- Department of Crop Science, North Carolina State University, Raleigh, NC, USA
Extracellular polysaccharides are synthesized by a wide variety of species, from unicellular bacteria and Archaea to the largest multicellular plants and animals in the biosphere. In every case, the biosynthesis of these polymers requires transport across a membrane, from the cytosol to either the lumen of secretory pathway organelles or directly into the extracellular space. Although some polysaccharide biosynthetic substrates are moved across the membrane to sites of polysaccharide synthesis by separate transporter proteins before being incorporated into polymers by glycosyltransferase proteins, many polysaccharide biosynthetic enzymes appear to have both transporter and transferase activities. In these cases, the biosynthetic enzymes utilize substrate on one side of the membrane and deposit the polymer product on the other side. This review discusses structural characteristics of plant cell wall glycan synthases that couple synthesis with transport, drawing on what is known about such dual-function enzymes in other species.
Introduction
Extracellular matrices (ECMs) are complex biopolymer mixtures produced by cells and deposited outside the cell membrane. Diverse eukaryotic and prokaryotic organisms throughout the biosphere produce ECMs, making the ECM a common feature of most living cells. ECMs play an integral role in many essential processes, from influencing the growth and development of cellular and organismal morphology to mediating interactions between cells and their environments.
The specific composition and structure of the ECM varies widely depending on species, cell type, developmental stage, and environmental conditions. Despite these differences, there are some aspects of ECM composition that are similar throughout most of biology. One common feature of the ECM produced by diverse species is the prevalence of polysaccharides as major structural components. Examples include pectin, hemicellulose, and cellulose polymers of the plant cell wall, chitin found in fungal, mollusk, and arthropod ECMs, chondroitin and hyaluronan synthesized by vertebrates, and peptidoglycan of bacterial cell walls.
Although there is wide variation in composition, structure, and function of ECM polysaccharides found in nature, there are certain required steps that must be accomplished during the biosynthesis of any extracellular polysaccharide. Essential processes include the synthesis of precursor molecules and polymerization of the component sugars. Additionally, because the sugar-nucleotide precursors of ECM polymers are synthesized inside the cell, transport across a membrane of glycosyltransferase (GT) substrates or polysaccharide products is also required for ECM polysaccharide synthesis. This transport might occur across the plasma membrane (PM) directly into the extracellular space, or across an endomembrane into an intracellular compartment with the polymer later secreted to the ECM.
Transport across the membrane might occur before, during, or after polysaccharide synthesis. Each of these modes of transport probably occurs in nature, depending on the polysaccharide. In some cases, different sugar moieties incorporated into a polysaccharide may be transported across the membrane by different mechanisms. ECM polysaccharides whose activated sugar precursors are transported before polymerization include the glycan components of extracellular glycoproteins (Spiro, 2002), plant pectins (Mohnen, 2008), and ECM mannans in plants and fungi (Keegstra and Raikhel, 2001; Latgé, 2007; Reyes and Orellana, 2008). These polymers are synthesized in the lumen of secretory pathway compartments from sugar-nucleotides imported from the cytosol by transport proteins. ECM polymers transported across a membrane following at least partial synthesis include bacterial peptidoglycan precursor Lipid II (undecaprenyl-pyrophosphoryl-N-acetylmuramyl-(pentapeptide)-N-acetylglucosamine) and hyaluronan synthesized by “class II” hyaluronan synthases (HSs). These molecules are transported to the extracellular space by a lipid flippase (FtsW) and an ABC (ATP-binding cassette) transporter (such as MRP5), respectively (Schulz et al., 2007; Mohammadi et al., 2011).
In contrast to transport that occurs before or after polymer synthesis, this review focuses on another mechanism of transport across the membrane: transport that occurs at the same time as polymer synthesis and directly involves the biosynthetic enzyme. Enzymes that appear to processively synthesize ECM polysaccharides while transporting the polymer across a membrane are present in bacterial, fungal, animal, and plant systems. Such enzymes are referred to hereafter as dual-function GTs. Among the products of dual-function GTs are the some of the most economically and medically important polymers in nature. Included in this list are cellulose, the glucan backbone of the hemicellulose xyloglucan, chitin, much of the hyaluronan produced in animals, and at least two examples of polysaccharides produced by bacterial human pathogens (DeAngelis, 1999; Oglesby et al., 2008).
Because the structures have not been solved, the idea that some GTs also participate in polymer transport has not been rigorously proven. However, the topology of these proteins makes membrane transport of the polysaccharide by the glycan synthase seem likely. Dual-function GT proteins are characterized by a similar predicted topology with multiple transmembrane helices (TMHs) and an active site facing the cytosol.
In one mechanistic model for dual-function GTs, the TMHs are arranged to form a pore in the membrane. In this model, the cytosolic active site adds sugars from cytosolic substrate pools to an elongating polymer that extends across the membrane through the pore (Figure 1). Unfortunately, because dual-function GTs have multiple TMHs and many appear to function in protein complexes, determining their structures by traditional crystallographic methods is difficult. Nevertheless, evidence confirming the predicted topology described above continues to mount for a number of these proteins from different species (Heldermon et al., 2001; Urbanowicz et al., 2004; Zeng and Keegstra, 2008; Davis et al., 2010; Merzendorfer, 2011).
Although the group of proteins predicted to be dual-function GTs spans a large evolutionary distance, structural commonalities are likely considering their functional similarities. Because all dual-function GTs must accomplish similar processes, applying what we have learned about even distantly related proteins can be helpful in better understanding dual-function GTs in plants. Several plant ECM polysaccharide synthases have the topological requirements to be dual-function GTs. These enzymes include cellulose synthase (CESA), callose synthase (CalS), xyloglucan glucan synthase (cellulose synthase-like C4-CSLC4), and multiple related glycan synthases whose products have not been identified with certainty, including members of CSLD and CSLH clades (Verma and Hong, 2001; Zeng and Keegstra, 2008; Doblin et al., 2009; Davis et al., 2010; Carpita, 2011).
Structural Characteristics of Dual-Function Glycosyltransferases
Although we are just beginning to understand the fine structure of these proteins, we can identify some factors that are likely to be necessary for any dual-function GT. One strategy to accomplish this is to attempt to predict specific structural constraints based on how these proteins function. Another useful approach is to draw upon observations about diverse dual-function GTs to identify common features. These are important initial steps to generate realistic models and to design experimental approaches that are more likely to succeed.
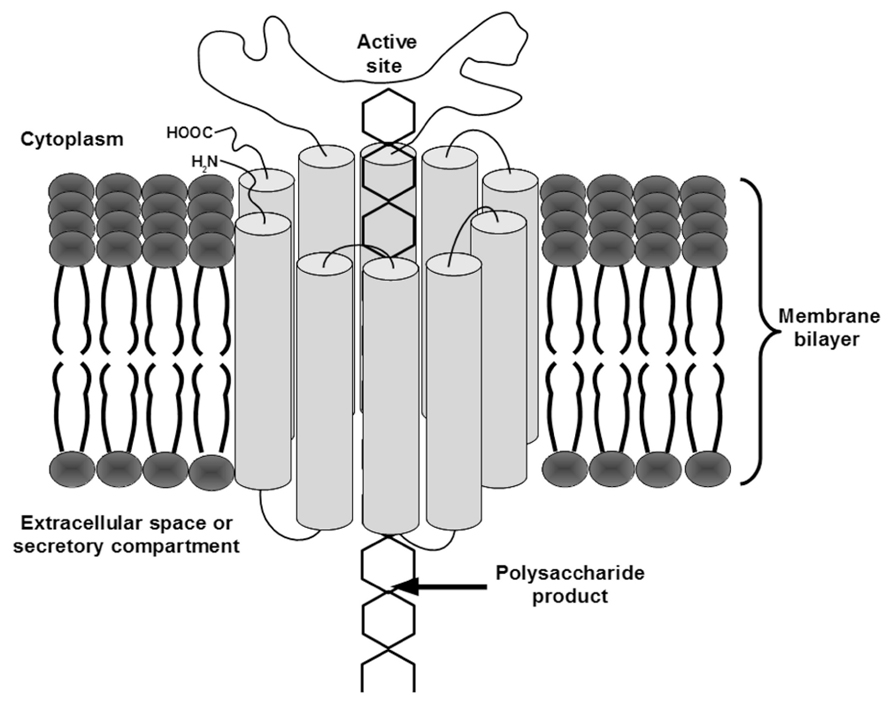
FIGURE 1. A model for dual-function GT transport mechanism. The TMHs of the enzyme are depicted as cylinders connected by curved lines representing the extra-membrane regions of the protein. A polymer is shown being transported through a pore formed by the TMHs, from the cytosolic active site where sugars are added, across the membrane to the extracellular space or lumen of the secretory pathway.
For a GT to transport its product across a membrane, the transmembrane regions of the protein or proteins comprising the active complex must create a pore large enough to accommodate the polysaccharide. An unsubstituted β-(1 → 4)-linked polymer of glucose (cellulose) is approximately 1 nm across in its widest axis. Because of the relatively high energy cost of partitioning glucose to a hydrophobic environment (Ha et al., 1991; Kollman, 1993; Mazzobre et al., 2005), it is likely that a glucose polymer remains hydrated as it moves across the membrane. Therefore, the absolute minimum diameter for a GT transmembrane pore would be about 1.8 nm, allowing for water molecules associated with the polysaccharide. Assuming the outer diameters of standard alpha helices range from 1 to 1.4 nm, the minimum number of TMHs required to form a pore larger than 1.8 nm is between 10 and 18 helices.
Although useful as a starting point for modeling the number of TMHs required for a dual-function GT, the calculations above are necessarily based on assumptions about the minimum diameter of a polysaccharide transport pore and the diameter of TMHs. In addition, this estimate assumes that TMHs are oriented perpendicular to the plane of the membrane. In reality, it is likely that some pore-forming TMHs of a dual-function GT span the membrane at an angle, creating a larger pore. For example, one bacterial HS has five to six membrane domains, functions as a monomer, and transports a polysaccharide with a wider diameter than the β-(1 → 4)-linked glucan described above (Tlapak-Simmons et al., 1998). This is possible in part because one or more of the membrane domains of this bacterial HS appear to be reentrant loops, occupying more space parallel to the plane of the membrane than a TMH inserted perpendicular to the membrane plane (Heldermon et al., 2001).
Another likely structural requirement for a pore-forming membrane protein is the ability to restrict free diffusion of water and other small molecules across the membrane. If a pore large enough to accommodate a hydrated polysaccharide were to remain open in the absence of a polymer occupying the pore, the electrochemical potential of the membrane would be compromised. Therefore, it is likely that dual-function GTs have a mechanism for pore gating. Gating could be mediated by the presence of an accessory protein, as occurs with the Sec61 translocon complex and the Hsp70 chaperone BiP (Alder et al., 2005). Alternatively, GT pore gating could be controlled by conformational changes in the GT itself. This might be accomplished through substrate binding or post-translational modification such as phosphorylation. Precedent for these mechanisms can be found in ligand-gated ion channels (Keramidasa et al., 2004) and the regulation of aquaporins by phosphorylation (Chaumont et al., 2005). In addition to the general requirement of cells to maintain membrane potential, this can be important for the function of dual-function GTs themselves, as evidenced by the ion potential requirement for the activity of CESA in plants and bacteria (Bacic and Delmer, 1981; Delmer et al., 1982).
There is a third possibility for how organisms with dual-function GTs might prevent compromise of the membrane gradient. By the time a dual-function GT protein is mature and competent to form a pore, it could be localized to a specialized vesicular compartment where free diffusion across the membrane has little consequence. Fusion of such vesicles to the appropriate membrane where polysaccharide biosynthesis occurs would be coupled with activation of the GT, followed by removal of the GT from the diffusion-sensitive membrane when synthesis is complete. If such a mechanism exists, it could provide a partial explanation for atypical vesicles observed in chitin (chitosomes) and cellulose biosynthesis (MASCs), and might relate to why CESA proteins spend such a short time at the PM (Bartnicki-Garcia, 2006; Crowell et al., 2010; Wightman and Turner, 2010).
Many dual-function GTs function in enzyme complexes, so proteins other than the glycan synthase may participate in the formation of a pore. Cocuron et al. (2007) found that Pichia pastoris expressing both the xyloglucan glucan synthase CSLC4 and the xyloglucan xylosyltransferase XXT1 accumulated insoluble, unsubstituted β-(1 → 4)-linked glucan. Much lower levels of this polymer accumulated in both wild-type Pichia and lines expressing only CSLC4, which produced shorter, soluble oligoglucans that were absent in dual-expressing and wild-type lines. In other words, CSLC4 functions as an active glucan synthase in both transgenic yeast cell lines, but produces a product with a much higher degree of polymerization when co-expressed with XXT1. Interestingly, Pichia does not synthesize UDP-xylose, the substrate of XXT1, and the β-(1 → 4)-linked glucan produced is unsubstituted. One explanation for this observation could be that XXT1 (a type II membrane protein) participates in the formation of a pore with CSLC4.
At least some dual-function GTs may be dependent on non-protein membrane components. A major advance in our understanding of plant CESA biochemistry has been the development of techniques to measure cellulose and callose synthesis in vitro (for a review, see Harris et al., 2010). This is accomplished through the isolation of detergent-resistant membranes (DRMs) and subsequent incubation of these membrane fractions with UDP-glucose. To date, there have been no successful attempts to solubilize active CESA away from DRMs.
Known as lipid “rafts” or membrane microdomains, the biological structures from which DRMs are derived have specific lipid and protein compositions that are distinct from the rest of the PM (Mongrand et al., 2010). A requirement for a specific lipid or sterol environment for active cellulose synthase complexes (CSCs) is consistent with these lipids playing a structural role in the complex, perhaps in allowing the formation of a transport pore(s). In support of this idea, a monomeric bacterial HS from Streptococcus requires multiple cardiolipin molecules for activity. It has been suggested that a specific number of cardiolipin molecules are associated with each active HS, playing a structural role to form a pore sufficient to accommodate the polymer product (Tlapak-Simmons et al., 1998).
One plant sterol in particular, sitosterol-β-glucoside (SG), has been implicated in cellulose biosynthesis. It has been suggested that this molecule may serve as a primer for initiation of cellulose synthesis (Peng et al., 2002). However, Arabidopsis plants with T-DNA insertions in two UDP-glucose:sterol glucosyltransferases are deficient in SG synthesis but not affected in cellulose accumulation (DeBolt et al., 2009). This observation is not consistent with SG functioning as a primer for cellulose, though the possibility that residual levels of SG in these plants is sufficient cannot be completely ruled out. An alternative explanation is that SG plays a structural role in the CSC, perhaps functioning in a manner similar to the putative role of cardiolipin in bacterial HS. If SG functions to promote proper folding of CESA proteins in the membrane, such a role might be more readily filled by unglycosylated sitosterol compared to the theoretical priming function. It is important to note that these two alternative functions for SG are not necessarily mutually exclusive.
Enzymes that are able to synthesize a polymer while extruding it across the membrane have a number of common structural features required for function, some of which are discussed above. Accomplishing membrane transport of a polymer product coupled with synthesis requires a certain level of complexity and imposes specific structural and compositional constraints on the enzyme and its lipid environment. It is perhaps surprising, then, that dual-function GTs are so widely distributed in diverse biological systems.
Why Did Dual-Function GTs Evolve?
With the prevalence of putative dual-function GTs in ECM polysaccharide biosynthesis from diverse species, it seems likely that the dual-function mechanism provides an evolutionary advantage. One possibility is that dual-function GTs allow greater control over enzyme function from within the cell. For example, the predicted topology of CESA proteins places over 90% of the hydrophilic regions of these proteins facing the cytosol. This presents large surfaces of CESA proteins to the cytosolic milieu, providing ample opportunity for interactions with cytosolic factors.
Multiple phosphorylation sites have been identified on CESA proteins using mass spectrometry-based approaches (Nühse et al., 2004; Taylor, 2007). Work by Taylor (2007) connected CESA7 protein phosphorylation at sites near the protein's amino terminus with proteasome-dependent degradation. This study opens many potential avenues of exploration. For instance, under what circumstances is CESA7 targeted for phosphorylation, and which proteins control the phosphorylation status? Does phosphorylation target CESA7 for proteasome degradation directly? Alternatively, might phosphorylation regulate another process such as CSC assembly or motility, which subsequently results in proteasome degradation?
Chen et al. (2010) investigated potential physiological roles of multiple phosphorylation sites on CESA1. The researchers expressed versions of CESA1 with mutations designed to eliminate or mimic protein phosphorylation at several sites. Depending on the phosphorylation site and modification, some transgenic plants exhibited altered cell expansion anisotropy. In the plants with altered cell expansion, movement of CSCs along microtubules was also affected. Though the molecular mechanisms are not understood, this work indicates that CESA phosphorylation can influence microtubule-directed translation of actively synthesizing CSCs, potentially through interactions with proteins connecting the CSC to microtubule “tracks.”
Recently, Gu et al. (2010) identified a protein that appears to be involved with physically connecting CSCs to cortical microtubules, highlighting another important interaction between the dual-function GT CESA proteins and cytosolic factors. Through a yeast two-hybrid approach, Gu et al. (2010) identified an armadillo repeat protein they termed cellulose synthase-interactive protein 1 (CSI1). Plants with disrupted CSI1 have reduced CSC motility and less uniformity of cellulose microfibril orientation (Gu et al., 2010). The CSI1 gene was later shown to be allelic with a previously identified cell expansion mutant POM-POM2 (Hauser et al., 1995) and further characterized by Bringmann et al. (2012). This work convincingly demonstrates that POM2/CSI1 protein is important for CESA protein association with microtubules (Bringmann et al., 2012). Considering the results of Chen et al. (2010) and the recent work on CSI1, it will be interesting to see whether CESA phosphorylation is related to CSI1 function.
In addition to controlling motility and degradation of CESA, another potential advantage of a cytosolic active site involves substrate availability. As mentioned above, the activated sugars that are substrates for polysaccharide synthesis are made primarily in the cytosol. So cytosolic active sites allow GTs to access substrate without requiring an independent transport protein. This might be of particular advantage for PM-localized dual-function GTs, where relatively labile activated sugars might degrade or diffuse away from sites of synthesis if exported before polymer synthesis. Taking this idea a step further, a cytosolic active site might allow the development of protein interactions for metabolite channeling. Indeed, sucrose synthase (SUS), which converts sucrose into fructose and UDP-glucose, the substrate for cellulose biosynthesis, has been observed at the PM (Amor et al., 1995) directly associated with sites of cellulose synthesis and structures isolated from membranes that appear to be CSCs (Salnikov et al., 2000; Fujii et al., 2010).
Another potentially advantageous structural property of dual-function GTs involves the TMHs. If the model shown in Figure 1 is representative of the true structures of these proteins, the acceptor substrate (elongating polysaccharide) is situated among the TMHs during elongation. These regions may have other functions in addition to forming a pore for polymer transport across the membrane. It is possible that the TMHs could affect enzyme processivity by holding the product/acceptor substrate in place during catalysis. Recent work by Harris et al. (2012) showed that mutations within a TMH of a CESA protein can reduce cellulose microfibril crystallinity and alter the speed of CSC movement on the PM. The authors present evidence that the TMHs of dual-function GTs may have a role in promoting appropriate interactions between the polymer product and other molecules in the extracellular space. For example, the TMHs might participate in orienting the polysaccharide as it is extruded across the membrane, which could in turn affect cellulose crystallinity.
Conclusion
Dual-function GTs are fascinating biochemical machines that synthesize ECM polysaccharides in a remarkable variety of biological systems. We are just beginning to understand how these proteins function, and ongoing research is likely to produce exciting advances in the coming years. A better understanding of these proteins will provide insight into ECM synthesis, one of the most fundamental biological processes in nature. Knowing how these enzymes function can also lead to the ability to modulate their activities, which could have tremendous impact on plant biotechnology and human health.
Conflict of Interest Statement
The author declares that the research was conducted in the absence of any commercial or financial relationships that could be construed as a potential con- flict of interest.
Acknowledgments
This manuscript is supported by The Center for Lignocellulose Structure and Formation, an Energy Frontiers Research Center funded by the U.S. Department of Energy, Office of Science, Office of Basic Energy Sciences under Award Number DE-SC0001090. The author also thanks Candace Haigler for assistance in editing the manuscript.
References
Alder, N. N., Shen, Y., Brodsky, J. L., Hendershot, L. M., and Johnson, A. E. (2005). The molecular mechanisms underlying BiP-mediated gating of the Sec61 translocon of the endoplasmic reticulum. J. Cell Biol. 168, 389–399.
Amor, Y., Haigler, C. H., Johnson, S., Wainscott, M., and Delmer, D. P. (1995). A membrane-associated form of sucrose synthase and its potential role in synthesis of cellulose and callose in plants. Proc. Natl. Acad. Sci. U.S.A. 92, 9353–9357.
Bacic, A., and Delmer, D. P. (1981). Stimulation of membrane-associated polysaccharide synthetases by a membrane potential in developing cotton fibers. Planta 152, 346–351.
Bringmann, M., Li, E., Sampathkumar, A., Kocabek, T., Hauser, M.-T., and Persson, S. (2012). POM-POM2/CELLULOSE SYNTHASE INTERACTING1 is essential for the functional association of cellulose synthase and microtubules in Arabidopsis. Plant Cell 24, 163–177.
Carpita, N. (2011). Update on mechanisms of plant cell wall biosynthesis: how plants make cellulose and other (1 → 4)-β-D-glycans. Plant Physiol. 155, 171–184.
Chaumont, F., Moshelion, M., and Daniels, M. J. (2005). Regulation of plant aquaporin activity. Biol. Cell 97, 749–764.
Chen, S., Ehrhardt, D. W., and Somerville, C. R. (2010). Mutations of cellulose synthase (CESA1) phosphorylation sites modulate anisotropic cell expansion and bidirectional mobility of cellulose synthase. Proc. Natl. Acad. Sci. U.S.A. 107, 17188–17193.
Cocuron, J.-C., Lerouxel, O., Drakakaki, G., Alonso, A.-P., Liepman, A., Keegstra, K., Raikhel, N., and Wilkerson, C. (2007). A gene from the cellulose synthase-like C family encodes a xyloglucan synthase. Proc. Natl. Acad. Sci. U.S.A. 104, 8550–8555.
Crowell, E. F., Gonneau, M., Stierhof, Y.-D., Höfte, H., and Vernhettes, S. (2010). Regulated trafficking of cellulose synthases. Curr. Opin. Plant Biol. 13, 700–705.
Davis, J., Brandizzi, F., Liepman, A., and Keegstra, K. (2010). Arabidopsis mannan synthase CSLA9 and glucan synthase CSLC4 have opposite orientations in the Golgi membrane. Plant J. 64, 1028–1037.
DeAngelis, P. L. (1999). Hyaluronan synthases: fascinating glycosyltransferases from vertebrates, bacterial pathogens, and algal viruses. Cell. Mol. Life Sci. 56, 670–682.
DeBolt, S., Scheible, W.-R., Schrick, K., Auer, M., Beisson, F., Bischoff, V., Bouvier-Navé, P., Carroll, A., Hematy, K., Li, Y., Milne, J., Nair, M., Schaller, H., Zemla, M., and Somerville, C. R. (2009). Mutations in UDP-glucose:sterol glucosyltransferase in Arabidopsis cause transparent testa phenotype and suberization defect in seeds. Plant Physiol. 151, 78–87.
Delmer, D. P., Benziman, M., and Padan, E. (1982). Requirement for a membrane potential for cellulose synthesis in intact cells of Acetobacter xylinum. Proc. Natl. Acad. Sci. U.S.A. 79, 5282–5286.
Doblin, M. S., Pettolino, F. A., Wilson, S. M., Campbell, R., Burton, R. A., Fincher, G. B., Newbigin, E., and Bacic, A. (2009). A barley cellulose synthase-like CSLH gene mediates (1,3;1,4)-β-D-glucan synthesis in transgenic Arabidopsis. Proc. Natl. Acad. Sci. U.S.A. 106, 5996–6001.
Fujii, S., Hayashi, T., and Mizuno, K. (2010). Sucrose synthase is an integral component of the cellulose synthesis machinery. Plant Cell Physiol. 51, 294–301.
Gu, Y., Kaplinsky, N., Bringmannc, M., Cobb, A., Carrolla, A., Sampathkumar, A., Baskin, T. I., Persson, S., and Somerville, C. R. (2010). Identification of a cellulose synthase-associated protein required for cellulose biosynthesis. Proc. Natl. Acad. Sci. U.S.A. 107, 12866–12871.
Ha, S., Gao, J., Tidor, B., Brady, J. W., and Karplus, M. (1991). Solvent effect on the anomeric equilibrium in D-glucose: a free energy simulation analysis. J. Am. Chem. Soc. 113, 1553–1557.
Harris, D. M., Bulone, V., Ding, S.-Y., and DeBolt, S. (2010). Tools for cellulose analysis in plant cell walls. Plant Physiol. 153, 420–426.
Harris, D. M., Corbin, K., Wang, T., Gutierrez, R., Bertolo, A. L., Petti, C., Smilgiese, D.-M., Estevez, J. M., Bonetta, D., Urbanowicz, B. R., Ehrhardt, D. W., Somerville, C. R., Rose, J. K. C., Hong, M., and DeBolt, S. (2012). Cellulose microfibril crystallinity is reduced by mutating C-terminal transmembrane region residues CESA1A903V and CESA3T942I of cellulose synthase. Proc. Natl. Acad. Sci. U.S.A. 109, 4098–4103.
Hauser, M. T., Morikami, A., and Benfey, P. N. (1995). Conditional root expansion mutants of Arabidopsis. Development 121, 1237–1252.
Heldermon, C., DeAngelis, P. L., and Weigel, P. (2001). Topological organization of the hyaluronan synthase from Streptococcus pyogenes. J. Biol. Chem. 276, 2037–2046.
Keegstra, K., and Raikhel, N. (2001). Plant glycosyltransferases. Curr. Opin. Plant Biol. 4, 219–224.
Keramidasa, A., Moorhousea, A. J., Schofieldb, P. R., and Barrya, P. H. (2004). Ligand-gated ion channels: mechanisms underlying ion selectivity. Prog. Biophys. Mol. Biol. 86, 161–204.
Kollman, P. (1993). Free energy calculations: applications to chemical and biochemical phenomena. Chem. Rev. 93, 2395–2417.
Latgé, J. (2007). The cell wall: a carbohydrate armour for the fungal cell. Mol. Microbiol. 66, 279–290.
Mazzobre, M. F., Román, M. V., Mourelle, A. F., and Corti, H. R. (2005). Octanol–water partition coefficient of glucose, sucrose, and trehalose. Carbohydr. Res. 340, 1207–1211.
Merzendorfer, H. (2011). The cellular basis of chitin synthesis in fungi and insects: common principles and differences. Eur. J. Cell Biol. 90, 759–769.
Mohammadi, T., van Dam, V., Sijbrandi, R., Vernet, T., Zapun, A., Bouhss, A., Diepeveen-de Bruin, M., Nguyen-Distèche, M., de Kruijff, B., and Breukink, E. (2011). Identification of FtsW as a transporter of lipid-linked cell wall precursors across the membrane. EMBO J. 30, 1425–1432.
Mongrand, S., Stanislas, T., Baye, E. M. F., Lherminier, J., and Simon-Plas, F. (2010). Membrane rafts in plant cells. Trends Plant Sci. 15, 656–663.
Nühse, T. S., Stensballe, A., Jensen, O. N., and Peck, S. C. (2004). Phosphoproteomics of the Arabidopsis plasma membrane and a new phosphorylation site database. Plant Cell 16, 2394–2405.
Oglesby, L. L., Jain, S., and Ohman, D. E. (2008). Membrane topology and roles of Pseudomonas aeruginosa Alg8 and Alg44 in alginate polymerization. Microbiology 154, 1605–1615.
Peng, L., Kawagoe, Y., Hogan, P., and Delmer, D. (2002). Sitosterol-β-glucoside as primer for cellulose synthesis in plants. Science 295, 147–150.
Salnikov, V. V., Grimson, M. J., Delmer, D. P., and Haigler, C. H. (2000). Sucrose synthase localizes to cellulose synthesis sites in tracheary elements. Phytochemistry 57, 823–833.
Schulz, T., Schumacher, U., and Prehm, P. (2007). Hyaluronan export by the ABC transporter MRP5 and its modulation by intracellular cGMP. J. Biol. Chem. 282, 20999–21004.
Spiro, R. G. (2002). Protein glycosylation: nature, distribution, enzymatic formation, and disease implications of glycopeptide bonds. Glycobiology 12, 43R–56R
Reyes, F., and Orellana, A. (2008). Golgi transporters: opening the gate to cell wall polysaccharide biosynthesis. Curr. Opin. Plant Biol. 11, 244–251.
Taylor, N. G. (2007). Identification of cellulose synthase AtCesA7 (IRX3) in vivo phosphorylation sites – a potential role in regulating protein degradation. Plant Mol. Biol. 64, 161–714.
Tlapak-Simmons, V. L., Kempner, E. S., Baggenstoss, B. A., and Weigel, P. H. (1998). The active streptococcal hyaluronan synthases (HASs) contain a single HAS monomer and multiple cardiolipin molecules. J. Biol. Chem. 273, 26100–26109.
Urbanowicz, B. R., Rayon, C., and Carpita, N. C. (2004). Topology of the maize mixed linkage (1 → 3),(1 → 4)-β-D-glucan synthase at the Golgi membrane. Plant Physiol. 134, 758–768.
Verma, D. P. S., and Hong, Z. (2001). Plant callose synthase complexes. Plant Mol. Biol. 47, 693–701.
Wightman, R., and Turner, S. (2010). Trafficking of the plant cellulose synthase complex. Plant Physiol. 153, 427–432.
Keywords: glycosyltransferase, membrane transport, polysaccharide synthesis, protein topology, cell wall
Citation: Davis JK (2012) Combining polysaccharide biosynthesis and transport in a single enzyme: dual-function cell wall glycan synthases. Front. Plant Sci. 3:138. doi: 10.3389/fpls.2012.00138
Received: 27 March 2012; Accepted: 07 June 2012;
Published online: 22 June 2012.
Edited by:
Seth DeBolt, University of Kentucky, USAReviewed by:
Robert L. Houtz, University of Kentucky, USAAriel Orellana, Universidad Andres Bello, Chile
Michael A. Held, Ohio University, USA
Copyright: © 2012 Davis. This is an open-access article distributed under the terms of the Creative Commons Attribution Non Commercial License, which permits non-commercial use, distribution, and reproduction in other forums, provided the original authors and source are credited.
*Correspondence: Jonathan K. Davis, Department of Crop Science, North Carolina State University, 4402A Williams Hall, Campus Box 7620, Raleigh, NC 27695-7620, USA. e-mail: jkdavis2@ncsu.edu