- Department of Environmental and Plant Biology, Molecular and Cellular Biology Program, Ohio University, Athens, OH, USA
Recent research, mostly in Arabidopsis thaliana, has led to the identification and characterization of the glycosyltransferases responsible for the biosynthesis of two of the most functionally important and abundant families of plant cell wall proteins, extensins, and arabinogalactan-proteins. Extensin glycosylation involves monogalactosylation of serine residues by O-α-serine galactosyltransferase and the addition of oligoarabinosides one to five arabinose units in length to contiguous hydroxyproline residues by a set of specific arabinosyltransferase enzymes, which includes hydroxyproline O-β-arabinosyltransferases, β-1,2-arabinosyltransferases, and at least one α-1,3-arabinosyltransferase. AGP glycosylation, however, is much more complex and involves the addition of large arabinogalactan polysaccharide chains to non-contiguous hydroxyproline residues. These arabinogalactan chains are composed of β-1,3-galactan backbones decorated with β-1,6-galactose side chains that are further modified with α-arabinose as well as other sugars, including β-(methyl)glucuronic acid, α-rhamnose, and α-fucose. Specific sets of hydroxyproline O-β-galactosyltransferases, β-1,3-galactosyltransferases, β-1,6-galactosyltransferases, α-arabinosyltransferases, β-glucuronosyltransferases, α-rhamnosyltransferases, and α-fucosyltransferases are responsible for the synthesis of these complex structures. This mini-review summarizes the EXT and AGP glycosyltransferases identified and characterized to date along with corresponding genetic mutant data, which addresses the functional importance of EXT and AGP glycosylation. In one case, genetic mutant data indicate that the carbohydrate moiety of arabinogalactan-proteins may serve as an extracellular biosensor or signal for normal cellular growth. Finally, future research challenges with respect to understanding the function of these enzymes more completely and discovering and characterizing additional glycosyltransferases responsible for extensin and arabinogalactan-protein biosynthesis are also discussed.
Introduction
Extensins (EXTs) and arabinogalactan-proteins (AGPs) are plant cell wall hydroxyproline-rich glycoproteins (HRGPs) and represent two of the most post-translationally modified and abundant protein families in plants and on Earth. EXTs are characterized by the repeating pentapeptide sequence Ser-Hyp-Hyp-Hyp-Hyp (SO4) or variations thereof, such as SO3 and SO5 as well as by monogalactosylation of some Ser residues and oligoarabinosylation of most Hyp residues (Showalter, 1993; Kieliszewski and Lamport, 1994; Liu et al., 2016). EXTs are rod-like glycoproteins that exist in a polyproline II helix stabilized by the oligoarabinosides wrapping around the protein backbone (Van Holst and Varner, 1984; Shpak et al., 2001). Some EXTs contain Tyr residues that are modified to form intramolecular isodityrosine crosslinks or intermolecular di-isodityrosine or pulcherosine crosslinks (Brady and Fry, 1997; Brady et al., 1998). Intramolecular crosslinks cause “kinks” in the rods, while intermolecular crosslinks result in formation of EXT networks in the wall. Such crosslinks may be important in regulating growth and development and in forming a defensive barrier to pathogens. EXT-pectin crosslinks also exist, but their extent and functional significance is unknown (Qi et al., 1995).
In contrast, AGPs are much more heavily glycosylated than EXTs and are not diagnostically characterized by SO3, SO4, and SO5 repeats. Instead, AGPs have an abundance of Hyp, Ala, Ser, and Thr residues and are often characterized by the occurrence of Ala-Pro, Pro-Ala, Ser-Pro, Thr-Pro, Val-Pro, and Gly-Pro dipeptide repeats. Extensive glycosylation of AGPs occurs on clustered, non-contiguous Hyp residues and consists of arabinose and galactose-rich polysaccharide side chains. Arabinogalactan (AG) side chains attached to the AGP core protein allow AGPs to react with a chemical called Yariv reagent, which is useful for identifying, quantitating, and localizing AGPs (Showalter, 2001; Seifert and Roberts, 2007). AG polysaccharides are composed of β-1,3-galactan backbones decorated with β-1,6-galactose side chains that are further modified with α-arabinose as well as other sugars, including β-(methyl)glucuronic acid, α-rhamnose, and α-fucose. Based on microscopic observations and modeling of Hyp-AG side chains, AGPs are spheroidal or rod-like molecules, corresponding to the wattle blossom and twisted hairy rope models of AGP structure, respectively. While there is little evidence for AGP-AGP crosslinking, there is evidence for AGP-pectin and AGP-pectin-arabinoxylan crosslinking (Kjellbom et al., 1997; Tan et al., 2013). Most AGPs have glycosylphosphatidylinositol (GPI) anchors, which allow them to attach to the plasma membrane and reside in the periplasm. Specific phospholipases are thought to allow for release of these AGPs into the wall.
Much of the recent work on EXTs and AGPs has focused on the biosynthesis of their carbohydrate moieties and the functional contributions of these moieties (Tan et al., 2012; Knoch et al., 2014). Most of this work has used Arabidopsis thaliana as the model. Interestingly, cellulosic biofuel research, which in part requires a deeper understanding of cell wall biosynthesis and the associated biosynthetic enzymes, has served as a major driving force to examine HRGP biosynthesis and particularly the associated glycosyltransferases (GTs) responsible for decorating their protein backbones with specific sugar side chains. This mini-review focuses on these GTs that act on EXTs (Figure 1A) and AGPs (Figure 1B), the use of reverse genetics/mutants to dissect the functional roles of these sugar moieties (Table 1), and research challenges in this field.
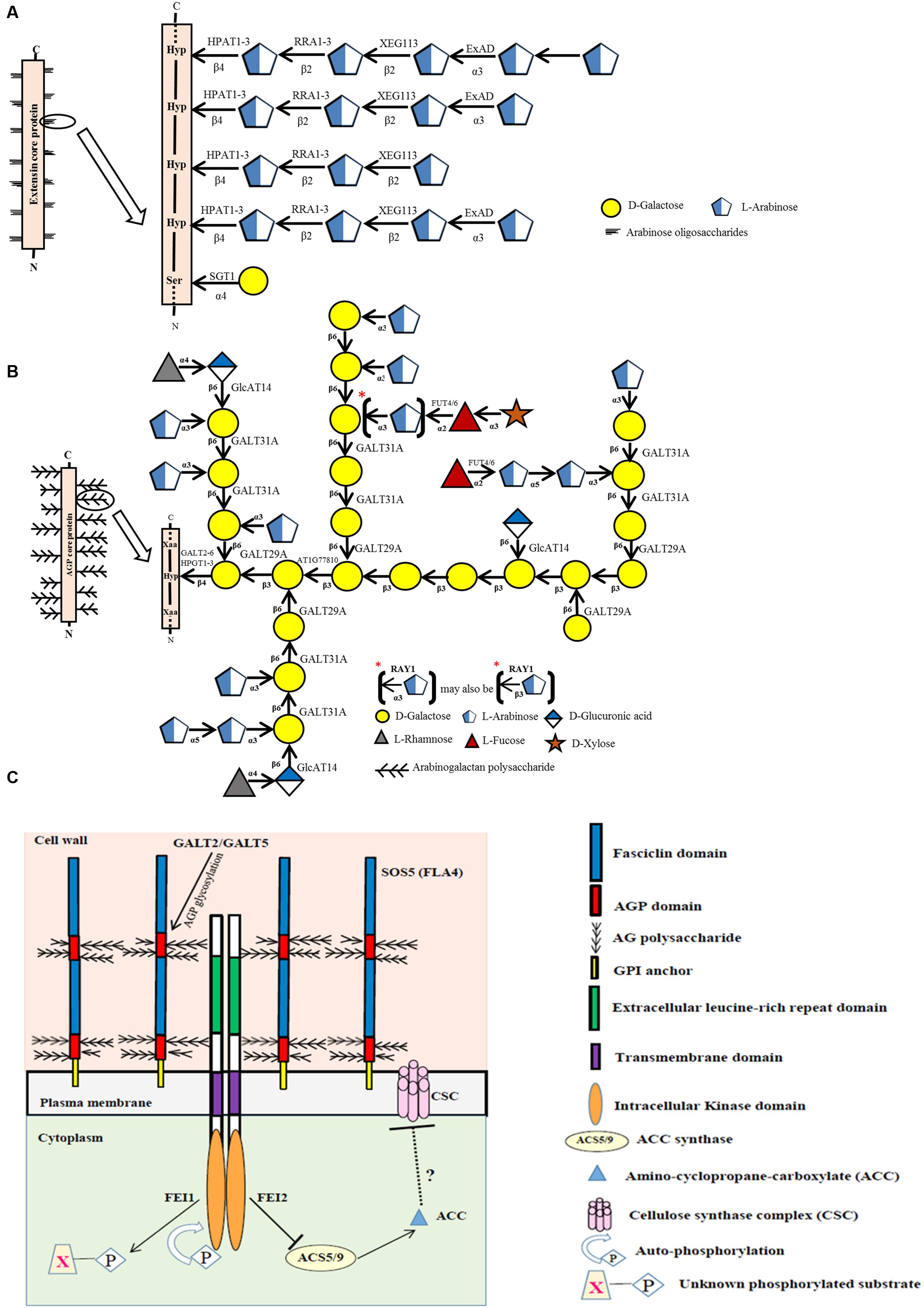
FIGURE 1. Biosynthesis of EXTs (A) and AGPs (B) and the possible role of a fasciclin-like AGP (SOS5/FLA4) as an extracellular biosensor (C). (A) Sites of action of known glycosyltransferases acting on EXTs are depicted within a representative EXT glycomodule sequence found in an EXT molecule. (B) Sites of action of known glycosyltransferases acting on AGPs are depicted within a representative AGP glycomodule sequence found within an AGP molecule. (C) Proposed model that links AGP-Hyp GALTs, GALT2 and GALT5 with SOS5 (FLA4) and FEI1/FEI2 in regulating cellular signaling of root growth. Signaling of normal root growth likely involves GALT2/GALT5-dependent glycosylation of SOS5 and glycosylated SOS5 binding FEI1/FEI2, which in turn triggers binding of FEI1/FEI2 to ACC synthase, specifically ACS5, to form a scaffold complex. The ACC synthase in the scaffold is thought to lead to the localized production of ACC, a potential signaling molecule of cell wall integrity. In contrast, when GALT2/GALT5-dependent SOS5 glycosylation is inhibited or when SOS5 or FEI1/FEI2 is disrupted, ACC synthase (ACS5) is not bound to FEI1/FEI2 and thus is not part of the scaffold. Consequently, the unbound ACC synthase produces non-localized ACC, which is converted to ethylene, and serves to inhibit cellulose synthase/cellulose biosynthesis. Such weaker or compromised cell walls lead to root tip swelling in response to elevated NaCl or sucrose treatment. It is also likely that GALT3, GALT4, and GALT6 as well as HPGT1, HPGT2, and HPGT3 play redundant or partially redundant roles to GALT2 and GALT5 in this pathway.
EXT Glycosyltransferases
At least nine genes are involved with EXT glycosylation in Arabidopsis (Figure 1A; Table 1). One of these genes, SERINE GALACTOSYLTRANSFERASE1 (SGT1), is responsible for adding single galactose residues to Ser residues in SO4 sequences, but not in SP4 sequences. This gene was identified in Chlamydomonas reinhardtii by sequencing a protein with O-α-serine galactosyltransferase activity; subsequently, homologous genes were identified in Nicotiana tabacum and Arabidopsis thaliana (Saito et al., 2014). The SGT1 enzyme was localized primarily to the endoplasmic reticulum (ER) membrane and to the Golgi, and its activity requires the presence of Hyp residues. SGT1 was initially absent from the CAZy database, but now is considered a member of the GT96 family.
The other eight genes involved with EXT glycosylation encode arabinosyltransferases with specific substrate specificities and enzymatic activities. Three of these genes (HPAT1, HPAT2, and HPAT3) encode hydroxyproline O-β-arabinosyltransferases (HPATs), which add single arabinose residues to Hyp residues in SO4 sequences (Ogawa-Ohnishi et al., 2013). These genes were identified in Arabidopsis by affinity purification and sequencing proteins that demonstrated HPAT activity. These three genes/enzymes are related to GT8 family members, but were placed in a new family, GT95. Three other genes, REDUCED RESIDUAL ARABINOSE1-3 (RRA1, RRA2, and RRA3), encode arabinosyltransferases that add the second arabinose residue in a β-1,2 linkage (Egelund et al., 2007; Velasquez et al., 2011). These three genes/enzymes are GT77 family members and were discovered by genetic mutant analysis. Another gene, XYLOGLUCANASE113 (XEG113), encodes the arabinosyltransferase which adds the third arabinose residue also in a β-1,2 linkage (Gille et al., 2009). This gene/enzyme is also in GT77 and was discovered from a genetic mutant analysis involving treatment of Arabidopsis with a fungal endogluconase. Another gene, EXTENSIN ARABINOSE DEFICIENT (ExAD), encodes the arabinosyltransferase that adds the fourth arabinose in an α-1,3 linkage (Velasquez et al., 2012). This gene/enzyme is a member of GT47 and was discovered using a genetic mutant approach similar to that used to find the RRAs. All the EXT arabinosyltransferases identified above are localized to the Golgi.
While the extent of Hyp-arabinosylation is variable for EXTs with most having three or four arabinose residues per Hyp, some EXTs have five arabinose residues. This indicates that at least one more arabinosyltransferase gene likely exists to encode the transfer of this fifth arabinose.
EXT Glycosyltransferase Mutants
EXT GT genetic mutants have provided a powerful approach to determine the functional contributions of the carbohydrate moieties that decorate EXTs. The current list of these mutants appears in Table 1 along with their respective mutant phenotypes. For example, sgt1 mutants display larger rosettes and longer roots (Saito et al., 2014; Velasquez et al., 2015). The hpat mutants demonstrate several pleiotropic phenotypes including impaired pollen tube growth, early senescence, early flowering, defects in cell wall thickening, enhanced hypocotyl elongation, and shorter root hairs (Ogawa-Ohnishi et al., 2013; Velasquez et al., 2015; MacAlister et al., 2016). Interestingly, rra and xeg113 mutants also share one of the phenotypes displayed by hpat mutants, namely reduced root hair growth (Egelund et al., 2007; Gille et al., 2009; Velasquez et al., 2011, 2015).
AGP Glycosyltransferases
To date, 17 different genes corresponding to seven distinct enzymes are involved with AGP glycosylation in Arabidopsis (Figure 1B; Table 1). AGP glycosylation is initiated by the action of hydroxyproline O-β-galactosyltransferase, which places the first galactose residue onto Hyp residues in AGP core proteins. Eight genes encoding this activity are known. Five of these were identified using bioinformatics and verified by heterologous expression coupled with an in vitro enzyme assay and by genetic mutant analysis (Basu et al., 2013, 2015a,b). These genes, named GALT2, GALT3, GALT4, GALT5, and GALT6, exist as a small gene family within GT31. Each gene encodes a GALT domain as well as a GALECTIN domain. Another similar gene in GT31, GALT1, also encodes both of these domains, but is not involved in AGP glycosylation; instead this gene is involved in adding galactose to Lewis a structures (Strasser et al., 2007). The other three genes were found by sequencing proteins selected by affinity chromatography with an AGP peptide and by heterologous expression coupled with an enzyme assay and by genetic mutant analysis (Ogawa-Ohnishi and Matsubayashi, 2015). These genes, named HPGT1, HPGT2, and HPGT3, form another small gene family within GT31, but lack a GALECTIN domain. GALT3-6 and HPGT1 are localized to Golgi; however, GALT2 is localized to both ER and Golgi, indicating AGP glycosylation may begin in the ER, but likely mainly occurs in Golgi.
Three additional AGP galactosyltransferase genes are known. One encodes a β–1,3-galactosyltransferase activity and likely functions in β–1,3-galactan backbone synthesis. This gene, At1g77810, was identified by bioinformatics and demonstrated to add galactose to a synthetic β–1,3-galactose disaccharide following heterologous expression in COS cells (Qu et al., 2008). This gene resides in GT31 along with a number of other putative Arabidopsis galactosyltransferases that may act on AGPs. Another GT31 gene, GALT31A, encodes a β–1,6-galactosyltransferase (Geshi et al., 2013). This gene was heterologously expressed in E. coli and Nicotiana benthamiana and elongated β–1,6-galactan side chains of AGP glycans. The other characterized galactosyltransferase gene, GALT29A, demonstrated the same enzymatic activity encoded by GALT31A as well as an additional branching activity, adding β–1,6-galactose to β–1,3-galactans of AGP glycans (Dilokpimol et al., 2014). GALT29A was identified as being co-expressed with GALT31A and resides in GT29, not GT31. GALT29A and GALT31A interact with one another based on fluorescence resonance energy transfer analysis and demonstrated enhanced enzymatic activity, suggesting these enzymes act cooperatively and exist in an enzyme complex (Dilokpimol et al., 2014).
Three β-glucuronosyltransferase genes, GlcAT14A, GlcAT14B, and GlcAT14C, add glucuronic acid to AGPs (Knoch et al., 2013; Dilokpimol and Geshi, 2014). All three genes/enzymes are members of GT14 and are reported to add glucuronic acid to both β–1,6- and β–1,3-galactose chains in an in vitro enzyme assay following heterologous expression in Pichia pastoris. Glucuronic acid imparts a negative charge to AGPs and provides a potential site for calcium binding; both of these properties likely have functional ramifications (Lamport and Várnai, 2013).
Two α-fucosyltransferase genes, FUT4 and FUT6, encode enzymes which add α-1,2-fucose residues to AGPs (Wu et al., 2010; Liang et al., 2013; Tryfona et al., 2014). These genes were identified by bioinformatics and demonstrated to add fucose to AGPs following heterologous expression in BY2 cells (Wu et al., 2010). These enzymes appear to be partially redundant as they display somewhat different AGP substrate specificities. Both genes/enzymes reside in GT37. Fucose is not present in all AGPs, including those produced by BY2 cells, a fact that was exploited to verify their activity.
Finally, one gene encodes an arabinosyltransferase that may act on AGPs based on genetic mutant analysis in Arabidopsis. Mutations in this gene resulted in plants with a reduced level of arabinose, particularly 3-linked arabinofuranose, in their AGPs, leading to its name, REDUCED ARABINOSE YARIV1, or RAY1 (Gille et al., 2013). This gene/enzyme is in GT77, the same family that contains RRA1-3 AND XEG113, enzymes responsible for EXT arabinosylation (Egelund et al., 2007; Gille et al., 2009; Velasquez et al., 2011). Thus, it was not surprising when heterologous expression of RAY1 in Nicotiana benthamiana demonstrated β–arabinosyltransferase activity to methyl β–galactose. Since α-linked arabinose, and not β-linked arabinose, is reported in AGPs, it remains unclear whether RAY1 functions in AGP biosynthesis.
AGP Glycosyltransferase Mutants
Many AGP GT genetic mutants are characterized and beginning to reveal the importance of AGP glycosylation to AGP function (Table 1). The galt2-6 single mutants revealed some physiological phenotypes under normal growth conditions, including reduced root hair length and density for galt2, galt3, and galt5, reduced seed set for galt4 and galt6, reduced adherent seed coat mucilage for galt3 and galt6, and premature senescence for galt6 (Basu et al., 2013, 2015a,b). However, galt2galt5 double mutants showed more severe and pleiotropic physiological phenotypes than the single mutants with respect to root hair length and density and seed coat mucilage; double mutants also displayed more rosette leaves, delayed flowering, and shorter siliques (Basu et al., 2013, 2015a,b). Such findings are consistent with the idea that as additional redundant or partially redundant genes are knocked-out for a particular enzyme activity, more severe physiological phenotypes will be revealed. The pleiotropic nature of these mutations highlights the widespread occurrence of AGPs throughout the plant and the functional importance of AGP carbohydrate moieties. These ideas were also supported by hpgt mutant data. Specifically, hpgt1-3 single mutants showed no obvious phenotypes under normal growth conditions, however, hpgt1hpgt2hpgt3 triple mutants showed several pleiotropic phenotypes including longer lateral roots, increased root hair length and density, thicker roots, smaller rosette leaves, shorter petioles, shorter inflorescence stems, reduced fertility, and shorter siliques (Ogawa-Ohnishi and Matsubayashi, 2015). Some of these phenotypes were observed in the galt mutants.
With respect to other AGP galactosyltransferase genes, a single knockout mutant only exists for GALT31A (Geshi et al., 2013). This mutant, however, is embryo lethal, exhibiting abnormal cell division in the hypophysis, despite specific expression of GALT31A in the embryo suspensor cells.
Knockout mutants for GlcAT14A-C showed enhanced cell elongation rates in dark grown hypocotyls and light grown roots during seedling growth (Knoch et al., 2013; Dilokpimol and Geshi, 2014). However, since several sugars were altered in these mutants in addition to reduced glucuronic acid, the mutant phenotypes may reflect contributions of these other Knockout mutants for FUT4 and FUT6 show no obvious phenotypes under normal conditions; however, root growth is significantly inhibited in these mutants when grown in salt (Liang et al., 2013; Tryfona et al., 2014). This conditional phenotype reveals the importance of this minor sugar component of some AGPs with respect to root growth and salt sensitivity.
Finally, a knockout mutant for RAY1 exhibits pleiotropic effects, reduced root growth, reduced rosette size, and reduced inflorescence size (Gille et al., 2013). The above ground mutant phenotypes, however, may be a secondary effect of impaired root growth.
Clearly, a range of phenotypes is observed for these AGP GT mutants. This indicates the important and diverse functions that AGP glycans play in plant vegetative and reproduction growth and development.
Research Challenges
As research on EXT and AGP GTs moves forward, several questions and challenges come to mind:
(1) There is a need to identify and characterize the remaining EXT and AGP GT genes/enzymes in Arabidopsis, including the EXT arabinosyltransferase for the fifth arabinose and other AGP β–1,3-galactosyltransferases and β–1,6-galactosyltransferases along with many α-arabinosyltransferases and α-rhamnosyltransferases, which await discovery. There is also a need to extend this research to other plant species. Interestingly, there is no evidence for any processive GTs involved in synthesizing HRGPs, such as the β–1,3-galactan backbone or β–1,6-galactan side chains of AGPs. Thus, it is conceivable that each sugar in an AGP (or EXT) is added by a specific, non-processive enzyme.
(2) It is important to determine the exact enzymatic activity for each EXT and AGP GT. In other words, does the GT act on all or only a subset of EXTs or AGPs? What is the peptide or glycan substrate specificity for each EXT or AGP GT? How do these GTs regulate the extent, length, sequence, and heterogeneity of the sugar additions?
(3) Clearly, multiple EXT and AGP GTs exist and catalyze identical, or very similar, enzymatic activities. Thus, it becomes important to determine whether these genes are redundant, partially redundant, or non-redundant. Such determinations are likely to involve examination of organ/tissue-specific expression patterns, elucidating substrate specificities, and genetic mutant analysis.
(4) For some AGP hydroxyproline O-β-galactosyltransferases, namely GALT2-6, what is the function of the GALECTIN domain?
(5) Do EXT and AGP GTs act cooperatively and in enzyme complexes? To date, only one study has provided support for this idea (Dilokpimol et al., 2014).
(6) For the GT mutants, it becomes important to know exactly which EXTs and AGPs are modified and the chemical details of such modifications in order to relate structure to function.
(7) It will be useful to grow and examine the various GT mutants side-by-side under identical environmental conditions, ideally in the same laboratory, to ensure accurate comparisons.
(8) Higher order GT mutants, particularly in cases where gene families encode a particular EXT GT (e.g., RRA1–3) or AGP GT (e.g., GALT2–6, HPGT1–3), should be produced and examined for functional redundancy.
AGPs as Extracellular Sensors or Signals
Based on bioinformatic predictions, approximately half of the AGPs in Arabidopsis are GPI-anchored to the outer leaflet of the plasma membrane (Showalter et al., 2010). This cell surface location provides an ideal venue for GPI-anchored AGPs to sense the extracellular environment and relay such information to other plasma membrane proteins involved in cellular signaling. For example, GPI-anchored AGPs may act as biochemical pressure/turgor sensors or may serve to selectively bind and release calcium ions (Lamport and Várnai, 2013). In recent years, evidence for a GPI-anchored AGP named SALT OVERLY SENSITIVE 5 (SOS5) being involved in cellular signaling of root growth has accumulated (Shi et al., 2003; Xu et al., 2008). SOS5, also known as FASCICLIN-LIKE AGP4 or FLA4, acts in a single genetic pathway that involves two cell wall leucine-rich, repeat receptor-like kinases (RLKs), called FEI1 and FEI2. Recently, GALT2 and GALT5 were shown to exist in this genetic pathway, and likely are responsible for glycosylating SOS5 to allow it to function in this signaling pathway by directly or indirectly interacting with FEI1/FEI2 (Figure 1C) (Basu et al., 2016). GALT3, GALT4, and GALT6 as well as HPGT1-3, may play redundant or partially redundant roles to GALT2 and GALT5 in this pathway. While the downstream mechanism remains to be elucidated, cellulose synthesis is the likely target, which leads to weaker cell walls and root tip swelling in the mutants.
GALT2, GALT5, SOS5, and FEI2 also function together in another signaling pathway leading to seed coat mucilage adherence (Basu et al., 2016). Mutations in these four genes, as well as higher order mutant combinations, lead to reduced mucilage adherence. While the downstream mechanism here may also involve cellulose synthesis, it is suggested to primarily involve pectin structure or assembly (Harpaz-Saad et al., 2011, 2012; Griffiths et al., 2014).
Concluding Remarks
Carbohydrate largely defines the molecular surfaces and hence the functions of EXTs and AGPs. Thus, it is of importance to understand how the various sugars are added to EXTs and AGPs and contribute to their functions not only in terms of basic science, but also for potential applications in biomimetics and biofuel production. Genetic mutant analysis of the various GT mutants is beginning to provide such insight. Finally, up to this point, GTs involved with EXT and AGP biosynthesis were identified and characterized mainly from Arabidopsis; however, future work on isolating and characterizing homologous or similar GTs acting on EXTs and AGPs from other members of the plant kingdom promises to extend and enrich our knowledge of these important enzymes.
Author Contributions
AS outlined and wrote the manuscript. DB assisted with writing the manuscript and prepared the figure and table for the manuscript.
Funding
The research reported in this mini-review from the Showalter laboratory was made possible by financial support from a National Science Foundation Grant (grant no. 0918661), an Ohio University Baker Grant FN1006071, an Ohio Plant Biotechnology Consortium Grant 020340004090 GR0017687.01, and two National Research Initiative Competitive Grants, 2008-35318-04563 and 2008-35318-04572, from the United States Department of Agriculture National Institute of Food and Agriculture.
Conflict of Interest Statement
The authors declare that the research was conducted in the absence of any commercial or financial relationships that could be construed as a potential conflict of interest.
Acknowledgment
We thank our colleagues Dr. Ahmed Faik and Dr. Naomi Geshi for helpful discussions of and suggestions to the manuscript.
Abbreviations
EXTs, Extensins; GALT, Galactosyltransferase; GT, Glycosyltransferase; Hyp, Hydroxyproline; Ser, Serine.
References
Basu, D., Liang, Y., Liu, X., Himmeldirk, K., Faik, A., Kieliszewski, M., et al. (2013). Functional identification of a hydroxyproline-O-galactosyltransferase specific for arabinogalactan protein biosynthesis in Arabidopsis. J. Biol. Chem. 288, 10132–10143. doi: 10.1074/jbc.M112.432609
Basu, D., Tian, L., DeBrosse, T., Poirier, E., Emch, K., Herock, H., et al. (2016). Glycosylation of a fasciclin-like arabinogalactan-protein (SOS5) mediates root growth and seed mucilage adherence via a cell wall receptor-like kinase (FEI1/FEI2) pathway in Arabidopsis. PLoS ONE 11:e0145092. doi: 10.1371/journal.pone.0145092
Basu, D., Tian, L., Wang, W., Bobbs, S., Herock, H., Travers, A., et al. (2015a). A small multigene hydroxyproline-O-galactosyltransferase family functions in arabinogalactan-protein glycosylation, growth and development in Arabidopsis. BMC Plant Biol. 15:295. doi: 10.1186/s12870-015-0670-7
Basu, D., Wang, W., Ma, S., DeBrosse, T., Poirier, E., Emch, K., et al. (2015b). Two hydroxyproline galactosyltransferases, GALT5 and GALT2, function in arabinogalactan-protein glycosylation, growth and development in Arabidopsis. PLoS ONE 10:e0125624. doi: 10.1371/journal.pone.0125624
Brady, J. D., and Fry, S. C. (1997). Formation of di-isodityrosine and loss of isodityrosine in the cell walls of tomato cell-suspension cultures treated with fungal elicitors or H2O2. Plant Physiol. 115, 87–92. doi: 10.1104/pp.115.1.87
Brady, J. D., Sadler, I. H., and Fry, S. C. (1998). Pulcherosine, an oxidatively coupled trimer of tyrosine in plant cell walls: its role in cross-link formation. Phytochemistry 47, 349–353. doi: 10.1016/S0031-9422(97)00592-X
Dilokpimol, A., and Geshi, N. (2014). Arabidopsis thaliana glucuronosyltransferase in family GT14. Plant Signal. Behav. 9, e28891. doi: 10.4161/psb.28891
Dilokpimol, A., Poulsen, C. P., Vereb, G., Kaneko, S., Schulz, A., and Geshi, N. (2014). Galactosyltransferases from Arabidopsis thaliana in the biosynthesis of type II arabinogalactan: molecular interaction enhances enzyme activity. BMC Plant Biol. 14:90. doi: 10.1186/1471-2229-14-90
Egelund, J., Obel, N., Ulvskov, P., Geshi, N., Pauly, M., Bacic, A., et al. (2007). Molecular characterization of two Arabidopsis thaliana glycosyltransferase mutants, rra1 and rra2, which have a reduced residual arabinose content in a polymer tightly associated with the cellulosic wall residue. Plant Mol. Biol. 64, 439–449. doi: 10.1007/s11103-007-9162-y
Geshi, N., Johansen, J. N., Dilokpimol, A., Rolland, A., Belcram, K., Verger, S., et al. (2013). A galactosyltransferase acting on arabinogalactan protein glycans is essential for embryo development in Arabidopsis. Plant J. 76, 128–137. doi: 10.1111/tpj.12281
Gille, S., Hänsel, U., Ziemann, M., and Pauly, M. (2009). Identification of plant cell wall mutants by means of a forward chemical genetic approach using hydrolases. Proc. Natl. Acad. Sci. U.S.A. 106, 14699–14704. doi: 10.1073/pnas.0905434106
Gille, S., Sharma, V., Baidoo, E. E. K., Keasling, J. D., Scheller, H. V., and Pauly, M. (2013). Arabinosylation of a yariv-precipitable cell wall polymer impacts plant growth as exemplified by the arabidopsis glycosyltransferase mutant ray1. Mol. Plant 6, 1369–1372. doi: 10.1093/mp/sst029
Griffiths, J. S., Tsai, A. Y.-L., Xue, H., Voiniciuc, C., Šola, K., Seifert, G. J., et al. (2014). SALT-OVERLY SENSITIVE5 mediates Arabidopsis seed coat mucilage adherence and organization through pectins. Plant Physiol. 165, 991–1004. doi: 10.1104/pp.114.239400
Harpaz-Saad, S., McFarlane, H. E., Xu, S., Divi, U. K., Forward, B., Western, T. L., et al. (2011). Cellulose synthesis via the FEI2 RLK/SOS5 pathway and cellulose synthase 5 is required for the structure of seed coat mucilage in Arabidopsis. Plant J. 68, 941–953. doi: 10.1111/j.1365-313X.2011.04760.x
Harpaz-Saad, S., Western, T. L., and Kieber, J. J. (2012). The FEI2-SOS5 pathway and CELLULOSE SYNTHASE 5 are required for cellulose biosynthesis in the Arabidopsis seed coat and affect pectin mucilage structure. Plant Signal. Behav. 7, 285–288. doi: 10.4161/psb.18819
Kieliszewski, M. J., and Lamport, D. T. A. (1994). Extensin: repetitive motifs, functional sites, post-translational codes, and phylogeny. Plant J. 5, 157–172. doi: 10.1046/j.1365-313X.1994.05020157.x
Kjellbom, P., Snogerup, L., Stöhr, C., Reuzeau, C., McCabe, P. F., and Pennell R. I. (1997). Oxidative cross-linking of plasma membrane arabinogalactan proteins. Plant J. 12, 1189–1196. doi: 10.1046/j.1365-313X.1997.12051189.x
Knoch, E., Dilokpimol, A., and Geshi, N. (2014). Arabinogalactan proteins: focus on carbohydrate active enzymes. Front. Plant Sci. 11:198. doi: 10.3389/fpls.2014.00198
Knoch, E., Dilokpimol, A., Tryfona, T., Poulsen, C. P., Xiong, G., Harholt, J., et al. (2013). A β-glucuronosyltransferase from Arabidopsis thaliana involved in biosynthesis of type II arabinogalactan has a role in cell elongation during seedling growth. Plant J. 76, 1016–1029. doi: 10.1111/tpj.12353
Lamport, D. T. A., and Várnai, P. (2013). Periplasmic arabinogalactan glycoproteins act as a calcium capacitor that regulates plant growth and development. New Phytol. 197, 58–64. doi: 10.1111/nph.12005
Liang, Y., Basu, D., Pattathil, S., Xu, W.-L., Venetos, A., Martin, S. L., et al. (2013). Biochemical and physiological characterization of fut4 and fut6 mutants defective in arabinogalactan-protein fucosylation in Arabidopsis. J. Exp. Bot. 64, 5537–5551. doi: 10.1093/jxb/ert321
Liu, X., Wolfe, R., Welch, L. R., Domozych, D. S., Popper, Z. A., and Showalter, A. M. (2016). Bioinformatic identification and analysis of extensins in the plant kingdom. PLoS ONE 11:e0150177. doi: 10.1371/journal.pone.0150177
MacAlister, C. A., Ortiz-Ramırez, C., Becker, J. D., Feij, J. A., and Lippman, Z. B. (2016). Hydroxyproline O-arabinosyltransferase mutants oppositely alter tip growth in Arabidopsis thaliana and Physcomitrella patens. Plant J. 85, 193–208. doi: 10.1111/tpj.13079
Ogawa-Ohnishi, M., Matsushita, W., and Matsubayashi, Y. (2013). Identification of three hydroxyproline O-arabinosyltransferases in Arabidopsis thaliana. Nat. Chem. Biol. 9, 726–730. doi: 10.1038/nchembio.1351
Ogawa-Ohnishi, M., and Matsubayashi, Y. (2015). Identification of three potent hydroxyproline O-galactosyltransferases in Arabidopsis. Plant J. 81, 736–746. doi: 10.1111/tpj.12764
Qi, X., Behrens, B. X., West, P. R., and Mort, A. J. (1995). Solubilization and partial characterization of extensin fragments from cell walls of cotton suspension cultures: evidence for a covalent cross-link between extensin and pectin. Plant Physiol. 108, 1691–1701. doi: 10.1104/pp.108.4.1691
Qu, Y., Egelund, J., Gilson, P. R., Houghton, F., Gleeson, P. A., Schultz, C. J., et al. (2008). Identification of a novel group of putative Arabidopsis thaliana beta-(1,3)-galactosyltransferases. Plant Mol. Biol. 68, 43–59. doi: 10.1007/s11103-008-9351-3
Saito, F., Suyama, A., Oka, T., Yoko-o, T., Matsuoka, K., Jigami, Y., et al. (2014). Identification of novel peptidyl serine α-galactosyltransferase gene family in plants. J. Biol. Chem. 289, 20405–20420. doi: 10.1074/jbc.M114.553933
Seifert, G. J., and Roberts, K. (2007). The biology of arabinogalactan proteins. Annu. Rev. Plant Biol. 58, 137–161. doi: 10.1146/annurev.arplant.58.032806.103801
Shi, H., Kim, Y., Guo, Y., Stevenson, B., and Zhu, J.-K. (2003). The Arabidopsis SOS5 locus encodes a putative cell surface adhesion protein and is required for normal cell expansion. Plant Cell 15, 19–32. doi: 10.1105/tpc.007872
Showalter, A. M. (1993). Structure and function of plant cell wall proteins. Plant Cell 5, 9–23. doi: 10.1105/tpc.5.1.9
Showalter, A. M. (2001). Arabinogalactan-proteins: structure, expression and function. Cell. Mol. Life Sci. 58, 1399–1417. doi: 10.1007/PL00000784
Showalter, A. M., Keppler, B., Lichtenberg, J., Gu, D., and Welch, L. R. (2010). A bioinformatics approach to the identification, classification, and analysis of hydroxyproline-rich glycoproteins. Plant Physiol. 153, 485–513. doi: 10.1104/pp.110.156554
Shpak, E., Barbar, E., Leykam, J. F., and Kieliszewski, M. J. (2001). Contiguous hydroxyproline residues direct hydroxyproline arabinosylation in Nicotiana tabacum. J. Biol. Chem. 276, 11272–11278. doi: 10.1074/jbc.M011323200
Strasser, R., Bondili, J. S., Vavra, U., Schoberer, J., Svoboda, B., Glössl, J., et al. (2007). A unique beta1,3-galactosyltransferase is indispensable for the biosynthesis of N-glycans containing Lewis a structures in Arabidopsis thaliana. Plant Cell 19, 2278–2292. doi: 10.1105/tpc.107.052985
Tan, L., Eberhard, S., Pattathil, S., Warder, C., Glushka, J., Yuan, C., et al. (2013). An Arabidopsis cell wall proteoglycan consists of pectin and arabinoxylan covalently linked to an arabinogalactan protein. Plant Cell 25, 270–287. doi: 10.1105/tpc.112.107334
Tan, L., Showalter, A. M., Egelund, J., Hernandez-Sanchez, A., Doblin, M. S., and Bacic, A. (2012). Arabinogalactan-proteins and the research challenges for these enigmatic plant cell surface proteoglycans. Front. Plant Sci. 3:140. doi: 10.3389/fpls.2012.00140
Tryfona, T., Theys, T. E. Wagner, T., Stott, K., Keegstra, K., and Dupree, P. (2014). Characterisation of FUT4 and FUT6 α-(1→2)-fucosyltransferases reveals that absence of root arabinogalactan fucosylation increases Arabidopsis root growth salt sensitivity. PLoS ONE 9:e93291. doi: 10.1371/journal.pone.0093291
Van Holst, G. J., and Varner, J. E. (1984). Reinforced polyproline II conformation in a hydroxyproline-rich cell wall glycoprotein from carrot root. Plant Physiol. 74, 247–251. doi: 10.1104/pp.74.2.247
Velasquez, M., Salter, J. S., Dorosz, J. G., Petersen, B. L., and Estevez J. M. (2012). Recent advances on the posttranslational modifications of EXTs and their roles in plant cell walls. Front. Plant Sci. 3:93. doi: 10.3389/fpls.2012.00093
Velasquez, S. M., Marzol, E., Borassi, C., Pol-Fachin, L., Ricardi, M. M., Mangano, S., et al. (2015). Low sugar is not always good: Impact of specific O-glycan defects on tip growth in Arabidopsis. Plant Physiol. 168, 808–813. doi: 10.1104/pp.114.255521
Velasquez, S. M., Ricardi, M. M., Dorosz, J. G., Fernandez, P. V., Nadra, A. D., Pol-Fachin, L., et al. (2011). O-glycosylated cell wall extensins are essential in root hair growth. Science 33, 1401–1403. doi: 10.1126/science.1206657
Wu, Y., Williams, M., Bernard, S., Driouich, A., Showalter, A. M., and Faik, A. (2010). Functional identification of two nonredundant Arabidopsis alpha(1,2)fucosyltransferases specific to arabinogalactan proteins. J. Biol. Chem. 285, 13638–13645. doi: 10.1074/jbc.M110.102715
Keywords: biosynthesis, cell wall, extensin, arabinogalactan-protein, hydroxyproline, hydroxyproline-rich glycoproteins, glycosyltransferases, signaling
Citation: Showalter AM and Basu D (2016) Extensin and Arabinogalactan-Protein Biosynthesis: Glycosyltransferases, Research Challenges, and Biosensors. Front. Plant Sci. 7:814. doi: 10.3389/fpls.2016.00814
Received: 04 March 2016; Accepted: 25 May 2016;
Published: 15 June 2016.
Edited by:
Huanzhong Wang, University of Connecticut, USAReviewed by:
Yumiko Sakuragi, University of Copenhagen, DenmarkTaras P. Pasternak, University of Freiburg, Germany
Copyright © 2016 Showalter and Basu. This is an open-access article distributed under the terms of the Creative Commons Attribution License (CC BY). The use, distribution or reproduction in other forums is permitted, provided the original author(s) or licensor are credited and that the original publication in this journal is cited, in accordance with accepted academic practice. No use, distribution or reproduction is permitted which does not comply with these terms.
*Correspondence: Allan M. Showalter, showalte@ohio.edu