- 1Department of Biology, School of Environmental and Life Sciences, The University of Newcastle, Callaghan, NSW, Australia
- 2Australia-China Research Centre for Crop Improvement, The University of Newcastle, Callaghan, NSW, Australia
Plant growth and development are modulated by concerted actions of a variety of signaling molecules. In recent years, evidence has emerged on the roles of sugar and auxin signals network in diverse aspects of plant growth and development. Here, based on recent progress of genetic analyses and gene expression profiling studies, we summarize the functional similarities, diversities, and their interactions of sugar and auxin signals in regulating two major processes of plant development: cell division and cell expansion. We focus on roles of sugar and auxin signaling in both vegetative and reproductive tissues including developing seed.
Introduction
Plant growth and development results from a combination of three processes at the cellular level: cell division, cell expansion, and cell differentiation. Cell division or mitosis involves the duplication and separation of complete sets of genetic materials. This genetic information is then selectively transcribed and translated to determine the final shape of cells through cell expansion and differentiation. Cell division and expansion determines cell number and cell size in a mature organ, hence its yield. Over the last decade, genetic analyses and genome-wide gene expression profiling studies have significantly advanced our understanding of the signaling pathways regulating cell proliferation and expansion. In this context, the phytohormone auxin plays prominent roles in regulating both cell proliferation and cell expansion (reviewed in Perrot-Rechenmann, 2010). Rapid advances in the area have helped shed light on the molecular mechanisms regulating auxin homeostasis, transport, and signaling.
Sugars, in addition to their fundamental roles as carbon and energy sources, also act as signaling molecules to regulate gene expression (e.g., Rolland et al., 2002; Hartig and Beck, 2006). The disaccharide sucrose (Suc) is transported through phloem from photosynthetic leaves (source) to sink organs such as root, meristem, flower, developing fruit, and seed. In general, lowered Suc levels stimulate source activities, including photosynthesis, nutrient mobilization, and export. In contrast, higher Suc levels is believed to inhibit photosynthesis in source leaves, but stimulate growth and storage in sink tissues. Phloem unloading of Suc in companion cell and sieve element (CC/SE) complex and its post-phloem transport to recipient sink cells may occur either apoplasmically into cell wall matrix or symplasmically through plasmodesmata. Prior to its use for metabolism and biosynthesis, Suc must be degraded either by invertase (Inv) into glucose (Glc) and fructose (Fru), or by Suc synthase (Sus) into UDP-glucose (UDPG) and Fru. Based on the subcellular localization, Inv is usually divided as cell wall Inv (cw-Inv), vacuolar Inv (vac-Inv), and cytosolic Inv (cyto-Inv; but it may also be expressed in mitochondria, plastids, and nuclei); while Sus may exist as soluble protein in cytoplasm (cyto-Sus) or insoluble isoform bound to the plasma membrane (PM-Sus; Cai et al., 2011) and other intracellular organelles (Ruan, 2012). These sucrolytic enzyme activities are not only critical for primary and secondary metabolism by supplying essential energy and building blocks for plant growth, but also play direct roles in signaling (Ruan, 2012), since Suc and its cleavage hexose are also signaling molecules regulating gene expression (reviewed by Gibson, 2005; Rolland et al., 2006; Eveland and Jackson, 2012). So far, the best studied plant sugar signaling pathway is Glc signaling, mediated by its sensor hexokinase (HXK), which sequentially regulates plant gene expression at transcriptional, translational, and post-translational levels (e.g., Sheen, 1990; Ho et al., 2001; Yanasigawa et al., 2003). In the past few years, a large number of sugar-responsive loci have been identified by genetic approaches, and different sugar-based signaling pathways are deciphered in specific developmental process (reviewed in Smeekens et al., 2010). Mounting evidence also suggests crosstalks among sugar and various hormone signals, e.g., abscisic acid, ethylene, and cytokinins (Gazzarrini and McCourt, 2001; Hartig and Beck, 2006). Among these, close interactions between sugar and auxin signaling play major roles in various aspects of plant development (Eveland and Jackson, 2012).
This review aims to evaluate recent progress on the regulatory roles of sugar and auxin signaling and their interactions in cell division and expansion from a developmental perspective in both vegetative and reproductive organs.
Auxin and Sugar Signaling in Cell Proliferation
The eukaryotic cell cycle consists of DNA synthesis (S phase) and mitosis (M phase), separated by two gap phases G1 and G2 (Figure 1). Mitogenic signals are required for completion of cell cycle, in particular during the transitions from G1 to S and G2 to M phases for proper progression of the cycle; otherwise the cell cycle will be arrested. Some plant cells may skip the M phase under certain developmental processes resulting in endoreduplication and an increase in the degree of ploidy (Figure 1; Joubés and Chevalier, 2000). A typical example of cell cycle without mitosis occurs in the syncytial endosperm early in seed development.
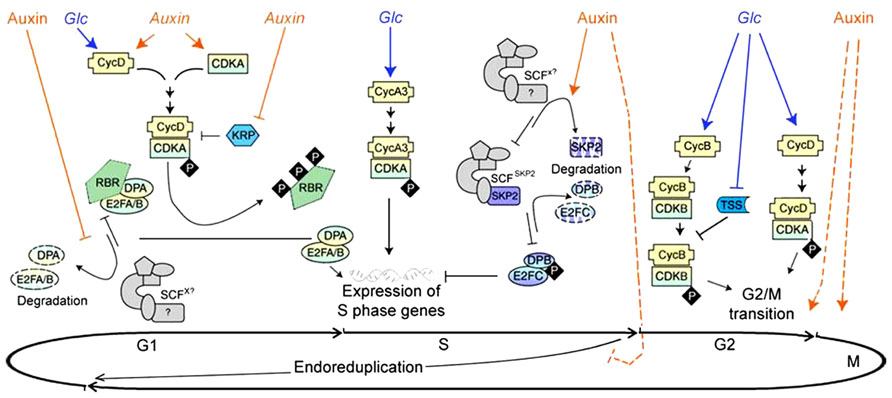
FIGURE 1. Glucose and auxin signal in cell cycle regulation (modified from Perrot-Rechenmann, 2010, Figure 2). The cell cycle is divided into four phases: DNA replication (S), mitosis (M), and two gap phases (G1 and G2, between M/S and S/M, respectively). Some plant cells may skip the M phase under certain developmental processes, resulting “endoreduplication.” Cell cycle starts in G1. During this phase, Glc and auxin signals could induce the expression of CycD, while auxin is also able to increase CDKA transcription. The CycD/CDKA complex is activated by phosphorylation but can still be blocked by CDK inhibitor KRPs. Auxin was reported to reduce the expression of some KRPs. The active CycD/CDKA complex provokes phosphorylation of the transcriptional repressor RBR, and release the transcription regulator E2FA/B and DPA complex. By post-transcriptional regulation, auxin stabilizes the E2FA/B and DPA complex, which promote the expression of genes essential for the beginning of the S phase. The expression of CycA3 could be up-regulated by Glc signal, which is required to drive the cells from G1 to S phase. Auxin was shown to increase the degradation of the F-box SKP2 later in S phase, which indirectly stabilizes E2FC/DPB complex, and represses the S phase genes expression. As cell cycle processes into G2 phase, Glc signal was found to initiate the G2/M transition by repressing TSS transcription, and activating the expression of key cell cycle genes, such as CycB and CycD. Auxin signal is required for the initiation and completion of mitosis, probably though an unknown pathway independent or in parallel to Glc. Auxin is also likely to emit a negative signal to prevent cell from going into endoreduplication hence sustaining cell divisions. Glc and Auxin in italic indicate regulation at transcription level and those in non-italic suggest regulation at protein level.
As a class of essential plant hormones, auxin has been demonstrated to play a leading role in regulating cell proliferation, especially in the preparation of replication (G1 to S phases). A set of in vitro cell culture studies have provided insights into the molecular mechanisms by which auxin regulates cell cycle (Figure 1; reviewed in Perrot-Rechenmann, 2010). During G1 phase, auxin was shown to induce the expression of cyclin D gene cycD3;1 and cyclin-dependent kinase gene CDKA;1, and to play important roles in CDKA/CYCD complex assembling. Meanwhile, KRP1 and KRP2 transcripts, encoding two of the CDK inhibitors, were reported to be down-regulated after auxin treatment, thereby preserving the phosphorylated CDKA/CYCD complex. Activated CDKA/CYCD complex could provoke phosphorylation of the transcriptional repressor retinoblastoma-related (RBR) protein, and release its target Adenovirus E2 promoter-binding factor A/B (E2FA/B) and dimerization partener A (DPA) complex. Through this post-transcriptional regulation, auxin stabilizes the E2FA/B and DPA complex, which promotes the expression of genes essential for initiating the S phase. Later in the S phase, auxin stimulates the degradation of the F-box SKP2A (S phase kinase-associated protein 2A) by E3 ubiquitin ligase complex SCF (Skp, Cullin, F-box containing complex), indirectly stabilizing the E2 promoter-binding factor C (E2FC) and dimerization parterner B (DPB) complex. The latter represses the expression of S phase genes. Although most data suggest auxin acts as a permissive signal for achieving competence to enter DNA synthesis (G1/S transition), it is also required in the later G2/M transition to complete the mitosis process (Figure 1; Urano et al., 2012). However, it is difficult to dissect the effect of auxin on later phases from that at the initial step of the cell cycle. Consistent with its role in expedition of cell cycle process, auxin was also found to promote cell division and delay endoreduplication in developing seeds of legume species Medicago truncatula (Figure 1; Atif et al., 2012).
Comparing to auxin, our current knowledge about the molecular mechanism of sugar-mediated regulation of cell division is largely derived from studies on cultured suspension cells and mutant seedlings subjected to various sugar treatments. A close correlation was observed between the supply of Glc and the expressions of cyclins, e.g., cycD2;1, D3;2, A3;2, and B1;2 (Figure 1; Riou-Khamlichi et al., 2000; Hartig and Beck, 2006). The D-type cyclins are often mentioned as sensors of external conditions, and associate with cyclin-dependent kinase (e.g., CDKA) to regulate cell cycles (Nieuwland et al., 2007). Meanwhile, A3 and B1 cyclins are required to drive G1/S and G2/M transitions, respectively (Menges et al., 2005). These observations suggest that Glc signaling regulates cell cycle throughout the whole cell cycle process. Noteworthy is that the regulatory effect of Glc on the rate of cell division primarily results from signaling rather than nutrient availability and energy status, as cell proliferating activity positively correlated with endogenous hexose levels, but not their uptake rate (Hartig and Beck, 2006). A recent study in Arabidopsis meristematic tissues has shown that Glc signal initiates the G2/M transition by repressing transcription of the negative regulator TPR-DOMAIN SUPPRESSOR OF STIMPY (TSS), thereby activating the expression of key cell cycle components required for G2/M transition, such as CYCB1;1 and CDKB1;1 (Figure 1; Skylar et al., 2011). Noteworthy is that Glc feeding is insufficient to trigger mitosis, and auxin is also required for the completion of this process, indicating distinct but coordinated roles of sugar and auxin in G2/M regulation (Skylar et al., 2011).
Other than hexose, downstream components of Suc/Glc signaling factors may also be involved in cell cycle regulation. Trehalose-6-phosphate (T6P) is a newly identified signal molecule which is synthesized from G6P and UDPG by T6P synthase (TPS; Paul et al., 2008). AtTPS1, being the only functional Arabidopsis TPS enzyme catalyzes T6P synthesis reaction, was observed interacting with CDKA1 and the kinesin KCA1, while its loss of function mutant tps1 shown embryo lethal (reviewed in Smeekens et al., 2010). The exact role of T6P in cell cycle remains unclear. However, a downstream target of T6P, Suc non-fermenting1 (Snf1)-related protein kinase (SnRK1), is considered to be a sensor negatively regulating plant growth through crosstalk with cell cycle signaling factors as indicated by studies of its homolog Snf1 in yeast (Francis and Halford, 2006). An inhibition of the catalytic activity of SnRK1 by T6P was revealed both in vitro and in vivo (see O’Hara et al., 2013). A link between T6P/SnRK1 regulatory system and auxin signaling has also been revealed (discussed later, reviewed in O’Hara et al., 2013), implying a potential molecular mechanism of T6P and SnRK1 signaling in regulating cell cycle. However, SnRK1 signal is currently associated with the response to starvation or low energy status during plant development, as its activity leads to down-regulation of carbon-consuming processes but enhancement of photosynthetic processes, thereby increasing carbon availability (Halford and Hey, 2009; O’Hara et al., 2013). It remains to be determined whether SnRK1 signal plays a role in cells division. Similarly, the perception of cellular energy and nutrient levels typically leads to the activation of the growth promoting target of rapamycin (TOR) protein kinase signaling pathway, which then adjusts cell growth and proliferation accordingly. It has been reported that TOR promotes Drosophila female germline stem cells proliferation during G2 phase (LaFever et al., 2010). A single copy of TOR has been found in Arabidopsis, the loss of which results in embryonic lethality (Menand et al., 2002). Whether the TOR complex functions in regulating plant cell division remains to be investigated.
Roles of Auxin and Sugar in Cell Expansion
Cell expansion is another important cellular process for plant growth, which is a net result of internal turgor pressure and irreversible cell wall extension. By accumulating sugars, irons and other osmotically active solutes, plant cell generates a lower osmotic potential to attract water flux into the cell, thereby generating a turgor pressure to drive cell expansion. This process also requires the cell wall to be irreversibly stretched through a wall loosening process, followed by deposition of new wall material. The extent and direction of cell expansion is modulated by many factors including cytoskeletons (Li et al., 2001; Pollard and Cooper, 2009).
Auxin was shown to induce rapid cell elongation in stem, coleoptiles, or hypocotyls segments within minutes after auxin treatment (Rayle and Cleland, 1992). Current model of auxin-regulated cell expansion is based on an acid growth theory (Figure 2; reviewed in Mockaitis and Estelle, 2008; Perrot-Rechenmann, 2010). An extracellular localized auxin is perceived by the auxin receptor, auxin binding protein 1 (ABP1). An interaction between the ABP1 and some unknown membrane-associated proteins may activate the plasma membrane (PM)-H+-ATPase, pumping proton into the extracellular space. This lowers the pH in cell wall matrix, activating cell wall loosening proteins such as expansins and xyloglucan endotransglycosylase/hydrolases (XTH), and consequently making the cell wall relaxed for expansion. The PM-H+-ATPase activity also promotes hyperpolarization of membrane potential which activates voltage-dependent potassium inward channels, thereby contributing to the osmotically driven water uptake for expansion.
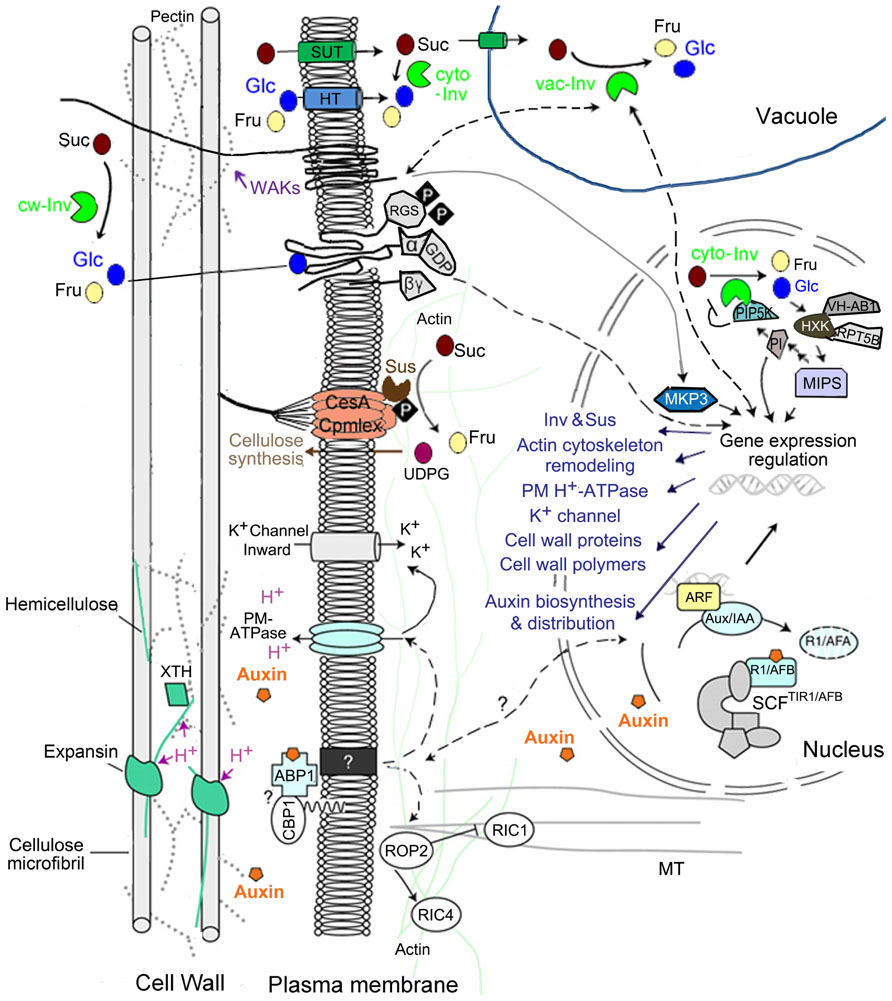
FIGURE 2. Sugar and auxin signal in regulating sink cell expansion (modified from Perrot-Rechenmann, 2010, Figure 3). Unloaded Suc in sink tissues may enter into recipient cells either apoplasmically through cell wall matrix or symplasmically via plasmodesmatal. In the former case, sucrose could be taken up by sucrose transporter on plasma membrane, or be hydrolyzed by cw-Inv into Glc and Fru, and then be transported into cells by hexose transporter (HT). Apoplasmic Glc could be recognized by RGS1, which transmits extracellular sugar signal into the cell through G-proteins. In cytoplasm, Suc may be hydrolyzed by cyto-Inv or degraded by Sus. In the present of high Suc level, Sus intends to bind actin filaments and form a multi-protein complex bound to plasma membrane, which may facilitate cell expansion by providing UDPG for cellulose/callose biosynthesis. Cytoplasmic Suc could also be transported into nucleus, vacuole, plastid or mitochondrion. In vacuole, Suc could be hydrolyzed by vac-Inv, thus doubling the osmotic contribution of Suc, which has the potential to positively impact on cell turgor. Moreover, vac-Inv could also promote cell expansion via sugar signaling involving WAKs, which subsequently activates MPK3 in nucleus, and induces downstream gene expression for cell wall biosynthesis. Suc hydrolysis in vacuole could also regulates nuclear gene transcription, involving in auxin biosynthesis, distribution and signaling. An Arabidopsis cyto-Inv isoform was found in nucleus, where it interacts with and negatively regulated by a phosphatidylinositol monophosphate 5-kinase (AtPIP5K9). Hexoses generated by cyto-Inv could be sensed by a nuclear-localized HXK, producing a Glc signaling complex core combining VHA-B1 and RPT5B, which is sequentially integrated into a signal/metabolites loop modulating cell expansion. Auxin is perceived by the auxin receptor ABP1, which interacts with unknown membrane-associated proteins at the plasma membrane [such as glycosylphosphatidylinositol (GPI)-anchored protein C-terminal peptide-binding protein 1. (CBP1)]. This activates the proton pump ATPase, acidifying extracellular space for optimal function of expansins and XTH and activating K+ inward rectifying channels, essential for water uptake to sustain cell expansion. Auxin could also enhance these effects by promoting the transcription of these genes. Moreover, auxin is likely to act on actin microfilaments and microtubules via the modulation of ROP GTPases, thereby affecting vesicle delivery to plasma membrane and cell wall matrix.
In parallel to its role in activating proteins essential for cell expansion at the post-translational level, auxin signal in the nuclei also enhances the transcription of these genes including those encoding PM-ATPase, K+ channels, expansins, and cell wall remodeling enzymes. Furthermore, auxin promotes exocytosis of vesicles containing new cell wall material (Figure 2; reviewed in Perrot-Rechenmann, 2010). In addition, auxin may affect F-actin through ABP1 and its downstream Rho GTPases and their effectors CRIB MOTIF-CONTAINING proteins (RICs), which consequently modulates asymmetric cell expansion (Figure 2; Yang and Fu, 2007; Xu et al., 2010).
Different from auxin which regulates cell growth solely through signaling, sugars modulate cell expansion both as important signals and metabolites. The latter serves as osmotically active solutes and substrates for biosynthesis of diverse products including cell wall material required for cell expansion.
By hydrolyzing Suc into Glc and Fru, vac-Inv doubles the osmotic contribution of Suc in vacuole, and thus has the potential to positively impact cell turgor in cells accumulating hexoses to high levels, such as elongating cotton fiber (Figure 2; Wang et al., 2010). Consistently, high vac-Inv expression or activity has been observed in a range of expanding tissues, for example, maize ovaries (Andersen et al., 2002), grape berry (Davies and Robinson, 1996), carrot taproot (Tang et al., 1999), and a reduction of maize ovary expansion was associated with the decrease of a vac-Inv gene Ivr2 expression under drought (Andersen et al., 2002).
In agreement with the idea that sugar could also act as signal for cell expansion, vac-Inv has been shown to promote cell expansion in Arabidopsis root through an osmotic-independent pathway (Wang et al., 2010). One possible explanation is that vac-Inv may crosstalk with wall-associated kinases (WAKs) through sugar signaling to regulate cell wall extensibility (Figure 2). Here, as receptor-like proteins, WAKs are bound to pectin in cell walls, and their activity is required for cell expansion (Anderson et al., 2001; Lally et al., 2001). The observations that vac-Inv activity and AtvacINV2 transcription were dramatically reduced in Arabidopsis wak2-1 mutant (Kohorn et al., 2006), and the deletion of AtvacINV2 (vin, Salk_100813) down-regulated the expression of AtWAK2 (Wang and Ruan, unpublished data), strongly suggest an interplay between vacuole sugar homeostasis and extracellular matrix signaling during cell expansion. Microarray analysis revealed a WAK2-dependent pectin activation of many genes involved in cell wall biosynthesis, which is likely achieved via a downstream mitogen-activated protein kinase AtMAPK3 (Figure 2; Kohorn et al., 2009; Kohorn and Kohorn, 2012). This, together with our finding on repression of WAK expression in vin mutant also implies that a sugar signal derived from vacuole could play roles in transcription of genes required for cell expansion. Indeed, Suc hydrolysis in vacuole was able to evoke a sugar signal effect on numerous gene expressions, involving auxin biosynthesis, distribution, and auxin signal sensing (Mishra et al., 2009; discussed below). Suggested by the finding that vacuolar H+-ATPase B1 unit (VHA-B1) interacts with the nuclear-localized AtHXK1 (Cho et al., 2006), a similar protein complex on tonoplast in transmitting vacuolar sugar signals might be expected. However, it remains to be determined as to what is the exact downstream signal pathway of this vacuole sugar signal, and how vac-Inv interacts with cell wall protein WAKs to regulate cell expansion.
Other than vac-Inv, cyto-Inv was also described to play a role in regulating root cell elongation in Arabidopsis and rice (Lou et al., 2007; Jia et al., 2008). Interestingly, the Arabidopsis cyto-Inv isoform AtCIN1 (At1g35580) was observed in nuclei, where it interacts with a phosphatidylinositol monophosphate 5-kinase (AtPIP5K9), and is negatively regulated by AtPIP5K9 (Figure 2; Lou et al., 2007). AtPIP5K is a key enzyme in phosphatidylinositol (PI) signaling pathway, and may directly or indirectly regulate cytoskeleton dynamics via myo-inositol (Lou et al., 2007). Meanwhile, the hexoses derived from nuclear Suc degradation catalyzed by AtCIN1, may be sensed by nuclear-localized AtHXK1 (Cho et al., 2006), and sequentially modulates transcriptions of specific target genes (Figure 2). However, the biological functions of the nuclear-localized AtCIN1 and AtHXK1 protein complex are still unclear.
In contrast to Inv, the second Suc-degrading enzyme, Sus, contributes to cell expansion primarily through providing one of the cleavage reaction products, UDPG for cell wall biosynthesis. For example, Sus is highly expressed in cotton cellularizing endosperm cells (Ruan et al., 2008) and seed coat transfer cell undergoing wall in growth (Pugh et al., 2010). Recent studies in Arabidopsis and tobacco support a model of PM-Sus-mediated cell wall biosynthesis where PM-Sus binds to actin filaments to initiate the formation of a multi-protein complex and to provide UDPG to callose synthase and cellulose synthase, thus facilitating cell expansion via cellulose/callose biosynthesis (Figure 2; Fujii et al., 2010; Cai et al., 2011). Interestingly, a conversion from the cytosolic Sus to its membrane-associated form was detected in the presence of high Suc level, indicating a role of Suc homeostasis in cell wall deposition (Cai et al., 2011). Recently, during Arabidopsis seed development, hexose signaling was observed to induce the expression of Sus genes via a HXK-independent pathway (Angeles-Núñez and Tiessen, 2012). Together, these results show that sugars may regulate Sus-mediated cell wall biosynthesis through cellular metabolism as well as signaling network.
Research on sugar signaling has been primarily focused on intracellular processes. It remains virtually unknown how cell senses extracellular signals such as sugars to elicit downstream cellular processes. Ruan et al. (2009) proposed that the apoplasmic Glc generated by cw-Inv could be recognized by a membrane protein, RGS1 (regulator of G-protein signaling 1), which transmits extracellular sugar signal into the cell (Figure 2). An insight into the molecular basis of sugar and G-protein signaling crosstalk comes from a recent discovery by Urano et al. (2012) in which Glc could be sensed by AtRGS1 that represses the activity of heterotrimeric G-protein complex. This Glc sensing leads to endocytosis of AtRGS1, hence uncoupling the inhibitory effect of AtRGS1 on AtGPA1 (G-protein α subunit) and consequently activating G-protein signaling (Urano et al., 2012).
Other than G-protein, HXK could also be a potential integrator in regulating cell expansion through Glc signaling, probably via modulating cytoskeleton dynamics (Karve et al., 2008; see Figure 2). AtHXK1 was shown to affect F-actin dynamics, thereby influencing the formation and the stability of cytoskeleton-bound polysomes, and the complex membrane trafficking involved in expansion (Balasubramanian et al., 2007). In addition, Cho et al. (2006) suggested a nuclear Glc signaling core formed by nuclear-localized AtHXK1 interacts with VHA-B1 and the 19S regulatory particle of proteasome subunit (RPT5B). Arabidopsis VHA-Bs are involved in actin cytoskeleton remodeling via binding and stabilizing F-actin in vitro (Ma et al., 2012), thereby potentially affecting cell expansion through modulating actin-guided vesicle trafficking for cell wall synthesis.
Together, the above analyses allow us to formulate a model of cell expansion regulated by sugar and auxin signaling network, covering WAKs, G-protein and nuclear PI signaling, and linking transmission of signals from extracellular environment to different subcellular compartments for a range of cellular processes required for cell enlargement (Figure 2).
The Interplay of Sugar and Auxin Signaling Pathways
As discussed above, sugar and auxin signals play distinctive roles in regulating cell division and expansion. However, the two pathways also interact with each other to regulate plant development and recent studies indeed show that auxin biosynthesis, distribution, and response is regulated directly by sugar signal.
Several biosynthesis pathways of the main auxin, IAA (indole-3-acetic acid) have been postulated in plant. These include the widely distributed IAM (indole-3-acetamide) pathway, the IPA (indole-3-pyruvic acid) pathway, and two possible Brassicaceae species-specific pathways using IAOX (indole-3-acetaldoxime) and IAN (indole-3-acetonitrile) as intermediates (Mano and Nemoto, 2012). All the above pathways synthesize TRP (L-tryptophan) as a precursor (Mano and Nemoto, 2012). Importantly, Glc increases the concentrations of many IAA precursors related to IAM, IAOX, and IAN pathways, as well as three major IAA metabolites and conjugates (oxIAA, IAAsp, and IAGlu), demonstrating a direct positive regulation of Glc in auxin synthesis (Sairanen et al., 2012). In vivo evidence consistent with this finding comes from Arabidopsis hypocotyls, where an endogenous carbon-sensing pathway triggers increased auxin flux and hypocotyl elongation (Lilley et al., 2012).Another important founding by Sairanen et al. (2012) is that the Glc-induced auxin synthesis is negatively regulated by the PHYTOCHROME-INTERACTING FACTOR (PIF) transcription factor family. Considering the up-regulation of PIF gene expression by Suc (Stewart et al., 2011), it is possible that PIF protein act as a switch-off button in Glc induction of auxin biosynthesis at high Suc level or by some specific developmental cues. Further evidence on the positive role of Glc in auxin biosynthesis comes from the maize miniature1 mutant which lacks the expression of basal kernel-specific cw-Inv (INCW2), leading to miniature seed phenotype. In the mutant seed, the IPA auxin synthesis pathway was down-regulated through decreased expression of a maize YUCCA gene (LeClere et al., 2010). YUCCA encodes a flavin monooxygenase-like enzyme, which uses IPA as a substrate to produce IAA. This observation suggests that Inv-mediated generation of hexoses is required for auxin biosynthesis in developing seed.
In addition to its role in synthesis, Glc is also implicated in auxin distribution and signaling. To this end, microarray analysis revealed that Glc could regulate as much as 62% of IAA related genes in Arabidopsis seedlings, including those encoding auxin receptors TIR1 (transport inhibitor response 1) and ABP1, auxin transporter PIN1, auxin response factors ARF4, ARF8, and a number of genes belonging to auxin induced gene families such as AUX/IAA, GH3, and SAUR (Mishra et al., 2009). Studies using Glc-insensitive mutants also revealed that hexose-mediated sugar signaling partially functions through auxin response (Moore et al., 2003). Genetic studies showed that Suc and Glc stabilize N-MYC DOWN-REGULATED-LIKE1 (NDL1) protein, which interacts with Gβγ dimmers of heterotrimeric G-protein complex, thereby positively regulating auxin transport in root to promote lateral root initiation and emergence (Mudgil et al., 2009). During Arabidopsis embryogenesis, nuclear PI signaling was involved in regulating polar transport of auxin effluxer PIN1 via modulating endomembrane structure and trafficking (Luo et al., 2011). Given the importance of hexose substrate for PIs biosynthesis (see previous discussions), this observation implies a possible link of Glc-mediated auxin transport through PI signaling.
Apart from Glc, emerging evidence also indicates connections between the T6P/SnRK1 regulatory system and auxin signaling. For example, microarray analysis in Arabidopsis seedlings has demonstrated a down-regulation of AUX/IAA genes and auxin receptor gene TIR1 by elevated T6P level (Paul et al., 2008). SnRK1 has been shown to interact with the SKP1 domain of the SCF complex and the 26S proteasome, possibly through phosphorylating targeted proteins such as AUX/IAA for degradation in SCF–TIR1 complex (Farras et al., 2001).
Most of the studies described above on sugar and auxin signaling were conducted in cell culture systems and vegetative tissues such as developing roots, hypocotyls, leaves, and young seedlings. Reproductive organs are equally, or even more complex than, vegetative tissues in response to sugar and hormonal signals (Ruan et al., 2012). We discussed this issue below by focusing on recent advance obtained from developing seed.
Roles of Sugar and Auxin Signaling in Seed Development
Angiosperm seeds originate from double fertilization, which give birth to the diploid embryo and the triploid endosperm, wrapped by the maternal tissue known as seed coat. Seed formation proceeds by a phase of cell division, which represents a crucial period for seed set, highly sensitive to biotic and abiotic stress, and impacting significantly on seed yield potential (Ruan et al., 2012).
During seed development, the endosperm mother cell initially undergoes nuclear divisions without cell wall formation to generate syncytial endosperm soon after fertilization (Olsen, 2004). Endosperm nuclear division occurs earlier and faster than the embryo cell proliferation (Bate et al., 2004; Nowack et al., 2007). This sequential endosperm and embryo proliferation are tightly coupled to different regulatory mechanisms, for example, sugar signaling. Recent findings on the asymmetric spatial expression of cw-Inv gene GhCWIN1 in cotton embryo sac, implied a control of the sequential development of endosperm and embryo by Inv-mediated sugar signaling. This may be achieved through establishing a spatial gradient of Glc concentration being higher in the endosperm region than that in the embryo region, thus favoring endosperm nuclear division over embryo cell proliferation during the seed set phase (Wang and Ruan, 2012). Consistently, a cw-Inv inhibitor was localized at the boundary between the endosperm and embryo in developing maize seed (Bate et al., 2004), which could help to minimize hexose flow to young embryo, to ensure nuclear division in endosperm but a quiescent status in embryo at this stage.
Later, embryo develops starting from the acquisition of zygote polarity and elongation, follows by a serial of cell division. Soon after the asymmetric zygote division, the separated cells quickly establish an apical–basal axis of polarity, then the differentiation of an epidermis and the formation of the shoot and root meristem during the next rounds of cell division (Dumas and Rogowsky, 2008). Auxin plays a prominent role in regulating these pattern formations in a cell type-dependent manner (Rademacher et al., 2012). The molecular mechanisms of auxin action in early embryogenesis have been reviewed (e.g., Möller and Weijers, 2009; Lau et al., 2012), and will not be discussed here. Comparing to auxin, it remains unknown whether sugar signaling affects embryo pattern formation. However, significant correlations between Glc concentration and CWIN expression and early embryo (pre-heart stage) mitotic activity have been revealed in many species, such as Arabidopsis, faba bean, and cotton (Weber et al., 1996; Morley-Smith et al., 2008; Wang and Ruan, 2012), indicating Glc as a signal to stimulate cytokinesis during embryogenesis. Apart from Glc, T6P signaling may also affect mitosis activity in early embryo, as Arabidopsis TPS1-deficient mutant (tps1) shown slower embryo development compare to that of wild-type (Gómez et al., 2010). However, tps1 embryos are eventually stopped at torpedo stage, implying T6P signal may not essential for rapid cell division in early development, but indispensable for the transition into late stage of embryo development (Gómez et al., 2010).
As embryo develops to heart-torpedo stages, cells undergo rapid expansion, and gradually start to accumulate storage materials. As discussed before, cell expansion could be regulated by the concerted actions of sugar and auxin signaling network. Many studies have shown the impacts of sugar and auxin on seed size (e.g., Cheng and Chourey, 1999; Andersen et al., 2002; Schruff et al., 2006). However, most of the observations are not able to differentiate signaling roles of sugar from its potential nutrient or osmotic effect. The contribution of auxin signal to seed size is largely due to its roles in cell division but not expansion. There is little direct evidence about the role of sugar and auxin signaling in cell enlargement so far, probably due to, in part, the complexity of cell types in seed.
By contrast, many studies have demonstrated that the switch from seed expansion to storage phase is strongly affected by sugar signals. A general finding is that the transition from cell division and expansion to storage activities in seed is usually associated with a decrease in Inv expression and activity and an increase in Sus activity, and an increased ratio of Suc to Glc. Using high-resolution histographical mapping, a clear correlation was observed between decreased Glc concentration and reduced mitotic activity in legume embryo (Borisjuk et al., 1998), largely due to the decrease of cw-Inv genes expression (Weber et al., 1995). In cytoplasm, an increase of Sus activity leads a shift in carbon use toward starch synthesis in plastid. For example, highest expression of Sus gene temporally matches with rapid starch filling in rice grain (Wang et al., 1999). In correlation to Suc level, T6P synthesis could be induced, which may then result in an increased expression and activity of the ADP-glucose pyrophosphorylase (AGPase), as well as the redox activation of AGPase, essential for starch accumulation in the plastid (O’Hara et al., 2013).
Clearly, much has been learned about the complexity of sugar and auxin network in regulating plant cell division and expansion. Further research on dissecting the complex network of sugar and auxin signaling pathways in specific plant tissues under particular growth stages or environment conditions, will not only contribute to a better understanding of the regulatory systems underpinning complex developmental processes, but could also have great importance in crop improvement through designing innovative approaches to optimize plant performance for yield and stress tolerance.
Conflict of Interest Statement
The authors declare that the research was conducted in the absence of any commercial or financial relationships that could be construed as a potential conflict of interest.
References
Andersen, M. N., Asch, F. A., Wu, Y., Jensen, C. R., Næsted, H., Mogensen, V. O., et al. (2002). Soluble invertase expression is an early target of stress during the critical, abortion sensitive phase of young ovary development in maize. Plant Physiol. 130, 591–604. doi: 10.1104/pp.005637
Anderson, C. M., Wagner, T. A., Perret, M., He, Z. H., He, D., and Kohorn, B. D. (2001). WAKs: cell wall-associated kinases linking the cytoplasm to the extracellular matrix. Plant Mol. Biol. 47, 197–206. doi: 10.1023/A:1010691701578
Angeles-Núñez, J. G., and Tiessen, A. (2012). Regulation of AtSUS2 and AtSUS3 by glucose and the transcription factor LEC2 in different tissues and at different stages of Arabidopsis seed development. Plant Mol. Biol. 78, 377–392. doi: 10.1007/s11103-011-9871-0
Atif, R. M., Boulisset, F., Conreux, C., Thompson, R., and Ochatt, S. J. (2012). In vitro auxin treatment promotes cell division and delays endoreduplication in developing seeds of the model legume species Medicago truncatula. Physiol. Plant. doi: 10.1111/j.1399-3054.2012.01719.x [Epub ahead of print].
Balasubramanian, R., Karve, A., Kandasamy, M., Meagher, R. B., and Moore, B. D. (2007). A role for F-actin in hexokinase-mediated glucose signaling. Plant Physiol. 145, 1423–1434. doi: 10.1104/pp.107.108704
Bate, N. J., Niu, X., Wang, Y., Reimann, K. S., and Helentharis, T. G. (2004). An invertase inhibitor from maize localizes to the embryo surrounding region during early kernel development. Plant Physiol. 134, 246–154. doi: 10.1104/ pp.103.027466
Borisjuk, L., Walenta, S., Weber, H., Mueller-Klieser, W., and Wobus, U. (1998). High-resolution histographical mapping of glucose concentrations in developing cotyledons of Vicia faba in relation to mitotic activity and storage processes: glucose as a possible developmental trigger. Plant J. 15, 583–591. doi: 10.1046/j.1365-313X.1998.00214.x
Cai, G., Faleri, C., Del Casino, C., Emons, A. M., and Cresti, M. (2011). Distribution of callose synthase, cellulose synthase, and sucrose synthase in tobacco pollen tube is controlled in dissimilar ways by actin filaments and microtubules. Plant Physiol. 155, 1169–1190. doi: 10.1104/pp.110.171371
Cheng, W. H., and Chourey, P. S. (1999). Genetic evidence that invertase mediated released of hexoses is critical for appropriate carbon partitioning and normal seed development in maize. Theor. Appl. Genet. 98, 485–495. doi: 10.1007/s001220051096
Cho, Y. H., Yoo, S. D., and Sheen, J. (2006). Regulatory functions of nuclear hexokinase 1 complex in glucose signaling. Cell 127, 579–589. doi: 10.1016/j.cell.2006.09.028
Davies, C., and Robinson, S. P. (1996). Cloning of two putative vacuolar invertase cDNAs and their expression in grapevine tissues. Plant Physiol. 111, 275–283. doi: 10.1104/pp.111.1.275
Dumas, C., and Rogowsky, P. (2008). Fertilization and early seed formation. C.R. Biol. 331, 715–725. doi: 10.1016/j.crvi.2008.07.013
Eveland, A. L., and Jackson, D. P. (2012). Sugar, signaling, and plant development. J. Exp. Bot. 63, 3367–3377. doi: 10.1093/jxb/err379
Farras, R., Ferrando, A., Jasik, J., Kleinow, T., Okresz, L., Tiburcio, A., et al. (2001). SKP1–SnRK protein kinase interactions mediate proteasomal binding of a plant SCF ubiquitin ligase. EMBO J. 20, 2742–2756. doi: 10.1093/emboj/20.11.2742
Francis, D., and Halford, G. (2006). Nutrient sensing in plant meristems. Plant Mol. Biol. 60, 981–993. doi: 10.1007/s11103-005-5749-3
Fujii, S., Hayashi, T., and Mizuno, K. (2010). Sucrose synthase is an integral component of the cellulose synthesis machinery. Plant Cell Physiol. 51, 294–301. doi: 10.1093/pcp/pcp190
Gazzarrini, S., and McCourt, P. (2001). Genetic interaction between ABA, ethylene and sugar signaling pathways. Curr. Opin. Plant Biol. 4, 387–391. doi: 10.1016/S1369-5266(00)00190-4
Gibson, S. I. (2005). Control of plant development and gene expression by sugar signaling. Curr. Opin. Plant Biol. 8, 93–102. doi: 10.1016/j.pbi.2004.11.003
Gómez, L. D., Gilday, A., Feil, R., Lunn, J. E., and Graham, I. A. (2010). AtTPS1-mediated trehalose 6-phosphate synthesis is essential for embryogenic and vegetative growth and responsiveness to ABA in germinating seeds and stomatal guard cells. Plant J. 64, 1–13. doi: 10.1111/j.1365-313X.2010.04312.x.
Halford, N. G., and Hey, S. J. (2009). Snf1-related protein kinases (SnRKs) act within an intricate network that links metabolic and stress signaling in plants. Biochem. J. 419, 247–259. doi: 10.1042/BJ20082408
Hartig, K., and Beck, E. (2006). Crosstalk between auxin, cytokinins, and sugars in the plant cell cycle. Plant Biol. 8, 389–396. doi: 10.1055/s-2006-923797
Ho, S. L., Chao, Y. C., Tong, W. F., and Yu, S. M. (2001). Sugar coordinately and differentially regulates growth and stress-related gene expression via a complex signal transduction network and multiple control mechanisms. Plant Physiol. 125, 877–890. doi: 10.1104/ pp.125.2.877
Jia, L., Zhang, B., Mao, C., Li, J., Wu, Y., Wu, P., et al. (2008). OsCYT-INV1 for alkaline/neutral invertase is involved in root cell development and reproductivity in rice (Oryza sativa L.). Planta 228, 51–59. doi: 10.1007/s00425-008-0718-0
Joubés, J., and Chevalier, C. (2000). Endoreduplication in higher plants. Plant Mol. Biol. 43, 735–745. doi: 10.1023/A:1006446417196
Karve, A., Rauh, B. L., Xia, X., Kandasamy, M., Meagher, R. B., Sheen, J., et al. (2008). Expression and evolutionary features of the hexokinase gene family in Arabidopsis. Planta 228, 411–425. doi: 10.1007/s00425-008-0746-9
Kohorn, B. D., Johansen, S., Shishido, A., Todorova, T., Martinez, R., Defeo, E., et al. (2009). Pectin activation of MAP kinase and gene expression is WAK2 dependent. Plant J. 60, 974–982. doi: 10.1111/j.1365-313X.2009.04016.x
Kohorn, B. D., Kobayashi, M., Johansen, S., Riese, J., Huang, L. F., Koch, K., et al. (2006). An Arabidopsis cell wall-associated kinase required for invertase activity and cell growth. Plant J. 46, 307–316. doi: 10.1111/j.1365-313X.2006.02695.x
Kohorn, B. D., and Kohorn, S. L. (2012). The cell wall-associated kinases, WAKs, as pectin receptors. Front. Plant Sci. 3:88. doi: 10.3389/fpls.2012.00088
LaFever, L., Feoktistov, A., Hsu, H. J., and Drummond-Barbosa, D. (2010). Specific roles of target of rapamycin in the control of stem cells and their progeny in the Drosophila ovary. Development 137, 2117–2126. doi: 10.1242/dev.050351
Lally, D., Ingmire, P., Tong, H. Y., and He, Z. H. (2001). Antisense expression of a cell wall-associated protein kinase, WAK4, inhibits cell elongation and alters morphology. Plant Cell 13, 1317–1331.
Lau, S., Slane, D., Herud, O., Kong, J., and Jürgens, G. (2012). Early embryogenesis in flowering plants: setting up the basic body pattern. Annu. Rev. Plant Biol. 63, 6.1–6.24. doi: 10.1146/annurev-arplant-042811-105507
LeClere, S., Schmelz, E. A., and Chourey, P. S. (2010). Sugar levels regulate tryptophan-dependent auxin biosynthesis in developing maize kernels. Plant Physiol. 153, 306–318. doi: 10.1104/pp.110.155226
Li, Y., Zee, S. Y., Liu, Y. M., Huang, B. Q., and Yen, L. F. (2001). Circular F-actin bundles and a G-actin gradient in pollen and pollen tubes of Lilium davidii. Planta 213, 722–730. doi: 10.1007/s004250100543
Lilley, J. L., Gee, C. W., Sairanen, I., Ljung, K., and Nembhauser, J. L. (2012). An endogenous carbon-sensing pathway triggers increased auxin flux and hypocotyl elongation. Plant Physiol. 160, 2261–2270. doi: 10.1104/pp.112.205575
Lou, Y., Gou, J. Y., and Xue, H. W. (2007). PIP5K9, an Arabidopsis phosphatidylinositol monophosphate kinase, interacts with a cytoplast invertase to negatively regulate sugar-mediated root growth. Plant Cell 19, 163–181. doi: 10.1105/ tpc.106.045658
Luo, Y., Qin, G., Zhang, J., Liang, Y., Song, Y., Zhao, M., et al. (2011). D-myo-inositol-3-phosphate affects phosphatidylinositol-mediated endomembrane function in Arabidopsis and is essential for auxin-regulated embryogenesis. Plant Cell 23, 1352–1372. doi: 10.1105/tpc.111.083337
Ma, B., Qian, D., Nan, Q., Tan, C., An, L., and Xiang, Y. (2012). Arabidopsis vacuolar H+-ATPase (V-ATPase) B subunits are involved in actin cytoskeleton remodeling via binding to, bundling, and stabilizing F-actin. J. Biol. Chem. 287, 19008–19017. doi: 10.1074/jbc.M111.281873
Mano, Y., and Nemoto, K. (2012). The pathway of auxin biosynthesis in plants. J. Exp. Bot. 63, 2853–2872. doi: 10.1093/jxb/ers091
Menand, B., Desnos, T., Nussaume, L., Berger, F., Bouchez, D., Meyer, C., et al. (2002). Expression and disruption of the Arabidopsis TOR (target of rapamycin) gene. Proc. Natl. Acad. Sci. U.S.A. 99, 6422–6427. doi: 10.1073/pnas.092141899
Menges, M., de Jager, S. M., Gruissem, W., and Murray, J. A. (2005). Global analysis of the core cell cycle regulators of Arabidopsis identifies novel genes, reveals multiple and highly specific profiles of expression and provides a coherent model for plant cell cycle control. Plant J. 41, 546–566. doi: 10.1111/j.1365-313X.2004.02319.x
Mishra, B. S., Singh, M., Aggrawal, P., and Laxmi, A. (2009). Glucose and auxin signaling interaction in controlling Arabidopsis thaliana seedlings root growth and development. PLoS ONE 4:e4502. doi: 10.1371/ journal.pone.0004502
Mockaitis, K., and Estelle, M. (2008). Auxin receptors and plant development: a new signaling paradigm. Annu. Rev. Cell Dev. Biol. 24, 55–80. doi: 10.1146/annurev.cellbio.23.090506.123214
Möller, B., and Weijers, D. (2009). Auxin control of embryo patterning. Cold Spring Harb. Perspect. Biol. 1, a001545. doi: 10.1101/cshperspect.a001545
Moore, B., Zhou, L., Rolland, F., Hall, Q., Cheng, W. H., Liu, Y. X., et al. (2003). Roles of the Arabidopsis glucose sensor HXK1 in nutrient, light, and hormonal signaling. Science 300, 332–336. doi: 10.1126/science.1080585
Morley-Smith, E. R., Pike, M. J., Findlay, K., Kockenberger, W., Hill, L. M., Smith, A. M., et al. (2008). The transport of sugars to developing embryos is not via the bulk endosperm in oilseed rape seeds. Plant Physiol. 147, 2121–2130. doi: 10.1104/pp.108.124644
Mudgil, Y., Uhrig, J. F., Zhou, J., Temple, B., Jiang, K., and Jones, A. M. (2009). Arabidopsis N-MYC DOWNREGULATED-LIKE1, a positive regulator of auxin transport in a G protein-mediated pathway. Plant Cell 21, 3591–3609. doi: 10.1105/tpc.109.065557
Nieuwland, J., Menges, M., and Murray, J. A. H. (2007). “The plant cyclins,” in Cell Cycle Control and Plant Development, ed. D. Inze (Oxford: Blackwell Publishing), 31–61. doi: 10.1002/9780470988923.ch2
Nowack, M. K., Shirzadi, R., Dissmeyer, N., Dolf, A., Endl, E., Grini, P. E., et al. (2007). Bypassing genomic imprinting allows seed development. Nature 447, 312–315. doi: 10.1038/nature05770
O’Hara, L. E., Paul, M. J., and Wingler, A. (2013). How do sugars regulate plant growth and development? New insight into the role of trehalose-6-phosphate. Mol. Plant 6, 261–274. doi: 10.1093/mp/sss120
Olsen, O.-A. (2004). Nuclear endosperm development in Cereals and Arabidopsis thaliana. Plant Cell 16(Suppl. 1), S214–227. doi: 10.1105/tpc.017111
Paul, M. J., Primavesi, L. F., Jhurreea, D., and Zhang, Y. (2008). Trehalose metabolism and signaling. Annu. Rev. Plant Biol. 59, 417–441. doi: 10.1146/annurev.arplant.59.032607.092945
Perrot-Rechenmann, C. (2010). Cellular responses to auxin: division versus expansion. Cold Spring Harb. Perspect. Biol. 2, a001446. doi: 10.1101/cshperspect.a001446
Pollard, T. D., and Cooper, J. A. (2009). Actin, a central player in cell shape and movement. Science 326, 1208–1212. doi: 10.1126/science.1175862
Pugh, D. A., Offler, C. E., Talbot, M. J., and Ruan, Y. L. (2010). Evidence for the role of transfer cells in the evolutionary increase in seed and fiber biomass yield in cotton. Mol. Plant 3, 1075–1086. doi: 10.1093/mp/ssq054
Rademacher, E. H., Lokerse, A. S., Schlereth, A., Llavata-Peris, C. I., Bayer, M., Kientz, M., et al. (2012). Different auxin response machineries control distinct cell fates in the early plant embryo. Dev. Cell 22, 211–222. doi: 10.1016/ j.devcel.2011. 10.026
Rayle, D. L., and Cleland, R. E. (1992). The acid growth theory of auxin-induced cell elongation is alive and well. Plant Physiol. 99, 1271–1274. doi: 10.1104/pp.99.4.1271
Riou-Khamlichi, C., Menges, M., Healy, J. M., and Murray, J. A. (2000). Sugar control of the plant cell cycle: differential regulation of Arabidopsis D-type cyclin gene expression. Mol. Cell. Biol. 20, 4513–4521. doi: 10.1128/MCB.20.13.4513-4521.2000
Rolland, F., Baena-Gonzalez, E., and Sheen, J. (2006). Sugar sensing and signaling in plant: conserved and novel mechanisms. Annu. Rev. Plant Biol. 57, 675–709. doi: 10.1146/annurev.arplant.57.032905.105441
Rolland, F., Moore, B., and Sheen, J. (2002). Sugar sensing and signaling in plants. Plant Cell 14, S185–S205. doi: 10.1105/tpc.010455
Ruan, Y.-L. (2012). Signaling roles of sucrose metabolism in plant development Mol. Plant 5, 763–765. doi: 10.1093/mp/sss046
Ruan, Y.-L., Jin, Y., and Huang, J. (2009). Capping invertase activity by its inhibitor: roles and implications in sugar signaling, carbon allocation, senescence and evolution. Plant Signal. Behav. 4, 983–986. doi: 10.4161/psb.4.10.9667
Ruan, Y.-L., Llewellyn, D. J., Liu, Q., Xu, S. M., Wu, L. M., Wang, L., et al. (2008). Expression of sucrose synthase in the developing endosperm is essential for early seed development in cotton. Funct. Plant Biol. 35, 382–393. doi: 10.1071/FP08017
Ruan, Y. L., Patrick, J. W., Bouzayen, M., Osorio, S., and Fernie, A. R. (2012). Molecular regulation of seed and fruit set. Trends Plant Sci. 17, 656–665. doi: 10.1016/j.tplants.2012.06.005
Sairanen, I., Novák, O., Pencïk, A., Ikeda, Y., Jones, B., Sandberg, G., et al. (2012). Soluble carbohydrates regulate auxin biosynthesis via PIF proteins in Arabidopsis. Plant Cell 24, 4907–4916. doi: 10.1105/tpc.112.104794
Schruff, M. C., Spielman, M., Tiwari, S., Adams, S., Fenby, N., and Scott, R. J. (2006). The AUXIN RESPONSE FACTOR 2 gene of Arabidopsis links auxin signalling, cell division, and the size of seeds and other organs. Development 133, 251–261. doi: 10.1242/dev.02194
Sheen, J. (1990). Metabolic repression of transcription in higher plants. Plant Cell 2, 1027–1038. doi: 10.1105/tpc.2.10.1027
Skylar, A., Sung, F., Hong, F., Chory, J., and Wu, X. (2011). Metabolic sugar signal promotes Arabidopsis meristematic proliferation via G2. Dev. Biol. 351, 82–89. doi: 10.1016/j.ydbio.2010.12.019
Smeekens, S., Ma, J., and Rolland, F. (2010). Sugar signals and molecular networks controlling plant growth. Curr. Opin. Plant Biol. 13, 177–191. doi: 10.1016/ j.pbi.2009.12.002
Stewart, J. L., Maloof, J. N., and Nemhauser, J. L. (2011). PIF genes mediate the effect of sucrose on seedling growth dynamics. PLoS Genet. 8:e1002594. doi: 10.1371/journal.pone.0019894
Tang, G. Q., Luscher, M., and Sturm, A. (1999). Antisense repression of vacuolar and cell wall invertase in transgenic carrot alters early plant development and sucrose partitioning. Plant Cell 11, 177–189. doi: 10.1105/tpc.11.2.177
Urano, D., Phan, N., Jones, J. C., Yang, J., Huang, J. R., Grigston, J., et al. (2012). Endocytosis of the seven-transmembrane RGS1 protein activates G-protein-coupled signalling in Arabidopsis. Nat. Cell Biol. 14, 1079–1088. doi: 10.1038/ncb2568
Wang, A.-Y., Kao, M.-H., Yang, W.-H., Sayion, Y., Liu, L.-F., Lee, P.-D., et al. (1999). Differentially and developmentally regulated expression of three rice sucrose synthase genes. Plant Cell Physiol. 40, 800–807. doi: 10.1093/oxfordjournals.pcp.a029608
Wang, L., Li, X. R., Lian, H., Ni, D. A., He, Y. K., Chen, X. Y., et al. (2010). Evidence that high activity of vacuolar invertase is required for cotton fiber and Arabidopsis root elongation through osmotic dependent and independent pathway, respectively. Plant Physiol. 154, 744–756. doi: 10.1104/pp.110.162487
Wang, L., and Ruan, Y.-L. (2012). New insights into roles of cell wall invertase in early seed development revealed by comprehensive spatial and temporal expression patterns of GhCWIN1 in cotton. Plant Physiol. 160, 777–787. doi: 10.1104/ pp.112.203893
Weber, H., Borisjuk, L., Heim, U., Buchner, P., and Wobus, U. (1995). Seed coat-associated invertases of fava bean control both unloading and storage functions: cloning of cDNAs and cell type-specific expression. Plant Cell 7, 1835–1846. doi: 10.1105/tpc.7.11.1835
Weber, H., Borisjuk, L., and Wobus, U. (1996). Controlling seed development and seed size in Vicia faba: a role for seed coat-associated invertases and carbohydrate state. Plant J. 10, 823–834. doi: 10.1046/j.1365-313X. 1996.10050823.x
Xu, T., Wen, M., Nagawa, S., Fu, Y., Chen, J.-G., Wu, M.-J., et al. (2010). Cell surface- and Rho GTPase-based auxin signaling controls cellular interdigitation in Arabidopsis. Cell 143, 99–110. doi: 10.1016/ j.cell.2010.09.003
Yanasigawa, S., Yoo, S. D., and Sheen, J. (2003). Differential regulation of EIN3 stability by glucose and ethylene signaling in plants. Nature 425, 521–525. doi: 10.1038/ nature01984
Keywords: sugar signaling, auxin signaling, cell division, cell expansion, seed development
Citation: Wang L and Ruan Y-L (2013) Regulation of cell division and expansion by sugar and auxin signaling. Front. Plant Sci. 4:163. doi:10.3389/fpls.2013.00163
Received: 09 April 2013; Paper pending published: 24 April 2013;
Accepted: 10 May 2013; Published online: 30 May 2013.
Edited by:
Thomas L. Slewinski, Cornell University, USAReviewed by:
Sjef Smeekens, Utrecht University, NetherlandsPrem S. Chourey, United States Department of Agriculture-Agricultural Research Service, USA
Copyright: © 2013 Wang and Ruan. This is an open-access article distributed under the terms of the Creative Commons Attribution License, which permits use, distribution and reproduction in other forums, provided the original authors and source are credited and subject to any copyright notices concerning any third-party graphics etc.
*Correspondence: Lu Wang, Department of Biology, School of Environmental and Life Sciences, The University of Newcastle, B110, Biology building, Callaghan, NSW 2308, Australia e-mail:bHUud2FuZ0BuZXdjYXN0bGUuZWR1LmF1; Yong-Ling Ruan, Department of Biology, School of Environmental and Life Sciences, The University of Newcastle, B112, Biology building, Callaghan, NSW 2308, Australia e-mail:eW9uZy1saW5nLnJ1YW5AbmV3Y2FzdGxlLmVkdS5hdQ==