- 1Ryan Institute, School of Natural Sciences, University of Galway, Galway, Ireland
- 2Environmental Biotechnology Lab, Department of Biosciences & Bioengineering, Indian Institute of Technology, Jodhpur, India
Biofuels hold particular promise as these can replace fossil fuels. Algae, in particular, are envisioned as a sustainable source of third-generation biofuels. Algae also produce several low volume high-value products, which enhance their prospects of use in a biorefinery. Bio-electrochemical systems such as microbial fuel cell (MFC) can be used for algae cultivation and bioelectricity production. MFCs find applications in wastewater treatment, CO2 sequestration, heavy metal removal and bio-remediation. Oxidation of electron donor by microbial catalysts in the anodic chamber gives electrons (reducing the anode), CO2, and electrical energy. The electron acceptor at the cathode can be oxygen/NO3-/NO2-/metal ions. However, the need for a continuous supply of terminal electron acceptor in the cathode can be eliminated by growing algae in the cathodic chamber, as they produce enough oxygen through photosynthesis. On the other hand, conventional algae cultivation systems require periodic oxygen quenching, which involves further energy consumption and adds cost to the process. Therefore, the integration of algae cultivation and MFC technology can eliminate the need of oxygen quenching and external aeration in the MFC system and thus make the overall process sustainable and a net energy producer. In addition to this, the CO2 gas produced in the anodic chamber can promote the algal growth in the cathodic chamber. Hence, the energy and cost invested for CO2 transportation in an open pond system can be saved. In this context, the present review outlines the bottlenecks of first- and second-generation biofuels along with the conventional algae cultivation systems such as open ponds and photobioreactors. Furthermore, it discusses about the process sustainability and efficiency of integrating algae cultivation with MFC technology in detail.
1 Introduction
Biofuels hold tremendous promise in providing energy security for the future. These are renewable, environment friendly, usable in existing engines, blendable with diesel, and available in liquid, gas, and solid form. Biofuels have been explored extensively during the last few decades (Chowdhury and Loganathan, 2019). Based on the original raw material for biofuel production, biofuels are categorized as first, second, and third generation. The first generation (1G) biofuels involve the use of food-based biomass feedstock like sugarcane, potato, corn, beet, sunflower, rapeseed and so forth. The use of 1G biofuels trigger the food versus fuel debate and is often limited by the availability of agricultural land. The direct use of food crops is highly unsustainable, particularly in highly populated developing countries. The 2G biofuels are derived from inedible portions of the plant and non-food items such as ligno-cellulosic wastes, waste cooking oil and carbon rich industry waste. (Chowdhury and Loganathan, 2019). The production of 2G biofuels is limited by the need to pretreat biomass, remove inhibitors, develop an enzymatic cocktail for hydrolysis, and develop an efficient fermenting strain.
The bottlenecks associated with the 1G and 2G biofuels switched researcher’s focus towards the evolution of 3G biofuels (Brennan and Owende, 2010). The third-generation biofuels are obtained from microalgae biomass. This generation of biofuels circumvents some of the problems associated with 1G and 2G biofuels and is relatively sustainable (Nigam and Singh, 2011). Algae is a source of several other high-value low-volume products that enable their use in a biorefinery (Chisti, 2007). The 3G biofuels hold several advantages over 1G and 2G biofuels, such as shorter harvesting cycle, higher growth rate, and higher oil production rate (Schenk et al., 2008). Algae cultivation does not depend on agricultural land eliminating the food versus fuel issue (Scott et al., 2010). Estimates show that a bio-oil productivity of 10000 L/hectare/year of bio-oil can be obtained from microalgae (Alam et al., 2015).
Algae are classified into two major categories based on their external morphology, i.e. microalgae and macroalgae. Brown and red algae along with green seaweed are prominent examples of macroalgae, whereas microalgae include Chlorella, Spirulina and other green algae (Demirbas, 2010). The microalgae are superior to macroalgae in terms of oil content, microscopic cell size, and higher growth rate. Algae biomass can be converted to bioethanol, biodiesel, biomethane, biohydrogen, biochar and some value added pigments or other value added products (Kumar et al., 2020).
1.1 Biodiesel
Biodiesel is mainly derived from the intracellular lipids of oleaginous microalgae. Algal lipids consist of triglycerides (TAG) along with mono and diglycerides and free fatty acids. Stearic acid, palmitic acid, and oleic acid are the predominant fatty acids types in the algal lipids (Tripathi et al., 2015). Algae biomass displays a variable amount of lipid content depending on the strain type and cultivation condition. For example, the lipid content of Chlorella vulgaris varies from 11% to 43% (Mitra et al., 2012). Enamala et al. (2018) reviewed several studies and found that the lipid content varies from 2.4% to 62% of dry algal cell weight (Enamala et al., 2018).
Algal lipids are converted to biodiesel through a catalytic transesterification reaction between triacylglycerols and methanol. The transesterification process results in fatty acid methyl ester (FAME) and glycerol (Kumar et al., 2020). The purified FAMEs are known as biodiesel. The lipid composition, such as the percentage of saturated fatty acids, affects the fuel properties. The algal oil contains more unsaturated fatty acids than saturated ones, which improves cold flow and make it a suitable feedstock (Demirbaş, 2009; Tripathi et al., 2015). However, it also triggers the production of hydroperoxide and insoluble substances which collectively lead to choking of the filter (Kumar and Thakur, 2018).
1.2 Biomethane
Algae biomass or leftover algal biomass after lipid extraction (Lipid extracted algae) produces biogas when subjected to anaerobic digestion. This biogas is composed of CH4 (50-70%) and CO2 (30-50%) (Kumar et al., 2020). The algal biomass can generate 0.024 –0.6 L CH4/g VS (volatile solid) or 0.2 –0.4 m3 CH4/kg biomass. The biogas yields vary from one species to another and depend on process conditions (Milledge et al., 2019; Rabii et al., 2019). The factors affecting biogas production include algae cell wall composition, process temperature, C/N ratio, biomass loading rate and reactor configuration (Sialve et al., 2009; McKennedy and Sherlock, 2015; Barbot et al., 2016). The biogas production process when integrated with other bioenergy processes adds value and makes it sustainable (Cesaro and Belgiorno, 2015).
1.3 Biochar
Biochar is produced through hydrothermal carbonization (HTC) of dry biomass (Gollakota et al., 2018). Algae based biochar has a high cation exchange capacity, lesser carbon proportion, and lesser surface area than lignocellulosic biomass based biochar (Michalak et al., 2019). Algae based biochar has higher yield compared to other feedstocks and the yield ranges from 8.1% to 64.2% of dry biomass (Yu et al., 2017; Michalak et al., 2019). The high ash content blocks micropores resulting in a low active surface area (Leng et al., 2021). Algae biochar also possesses several functional groups making it suitable for the remediation of inorganic and organic contaminants from wastewater (Kumar et al., 2020).
1.4 Bioethanol
Algal biomass can ferment to bioethanol under anaerobic conditions. The process is mediated by yeast, bacteria and/or fungi (Minh and Hanh, 2012; Robak and Balcerek, 2018). The algae biomass contains several polymers like mannitol and agar (Kostas et al., 2016; Offei et al., 2018). Brown algae are rich in carbohydrates such as alginate, mannitol, laminarin, glucose, fucoidan, and cellulose (Ale and Meyer, 2013). Similarly, red algae have a diverse range of hydrolysable polymers, which can be converted to ethanol (Behera et al., 2015).
1.5 Other value-added products
Intact algae biomass or algae products find applications in industries such as food, pharmaceutical, healthcare and cosmetics. Algal species such as Spirulina and Chlorella serve as a food supplement and source of protein (Kumar et al., 2020). Algae produce pigments like carotenoids, phycocyanin and chlorophyll (Barkia et al., 2019). Carotenoids such as zeaxanthin, α-carotene, β-carotene, and lutein are antioxidants and have anticancer properties (Dickinson et al., 2017; Matos, 2017). The polyunsaturated fatty acids (PUFA) derived from algae serve as food supplements (Lee, 2013). In addition to this, some unconventional value-added products such as ubiquinone coenzyme Q10, ubiquinol, cannabinoids, anandamids, hoshinolactum, dolastatins, endotoxins and several therapeutic substances can be obtained from algae (Abu-ghosh et al., 2021; Hans et al., 2021; Mondal et al., 2020).
2 Oleaginous algae
Algae are classified in nine groups, namely cyanobacteria (Cyanophyceae), diatoms (Bacillariophyceae), brown algae (Phaeophyceae), yellow-green algae (Xanthophyceae), red algae (Rhodophyceae), green algae (Chlorophyceae), golden algae (Chrysophyceae), “picoplankton” (Prasinophyceae and Eustigmatophyceae) and dinoflagellates (Dinophyceae) (Neto et al., 2019). Microalgae such as Chlorella, Spirulina, Haematococcus and Dunaliella are grown commercially with a production level of several 100 tons annually (Neto et al., 2019). These algae are a rich source of protein, carbohydrate, and lipid (Table 1). However, the chemical constitution of a microalgae cell can differ according to the species, strain and cultivation conditions (Lim et al., 2021). For example, it is reported that microalgae species such as Trachydiscus and Nanochloropsis are unable to produce carbohydrates (Hildebrand et al., 2013). Similarly, Dunaliella tertiolecta ATCC 30929 can produce lipids up to 74% (w/w) (Takagi et al., 2006), while Chlorella vulgaris CCAP 211/11B majorly produces carbohydrates (55% w/w) (Illman et al., 2000). The selection of a suitable strain for maximizing the biofuel production is crucial for the downstream processes (Debnath et al., 2021; Lim et al., 2021).
The presence of saturated and unsaturated fatty acids and their amounts also affect their suitability for employing them as engine oil. In a study, 7 freshwater microalgae species were compared by the presence of fatty acids. It was discovered that the C16:2, C16:3 and C20:5, C16:4 and C18:4, and C18:4 and C22:6 are only produced in Nannochloropsis sp., Ankistrodesmus sp., and Isochrysis sp., respectively (Thomas et al., 1984). Studies have confirmed that microalgal lipids are high in energy rich fatty acids and suitable for biofuel production (Steen et al., 2010). Furthermore, researchers around the globe have succeeded in developing strategies to improve lipid productivity from microalgae spp. In this context, growing microalgae in stress conditions such as nitrogen limitation has been shown very effective for some species (Levasseur et al., 2020). Recently, the development of genetic engineering tools and omics technologies have significantly improved lipid productivities in many strains (Muñoz et al., 2021).
3 Modes of algae cultivation
3.1 Open cultivation systems
3.1.1 Open unagitated ponds
Unagitated and shallow open ponds require little effort for algae cultivation on a large scale. Natural water bodies having 50 cm depth are ideal for this kind of cultivation. The disadvantages associated with such systems include frequent contamination, slower diffusion of nutrients, and the formation of algal bloom (Chew et al., 2018).
3.1.2 Circular ponds
Circular ponds are similar to unagitated open ponds except that they are equipped with a stirring unit. The mixing in circular ponds is enabled by a rotating shaft which moves in axial direction in order to create a homogenous mixing of nutrients (Ting et al., 2017). The circular ponds are also prone to contamination.
3.1.3 Raceway ponds
Raceway ponds are extensively used for the commercial production of algae biomass. Raceway ponds have a race track type design and can have a single channel or multiple channels (Ting et al., 2017). Paddle wheels in these systems ensure mixing and homogenous suspension of algae cells.
3.2 Closed cultivation systems
3.2.1 Horizontal tube photo-bioreactor
The horizontal tube photo-bioreactor (PBR) has long horizontal tubes arranged as panels, walls or helices (Chew et al., 2018). Mixing is achieved through a centrifugal pump (Klinthong et al., 2015). The reactors can run using either natural or artificial light. The limitation is the requirement of large surface areas.
3.2.2 Vertical tube PBRs
Vertical tube PBRs, such as airlift and bubble column PBRs has an air sparger at the bottom of the reactor enabling mixing, nutrient, and gas exchange. The liquid flow in a bubble column reactor is triggered by the air bubbles produced at the bottom of the vessel. The high surface area to volume ratio of bubbles allows efficient gas exchange. An airlift reactor contains two interconnected regions, namely, dark and illuminated zones. Air bubbles lift the liquid from dark to light zones, leading to homogenous mixing of nutrients and fluids between the two zones. Vertical tube type PBRs offer homogenous mixing, low shear stress on cells, high photosynthetic efficiency, and high algal productivities (Chew et al., 2018).
3.2.3 Flat panel PBR
Flat panel PBRs consist of two transparent plates arranged as rectangular box. The light source orientation ensures equal light intensity at all positions of the reactor. The air sparger and pump enables mixing and gas exchange (Klinthong et al., 2015). These systems have a high surface area to volume ratio, suitable design for scaling up, and low level of oxygen retention inside the reactor (Ting et al., 2017).
3.2.4 Continuous stirred tank PBR
Continuous stirred tank reactor (CSTR) is similar to conventional CSTR bioreactors except for the presence of an external light source. These systems offer lower productivities due to inefficient light penetration and low surface area to volume ratio (Chew et al., 2018).
4 Integrating algae cultivation with bio-electrochemical systems
The main focus of all the commercial industries dealing with third generation biofuels is to optimize and develop efficient and cost-effective approaches for maximizing the algal biomass production. However, the existing algae cultivation strategies have several drawbacks which need to be addressed in order to commercialize the third-generation biofuels (Table 2). The major drawbacks associated with open ponds are that they are prone to contamination and evaporation losses. On the other hand, closed algae cultivation systems are highly expensive and often require oxygen quenching (Table 2).
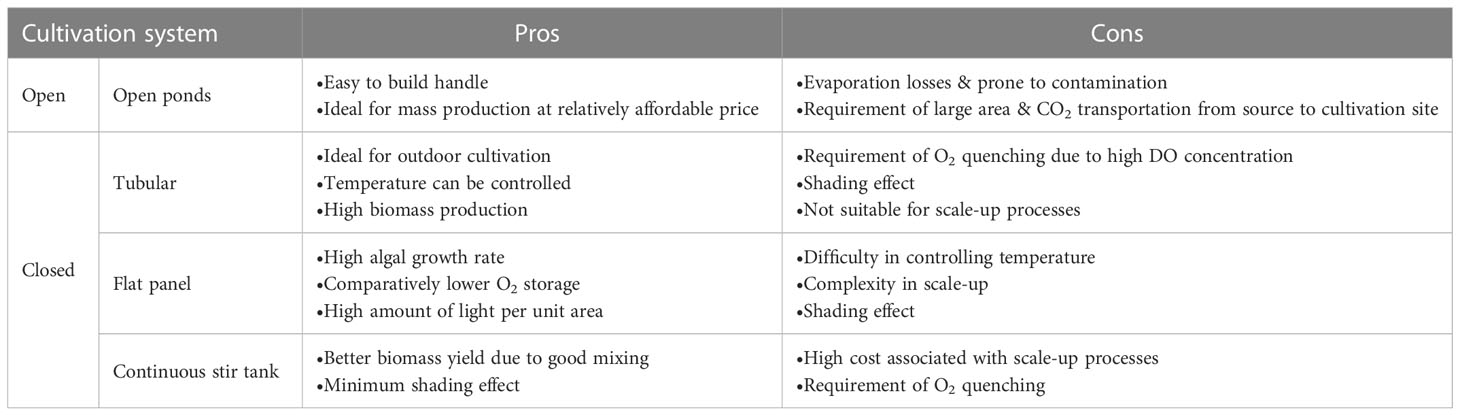
Table 2 Pros and cons associated with conventional algae cultivation systems (Ting et al., 2017; Chew et al., 2018).
Bio-electrochemical systems such as microbial fuel cell (MFC) can be used for algae cultivation and bioelectricity production as they offer advantages over conventional algae cultivation systems. MFCs find applications in wastewater treatment, CO2 sequestration, heavy metal removal, and bio-remediation. (Zhang et al., 2011). A typical MFC consists of an anode and cathode placed in two chambers separated by an ion-exchange membrane. Oxidation of electron donor by microbial catalysts in the anodic chamber gives electrons (reducing anode), CO2, and electrical energy (Khandelwal et al., 2022; Neethu et al., 2022). The electrons flow through the external circuit to be captured by the terminal electron acceptor present at the cathode (Trapero et al., 2017). The anode and cathode chamber have differences in redox potential, which is often maintained with the help of the ion-exchange membrane. The detailed description of MFCs can be found in the sections below. The electron acceptor at the cathode can be oxygen/NO3-/NO2-/metal ions. However, the need for a continuous supply of terminal electron acceptor in the cathode can be eliminated by growing algae in the cathodic chamber, as it produces enough oxygen through photosynthesis (Khandelwal et al., 2018). On the other hand, the conventional algae cultivation systems require periodic oxygen quenching, which involves further energy consumption and adds cost to the process. Therefore, the integration of algae cultivation and MFC technology can eliminate the need of oxygen quenching and external aeration in the MFC system and make the overall process sustainable and net energy producer. In addition to this, the CO2 gas produced in the anodic chamber can promote the algal growth in the cathodic chamber. Hence, the energy and cost invested for CO2 transportation in an open pond system can be saved (Figure 1).
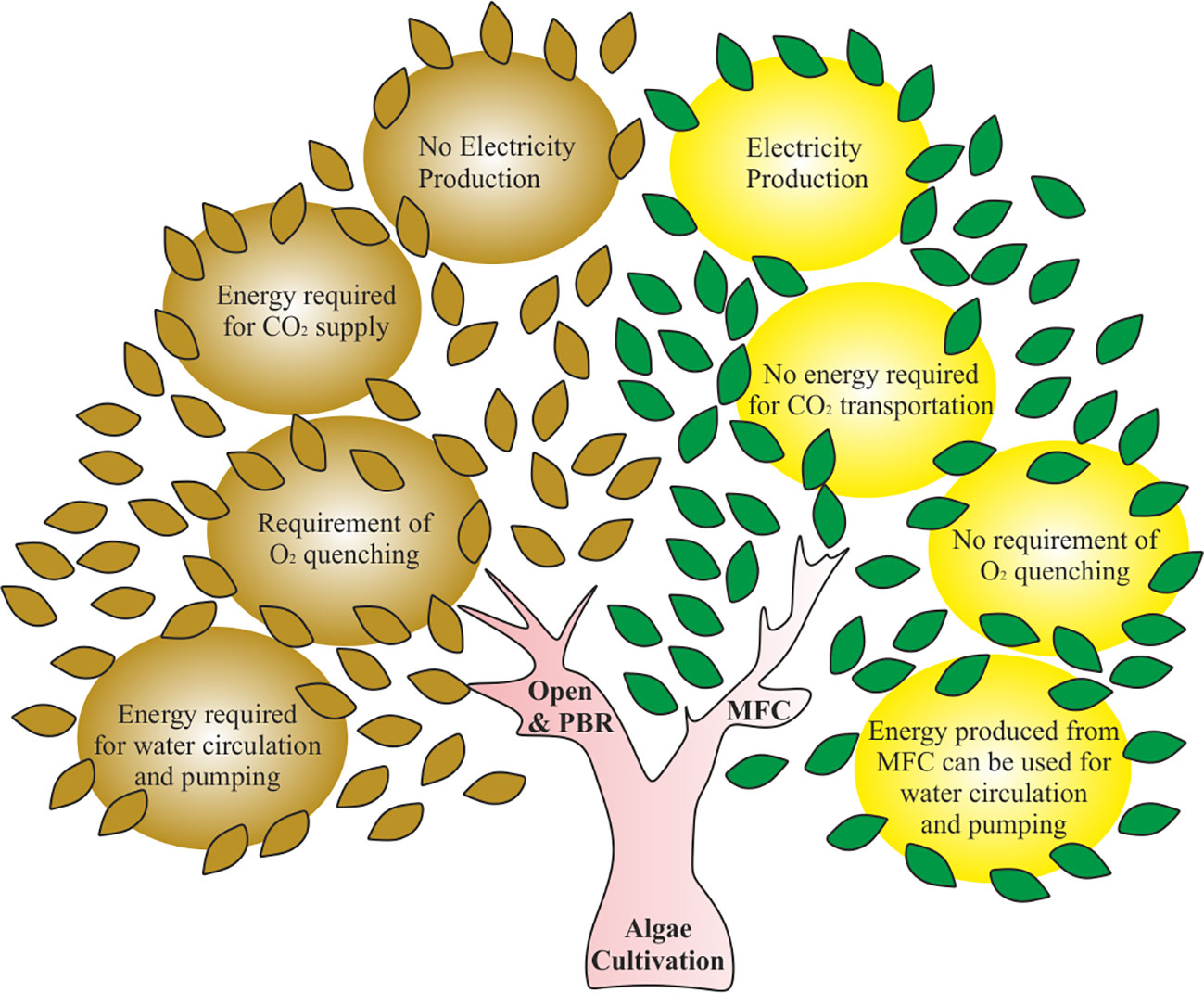
Figure 1 Illustration showing the comparison between conventional algae cultivation systems and microbial fuel cell based algae cultivation. PBR, Photobioreactor and MFC, Microbial fuel cell.
4.1 MFC principles and components
The electrigens reduce the anode by oxidizing the organic matter present in the anodic chamber. The process of anode reduction is thermodynamically favorable and hence spontaneous. The anodic redox potential is dependent upon the chemical nature of organic matter and can be calculated using the well-known Nernst equation. On the other hand, electrons in the cathodic chamber are commonly accepted by oxygen due to their availability and high redox potential (+0.82 V). Still, a number of other chemical acceptors are also used that include nitrate, manganese oxide, iron, hydrogen peroxide and nitrite (Chaudhuri and Lovley, 2003). The schematic representation of MFC is shown in Figure 2. The basic functional mechanisms of MFC would be clearer by an example of reactions on the electrodes surface, as shown below:
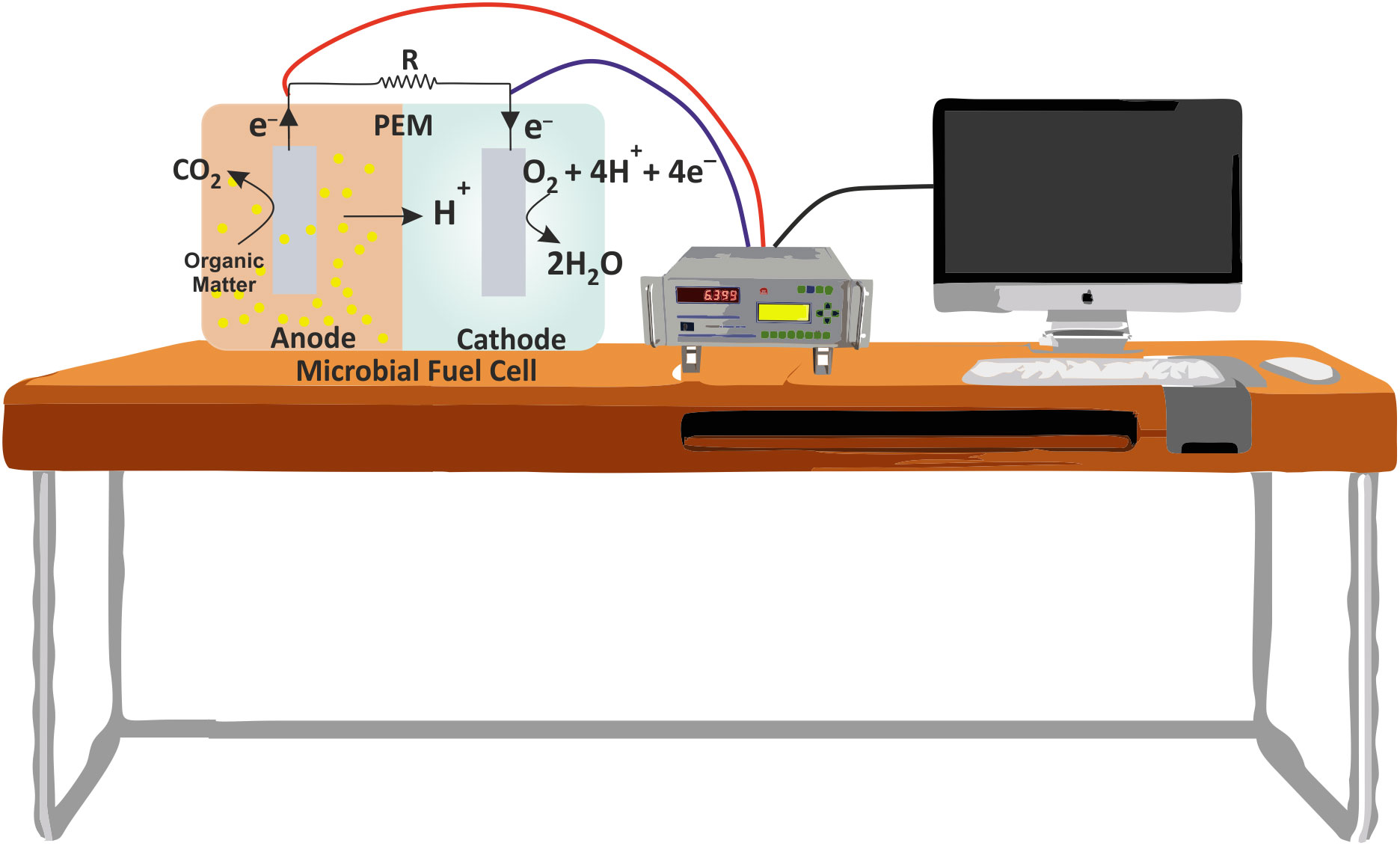
Figure 2 Schematic showing a typical MFC and its working principle. PEM, Proton exchange membraner and R, Resistance.
Anode:
Cathode:
The overall cell voltage can be described as:
The ideal cell voltage that a system can generate is represented by equation 2.3, but due to the association of several losses in real MFC, the operating voltage is lowered. Primarily, there are 3 integral components of a typical MFC, namely anode, cathode and proton/cation exchange membrane.
4.1.1 Anode
The anode should have the following properties: (i) corrosion resistance, (ii) high electrical conductivity, (iii) biocompatibility, (iv) high surface area, (v) chemical stability and mechanical strength (Rinaldi et al., 2008; Guo et al., 2015). Carbon based conductive electrodes are frequently used in an anodic chamber. Classical examples include carbon paper, carbon brushes, carbon felt, reticulated vitreous carbon, graphite fiber brush, granular graphite, graphite plates, and rods (Zhou et al., 2011; Hindatu et al., 2017).
4.1.2 Cathode
The MFC cathode can be biotic or abiotic. Carbon based electrodes are the most preferred choice as a cathode as well. The abiotic electrodes generally have chemical/metal catalysts for acceptor reduction. Biotic electrodes, on the other hand, have algae/bacteria which aid both in acceptor reduction and production. Platinum based electrodes find applications in chemical fuel cells for high efficiency oxygen reduction at the cathode (Liu et al., 2004). Pt based electrodes are not suitable for biotic cathodes because of several reasons. Pt is poisoned by phosphates, nitrates, and chlorides often used in the microbial growth medium. Pt is costly and also toxic to microorganisms (Khandelwal et al., 2021). Non-platinum based catalysts like carbon nanotube, conductive polyaniline, metal oxide (lead oxide- PbO2, manganese (IV) dioxide), metals (cobalt and iron) serve well in biotic cathodes (He et al., 2017).
4.1.3 Membrane/Separator
In order to maintain chemical equilibrium in the cell, usually, a membrane or separator is placed between the anode and cathode which ensures the protons and/or cations transport from the anodic to the cathodic chamber. Table 3 summarizes the membranes or separators employed in MFCs. The most commonly used membrane in conventional MFCs is Nafion 117. It is resistant to biofouling, has high ionic conductivity, and is impermeable to oxygen and organic acids (Logan and Regan, 2006). Its employability is limited by its high cost. In addition to this, researchers have used glass fibers, J-cloth, earthenware, nylon fibers, ceramics, and biodegradable shopping bags as alternative membrane separators (Santoro et al., 2017).
4.2 Photosynthetic or algae assisted MFCs
As mentioned earlier, algae assisted MFCs hold significant promise in making MFC technology sustainable. Algae-assisted MFCs can be powered by low-cost algae biomass; can produce algae biomass which serves the dual purpose of carbon capture, and oxygen generation. Oxygen is the most preferred electron acceptor in MFCs as it supports high potential differences. Algae cultivation at the cathode provides the system with a continuous supply of oxygen (during the light period) and helps circumvent the installation of mechanical aerators. Algae biomass also serves as feedstock for biodiesel generation and several other products. Wang et al. reported a power density of 5.6 W/m3 in a Chlorella based MFC (Wang et al., 2010). A culture of cyanobacteria, Anabaena, at the cathode sparged with a CO2-air mixture gave a power density of 57.8 mW/m2 (Pandit et al., 2012). In a study, a power density of 2.48 W/m3 and a Coulombic efficiency (CE) of 9.4% were attained using immobilized algae systems (Zhou et al., 2012). Photosynthetic microbial fuel cell (PMFC), algae assisted microbial fuel cell (AMFC) or microbial carbon capture cell (MCCs) also serve as a modified photo bioreactor equipped with an inherent oxygen quenching mechanism and carbon dioxide supply. The process of algae cultivation at the cathode also complements the effective carbon removal at the anode. Microalgae biomass is rich in hydrolysable carbohydrates, fats, and proteins and can serve as an electron donor substrate at the anode (Cui et al., 2014).
An algae assisted MFC can take different configurations depending on the intended application, i.e., algal production, power generation and wastewater treatment. Various kinds of algae-based MFC configurations are shown in Figure 3. The configuration varies from triple chamber to single chamber. A single chamber algae assisted MFC involves bacterial and algae cultivation in the same chamber. The CO2 produced by bacteria is effectively sequestered by the microalgae present in the same chamber. The carbon capture is efficient and the system is easily maintainable. In a two chambered system, algae and bacterial consortia are separated by a proton exchange membrane (PEM). These systems are used for algae cultivation for bioenergy or other applications. A separate photo-bioreactor is sometimes coupled with the system to enhance the algae growth rate and power generation. A three chamber algae-based MFC finds application in water desalination, where saltwater is fed to the middle chamber to facilitate flow of positive and negative ions. Researchers have also used uplift aeration type MFC to support high algae growth rates (Saba et al., 2017).
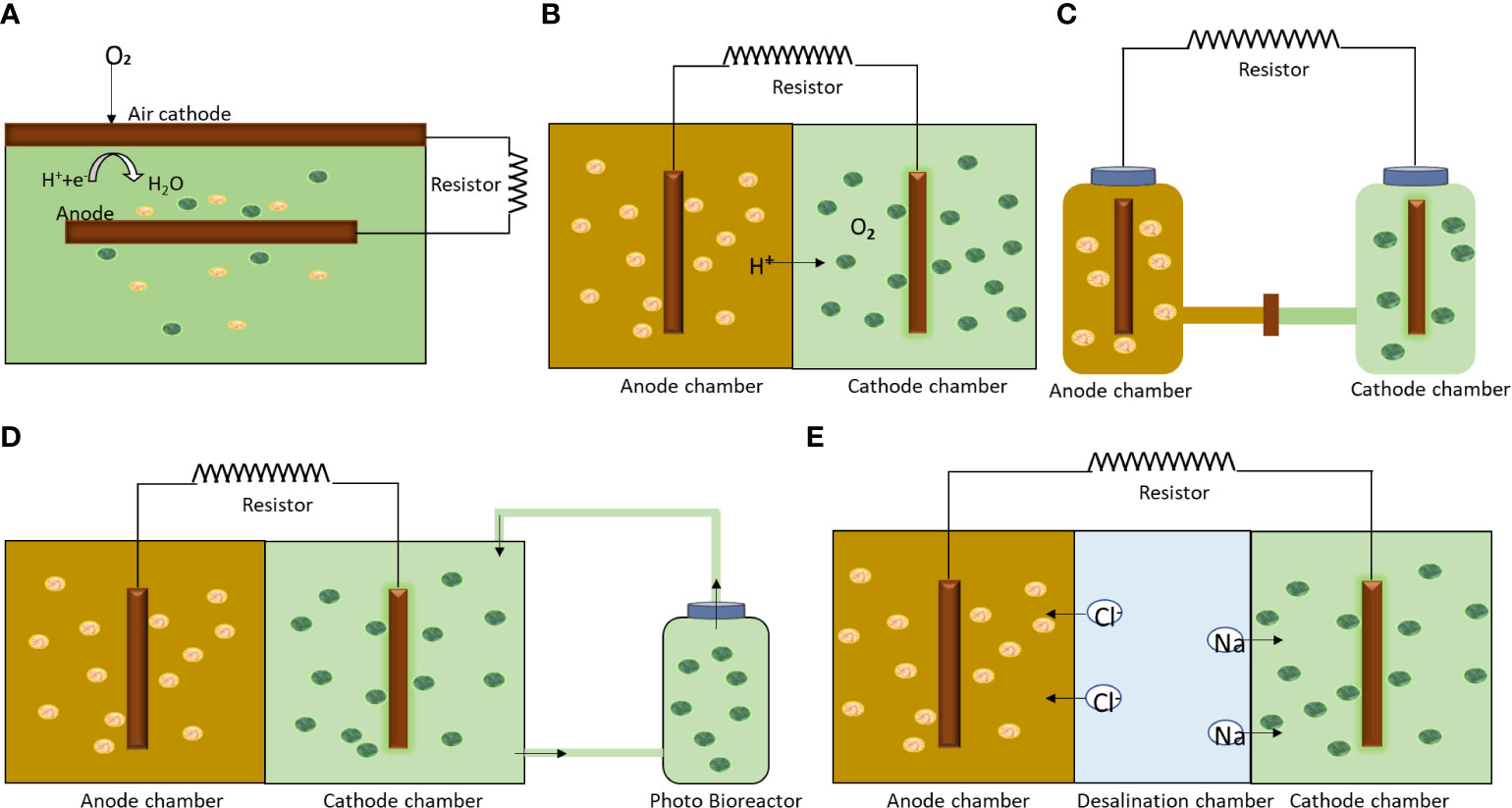
Figure 3 Schematic showing different algae assisted MFC configurations (A) single chamber; (B) Dual chamber; (C) H-shaped; (D) dual chamber integrated with external photobioreactor and (E) three chamber with desalination.
5 Use of algae in MFCs
5.1 Algae biomass as anodic substrate
Algae biomass is rich in decomposable carbohydrates, lipids and proteins. Therefore, algae serve as a good source of electron donor at the anode. The primary challenge with the use of intact algae biomass is its complex cell wall. Algae cell wall composition varies from class to class and species to species. The chlorophycophyta contain a wide array of cell walls ranging from cellulose pectin complexes to hydroxyproline rich glycoproteins. Like plants cells, algae cell walls are intricate mix of polymers such as cellulose, hemicellulose, lignin, pectin and arabinogalactan proteins. This complex assembly of polymers in the algae cell wall necessitates the biomass pretreatment to break open the structure, enhance the surface area, and hydrolyze polymers. Researchers have used both intact and pretreated micro and macroalgae as anodic substrates and reported good power outputs (Velasquez-orta et al., 2009; Cui et al., 2014). The use of pre-digested algae biomass also supports high power output over undigested biomass (Salar-garcía et al., 2016).
5.2 Algae biomass at the cathodic chamber
Algae at the cathode not only serve as oxygen supplier but also as catalysts for oxygen reduction at the electrode surface. Algae produced metabolites also serve as electron acceptors in the absence of oxygen particularly during the dark period. The success of algae assisted MFC depends on the process of photosynthesis which is driven by light energy and carbon dioxide supply (González Del Campo et al., 2013; Elmekawy et al., 2014).
Additionally, algae can effectively remove nitrates and phosphates from the water. Algae can grow in autotrophic, heterotrophic, and mixotrophic mode. Heterotrophic and mixotrophic modes assist with carbon removal. The simultaneous carbon, nitrogen, and phosphorus removal is possible using dual chamber algae assisted MFCs. The anode and cathode both can contribute towards carbon removal while the algae assisted cathode can help with nitrogen and phosphorus removal (Commault et al., 2017). The success of algae assisted MFC in wastewater treatment depends on the algal strain, inoculum size, density, temperature, N/P ratio, salinity, pH, light intensity and CO2 supply and capture rate. An algae assisted MFC thus needs optimization with respect to all these parameters (Nagendranatha Reddy et al. 2019).
6 Output from algae assisted MFC
Algae assisted MFCs can generate both bioelectricity and algal biomass. Table 4 summarizes the prominent studies in terms of power output obtained from different algal strains employed in algae assisted MFCs. Power output from algae assisted MFC can be optimized by choosing an appropriate algae species, electrode material, catalyst coating, chamber design, light duration and intensity, electron donor substrate, and CO2 source. The microalgae can directly generate current either by introducing it in the anodic chamber as an electron donor substrate or in the cathodic chamber as a biocatalyst for generation of oxygen (Elmekawy et al., 2014).
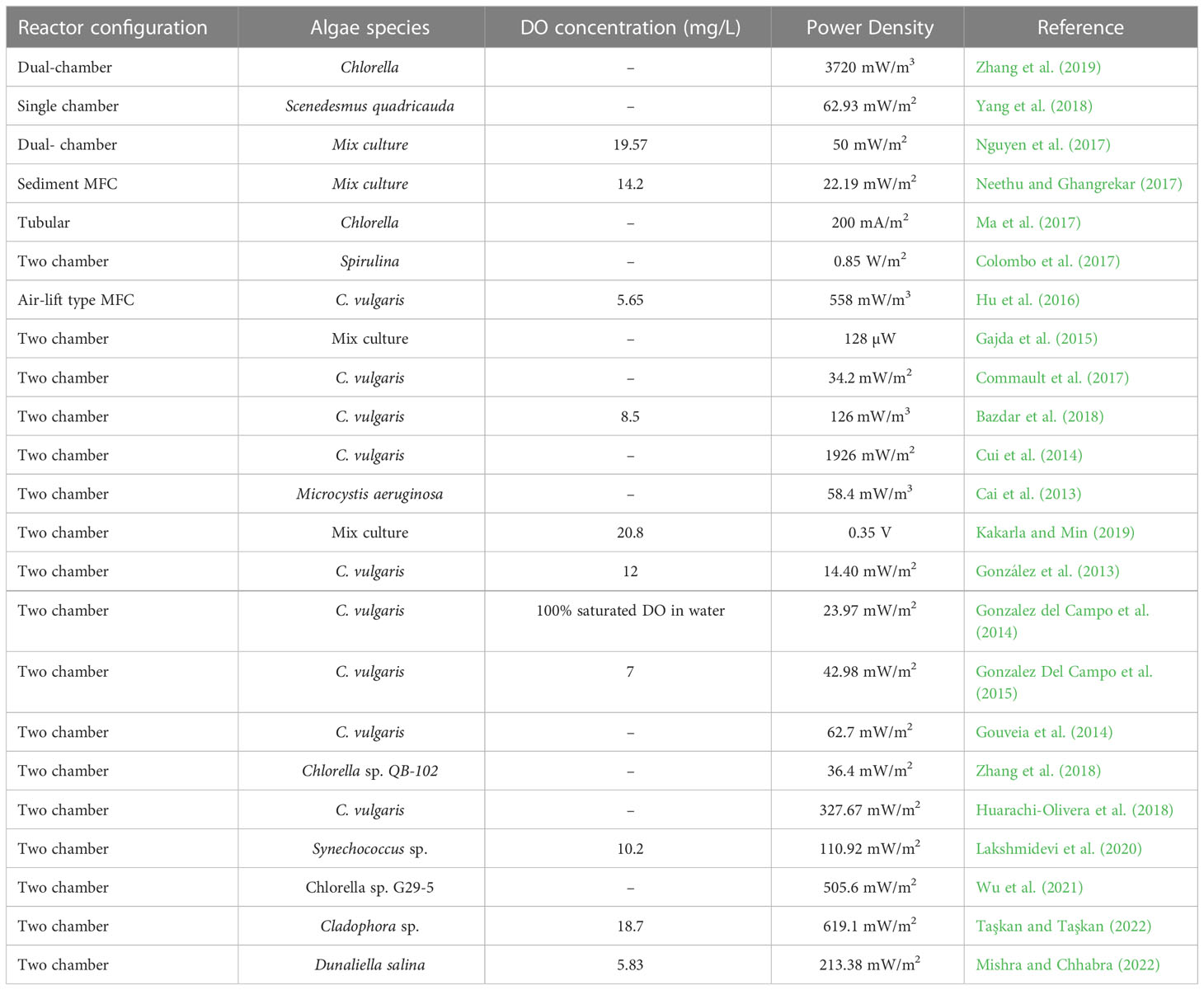
Table 4 Different species of microalgae and the corresponding dissolved oxygen (DO) and power output obtained in algae assisted MFCs.
The algal biomass production in algae assisted MFC is important to assess the overall system performance and net energy recovery. The Table 5 summarizes the key studies reporting the chemical oxygen demand (COD) removal by particular algal strains and their net biomass production. The main factors which affect the algal growth in MFC include reactor configuration, wastewater composition and light intensity (Luo et al., 2017). Despite some obvious advantages, the algae growth rates and productivities achieved in MFCs are low. This is primarily due to the lack of studies specifically investigating the algae growth rate in MFCs and on system scale up.
7 Factors affecting power output in algae assisted MFC
7.1 Light
Light is the primary requirement for photosynthesis. Light intensity, its duration (light/dark period), and wavelength all affect algae growth. High light intensities lead to photo oxidation and growth inhibition. On the other hand, low light intensities lower algae growth rates and promote bacterial growth. Both polychromatic and monochromatic light is used for cultivating algae. Amongst the monochromatic light, the red and blue light is most preferred for high rate algal cultures. Light source and its orientation with respect to MFC affect algae growth. Researchers prefer using inbuilt LED lights that ensure direct illumination and minimize the self-shading effect. It also helps to regulate temperature and ensure low temperatures enabling optimized algae growth. The ratio of light/dark period is also critical and varies from species to species and system to system (Saba et al., 2017; Nagendranatha Reddy et al. 2019).
7.2 CO2 concentration
Carbon dioxide is another key ingredient required for algae growth. Most of the algae grow well at atmospheric CO2 levels. However, higher concentrations are shown to promote algae growth and carbon capture (Singh and Singh, 2014). Researchers have studied the impact CO2 concentration on growth and lipid production in algae (Sato et al., 2003; Wang et al., 2010). The response varies from species to species and also on the cultivation conditions. In an algae assisted MFC, the CO2 required by the microalgae can either be the CO2 present in the anodic off-gas (Wang et al., 2010) or it can be CO2 sparged separately (González Del Campo et al., 2013). However, CO2 sparging is associated with certain disadvantages, including the lowering of the pH on the dissolution of CO2 in water, which can be resolved by the use of a higher initial inoculum concentration (Chiu et al., 2009; Zhang et al., 2014). Increasing the CO2 concentration by 10-15% has resulted in a 6% increase in the lipid content, confirming the significance of the CO2 concentration in the lipid content of algal cells (Liu et al., 2011).
7.3 Dissolved oxygen
Photosynthesis liberates oxygen via light reaction and algae consume oxygen while respiring. A high concentration of oxygen becomes inhibitory for algae growth and leads to photo-oxidative damage. It was found that a DO concentration exceeding 30 mg/l inhibited the C. vulgaris growth by 30% (Kazbar et al., 2019). MFC circumvents this problem as oxygen is quenched through the reduction reaction in a circuit MFC. The solubility of oxygen in water is also dependent on temperature, salt content, and duration of light/dark cycles. It is often observed that during night time, the DO level drops and so is the power output from a MFC (Gonzalez Del Campo et al., 2015). A DO level of 4.5-5.5 mg/l is suitable for supporting continuous power output from a MFC (Rodrigo et al., 2010), while an algae based cathode can realize DO levels in the order of 6.6 mg/l (Kang et al., 2003). Another major factor that determines the DO concentration in water is temperature. Hence, the use of proper lighting equipment with useful wavelengths is of utmost importance. In other words, DO is dependent on the temperature as well as the duration of the light/dark cycles. One important investigation to show this relationship was the one carried out by Gouveia et al. (2014) wherein experiments were conducted using two light intensities 26 µE/m2 and 96 µE/m2. An increase in algal growth rate with increased oxygen concentration resulting in enhanced power generation at higher light intensity was observed in this investigation (Gouveia et al., 2014).
8 Scaled-up studies on algae-assisted MFCs
The employment of microalgae in the cathodic chamber of MFC is comparatively a new technology, therefore, there are thus far not so many large-scale studies involving AMFCs. However, there are numerous reports for MFC applications on larger scale for wastewater treatment and bioelectricity generation. Scaled-up implementation of MFCs can be done either by increasing the size of a single reactor or stacking several miniature reactors. However, miniaturization does not increase internal resistance much, hence this strategy can provide uncompromised power output. In a recent study, a low-cost liter scale AMFC was constructed using inexpensive materials such as rock phosphate (RP) blended clayware as anodic and low-density polythene bag as cathodic chamber. This study was carried out under natural sunlight in outdoor conditions without controlling temperature and pH. The slow release of P from RP resulted in enhanced algal growth of 4.6 g/l along with a power density of 1.2 W/m3. This inexpensive AMFC assembly costs only 11.25 USD, implying the possibility of large-scale application (Khandelwal et al., 2020). In an another study, multiple anodes were connected in series with capacitors and this stacked assembly was operated in an algal raceway pond (16 L). The highest voltage and power output obtained from this study was 1.4 V and 2.34 W/m3, respectively (Yang et al., 2019).
Recently, sediment microbial fuel cells (SMFCs) have also emerged as a novel technology to treat sedimental wastes. Sharma et al. (2021), compared two SMFCs, having plant and microalgae in the cathode chamber. The algae-based SMFC performed better in terms of COD, phosphate and nitrate removal and the algae biomass productivity of 0.031 Kg/m3/d was attained (Sharma et al., 2021). Similarly, in another study involving SMFC, a power density of 5.17 W/m3 was obtained using Chlorella vulgaris at the cathode (Song et al., 2020).
In addition to this, most of the AMFC studies have been carried out in batch mode, whereas to bring the technology from lab to the real world the process should be either in continuous or semi-continuous mode (Kannan and Donnellan, 2021). Nguyen and Min (2020) treated leachate wastewater in continuous mode with a hydraulic retention time of 20 h. The effluent from anodic compartment was recycled to the cathodic chamber for the growth of algae, ensuring maximum removal of COD and reutilization of nutrients (Nguyen and Min, 2020).
9 Future outlook
This review summarizes the potential of third generation biofuels and their integration with BESs. Several studies have shown the possible applications of AMFCs at commercial scale. Researchers have explored few macroalgae spp. in AMFCs and concluded that employment of macroalgae in AMFCs can be more beneficial than that of microalgae in terms of waste treatment and algal biomass harvesting. Further research is required to assess the full potential of AFMCs. In addition, approaches to increase the efficiency by selecting the correct microbial consortia/microalgae, required for wastewater treatment, biofuel, biomass and bioelectricity generation are of paramount importance.
Considerable research has also been carried out on genetical modification of microalgae to magnify their cellular potential. These genetic engineering strategies along with omics would extend the existing knowledge of metabolic pathways. However, studies on employment of genetically modified algae in AMFCs are very limited and need further investigation.
The better understanding of the electron transfer mechanisms between electrode and microbes can further aid in selecting suitable strains and electrode materials to boost the power output. Additionally, the selection of better membranes and electrodes which are easily scalable at affordable cost needs further research. Also, novel engineering solutions for reactor design to promote algal growth and boost power output along with easy harvesting of algal biomass, are required. Furthermore, integration of AMFCs with different technologies such as anaerobic digestion (AD) can add more value to the overall operation and increase process efficiency, nutrient uptake and waste removal. For example, a typical AD process treating food waste can generate biomethane and the AD effluent can be utilized in AMFCs for both bacteria and algae in the anodic and cathodic chamber, respectively. The overall output from this coupled process such as biomethane, bioelectricity, waste treatment and algal biomass can be developed as a biorefinery.
Author contributions
AK: Conceptualization, Writing - original draft. MC: Conceptualization, Writing - review and editing, Visualization, Supervision. PL: Writing - review and editing, Supervision, Resources. All authors contributed to the article and approved the submitted version.
Funding
This publication has emanated from research supported by Science Foundation Ireland (SFI) through the SFI Research Professorship Programme entitled Innovative Energy Technologies for Biofuels, Bioenergy and a Sustainable Irish Bioeconomy (IETSBIO3; grant number 15/RP/2763), the Research Infrastructure research grant Platform for Biofuel Analysis (Grant Number 16/RI/3401) and the Science Foundation Ireland (SFI) and Department of Foreign Affairs (DFA) under the SDG Challenge project Floating Treatment Wetland (grant number SFI/21/FIP/SDG/9933).
Conflict of interest
The authors declare that the research was conducted in the absence of any commercial or financial relationships that could be construed as a potential conflict of interest.
Publisher’s note
All claims expressed in this article are solely those of the authors and do not necessarily represent those of their affiliated organizations, or those of the publisher, the editors and the reviewers. Any product that may be evaluated in this article, or claim that may be made by its manufacturer, is not guaranteed or endorsed by the publisher.
References
Abu-Ghosh, S., Dubinsky, Z., Verdelho, V., Iluz, D. (2021). Unconventional high-value products from microalgae: A review. Bioresource Technol. 329, 124895.
Alam, F., Mobin, S., Chowdhury, H. (2015). Third generation biofuel from algae. Proc. Eng. 105, 763–768. doi: 10.1016/j.proeng.2015.05.068
Ale, M. T., Meyer, A. S. (2013). Fucoidans from brown seaweeds: An update on structures, extraction techniques and use of enzymes as tools for structural elucidation. RSC Adv. 3, 8131–8141. doi: 10.1039/c3ra23373a
Angioni, S., Millia, L., Mustarelli, P., Doria, E., Temporiti, M. E., Mannucci, B., et al. (2018). Photosynthetic microbial fuel cell with polybenzimidazole membrane: Synergy between bacteria and algae for wastewater removal and biore fi nery. Heliyon 4, e00560. doi: 10.1016/j.heliyon.2018.e00560
Barbot, Y. N., Al-Ghaili, H., Benz, R. (2016). A review on the valorization of macroalgal wastes for biomethane production. Mar. Drugs 14, 1–27. doi: 10.3390/md14060120
Barkia, I., Saari, N., Manning, S. R. (2019). Microalgae for high-value products towards human health and nutrition. Mar. Drugs 17, 1–29. doi: 10.3390/md17050304
Bazdar, E., Roshandel, R., Yaghmaei, S., Mardanpour, M. M. (2018). The effect of different light intensities and light/dark regimes on the performance of photosynthetic microalgae microbial fuel cell. Bioresour. Technol. 261, 350–360. doi: 10.1016/j.biortech.2018.04.026
Behera, S., Singh, R., Arora, R., Sharma, N. K., Shukla, M., Kumar, S. (2015). Scope of algae as third generation biofuels. Front. Bioeng. Biotechnol. 2. doi: 10.3389/fbioe.2014.00090
Brennan, L., Owende, P. (2010). Biofuels from microalgae-a review of technologies for production, processing, and extractions of biofuels and co-products. Renew. Sustain. Energy Rev. 14, 557–577. doi: 10.1016/j.rser.2009.10.009
Cai, P. J., Xiao, X., He, Y. R., Li, W. W., Zang, G. L., Sheng, G. P., et al. (2013). Reactive oxygen species (ROS) generated by cyanobacteria act as an electron acceptor in the biocathode of a bio-electrochemical system. Biosens. Bioelectron. 39, 306–310. doi: 10.1016/j.bios.2012.06.058
Cesaro, A., Belgiorno, V. (2015). Combined biogas and bioethanol production: Opportunities and challenges for industrial application. Energies 8, 8121–8144. doi: 10.3390/en8088121
Chaudhuri, S. K., Lovley, D. R. (2003). Electricity generation by direct oxidation of glucose in mediatorless microbial fuel cells. Nat. Biotechnol. 21, 1229–1232. doi: 10.1038/nbt867
Chew, K. W., Chia, S. R., Show, P. L., Yap, Y. J., Ling, T. C., Chang, J. S. (2018). Effects of water culture medium, cultivation systems and growth modes for microalgae cultivation: A review. J. Taiwan Inst. Chem. Eng. 91, 332–344. doi: 10.1016/j.jtice.2018.05.039
Chisti, Y. (2007). Biodiesel from microalgae. Biotechnol. Adv. 25, 294–306. doi: 10.1016/j.biotechadv.2007.02.001
Chiu, S., Kao, C., Tsai, M., Ong, S., Chen, C., Lin, C. (2009). Bioresource technology lipid accumulation and CO 2 utilization of nannochloropsis oculata in response to CO 2 aeration. Bioresour. Technol. 100, 833–838. doi: 10.1016/j.biortech.2008.06.061
Chowdhury, H., Loganathan, B. (2019). ScienceDirect third-generation biofuels from microalgae: a review. Curr. Opin. Green Sustain. Chem. 20, 39–44. doi: 10.1016/j.cogsc.2019.09.003
Colombo, A., Marzorati, S., Lucchini, G., Cristiani, P., Pant, D., Schievano, A. (2017). Assisting cultivation of photosynthetic microorganisms by microbial fuel cells to enhance nutrients recovery from wastewater. Bioresour. Technol. 237, 240–248. doi: 10.1016/j.biortech.2017.03.038
Commault, A. S., Laczka, O., Siboni, N., Tamburic, B., Crosswell, J. R., Seymour, J. R., et al. (2017). Electricity and biomass production in a bacteria-chlorella based microbial fuel cell treating wastewater. J. Power Sources 356, 299–309. doi: 10.1016/j.jpowsour.2017.03.097
Cui, Y., Rashid, N., Hu, N., Saif, M., Rehman, U., Han, J. (2014). Electricity generation and microalgae cultivation in microbial fuel cell using microalgae-enriched anode and bio-cathode. Energy Convers. Manage. 79, 674–680. doi: 10.1016/j.enconman.2013.12.032
Debnath, C., Bandyopadhyay, T. K., Bhunia, B., Mishra, U., Narayanasamy, S., Muthuraj, M. (2021). Microalgae: Sustainable resource of carbohydrates in third-generation biofuel production. Renew. Sustain. Energy Rev. 150, 111464. doi: 10.1016/j.rser.2021.111464
De Farias Silva, C. E., Sforza, E., Bertucco, A. (2019). Enhancing carbohydrate productivity in photosynthetic microorganism production: a comparison between cyanobacteria and microalgae and the effect of cultivation systems," in Advances in feedstock conversion technologies for alternative fuels and bioproducts. (United Kingdom Woodhead Publishing: Elsevier), 36–67.
Demirbaş, A. (2009). Production of biodiesel from algae oils. Energy Sources Part A Recover. Util. Environ. Eff. 31, 163–168. doi: 10.1080/15567030701521775
Demirbas, A. (2010). Use of algae as biofuel sources. Energy Convers. Manage. 51, 2738–2749. doi: 10.1016/j.enconman.2010.06.010
Dickinson, S., Mientus, M., Frey, D., Amini-Hajibashi, A., Ozturk, S., Shaikh, F., et al. (2017). A review of biodiesel production from microalgae. Clean Technol. Environ. Policy 19, 637–668. doi: 10.1007/s10098-016-1309-6
Efremenko, E. N., Nikolskaya, A. B., Lyagin, I. V., Senko, O. V., Makhlis, T. A., Stepanov, N. A., et al. (2012). Production of biofuels from pretreated microalgae biomass by anaerobic fermentation with immobilized clostridium acetobutylicum cells. Bioresour. Technol. 114, 342–348. doi: 10.1016/j.biortech.2012.03.049
Elmekawy, A., Hegab, H. M., Vanbroekhoven, K., Pant, D. (2014). Techno-productive potential of photosynthetic microbial fuel cells through different con fi gurations. Renew. Sustain. Energy Rev. 39, 617–627. doi: 10.1016/j.rser.2014.07.116
Enamala, M. K., Enamala, S., Chavali, M., Donepudi, J., Yadavalli, R., Kolapalli, B., et al. (2018). Production of biofuels from microalgae - a review on cultivation, harvesting, lipid extraction, and numerous applications of microalgae. Renew. Sustain. Energy Rev. 94, 49–68. doi: 10.1016/j.rser.2018.05.012
Gajda, I., Greenman, J., Melhuish, C., Ieropoulos, I. (2015). Self-sustainable electricity production from algae grown in a microbial fuel cell system. Biomass Bioenergy 82, 87–93. doi: 10.1016/j.biombioe.2015.05.017
Ghasemi, M., Daud, W. R. W., Ismail, A. F., Jafari, Y., Ismail, M., Mayahi, A., et al. (2013). Simultaneous wastewater treatment and electricity generation by microbial fuel cell: Performance comparison and cost investigation of using nafion 117 and SPEEK as separators. Desalination 325, 1–6. doi: 10.1016/j.desal.2013.06.013
Ghasemi, M., Wan Daud, W. R., Alam, J., Ilbeygi, H., Sedighi, M., Ismail, A. F., et al. (2016). Treatment of two different water resources in desalination and microbial fuel cell processes by poly sulfone/Sulfonated poly ether ether ketone hybrid membrane. Energy 96, 303–313. doi: 10.1016/j.energy.2015.12.053
Gollakota, A. R. K., Kishore, N., Gu, S. (2018). A review on hydrothermal liquefaction of biomass. Renew. Sustain. Energy Rev. 81, 1378–1392. doi: 10.1016/j.rser.2017.05.178
González, A., Cañizares, P., Rodrigo, M. A., Fernández, F. J., Lobato, J. (2013). Microbial fuel cell with an algae-assisted cathode: A preliminary assessment. J. Power Sources 242, 638–645. doi: 10.1016/j.jpowsour.2013.05.110
González Del Campo, A., Cañizares, P., Rodrigo, M. A., Fernández, F. J., Lobato, J. (2013). Microbial fuel cell with an algae-assisted cathode: A preliminary assessment. J. Power Sources 242, 638–645. doi: 10.1016/j.jpowsour.2013.05.110
Gonzalez del Campo, A., Perez, J. F., Cañizares, P., Rodrigo, M. A., Fernandez, F. J., Lobato, J. (2014). Study of a photosynthetic MFC for energy recovery from synthetic industrial fruit juice wastewater. Int. J. Hydrogen Energy 39, 21828–21836. doi: 10.1016/j.ijhydene.2014.07.055
Gonzalez Del Campo, A., Perez, J. F., Cañizares, P., Rodrigo, M. A., Fernandez, F. J., Lobato, J. (2015). Characterization of light/dark cycle and long-term performance test in a photosynthetic microbial fuel cell. Fuel 140, 209–216. doi: 10.1016/j.fuel.2014.09.087
Gouveia, L., Neves, C., Sebastião, D., Nobre, B. P., Matos, C. T. (2014). Effect of light on the production of bioelectricity and added-value microalgae biomass in a photosynthetic alga microbial fuel cell. Bioresour. Technol. 154, 171–177. doi: 10.1016/j.biortech.2013.12.049
Guo, K., Prévoteau, A., Patil, S. A., Rabaey, K. (2015). Engineering electrodes for microbial electrocatalysis. Curr. Opin. Biotechnol. 33, 149–156. doi: 10.1016/j.copbio.2015.02.014
Hans, N., Malik, A., Naik, S. (2021). Antiviral activity of sulfated polysaccharides from marine algae and its application in combating COVID-19: Mini review. Bioresource Technol. Rep. 13, 100623.
He, L., Du, P., Chen, Y., Lu, H., Cheng, X., Chang, B., et al. (2017). Advances in microbial fuel cells for wastewater treatment. Renew. Sustain. Energy Rev. 71, 388–403. doi: 10.1016/j.rser.2016.12.069
Hildebrand, M., Abbriano, R. M., Polle, J. E. W., Traller, J. C., Trentacoste, E. M., Smith, S. R., et al. (2013). Metabolic and cellular organization in evolutionarily diverse microalgae as related to biofuels production. Curr. Opin. Chem. Biol. 17, 506–514. doi: 10.1016/j.cbpa.2013.02.027
Hindatu, Y., Annuar, M. S. M., Gumel, A. M. (2017). Mini-review: Anode modification for improved performance of microbial fuel cell. Renew. Sustain. Energy Rev. 73, 236–248. doi: 10.1016/j.rser.2017.01.138
Hossain, N., Mahlia, T. M. I. (2019). Progress in physicochemical parameters of microalgae cultivation for biofuel production. Crit. Rev. Biotechnol. 39, 835–859. doi: 10.1080/07388551.2019.1624945
Hosseini, M. G., Ahadzadeh, I. (2012). A dual-chambered microbial fuel cell with ti/nano-TiO 2/Pd nano-structure cathode. J. Power Sources 220, 292–297. doi: 10.1016/j.jpowsour.2012.07.096
Hou, Q., Nie, C., Pei, H., Hu, W., Jiang, L., Yang, Z. (2016). Bioresource technology the effect of algae species on the bioelectricity and biodiesel generation through open-air cathode microbial fuel cell with kitchen waste anaerobically digested effluent as substrate. Bioresour. Technol. 218, 902–908. doi: 10.1016/j.biortech.2016.07.035
Hou, B., Sun, J., Hu, Y.y. (2011). Simultaneous Congo red decolorization and electricity generation in air-cathode single-chamber microbial fuel cell with different microfiltration, ultrafiltration and proton exchange membranes. Bioresour. Technol. 102, 4433–4438. doi: 10.1016/j.biortech.2010.12.092
Huarachi-Olivera, R., Dueñas-Gonza, A., Yapo-Pari, U., Vega, P., Romero-Ugarte, M., Tapia, J., et al. (2018). Bioelectrogenesis with microbial fuel cells (MFCs) using the microalga chlorella vulgaris and bacterial communities. Electron. J. Biotechnol. 31, 34–43. doi: 10.1016/j.ejbt.2017.10.013
Hu, X., Zhou, J., Liu, B. (2016). Effect of algal species and light intensity on the performance of an air-lift-type microbial carbon capture cell with an algae-assisted cathode. RSC Adv. 6, 25094–25100. doi: 10.1039/c5ra26299b
Ieropoulos, I. A., Stinchcombe, A., Gajda, I., Forbes, S., Merino-Jimenez, I., Pasternak, G., et al. (2016). Pee power urinal-microbial fuel cell technology field trials in the context of sanitation. Environ. Sci. Water Res. Technol. 2, 336–343. doi: 10.1039/c5ew00270b
Ilbeygi, H., Ghasemi, M., Emadzadeh, D., Ismail, A. F., Zaidi, S. M. J., Aljlil, S. A., et al. (2015). Power generation and wastewater treatment using a novel SPEEK nanocomposite membrane in a dual chamber microbial fuel cell. Int. J. Hydrogen Energy 40, 477–487. doi: 10.1016/j.ijhydene.2014.10.026
Illman, A. M., Scragg, A. H., Shales, S. W. (2000). Increase in chlorella strains calorific values when grown in low nitrogen medium. Enzyme Microb. Technol. 27, 631–635. doi: 10.1016/S0141-0229(00)00266-0
Jadhav, D. A., Jain, S. C., Ghangrekar, M. M. (2016). Cow’s urine as a yellow gold for bioelectricity generation in low cost clayware microbial fuel cell. Energy 113, 76–84. doi: 10.1016/j.energy.2016.07.025
Kakarla, R., Min, B. (2019). Sustainable electricity generation and ammonium removal by microbial fuel cell with a microalgae assisted cathode at various environmental conditions. Bioresour. Technol. 284, 161–167. doi: 10.1016/j.biortech.2019.03.111
Kang, K. H., Jang, J. K., Pham, T. H., Moon, H., Chang, I. S., Kim, B. H. (2003). A microbial fuel cell with improved cathode reaction as a low biochemical oxygen demand sensor. Biotechnol. Lett. 25, 1357–1361. doi: 10.1023/A:1024984521699
Kannan, N., Donnellan, P. (2021). Algae-assisted microbial fuel cells: A practical overview. Bioresour. Technol. Rep. 15, 100747. doi: 10.1016/j.biteb.2021.100747
Kazbar, A., Cogne, G., Urbain, B., Marec, H., Le-Gouic, B., Tallec, J., et al. (2019). Effect of dissolved oxygen concentration on microalgal culture in photobioreactors. Algal Res. 39, 101432. doi: 10.1016/j.algal.2019.101432
Khandelwal, A., Chhabra, M., Yadav, P. (2020). Performance evaluation of algae assisted microbial fuel cell under outdoor conditions. Bioresour. Technol. 310, 123418. doi: 10.1016/j.biortech.2020.123418
Khandelwal, A., Dhindhoria, K., Dixit, A., Chhabra, M. (2021). Superiority of activated graphite/CuO composite electrode over platinum based electrodes as cathode in algae assisted microbial fuel cell. Environ. Technol. Innov. 24, 101891. doi: 10.1016/j.eti.2021.101891
Khandelwal, A., Vijay, A., Dixit, A., Chhabra, M. (2018). Microbial fuel cell powered by lipid extracted algae: A promising system for algal lipids and power generation. Bioresour. Technol. 247, 520–527. doi: 10.1016/j.biortech.2017.09.119
Khandelwal, A., Vijay, A., Jadhav, D. A., Lens, P. N. L., Swaminathan, J., Ghosh, P. C., et al. (2022). Genesis and recent advancement in microbial fuel cells: Wastewater treatment and resource recovery perspectives. Nov. Approaches Towar. Wastewater Treat. Resour. Recover. Technol., 23–36. doi: 10.1016/b978-0-323-90627-2.00016-2
Klinthong, W., Yang, Y. H., Huang, C. H., Tan, C. S. (2015). A review: Microalgae and their applications in CO2 capture and renewable energy. Aerosol Air Qual. Res. 15, 712–742. doi: 10.4209/aaqr.2014.11.0299
Kostas, E. T., White, D. A., Du, C., Cook, D. J. (2016). Selection of yeast strains for bioethanol production from UK seaweeds. J. Appl. Phycol. 28, 1427–1441. doi: 10.1007/s10811-015-0633-2
Kumar, M., Sun, Y., Rathour, R., Pandey, A., Thakur, I. S., Tsang, D. C. W. (2020). Algae as potential feedstock for the production of biofuels and value-added products: Opportunities and challenges. Sci. Total Environ. 716, 137116. doi: 10.1016/j.scitotenv.2020.137116
Kumar, M., Thakur, I. S. (2018). Municipal secondary sludge as carbon source for production and characterization of biodiesel from oleaginous bacteria. Bioresour. Technol. Rep. 4, 106–113. doi: 10.1016/j.biteb.2018.09.011
Lakshmidevi, R., Gandhi, N. N., Muthukumar, K. (2020). Carbon neutral electricity production from municipal solid waste landfill leachate using algal-assisted microbial fuel cell. Appl. Biochem. Biotechnol. 191, 852–866. doi: 10.1007/s12010-019-03160-5
Lee, J. H. (2013). Polyunsaturated fatty acids in children. Pediatr. Gastroenterol. Hepatol. Nutr. 16, 153–161. doi: 10.5223/pghn.2013.16.3.153
Leng, L., Xiong, Q., Yang, L., Li, H., Zhou, Y., Zhang, W., et al. (2021). An overview on engineering the surface area and porosity of biochar. Sci. total Environ. 763, 144204.
Levasseur, W., Perré, P., Pozzobon, V. (2020). A review of high value-added molecules production by microalgae in light of the classification. Biotechnol. Adv. 41, 107545. doi: 10.1016/j.biotechadv.2020.107545
Lim, S. S., Daud, W. R. W., Md Jahim, J., Ghasemi, M., Chong, P. S., Ismail, M. (2012). Sulfonated poly(ether ether ketone)/poly(ether sulfone) composite membranes as an alternative proton exchange membrane in microbial fuel cells. Int. J. Hydrogen Energy 37, 11409–11424. doi: 10.1016/j.ijhydene.2012.04.155
Lim, J. H. K., Gan, Y. Y., Ong, H. C., Lau, B. F., Chen, W. H., Chong, C. T., et al. (2021). Utilization of microalgae for bio-jet fuel production in the aviation sector: Challenges and perspective. Renew. Sustain. Energy Rev. 149, 111396. doi: 10.1016/j.rser.2021.111396
Liu, J., Huang, J., Sun, Z., Zhong, Y., Jiang, Y., Chen, F. (2011). Bioresource technology differential lipid and fatty acid profiles of photoautotrophic and heterotrophic chlorella zofingiensis: Assessment of algal oils for biodiesel production. Bioresour. Technol. 102, 106–110. doi: 10.1016/j.biortech.2010.06.017
Liu, H., Ramnarayanan, R., Logan, B. E. (2004). Production of electricity during wastewater treatment using a single chamber microbial fuel cell. Environ. Sci. Technol. 38, 2281–2285. doi: 10.1021/es034923g
Logan, B. E., Regan, J. M. (2006). Microbial fuel cells–challenges and applications. Environ. Sci. Technol. 40, 5172–5180. doi: 10.1021/es0627592
Luo, S., Berges, J. A., He, Z., Young, E. B. (2017). Algal-microbial community collaboration for energy recovery and nutrient remediation from wastewater in integrated photobioelectrochemical systems. Algal Res 24, 527–539. doi: 10.1016/j.algal.2016.10.006
Matos, Â.P. (2017). The impact of microalgae in food science and technology. JAOCS J. Am. Oil Chem. Soc 94, 1333–1350. doi: 10.1007/s11746-017-3050-7
Ma, J., Wang, Z., Zhang, J., Waite, T. D., Wu, Z. (2017). Cost-effective chlorella biomass production from dilute wastewater using a novel photosynthetic microbial fuel cell (PMFC). Water Res. 108, 356–364. doi: 10.1016/j.watres.2016.11.016
McKennedy, J., Sherlock, O. (2015). Anaerobic digestion of marine macroalgae: A review. Renew. Sustain. Energy Rev. 52, 1781–1790. doi: 10.1016/j.rser.2015.07.101
Merino-Jimenez, I., Celorrio, V., Fermin, D. J., Greenman, J., Ieropoulos, I. (2017). Enhanced MFC power production and struvite recovery by the addition of sea salts to urine. Water Res. 109, 46–53. doi: 10.1016/j.watres.2016.11.017
Michalak, I., Baśladyńska, S., Mokrzycki, J., Rutkowski, P. (2019). Biochar from a freshwater macroalga as a potential biosorbent for wastewater treatment. Water (Switzerland) 11, 4–6. doi: 10.3390/w11071390
Milledge, J. J., Nielsen, B. V., Maneein, S., Harvey, P. J. (2019). A brief review of anaerobic digestion of algae for BioEnergy. Energies 12, 1–22. doi: 10.3390/en12061166
Minh, T. H., Hanh, V. (2012). Bioethanol production from marine algae biomass: prospect and troubles. J. Vietnamise Environ. 3, 55–29. doi: 10.13141/jve.vol3.no1.pp25-29
Mishra, A., Chhabra, M. (2022). Performance of photo-microbial fuel cell with dunaliella salina at the saline cathode. Bioresour. Technol. Rep. 19, 101199. doi: 10.1016/j.biteb.2022.101199
Mitra, D., van Leeuwen, J.(., Lamsal, B. (2012). Heterotrophic/mixotrophic cultivation of oleaginous chlorella vulgaris on industrial co-products. Algal Res. 1, 40–48. doi: 10.1016/j.algal.2012.03.002
Mondal, A., Bose, S., Banerjee, S., Patra, J. K., Malik, J., Mandal, S. K., et al. (2020). Marine cyanobacteria and microalgae metabolites–a rich source of potential anticancer drugs. Mar. Drugs 18 (9), 476.
Muñoz, C. F., Südfeld, C., Naduthodi, M. I. S., Weusthuis, R. A., Barbosa, M. J., Wijffels, R. H., et al. (2021). Genetic engineering of microalgae for enhanced lipid production. Biotechnol. Adv. 52, 107836. doi: 10.1016/j.biotechadv.2021.107836
Muthuraj, M., Palabhanvi, B., Misra, S., Kumar, V., Sivalingavasu, K., Das, D. (2013). Flux balance analysis of chlorella sp. FC2 IITG under photoautotrophic and heterotrophic growth conditions. Photosynth. Res. 118, 167–179. doi: 10.1007/s11120-013-9943-x
Nagendranatha Reddy, C., Nguyen, H. T. H., Noori, M. T., Min, B. (2019). Potential applications of algae in the cathode of microbial fuel cells for enhanced electricity generation with simultaneous nutrient removal and algae biorefinery: Current status and future perspectives. Bioresour. Technol. 292, 122010. doi: 10.1016/j.biortech.2019.122010
Narayanaswamy Venkatesan, P., Dharmalingam, S. (2015). Effect of cation transport of SPEEK - rutile TiO2 electrolyte on microbial fuel cell performance. J. Memb. Sci. 492, 518–527. doi: 10.1016/j.memsci.2015.06.025
Neethu, B., Ghangrekar, M. M. (2017). Electricity generation through a photo sediment microbial fuel cell using algae at the cathode. Water Sci. Technol. 76, 3269–3277. doi: 10.2166/wst.2017.485
Neethu, B., Khandelwal, A., Ghangrekar, M. M., Ihjas, K., Swaminathan, J. (2022). “Microbial fuel cells-challenges for commercialization and how they can be addressed,” Scaling Up of Microbial Electrochemical Systems. (Netherland: Elsevier), 393–418. doi: 10.1016/B978-0-323-90765-1.00021-6
Neto, J. M., Komesu, A., Martins, L. H., da, S., Gonçalves, V. O. O., de Oliveira, J. A. R., et al. (2019). “Third generation biofuels: An overview,” in Sustainable Bioenergy Advances and Impacts. (Netherland: Elsevier), 261–280. doi: 10.1016/B978-0-12-817654-2.00010-1
Nguyen, H. T. H., Kakarla, R., Min, B. (2017). Algae cathode microbial fuel cells for electricity generation and nutrient removal from landfill leachate wastewater. Int. J. Hydrogen Energy 42, 29433–29442. doi: 10.1016/j.ijhydene.2017.10.011
Nguyen, H. T. H., Min, B. (2020). Leachate treatment and electricity generation using an algae-cathode microbial fuel cell with continuous flow through the chambers in series. Sci. Total Environ. 723, 138054. doi: 10.1016/j.scitotenv.2020.138054
Nigam, P. S., Singh, A. (2011). Production of liquid biofuels from renewable resources. Prog. Energy Combust. Sci. 37, 52–68. doi: 10.1016/j.pecs.2010.01.003
Offei, F., Mensah, M., Thygesen, A., Kemausuor, F. (2018). Seaweed bioethanol production: A process selection review on hydrolysis and fermentation. Fermentation 4, 1–18. doi: 10.3390/fermentation4040099
Pandit, S., Nayak, B. K., Das, D. (2012). Bioresource technology microbial carbon capture cell using cyanobacteria for simultaneous power generation , carbon dioxide sequestration and wastewater treatment. Bioresour. Technol. 107, 97–102. doi: 10.1016/j.biortech.2011.12.067
Rabii, A., Aldin, S., Dahman, Y., Elbeshbishy, E. (2019). A review on anaerobic co-digestion with a focus on the microbial populations and the effect of multi-stage digester configuration. Energies 12, 1–25. doi: 10.3390/en12061106
Rinaldi, A., Mecheri, B., Garavaglia, V., Licoccia, S., Di Nardo, P., Traversa, E. (2008). Engineering materials and biology to boost performance of microbial fuel cells: A critical review. Energy Environ. Sci. 1, 417–429. doi: 10.1039/b806498a
Robak, K., Balcerek, M. (2018). Review of second generation bioethanol production from residual biomass. Food Technol. Biotechnol. 56, 174–187. doi: 10.17113/ftb.56.02.18.5428
Rodrigo, M. A., Cañizares, P., Lobato, J. (2010). Bioresource technology effect of the electron-acceptors on the performance of a MFC. Bioresour. Technol. 101, 7014–7018. doi: 10.1016/j.biortech.2010.04.013
Saba, B., Christy, A. D., Yu, Z., Co, A. C. (2017). Sustainable power generation from bacterio-algal microbial fuel cells (MFCs): An overview. Renew. Sustain. Energy Rev. 73, 75–84. doi: 10.1016/j.rser.2017.01.115
Salar-garcía, M. J., Gajda, I., Ortiz-martínez, V. M., Greenman, J., Hanczyc, M. M., Ríos, A. P. D. L. (2016). Microalgae as substrate in low cost terracotta-based microbial fuel cells: Novel application of the catholyte produced. Bioresour. Technol. (Netherland: Elsevier), 209, 380–385. doi: 10.1016/j.biortech.2016.02.083
Santoro, C., Arbizzani, C., Erable, B., Ieropoulos, I. (2017). Microbial fuel cells: From fundamentals to applications. a review. J. Power Sources 356, 225–244. doi: 10.1016/j.jpowsour.2017.03.109
Sato, N., Tsuzuki, M., Kawaguchi, A. (2003). Glycerolipid synthesis in chlorella kessleri 11 h II . effect of the CO 2 concentration during growth. Biochimica et Biophysica Acta (BBA) - Molecular and Cell Biology of Lipids (Elsevier) 1633, 35–42. doi: 10.1016/S1388-1981(03)00070-2
Schenk, P. M., Thomas-Hall, S. R., Stephens, E., Marx, U. C., Mussgnug, J. H., Posten, C., et al. (2008). Second generation biofuels: High-efficiency microalgae for biodiesel production. Bioenergy Res. 1, 20–43. doi: 10.1007/s12155-008-9008-8
Scott, S. A., Davey, M. P., Dennis, J. S., Horst, I., Howe, C. J., Lea-Smith, D. J., et al. (2010). Biodiesel from algae: Challenges and prospects. Curr. Opin. Biotechnol. 21, 277–286. doi: 10.1016/j.copbio.2010.03.005
Shabani, M., Younesi, H., Pontié, M., Rahimpour, A., Rahimnejad, M., Zinatizadeh, A. A. (2020). A critical review on recent proton exchange membranes applied in microbial fuel cells for renewable energy recovery. J. Clean. Prod. 264, 121446. doi: 10.1016/j.jclepro.2020.121446
Sharma, A., Gajbhiye, S., Chauhan, S., Chhabra, M. (2021). Effect of cathodic culture on wastewater treatment and power generation in a photosynthetic sediment microbial fuel cell (SMFC): Canna indica v/s chlorella vulgaris. Bioresour. Technol. 340, 125645. doi: 10.1016/j.biortech.2021.125645
Sialve, B., Bernet, N., Bernard, O. (2009). Anaerobic digestion of microalgae as a necessary step to make microalgal biodiesel sustainable. Biotechnol. Adv. 27, 409–416. doi: 10.1016/j.biotechadv.2009.03.001
Singh, S. P., Singh, P. (2014). Effect of CO 2 concentration on algal growth: A review. Renewable and Sustainable Energy Reviews (Elsevier) 38, 172–179. doi: 10.1016/j.rser.2014.05.043
Sivsankaran, A., Sangeetha, D. (2015). Influence of sulfonated SiO2 in sulfonated polyether ether ketone nanocomposite membrane in microbial fuel cell. Fuel 159, 689–696. doi: 10.1016/j.fuel.2015.07.002
Song, X., Wang, W., Cao, X., Wang, Y., Zou, L., Ge, X., et al. (2020). Chlorella vulgaris on the cathode promoted the performance of sediment microbial fuel cells for electrogenesis and pollutant removal. Sci. Total Environ. 728, 138011. doi: 10.1016/j.scitotenv.2020.138011
Steen, E. J., Kang, Y., Bokinsky, G., Hu, Z., Schirmer, A., McClure, A., et al. (2010). Microbial production of fatty-acid-derived fuels and chemicals from plant biomass. Nature 463, 559–562. doi: 10.1038/nature08721
Takagi, M., Karseno, Yoshida, T. (2006). Effect of salt concentration on intracellular accumulation of lipids and triacylglyceride in marine microalgae dunaliella cells. J. Biosci. Bioeng. 101, 223–226. doi: 10.1263/jbb.101.223
Taşkan, B., Taşkan, E. (2022). Sustainable bioelectricity generation using cladophora sp. as a biocathode in membrane-less microbial fuel cell. Bioresour. Technol. 347, 126704. doi: 10.1016/j.biortech.2022.126704
Thomas, W. H., Tornabene, T. G., Weissman., J. (1984). Screening for lipid yielding microalgae: activities for 1983. Final subcontract report. United States. doi: 10.2172/6838870
Ting, H., Haifeng, L., Shanshan, M., Zhang, Y., Zhidan, L., Na, D. (2017). Progress in microalgae cultivation photobioreactors and applications in wastewater treatment: A review. Int. J. Agric. Biol. Eng. 10, 1–29. doi: 10.3965/j.ijabe.20171001.2705
Tiwari, B. R., Noori, M. T., Ghangrekar, M. M. (2016). A novel low cost polyvinyl alcohol-nafion-borosilicate membrane separator for microbial fuel cell. Mater. Chem. Phys. 182, 86–93. doi: 10.1016/j.matchemphys.2016.07.008
Trapero, J. R., Horcajada, L., Linares, J. J., Lobato, J. (2017). Is microbial fuel cell technology ready? an economic answer towards industrial commercialization. Appl. Energy 185, 698–707. doi: 10.1016/j.apenergy.2016.10.109
Tremouli, A., Greenman, J., Ieropoulos, I. (2018). Investigation of ceramic MFC stacks for urine energy extraction. Bioelectrochemistry 123, 19–25. doi: 10.1016/j.bioelechem.2018.03.010
Tripathi, R., Singh, J., Thakur, I. S. (2015). Characterization of microalga scenedesmus sp. ISTGA1 for potential CO2 sequestration and biodiesel production. Renew. Energy 74, 774–781. doi: 10.1016/j.renene.2014.09.005
Velasquez-orta, S. B., Curtis, T. P., Logan, B. E. (2009). Energy from algae using microbial fuel cells. Biotechnology and Bioengineering (Wiley) 103, 1068–1076. doi: 10.1002/bit.22346
Wang, X., Feng, Y., Liu, J., Lee, H., Li, C., Li, N., et al. (2010). Biosensors and bioelectronics sequestration of CO 2 discharged from anode by algal cathode in microbial carbon capture cells ( MCCs ). Biosens. Bioelectron. 25, 2639–2643. doi: 10.1016/j.bios.2010.04.036
Wu, J. Y., Lay, C. H., Chia, S. R., Chew, K. W., Show, P. L., Hsieh, P. H., et al. (2021). Economic potential of bioremediation using immobilized microalgae-based microbial fuel cells. Clean Technol. Environ. Policy 23, 2251–2264. doi: 10.1007/s10098-021-02131-x
Xu, Q., Wang, L., Li, C., Wang, X., Li, C., Geng, Y. (2019). Study on improvement of the proton conductivity and anti-fouling of proton exchange membrane by doping SGO@SiO2 in microbial fuel cell applications. Int. J. Hydrogen Energy 44, 15322–15332. doi: 10.1016/j.ijhydene.2019.03.238
Yang, Z., Pei, H., Hou, Q., Jiang, L., Zhang, L., Nie, C. (2018). Algal biofilm-assisted microbial fuel cell to enhance domestic wastewater treatment: Nutrient, organics removal and bioenergy production. Chem. Eng. J. 332, 277–285. doi: 10.1016/j.cej.2017.09.096
Yang, Z., Zhang, L., Nie, C., Hou, Q., Zhang, S., Pei, H. (2019). Multiple anodic chambers sharing an algal raceway pond to establish a photosynthetic microbial fuel cell stack: Voltage boosting accompany wastewater treatment. Water Res. 164, 114955. doi: 10.1016/j.watres.2019.114955
Yu, K. L., Lau, B. F., Show, P. L., Ong, H. C., Ling, T. C., Chen, W. H., et al. (2017). Recent developments on algal biochar production and characterization. Bioresour. Technol. 246, 2–11. doi: 10.1016/j.biortech.2017.08.009
Zhang, Y., He, Q., Xia, L., Li, Y., Song, S. (2018). Algae cathode microbial fuel cells for cadmium removal with simultaneous electricity production using nickel foam/graphene electrode. Biochem. Eng. J. 138, 179–187. doi: 10.1016/j.bej.2018.07.021
Zhang, Y., Noori, J. S., Angelidaki, I. (2011). Simultaneous organic carbon, nutrients removal and energy production in a photomicrobial fuel cell (PFC). Energy Environ. Sci. 4, 4340–4346. doi: 10.1039/c1ee02089g
Zhang, X., Xia, X., Ivanov, I., Huang, X., Logan, B. E. (2014). Enhanced activated carbon cathode performance for microbial fuel cell by blending carbon black. Environmental science and technology (ACS) 48 (3), 2075–2081. doi: 10.1021/es405029y
Zhang, Y., Zhao, Y., Zhou, M. (2019). A photosynthetic algal microbial fuel cell for treating swine wastewater. Environ. Sci. pollut. Res. 26, 6182–6190. doi: 10.1007/s11356-018-3960-4
Zhou, M., Chi, M., Luo, J., He, H., Jin, T. (2011). An overview of electrode materials in microbial fuel cells. J. Power Sources 196, 4427–4435. doi: 10.1016/j.jpowsour.2011.01.012
Zhou, M., He, H., Jin, T., Wang, H. (2012). Power generation enhancement in novel microbial carbon capture cells with immobilized chlorella vulgaris. J. Power Sources 214, 216–219. doi: 10.1016/j.jpowsour.2012.04.043
Keywords: algae biomass, photobioreactor, microbial fuel cell, biofuels, bioelectricity
Citation: Khandelwal A, Chhabra M and Lens PNL (2023) Integration of third generation biofuels with bio-electrochemical systems: Current status and future perspective. Front. Plant Sci. 14:1081108. doi: 10.3389/fpls.2023.1081108
Received: 26 October 2022; Accepted: 20 January 2023;
Published: 10 February 2023.
Edited by:
Harish, Mohanlal Sukhadia University, IndiaReviewed by:
Quanyu Zhao, Najing Tech University, ChinaAmrik Singh Ahluwalia, Eternal University, India
Copyright © 2023 Khandelwal, Chhabra and Lens. This is an open-access article distributed under the terms of the Creative Commons Attribution License (CC BY). The use, distribution or reproduction in other forums is permitted, provided the original author(s) and the copyright owner(s) are credited and that the original publication in this journal is cited, in accordance with accepted academic practice. No use, distribution or reproduction is permitted which does not comply with these terms.
*Correspondence: Amitap Khandelwal, amitap.khandelwal@universityofgalway.ie, Khandelwal.1@iitj.ac.in
†These authors have contributed equally to this work