenic Arabidopsis. PLoS One 8:e54880. doi: 10.1371/journal.pone.0054880
Liu, C., Mao, B., Yuan, D., Chu, C., and Duan, M. (2022). Salt tolerance in rice: Physiological responses and molecular mechanisms. Crop J. 10, 13–25. doi: 10.1016/j.cj.2021.02.010
Liu, D. D., Sun, X. S., Liu, L., Shi, H. D., Chen, S. Y., and Zhao, D. K. (2019). Overexpression of the melatonin synthesis-related gene SlCOMT1 improves the resistance of tomato to salt stress. Molecules 24:1514. doi: 10.3390/molecules24081514
Liu, J., Fu, C., Li, G., Khan, M. N., and Wu, H. (2021). ROS homeostasis and plant salt tolerance: Plant nanobiotechnology updates. Sustainability 13:3552. doi: 10.3390/su13063552
Liu, J., and He, Z. (2020). Small DNA methylation, big player in plant abiotic stress responses and memory. Front. Plant Sci. 11:595603. doi: 10.3389/fpls.2020.595603
Liu, M., Yu, H., Ouyang, B., Shi, C., Demidchik, V., Hao, Z., et al. (2020). NADPH oxidases and the evolution of plant salinity tolerance. Plant Cell Environ. 43, 2957–2968. doi: 10.1111/pce.13907
Lopez-Delacalle, M., Silva, C. J., Mestre, T. C., Martinez, V., Blanco-Ulate, B., and Rivero, R. M. (2021). Synchronization of proline, ascorbate and oxidative stress pathways under the combination of salinity and heat in tomato plants. Environ. Exp. Bot. 183:104351. doi: 10.1016/j.envexpbot.2020.104351
Lovelli, S., Scopa, A., Perniola, M., Di Tommaso, T., and Sofo, A. (2012). Abscisic acid root and leaf concentration in relation to biomass partitioning in salinized tomato plants. J. Plant Physiol. 169, 226–233. doi: 10.1016/j.jplph.2011.09.009
Lv, X., Chen, S., and Wang, Y. (2019). Advances in understanding the physiological and molecular responses of sugar beet to salt stress. Front. Plant Sci. 10:1431. doi: 10.3389/fpls.2019.01431
Masmoudi, F., Tounsi, S., Dunlap, C. A., and Trigui, M. (2021). Endophytic halotolerant Bacillus velezensis FMH2 alleviates salt stress on tomato plants by improving plant growth and altering physiological and antioxidant responses. Plant Physiol. Biochem. 165, 217–227. doi: 10.1016/j.plaphy.2021.05.025
Massaretto, I. L., Albaladejo, I., Purgatto, E., Flores, F. B., Plasencia, F., Egea-Fernández, J. M., et al. (2018). Recovering tomato landraces to simultaneously improve fruit yield and nutritional quality against salt stress. Front. Plant Sci. 9:1778. doi: 10.3389/fpls.2018.01778
Meco, V., Egea, I., Ortíz-Atienza, A., Drevensek, S., Esch, E., Yuste-Lisbona, F. J., et al. (2020). The salt sensitivity induced by disruption of cell wall-associated kinase 1 (SlWAK1) tomato gene is linked to altered osmotic and metabolic homeostasis. Int. J. Mol. Sci. 21:6308. doi: 10.3390/ijms21176308
Mellidou, I., Ainalidou, A., Papadopoulou, A., Leontidou, K., Genitsaris, S., Karagiannis, E., et al. (2021). Comparative transcriptomics and metabolomics reveal an intricate priming mechanism involved in PGPR-mediated salt tolerance in tomato. Front. Plant Sci. 12:713984. doi: 10.3389/fpls.2021.713984
Meng, X., Cai, J., Deng, L., Li, G., Sun, J., Han, Y., et al. (2020). SlSTE1 promotes abscisic acid-dependent salt stress-responsive pathways via improving ion homeostasis and reactive oxygen species scavenging in tomato. J. Integr. Plant Biol. 62, 1942–1966. doi: 10.1111/jipb.12987
Meza, S. L., Egea, I., Massaretto, I. L., Morales, B., Purgatto, E., Egea-Fernández, J. M., et al. (2020). Traditional tomato varieties improve fruit quality without affecting fruit yield under moderate salt stress. Front. Plant Sci. 11:1717. doi: 10.3389/fpls.2020.587754
Miller, G., Suzuki, N., Ciftci-Yilmaz, S., and Mittler, R. (2010). Reactive oxygen species homeostasis and signalling during drought and salinity stresses. Plant Cell Environ. 33, 453–467. doi: 10.1111/j.1365-3040.2009.02041.x
Mishra, N. S., Tuteja, R., and Tuteja, N. (2006). Signaling through MAP kinase networks in plants. Arch. Biochem. Biophys. 452, 55–68. doi: 10.1016/j.abb.2006.05.001
Mittler, R., Zandalinas, S. I., Fichman, Y., and Van Breusegem, F. (2022). Reactive oxygen species signalling in plant stress responses. Nat. Rev. Mol. Cell Biol. 1–17. doi: 10.1038/s41580-022-00499-2 [Epub ahead of print].
Mittova, V., Guy, M., Tal, M., and Volokita, M. (2004). Salinity up-regulates the antioxidative system in root mitochondria and peroxisomes of the wild salt-tolerant tomato species Lycopersicon pennellii. J. Exp. Bot. 55, 1105–1113. doi: 10.1093/jxb/erh113
Mokrani, S., Nabti, E. H., and Cruz, C. (2020). Current advances in plant growth promoting bacteria alleviating salt stress for sustainable agriculture. Appl. Sci. 10:7025. doi: 10.3390/app10207025
Moretti, M. B., Maskin, L., Gudesblat, G., García, S. C., and Iusem, N. D. (2006). ASR1, a stress-induced tomato protein, protects yeast from osmotic stress. Physiol. Plant. 127, 111–118. doi: 10.1111/j.1399-3054.2006.00664.x
Morton, M. J., Awlia, M., Al-Tamimi, N., Saade, S., Pailles, Y., Negrão, S., et al. (2019). Salt stress under the scalpel–dissecting the genetics of salt tolerance. Plant J. 97, 148–163. doi: 10.1111/tpj.14189
Munns, R., and Gilliham, M. (2015). Salinity tolerance of crops–what is the cost? New Phytol. 208, 668–673. doi: 10.1111/nph.13519
Munns, R., Husain, S., Rivelli, A. R., James, R. A., Condon, A. G., Lindsay, M. P., et al. (2002). Avenues for increasing salt tolerance of crops, and the role of physiologically based selection traits. Plant Soil 247, 93–105. doi: 10.1007/978-94-017-2789-1_7
Munns, R., James, R. A., Gilliham, M., Flowers, T. J., and Colmer, T. D. (2016). Tissue tolerance: An essential but elusive trait for salt-tolerant crops. Funct. Plant Biol. 43, 1103–1113. doi: 10.1071/FP16187
Muñoz-Mayor, A., Pineda, B., Garcia-Abellán, J. O., Antón, T., Garcia-Sogo, B., Sanchez-Bel, P., et al. (2012). Overexpression of dehydrin tas14 gene improves the osmotic stress imposed by drought and salinity in tomato. J. Plant Physiol. 169, 459–468. doi: 10.1016/j.jplph.2011.11.018
Mutale-joan, C., Rachidi, F., Mohamed, H. A., Mernissi, N. E., Aasfar, A., Barakate, M., et al. (2021). Microalgae-cyanobacteria–based biostimulant effect on salinity tolerance mechanisms, nutrient uptake, and tomato plant growth under salt stress. J. Appl. Phycol. 33, 3779–3795. doi: 10.1007/s10811-021-02559-0
Nieves-Cordones, M., Miller, A. J., Alemán, F., Martínez, V., and Rubio, F. (2008). A putative role for the plasma membrane potential in the control of the expression of the gene encoding the tomato high-affinity potassium transporter HAK5. Plant Mol. Biol. 68, 521–532. doi: 10.1007/s11103-008-9388-3
Olías, R., Eljakaoui, Z., Li, J., De Morales, P. A., Marin-Manzano, M. C., Pardo, J. M., et al. (2009a). The plasma membrane Na+/H+ antiporter SOS1 is essential for salt tolerance in tomato and affects the partitioning of Na+ between plant organs. Plant Cell Environ. 32, 904–916. doi: 10.1111/j.1365-3040.2009.01971.x
Olías, R., Eljakaoui, Z., Pardo, J. M., and Belver, A. (2009b). The Na+/H+ exchanger SOS1 controls extrusion and distribution of Na+ in tomato plants under salinity conditions. Plant Signal. Behav. 4, 973–976. doi: 10.4161/psb.4.10.9679
Ouziad, F., Wilde, P., Schmelzer, E., Hildebrandt, U., and Bothe, H. (2006). Analysis of expression of aquaporins and Na+/H+ transporters in tomato colonized by arbuscular mycorrhizal fungi and affected by salt stress. Environ. Exp. Bot. 57, 177–186. doi: 10.1016/j.envexpbot.2005.05.011
Pailles, Y., Awlia, M., Julkowska, M., Passone, L., Zemmouri, K., Negrão, S., et al. (2020). Diverse traits contribute to salinity tolerance of wild tomato seedlings from the Galapagos Islands. Plant Physiol. 182, 534–546. doi: 10.1104/pp.19.00700
Pan, Y., Hu, X., Li, C., Xu, X., Su, C., Li, J., et al. (2017). SlbZIP38, a tomato bZIP family gene downregulated by abscisic acid, is a negative regulator of drought and salt stress tolerance. Genes 8:402. doi: 10.3390/genes8120402
Parthasarathi, T., Ephrath, J. E., and Lazarovitch, N. (2021). Grafting of tomato (Solanum lycopersicum L.) onto potato (Solanum tuberosum L.) to improve salinity tolerance. Sci. Hortic. 282:110050. doi: 10.1016/j.scienta.2021.110050
Parvin, K., Hasanuzzaman, M., Bhuyan, M., Nahar, K., Mohsin, S. M., and Fujita, M. (2019b). Comparative physiological and biochemical changes in tomato (Solanum lycopersicum L.) under salt stress and recovery: Role of antioxidant defense and glyoxalase systems. Antioxidants 8:350. doi: 10.3390/antiox8090350
Parvin, K., Hasanuzzaman, M., Bhuyan, M., Mohsin, S. M., and Fujita, M. (2019a). Quercetin mediated salt tolerance in tomato through the enhancement of plant antioxidant defense and glyoxalase systems. Plants 8:247. doi: 10.3390/plants8080247
Parvin, K., Nahar, K., Hasanuzzaman, M., Bhuyan, M. B., Mohsin, S. M., and Fujita, M. (2020). Exogenous vanillic acid enhances salt tolerance of tomato: Insight into plant antioxidant defense and glyoxalase systems. Plant Physiol. Biochem. 150, 109–120. doi: 10.1016/j.plaphy.2020.02.030
Pérez-Labrada, F., López-Vargas, E. R., Ortega-Ortiz, H., Cadenas-Pliego, G., Benavides-Mendoza, A., and Juárez-Maldonado, A. (2019). Responses of tomato plants under saline stress to foliar application of copper nanoparticles. Plants 8:151. doi: 10.3390/plants8060151
Pinedo-Guerrero, Z. H., Cadenas-Pliego, G., Ortega-Ortiz, H., González-Morales, S., Benavides-Mendoza, A., Valdés-Reyna, J., et al. (2020). Form of silica improves yield, fruit quality and antioxidant defense system of tomato plants under salt stress. Agriculture 10:367. doi: 10.3390/agriculture10090367
Poór, P., Gémes, K., Horváth, F., Szepesi, A., Simon, M. L., and Tari, I. (2011). Salicylic acid treatment via the rooting medium interferes with stomatal response, CO2 fixation rate and carbohydrate metabolism in tomato, and decreases harmful effects of subsequent salt stress. Plant Biol. 13, 105–114. doi: 10.1111/j.1438-8677.2010.00344.x
Poór, P., Kovács, J., Szopkó, D., and Tari, I. (2013). Ethylene signaling in salt stress-and salicylic acid-induced programmed cell death in tomato suspension cells. Protoplasma 250, 273–284. doi: 10.1007/s00709-012-0408-4
Poór, P., Patyi, G., Takács, Z., Szekeres, A., Bódi, N., Bagyánszki, M., et al. (2019). Salicylic acid-induced ROS production by mitochondrial electron transport chain depends on the activity of mitochondrial hexokinases in tomato (Solanum lycopersicum L.). J. Plant Res. 132, 273–283. doi: 10.1007/s10265-019-01085-y
Qi, J., Wang, J., Gong, Z., and Zhou, J. M. (2017). Apoplastic ROS signaling in plant immunity. Curr. Opin. Plant Biol. 38, 92–100. doi: 10.1016/j.pbi.2017.04.022
Qi, S., Lin, Q., Zhu, H., Gao, F., Zhang, W., and Hua, X. (2016). The RING finger E3 ligase SpRing is a positive regulator of salt stress signaling in salt-tolerant wild tomato species. Plant Cell Physiol. 57, 528–539. doi: 10.1093/pcp/pcw006
Qin, H., Li, Y., and Huang, R. (2020). Advances and challenges in the breeding of salt-tolerant rice. Int. J. Mol. Sci. 21:8385. doi: 10.3390/ijms21218385
Rahman, A., Nahar, K., Hasanuzzaman, M., and Fujita, M. (2016). Calcium supplementation improves Na+/K+ ratio, antioxidant defense and glyoxalase systems in salt-stressed rice seedlings. Front. Plant Sci. 7:609. doi: 10.3389/fpls.2016.00609
Raziq, A., Wang, Y., Mohi Ud Din, A., Sun, J., Shu, S., and Guo, S. (2022). A comprehensive evaluation of salt tolerance in tomato (Var. Ailsa Craig): Responses of physiological and transcriptional changes in RBOH’s and ABA biosynthesis and signalling genes. Int. J. Mol. Sci. 23:1603. doi: 10.3390/ijms23031603
Ricardi, M. M., González, R. M., Zhong, S., Domínguez, P. G., Duffy, T., Turjanski, P. G., et al. (2014). Genome-wide data (ChIP-seq) enabled identification of cell wall-related and aquaporin genes as targets of tomato ASR1, a drought stress-responsive transcription factor. BMC Plant Biol. 14:29. doi: 10.1186/1471-2229-14-29
Rivero, R. M., Mestre, T. C., Mittler, R., Rubio, F., Garcia-Sanchez, F., and Martinez, V. (2014). The combined effect of salinity and heat reveals a specific physiological, biochemical and molecular response in tomato plants. Plant Cell Environ. 37, 1059–1073. doi: 10.1111/pce.12199
Rodríguez-Rosales, M. P., Jiang, X., Gálvez, F. J., Aranda, M. N., Cubero, B., and Venema, K. (2008). Overexpression of the tomato K+/H+ antiporter LeNHX2 confers salt tolerance by improving potassium compartmentalization. New Phytol. 179, 366–377. doi: 10.1111/j.1469-8137.2008.02461.x
Romero-Aranda, M. R., Espinosa, J., González-Fernández, P., Jaime-Fernández, E., Traverso, J. Á., Asins, M. J., et al. (2021). Role of Na+ transporters HKT1;1 and HKT1;2 in tomato salt tolerance. I. Function loss of cheesmaniae alleles in roots and aerial parts. Plant Physiol. Biochem. 168, 282–293. doi: 10.1016/j.plaphy.2021.10.018
Romero-Aranda, M. R., González-Fernández, P., Pérez-Tienda, J. R., López-Diaz, M. R., Espinosa, J., Granum, E., et al. (2020). Na+ transporter HKT1;2 reduces flower Na+ content and considerably mitigates the decline in tomato fruit yields under saline conditions. Plant Physiol. Biochem. 154, 341–352. doi: 10.1016/j.plaphy.2020.05.012
Rothan, C., Diouf, I., and Causse, M. (2019). Trait discovery and editing in tomato. Plant J. 97, 73–90. doi: 10.1111/tpj.14152
Rouphael, Y., Raimondi, G., Lucini, L., Carillo, P., Kyriacou, M. C., Colla, G., et al. (2018). Physiological and metabolic responses triggered by omeprazole improve tomato plant tolerance to NaCl stress. Front. Plant Sci. 9:249. doi: 10.3389/fpls.2018.00249
Sánchez-Aguayo, I., Rodríguez-Galán, J. M., García, R., Torreblanca, J., and Pardo, J. M. (2004). Salt stress enhances xylem development and expression of S-adenosyl-L-methionine synthase in lignifying tissues of tomato plants. Planta 220, 278–285. doi: 10.1007/s00425-004-1350-2
Sanwal, S. K., Mann, A., Kumar, A., Kesh, H., Kaur, G., Rai, A. K., et al. (2022). Salt tolerant eggplant rootstocks modulate sodium partitioning in tomato scion and improve performance under saline conditions. Agriculture 12:183. doi: 10.3390/agriculture12020183
Sayed, E. G., Mahmoud, A. W. M., El-Mogy, M. M., Ali, M. A., Fahmy, M. A., and Tawfic, G. A. (2022). The effective role of nano-silicon application in improving the productivity and quality of grafted tomato grown under salinity stress. Horticulturae 8:293. doi: 10.3390/horticulturae8040293
Shu, P., Li, Y., Li, Z., Sheng, J., and Shen, L. (2022). SlMAPK3 enhances tolerance to salt stress in tomato plants by scavenging ROS accumulation and up-regulating the expression of ethylene signaling related genes. Environ. Exp. Bot. 193:104698. doi: 10.1016/j.envexpbot.2021.104698
Siddiqui, M. H., Alamri, S. A., Al-Khaishany, M. Y., Al-Qutami, M. A., Ali, H. M., Hala, A. R., et al. (2017). Exogenous application of nitric oxide and spermidine reduces the negative effects of salt stress on tomato. Hortic. Environ. Biotechnol. 58, 537–547. doi: 10.1007/s13580-017-0353-4
Singh, H., Kumar, P., Kumar, A., Kyriacou, M. C., Colla, G., and Rouphael, Y. (2020). Grafting tomato as a tool to improve salt tolerance. Agronomy 10:263. doi: 10.3390/agronomy10020263
Singh, J., Sastry, E. D., and Singh, V. (2012). Effect of salinity on tomato (Lycopersicon esculentum Mill.) during seed germination stage. Physiol. Mol. Biol. Plants 18, 45–50. doi: 10.1007/s12298-011-0097-z
Singh, V. K., Singh, A. K., Singh, P. P., and Kumar, A. (2018). Interaction of plant growth promoting bacteria with tomato under abiotic stress: A review. Agric. Ecosyst. Environ. 267, 129–140. doi: 10.1016/j.agee.2018.08.020
Skłodowska, M., Gapiñska, M., Gajewska, E., and Gabara, B. (2009). Tocopherol content and enzymatic antioxidant activities in chloroplasts from NaCl-stressed tomato plants. Acta Physiol. Plant. 31, 393–400. doi: 10.1007/s11738-008-0248-1
Sousa, B., Rodrigues, F., Soares, C., Martins, M., Azenha, M., Lino-Neto, T., et al. (2022). Impact of combined heat and salt stresses on tomato plants-insights into nutrient uptake and redox homeostasis. Antioxidants 11:478. doi: 10.3390/antiox11030478
Sun, S., Wen, D., Yang, W., Meng, Q., Shi, Q., and Gong, B. (2020). Overexpression of caffeic acid O-methyltransferase 1 (COMT1) increases melatonin level and salt stress tolerance in tomato plant. J. Plant Growth Regul. 39, 1221–1235. doi: 10.1007/s00344-019-10058-3
Sun, W., Xu, X., Zhu, H., Liu, A., Liu, L., Li, J., et al. (2010). Comparative transcriptomic profiling of a salt-tolerant wild tomato species and a salt-sensitive tomato cultivar. Plant Cell Physiol. 51, 997–1006. doi: 10.1093/pcp/pcq056
Sun, X. C., Gao, Y. F., Li, H. R., Yang, S. Z., and Liu, Y. S. (2015). Over-expression of SlWRKY39 leads to enhanced resistance to multiple stress factors in tomato. J. Plant Biol. 58, 52–60. doi: 10.1007/s12374-014-0407-4
Takács, Z., Poór, P., Szepesi, Á., and Tari, I. (2017). In vivo inhibition of polyamine oxidase by a spermine analogue, MDL-72527, in tomato exposed to sublethal and lethal salt stress. Funct. Plant Biol. 44, 480–492. doi: 10.1071/FP16280
Tanveer, K., Gilani, S., Hussain, Z., Ishaq, R., Adeel, M., and Ilyas, N. (2020). Effect of salt stress on tomato plant and the role of calcium. J. Plant Nutr. 43, 28–35. doi: 10.1080/01904167.2019.1659324
Tran, M. T., Doan, D. T. H., Kim, J., Song, Y. J., Sung, Y. W., Das, S., et al. (2021). CRISPR/Cas9-based precise excision of SlHyPRP1 domain(s) to obtain salt stress-tolerant tomato. Plant Cell Rep. 40, 999–1011. doi: 10.1007/s00299-020-02622-z
Van Oosten, M. J., Di Stasio, E., Cirillo, V., Silletti, S., Ventorino, V., Pepe, O., et al. (2018). Root inoculation with Azotobacter chroococcum 76A enhances tomato plants adaptation to salt stress under low N conditions. BMC Plant Biol. 18:205. doi: 10.1186/s12870-018-1411-5
van Zelm, E., Zhang, Y., and Testerink, C. (2020). Salt tolerance mechanisms of plants. Annu. Rev. Plant Biol. 71, 403–433. doi: 10.1146/annurev-arplant-050718-100005
Villalta, I., Reina-Sánchez, A., Bolarín, M. C., Cuartero, J., Belver, A., Venema, K., et al. (2008). Genetic analysis of Na+ and K+ concentrations in leaf and stem as physiological components of salt tolerance in tomato. Theor. Appl. Genet. 116, 869–880. doi: 10.1007/s00122-008-0720-8
Viveros, M. F. Á., Inostroza-Blancheteau, C., Timmermann, T., González, M., and Arce-Johnson, P. (2013). Overexpression of GlyI and GlyII genes in transgenic tomato (Solanum lycopersicum Mill.) plants confers salt tolerance by decreasing oxidative stress. Mol. Biol. Rep. 40, 3281–3290. doi: 10.1007/s11033-012-2403-4
Wang, G., Zhang, S., Ma, X., Wang, Y., Kong, F., and Meng, Q. (2016). A stress-associated NAC transcription factor (SlNAC35) from tomato plays a positive role in biotic and abiotic stresses. Physiol. Plant. 158, 45–64. doi: 10.1111/ppl.12444
Wang, X., Geng, S., Ri, Y. J., Cao, D., Liu, J., Shi, D., et al. (2011). Physiological responses and adaptive strategies of tomato plants to salt and alkali stresses. Sci. Hortic. 130, 248–255. doi: 10.1016/j.scienta.2011.07.006
Wang, Y., Bian, Z., Pan, T., Cao, K., and Zou, Z. (2021). Improvement of tomato salt tolerance by the regulation of photosynthetic performance and antioxidant enzyme capacity under a low red to far-red light ratio. Plant Physiol. Biochem. 167, 806–815. doi: 10.1016/j.plaphy.2021.09.008
Wang, Z., Hong, Y., Li, Y., Shi, H., Yao, J., Liu, X., et al. (2021). Natural variations in SlSOS1 contribute to the loss of salt tolerance during tomato domestication. Plant Biotechnol. J. 19, 20–22. doi: 10.1111/pbi.13443
Wang, Z., Hong, Y., Zhu, G., Li, Y., Niu, Q., Yao, J., et al. (2020). Loss of salt tolerance during tomato domestication conferred by variation in a Na+/K+ transporter. EMBO J. 39, e103256. doi: 10.15252/embj.2019103256
Waseem, M., Rong, X., and Li, Z. (2019). Dissecting the role of a basic helix-loop-helix transcription factor, SlbHLH22, under salt and drought stresses in transgenic Solanum lycopersicum L. Front. Plant Sci. 10:734. doi: 10.3389/fpls.2019.00734
Waszczak, C., Carmody, M., and Kangasjärvi, J. (2018). Reactive oxygen species in plant signaling. Annu. Rev. Plant Biol. 69, 209–236. doi: 10.1146/annurev-arplant-042817-040322
Wei, D., Zhang, W., Wang, C., Meng, Q., Li, G., Chen, T. H., et al. (2017). Genetic engineering of the biosynthesis of glycinebetaine leads to alleviate salt-induced potassium efflux and enhances salt tolerance in tomato plants. Plant Sci. 257, 74–83. doi: 10.1016/j.plantsci.2017.01.012
Win, K. T., Tanaka, F., Okazaki, K., and Ohwaki, Y. (2018). The ACC deaminase expressing endophyte Pseudomonas spp. Enhances NaCl stress tolerance by reducing stress-related ethylene production, resulting in improved growth, photosynthetic performance, and ionic balance in tomato plants. Plant Physiol. Biochem. 127, 599–607. doi: 10.1016/j.plaphy.2018.04.038
Wu, H., Zhang, X., Giraldo, J. P., and Shabala, S. (2018). It is not all about sodium: Revealing tissue specificity and signalling roles of potassium in plant responses to salt stress. Plant Soil 431, 1–17. doi: 10.1007/s11104-018-3770-y
Wu, J., Wang, J., Pan, C., Guan, X., Wang, Y., Liu, S., et al. (2014). Genome-wide identification of MAPKK and MAPKKK gene families in tomato and transcriptional profiling analysis during development and stress response. PLoS One 9:e103032. doi: 10.1371/journal.pone.0103032
Wu, X., Jia, Q., Ji, S., Gong, B., Li, J., Lü, G., et al. (2020). Gamma-aminobutyric acid (GABA) alleviates salt damage in tomato by modulating Na+ uptake, the GAD gene, amino acid synthesis and reactive oxygen species metabolism. BMC Plant Biol. 20:465. doi: 10.1186/s12870-020-02669-w
Xian, Z., Yang, Y., Huang, W., Tang, N., Wang, X., and Li, Z. (2013). Molecular cloning and characterisation of SlAGO family in tomato. BMC Plant Biol. 13:126. doi: 10.1186/1471-2229-13-126
Xiang, Y., and Jiménez-Gómez, J. M. (2020). SlHAK20: A new player in plant salt tolerance. EMBO J. 39:e104997. doi: 10.15252/embj.2020104997
Xu, J., Hua, K., and Lang, Z. (2019). Genome editing for horticultural crop improvement. Hortic. Res. 6:113. doi: 10.1038/s41438-019-0196-5
Xu, J., Kang, Z., Zhu, K., Zhao, D., Yuan, Y., Yang, S., et al. (2021). RBOH1-dependent H2O2 mediates spermine-induced antioxidant enzyme system to enhance tomato seedling tolerance to salinity-alkalinity stress. Plant Physiol. Biochem. 164, 237–246. doi: 10.1016/j.plaphy.2021.04.017
Xu, W., Jia, L., Shi, W., Baluıka, F., Kronzucker, H. J., Liang, J., et al. (2013). The tomato 14-3-3 protein TFT4 modulates H+ efflux, basipetal auxin transport, and the PKS5-J3 pathway in the root growth response to alkaline stress. Plant Physiol. 163, 1817–1828. doi: 10.1104/pp.113.224758
Xu, W. F., and Shi, W. M. (2006). Expression profiling of the 14-3-3 gene family in response to salt stress and potassium and iron deficiencies in young tomato (Solanum lycopersicum) roots: Analysis by real-time RT–PCR. Ann. Bot. 98, 965–974. doi: 10.1093/aob/mcl189
Xu, W. F., and Shi, W. M. (2007). Mechanisms of salt tolerance in transgenic Arabidopsis thaliana constitutively overexpressing the tomato 14-3-3 protein TFT7. Plant Soil 301, 17–28. doi: 10.1007/s11104-007-9403-5
Xu, Z., Wang, J., Zhen, W., Sun, T., and Hu, X. (2022). Abscisic acid alleviates harmful effect of saline–alkaline stress on tomato seedlings. Plant Physiol. Biochem. 175, 58–67. doi: 10.1016/j.plaphy.2022.01.018
Yamaguchi, T., and Blumwald, E. (2005). Developing salt-tolerant crop plants: Challenges and opportunities. Trends Plant Sci. 10, 615–620. doi: 10.1016/j.tplants.2005.10.002
Yang, J., Wang, P., Li, S., Liu, T., and Hu, X. (2022). Polyamine oxidase triggers H2O2-mediated spermidine improved oxidative stress tolerance of tomato seedlings subjected to saline-alkaline stress. Int. J. Mol. Sci. 23:1625.
Yang, X. J., and Seto, E. (2007). HATs and HDACs: From structure, function and regulation to novel strategies for therapy and prevention. Oncogene 26, 5310–5318. doi: 10.1038/sj.onc.1210599
Yang, Y., and Guo, Y. (2018a). Elucidating the molecular mechanisms mediating plant salt-stress responses. New Phytol. 217, 523–539. doi: 10.1111/nph.14920
Yang, Y., and Guo, Y. (2018b). Unraveling salt stress signaling in plants. J. Integr. Plant Biol. 60, 796–804. doi: 10.1111/jipb.12689
Yang, Y., Tang, N., Xian, Z., and Li, Z. (2015). Two SnRK2 protein kinases genes play a negative regulatory role in the osmotic stress response in tomato. Plant Cell Tiss. Organ Cult. 122, 421–434. doi: 10.1007/s11240-015-0779-2
Yang, Y., Yao, Y., Li, J., Zhang, J., Zhang, X., Hu, L., et al. (2022). Trehalose alleviated salt stress in tomato by regulating ROS metabolism, photosynthesis, osmolyte synthesis, and trehalose metabolic pathways. Front. Plant Sci. 13:772948. doi: 10.3389/fpls.2022.772948
Yang, Z., Wang, C., Xue, Y., Liu, X., Chen, S., Song, C., et al. (2019). Calcium-activated 14-3-3 proteins as a molecular switch in salt stress tolerance. Nat. Commun. 10, 1–12. doi: 10.1038/s41467-019-09181-2
Yi, C., Yao, K., Cai, S., Li, H., Zhou, J., Xia, X., et al. (2015). High atmospheric carbon dioxide-dependent alleviation of salt stress is linked to RESPIRATORY BURST OXIDASE 1 (RBOH1)-dependent H2O2 production in tomato (Solanum lycopersicum). J. Exp. Bot. 66, 7391–7404. doi: 10.1093/jxb/erv435
Yin, W., Hu, Z., Hu, J., Zhu, Z., Yu, X., Cui, B., et al. (2017). Tomato (Solanum lycopersicum) MADS-box transcription factor SlMBP8 regulates drought, salt tolerance and stress-related genes. Plant Growth Regul. 83, 55–68. doi: 10.1007/s10725-017-0283-2
Yin, Z., Lu, J., Meng, S., Liu, Y., Mostafa, I., Qi, M., et al. (2019). Exogenous melatonin improves salt tolerance in tomato by regulating photosynthetic electron flux and the ascorbate–glutathione cycle. J. Plant Interact. 14, 453–463. doi: 10.1080/17429145.2019.1645895
Yu, W., Wang, L., Zhao, R., Sheng, J., Zhang, S., Li, R., et al. (2019). Knockout of SlMAPK3 enhances tolerance to heat stress involving ROS homeostasis in tomato plants. BMC Plant Biol. 19:354. doi: 10.1186/s12870-019-1939-z
Yu, X., Gao, Q., Chen, G., Guo, J. E., Guo, X., Tang, B., et al. (2018). SlHDA5, a tomato histone deacetylase gene, is involved in responding to salt, drought, and ABA. Plant Mol. Biol. Rep. 36, 36–44. doi: 10.1007/s11105-017-1057-8
Yuan, F., Yang, H., Xue, Y., Kong, D., Ye, R., Li, C., et al. (2014). OSCA1 mediates osmotic-stress-evoked Ca2+ increases vital for osmosensing in Arabidopsis. Nature 514, 367–371. doi: 10.1038/nature13593
Zhang, Y., Kaiser, E., Zhang, Y., Yang, Q., and Li, T. (2018). Short-term salt stress strongly affects dynamic photosynthesis, but not steady-state photosynthesis, in tomato (Solanum lycopersicum). Environ. Exp. Bot. 149, 109–119. doi: 10.1016/j.envexpbot.2018.02.014
Zhang, X., Chen, L., Shi, Q., and Ren, Z. (2020b). SlMYB102, an R2R3-type MYB gene, confers salt tolerance in transgenic tomato. Plant Sci. 291:110356. doi: 10.1016/j.plantsci.2019.110356
Zhang, Y., Yao, Q., Shi, Y., Li, X., Hou, L., Xing, G., et al. (2020c). Elevated CO2 improves antioxidant capacity, ion homeostasis, and polyamine metabolism in tomato seedlings under Ca(NO3)2-induced salt stress. Sci. Hortic. 273:109644. doi: 10.1016/j.scienta.2020.109644
Zhang, X., Bao, Z., Gong, B., and Shi, Q. (2020a). S-adenosylmethionine synthetase 1 confers drought and salt tolerance in transgenic tomato. Environ. Exp. Bot. 179:104226. doi: 10.1016/j.envexpbot.2020.104226
Zhao, C., Zhang, H., Song, C., Zhu, J. K., and Shabala, S. (2020). Mechanisms of plant responses and adaptation to soil salinity. Innovation 1:100017. doi: 10.1016/j.xinn.2020.100017
Zhao, L., Lu, J., Zhang, J., Wu, P. Y., Yang, S., and Wu, K. (2015). Identification and characterization of histone deacetylases in tomato (Solanum lycopersicum). Front. Plant Sci. 5:760. doi: 10.3389/fpls.2014.00760
Zhao, S., Zhang, Q., Liu, M., Zhou, H., Ma, C., and Wang, P. (2021). Regulation of plant responses to salt stress. Int. J. Mol. Sci. 22:4609. doi: 10.3390/ijms22094609
Zheng, M., Liu, X., Lin, J., Liu, X., Wang, Z., Xin, M., et al. (2019). Histone acetyltransferase GCN5 contributes to cell wall integrity and salt stress tolerance by altering the expression of cellulose synthesis genes. Plant J. 97, 587–602. doi: 10.1111/tpj.14144
Zheng, Q., Liu, J., Liu, R., Wu, H., Jiang, C., Wang, C., et al. (2016). Temporal and spatial distributions of sodium and polyamines regulated by brassinosteroids in enhancing tomato salt resistance. Plant Soil 400, 147–164. doi: 10.1007/s11104-015-2712-1
Zhong, M., Song, R., Wang, Y., Shu, S., Sun, J., and Guo, S. (2020). TGase regulates salt stress tolerance through enhancing bound polyamines-mediated antioxidant enzymes activity in tomato. Environ. Exp. Bot. 179:104191. doi: 10.1016/j.envexpbot.2020.104191
Zhou, Y., Diao, M., Chen, X., Cui, J., Pang, S., Li, Y., et al. (2019). Application of exogenous glutathione confers salinity stress tolerance in tomato seedlings by modulating ions homeostasis and polyamine metabolism. Sci. Hortic. 250, 45–58. doi: 10.1016/j.scienta.2019.02.026
Zhou, Y., Ding, M., Cui, J. X., Chen, X. J., Wen, Z. L., Zhang, J. W., et al. (2018). Exogenous GSH protects tomatoes against salt stress by modulating photosystem II efficiency, absorbed light allocation and H2O2-scavenging system in chloroplasts. J. Integr. Agric. 17, 2257–2272. doi: 10.1016/S2095-3119(18)62068-4
Zhou, Y., Wen, Z., Zhang, J., Chen, X., Cui, J., Xu, W., et al. (2017). Exogenous glutathione alleviates salt-induced oxidative stress in tomato seedlings by regulating glutathione metabolism, redox status, and the antioxidant system. Sci. Hortic. 220, 90–101. doi: 10.1016/j.scienta.2017.02.021
Zhu, J. K. (2016). Abiotic stress signaling and responses in plants. Cell 167, 313–324. doi: 10.1016/j.cell.2016.08.029
Zhu, M., Meng, X., Cai, J., Li, G., Dong, T., and Li, Z. (2018). Basic leucine zipper transcription factor SlbZIP1 mediates salt and drought stress tolerance in tomato. BMC Plant Biol. 18:83. doi: 10.1186/s12870-018-1299-0
Zhu, T., Deng, X., Zhou, X., Zhu, L., Zou, L., Li, P., et al. (2016). Ethylene and hydrogen peroxide are involved in brassinosteroid-induced salt tolerance in tomato. Sci. Rep. 6:35392. doi: 10.1038/srep35392
Zilberman, D., Cao, X., and Jacobsen, S. E. (2003). ARGONAUTE4 control of locus-specific siRNA accumulation and DNA and histone methylation. Science 299, 716–719. doi: 10.1126/science.1079695
Zuluaga, M. Y. A., Milani, K. M. L., Miras-Moreno, M. B., Lucini, L., Valentinuzzi, F., Mimmo, T., et al. (2021). The adaptive metabolomic profile and functional activity of tomato rhizosphere are revealed upon PGPB inoculation under saline stress. Environ. Exp. Bot. 189:104552. doi: 10.1016/j.envexpbot.2021.104552
Keywords: tomato, salinity tolerance, mechanism, abiotic stress, genetic breeding
Citation: Guo M, Wang X-S, Guo H-D, Bai S-Y, Khan A, Wang X-M, Gao Y-M and Li J-S (2022) Tomato salt tolerance mechanisms and their potential applications for fighting salinity: A review. Front. Plant Sci. 13:949541. doi: 10.3389/fpls.2022.949541
Received: 21 May 2022; Accepted: 17 August 2022;
Published: 14 September 2022.
Edited by:
Andrea Miyasaka Almeida, Universidad Mayor, ChileReviewed by:
Mirza Hasanuzzaman, Sher-e-Bangla Agricultural University, BangladeshJosé M. Alvarez, Andrés Bello University, Chile
Copyright © 2022 Guo, Wang, Guo, Bai, Khan, Wang, Gao and Li. This is an open-access article distributed under the terms of the Creative Commons Attribution License (CC BY). The use, distribution or reproduction in other forums is permitted, provided the original author(s) and the copyright owner(s) are credited and that the original publication in this journal is cited, in accordance with accepted academic practice. No use, distribution or reproduction is permitted which does not comply with these terms.
*Correspondence: Meng Guo, mguo@nxu.edu.cn; Jian-She Li, 13709587801@163.com