- 1State Key Laboratory of Genetic Engineering, Institute of Plant Biology, School of Life Sciences, Fudan University, Shanghai, China
- 2Research Institute of Subtropical Forestry, Chinese Academy of Forestry, Fuyang, China
Low temperature affects gene regulatory networks and alters cellular metabolism to inhibit plant growth. Peroxidases are widely distributed in plants and play a large role in adjusting and controlling reactive oxygen species (ROS) homeostasis in response to abiotic stresses such as low temperature. The Rare Cold-Inducible 35 gene from Capsella bursa-pastoris (CbRCI35) belongs to the type III peroxidase family and has been reported to be a cold responsive gene in plants. Here we performed an expressional characterization of CbRCI35 under cold and ionic liquid treatments. The promoter of CbRCI35 was also cloned and its activity was examined using the GUS reporter system. CbRCI35 protein was localized in the cytoplasm according to sequence prediction and GFP fusion assay. Heterologous expression tests revealed that CbRCI35 conferred enhanced resistance to low temperature and activated endogenous cold responsive signaling in tobacco. Furthermore, in the normal condition the ROS accumulation was moderately enhanced while after chilling exposure superoxide dismutase activity was increased in CbRCI53 transgenic plants. The ROS metabolism related genes expression was altered accordingly. We conclude that CbRCI35 modulates ROS homeostasis and contributes to cold tolerance in plants.
Introduction
Low temperature impacts plant development as well as propagation, and imposes restriction on plant distribution (Thomashow, 1999; Beike et al., 2015). In the face of cold stress, plants reorganize gene regulatory networks and adjust cellular metabolic reactions to gain enhanced cold resistance (Narusaka et al., 2004; Yue et al., 2015). The ability of plants to tolerate environmental stresses is highly associated with signaling molecules (Mengel et al., 2013). Reactive oxygen species (ROS) is one of the key signals regulating plant bioprocesses through the activation of secondary messengers, the gene transcription switch and the enzyme activity variation (Lamotte et al., 2014; Farnese et al., 2016). Biosynthesis and metabolism of ROS such as hydrogen peroxide, superoxide, singlet oxygen and hydroxyl radicals influence the redox state of plant cells in the early stages of stress response (Mittler, 2002; Del Rio, 2015). During the cold response, a burst of ROS following a metabolic imbalance of energy appears to elicit cellular damage and abnormity (Potters et al., 2007). On the other hand, the rapidly accumulated ROS mediates stress responsive signaling transduction and functions in an essential manner for cold acclimation (Zhao et al., 2009; Hossain et al., 2015). In fact, low doses of NO or H2O2 contribute to cold hardiness in multiple plant species such as Arabidopsis thaliana and Brassica juncea (Neill et al., 2002; Abat and Deswal, 2009; Cantrel et al., 2011). It has been reported that non-toxic levels of H2O2 accumulation are necessary in the plant acclimation of various stresses including low temperature (Petrov and Van Breusegem, 2012). The maintained proper concentration of H2O2 plays a role in the delicate balancing between H2O2 production and scavenging during stress response (Hossain et al., 2015). Moderately elevated ROS can activate cold responsive gene expression and mediate protective mechanisms against damage to macromolecules and cell structures in cold acclimation of plants (Yu et al., 2003; Hung et al., 2007; Wang et al., 2010). In tomato it has been documented that H2O2 significantly strengthens antioxidant process to reduce oxidative damage (Iseri et al., 2013). Given all the clearly presented evidence showing the crucial role of ROS as a signaling molecule in cold response, information in regards to the function of ROS regulators in stress response needs to be enriched.
Plant cells possess a protective system that is comprised of enzymatic and non-enzymatic antioxidants against oxidative stress (Mittler et al., 2004). Peroxidases such as catalase (CAT) and ascorbate peroxidase (APX) act as key enzymes in modulating ROS homeostasis in response to environmental stimulus or developmental transitions (Navabpour et al., 2003). Peroxidases are widely distributed throughout the plant kingdom (Mishra et al., 2016). The type III peroxidase is a superfamily of plant-specific heme oxidoreductases that can be encoded by a large number of genes (Kvaratskhelia et al., 1997; Shigeto and Tsutsumi, 2016). In Arabidopsis, 73 type III peroxidase genes have been identified and they are implicated in a widely diverse range of bioprocesses (Tognolli et al., 2002). For example, type III peroxidases either augment or reduce the apoplastic ROS levels during cell wall development (Mangano et al., 2016). Among these genes the Rare Cold-Inducible 3 (AtRCI3) was isolated through a screening using a cDNA library from etiolated Arabidopsis plants after cold acclimation (Llorente et al., 2002). AtRCI3 showed a strong cold-induced expression pattern and encoded an active cationic peroxidase. Further analysis revealed that AtRCI3 enhanced the ROS production under potassium deficiency (Kim et al., 2010). Although identification of AtRCI3 is conducted by screening in cold responsive transcripts, studies exploring the molecular function of AtRCI3 or AtRCI3-like genes in cold response are limited.
The Rare cold-inducible 35 gene (CbRCI35) is cloned from Capsella bursa-pastoris that is grown in temperate regions but has a strong ability to tolerate low temperature (Lin et al., 2007). The CbRCI35 gene encodes a cold-inducible protein that is highly homologous to AtRCI3. In the present work, the expressional analysis and promoter activity test of CbRCI35 under cold temperatures, plant hormone and ionic treatments were performed. Functional characterization of transgenic tobacco was also performed to show that CbRCI35 regulates ROS homeostasis and cold tolerance of plants.
Materials and Methods
Plant Materials and Treatments
The seeds of C. bursa-pastoris and Nicotiana tabacum were stored as previously described (Zhou et al., 2012). The seeds of A. thaliana of Columbia (Col-0) accession were obtained from the Arabidopsis Biological Resource Center (ABRI: Columbus, OH, USA). Plants were grown at stable temperature (22°C) and light conditions (16 h light/8 h dark). For cold application of C. bursa-pastoris, the 4-week-old plants were subjected to 4°C for 4, 8, or 24 h. Meanwhile the seedlings of the same age were shifted from 22 to 12°C for 4 days, 4°C for 4 days, 0°C for 2 h continuously for cold acclimation. The roots, stems and leaves were collected at each time point and frozen in liquid nitrogen immediately. For ionic liquid treatments, 2-week-old C. bursa-pastoris seedlings were soaked with 80 mM KCl, 50 mM MgCl2, 5 mM ZnCl2, 30 mM LiCl, 0.1 mM CuCl2, 80 mM CaCl2 solution for 0, 4, 8, and 24 h. For phytohormone treatments, 2-week-old A. thaliana plants were soaked with 5 μM gibberellin (GA), 300 μM methyl jasmonate (MeJA), and 500 μM salicylic acid (SA) solution for 0, 1, 6, and 24 h, respectively. The whole seedlings were harvested for RNA extraction. For the cold tolerance test of N. tabacum, 4-week-old tobacco plants were subjected to 4°C for 24 h, -4°C for 1 h and 22°C for 2 days in turn.
Cloning of CbRCI35 Promoter from C. bursa-pastoris
The genomic DNA of C. bursa-pastoris is obtained by CTAB extraction method. According to the manual of the Universal Genome Walker TM Kit (CLONTECH), the genome walker library was constructed and nested amplification was conducted using specific primers RCI35GSP1 and RCI35GSP2 paired with the adaptor primers AP1 and AP2. A 1011 bp of 5′ upstream sequence of CbRCI35 was cloned and analyzed by PLANTCARE1 (Zhou et al., 2014).
Plant Transformation
For characterization of promoter activity, CbRCI35 promoter clones were constructed in the KpnI/NcoI site of the pCAMBIA1301 vector (CAMBIA, Australia) with primers pCbRCI35-F and pCbRCI35-R. The pCbRCI35::GUS plasmid was transformed into Arabidopsis of Col-0 plants with the Agrobacterium-mediated floral dip method. The T1 plants were selected on hygromycin (30 mg/L) media and further confirmed by PCR. The T2 lines showing 3:1 segregation were carried forward to the T3 generation. PCR positive homozygous T3 and T4 lines were used for further analyses of the CbRCI35 promoter.
For gene overexpression, CbRCI35 mRNA sequence information was previously described (Lin et al., 2007). The CbRCI35 cDNA clones were constructed in the NcoI/NheI site of the pCAMBIA1304 vector (CAMBIA, Australia) with primers CbRCI35-nco-F and CbRCI35-nhe-R, using hygromycin resistance as a selection marker. The 35S::CbRCI35 plasmid was introduced into leaf disks of tobacco using Agrobacterium EHA105. Plants transformed with the empty vector were used as a blank control. Transformants were selected on MS medium solidified with 0.8% agar containing 0.1 mg/L NAA, 1.0 mg/L 6-BA, 250 mg/L carbenicillin disodium and 30 mg/L hygromycin, and regenerated on hormone-free MS medium containing 250 mg/L carbenicillin disodium at 22°C. Transformants were identified by DNA-PCR analysis with primers Hyg-F and Hyg-R (Table 1).
Histochemical GUS Staining and Semi-Thin Section
The seedlings of 2.5-week-old transgenic Arabidopsis with pCbRCI35::GUS at 22°C or after 4°C treatment for 8 h were collected and soaked in GUS staining buffer containing 0.075% X-Gluc (5-bromo-4-chloro-3-indolyl-bd-glucuronic acid), 3 mM potassium ferricyanide, 7.2 mM EDTA, 57.7 mM disodium phosphate, 42.3 mM sodium phosphate, and 0.005% Triton X-100 followed by vacuum infiltration for 20 min. Afterward the seedling samples were incubated at 37°C overnight and de-stained in 75% ethanol at 60°C for several times until chlorophyll was removed. Another set of 4.5-week-old transgenic plants were stained and embedded with Technovit 7100 plastic embedding kit (Heraeus Kulzer, Germany) following the manufacturer’s instructions and semi-sectioned by Leica 2265 Rotary Microtome (Leica, Germany). The sections were observed with Zeiss Scope A1 microscope (Zeiss, Germany).
Subcellular Localization of CbRCI35
The CbRCI35 cDNA clones were constructed in the NcoI/BglII site of the pCAMBIA1302 vector (CAMBIA, Australia) with primers CbRCI35-nco-F and CbRCI35-bgl-R. The onion epidermis discs were incubated with a culture of Agrobacterium carrying the 35S::CbRCI35-GFP plasmid for 20 min. Acetosyringone (100 μM) was added to enhance the transformation efficiency. After grown in the dark at 25°C for 2 days, the GFP signal was detected by confocal laser scanning microscopy (Zeiss 710, Germany) and the images were analyzed with Zen software.
Quantitative Real-Time PCR
Quantitative real-time PCR (qPCR) was employed to analyze gene relative expression levels. The total RNA was extracted using Plant RNA Mini Kit (CW Biotech Inc., Ltd, China) and the cDNA was synthesized using PrimeScript® RT Master Mix (TaKaRa, China) according to the manufacturer’s instructions. The qPCR was carried out using SYBR® Premix Ex TaqTM II (Perfect Real-Time; TaKaRa, China) on a StepOnePlusTM Real-Time PCR System (Applied Biosystems) with three replicates. The PCR procedure was 95°C for 30 s, 40 cycles of 95°C for 5 s, and 60°C for 34 s, followed by 95°C for 15 s, 60°C for 1 min, and 95°C for 15 s. The CbACTIN (HQ880662), ACTIN2 (AK230311) and NtACTIN (AJ133422) were used as the internal control for C. bursa-pastoris. A. thaliana, and N. tabacum, respectively.
Physiological Indices Measurement
Physiological indices were measured as previously described (Wu et al., 2012). In brief, leaves of tobacco were incubated in 2 ml deionized water at room temperature for 12 h and the sample conductivities (C1) were measured with a DDS-11A meter (Shanghai SUOSHEN Electrical Equipment Co. Ltd., China). Then the samples were boiled in deionized water at 100°C for 1 h and cooled to room temperature to get the sample conductivities (C2). Relative electrolyte leakage was calculated using the following formula: C1/C2 × 100%. Relative water content (%) was calculated as 100% × (FW-DW)/(TW-DW), in which the fresh weight (FW) and the turgid weight (TW) were determined before and after leaves dipped in 4 ml deionized water for 12 h at room temperature, while the dry weights (DW) were measured after samples were oven dried at 65°C for 24 h. Glucose content was assayed by measuring the NADH production using the Glucose (HK) Assay kit (Sigma–Aldrich, Inc.) depending on absorbance at 340 nm (A340). The H2O2 content was measured according to A415 of the titanium-peroxide complex as previously described (Jiang and Zhang, 2001). The superoxide dismutase (SOD) activity was determined by an inhibited photoreduction rate of nitro blue tetrazolium (NBT) (Mckersie et al., 1993). The malondialdehyde (MDA) content was measured using the thiobarbituric acid (TBA)-based colorimetric method as reported previously (Draper et al., 1993). The BioPhotometer Plus (Eppendorf, Germany) was used for electromagnetic absorbance reading in each assay. Three biological replicates were used for each experiment and the data were analyzed by Student’s t-test.
Dichloro-Dihydro-Fluorescein Diacetate Assay
Tobacco leaf disk samples were immersed with 10 μM carboxy-2′,7′-dichloro- dihydro-fluorescein diacetate (DCFH-DA) probe at 37°C for 20 min in the dark (Ezaki et al., 2000). Samples were subsequently washed with 20 mM potassium phosphate buffer (pH 6.0) for three times to remove extra probe. Fluorescent signals were visualized using a confocal laser scanning microscope (Zeiss 710, Germany).
Results
Expression Pattern of CbRCI35 under Cold and Ionic Treatments
The RCI genes were identified according to their cold-induced expression pattern. CbRCI35 was responsive to cold temperatures in young C. bursa-pastoris plants (Lin et al., 2007). For a more detailed characterization, the expression patterns of CbRCI35 in different tissues in response to low temperature were detected in a cold acclimation assay and a time course assay. CbRCI35 showed a root-specific and temperature-dependent expression pattern in C. bursa-pastoris during cold acclimation from 12, 4 to 0°C. After 4 days of 12°C application, the CbRCI35 transcript did not change in leaves, stems or roots compared with the 22°C control. When treated at 4°C for 4 days followed by 0°C for 2 h, significant enhancements were observed only in roots and 0°C activated the highest observed transcription level (Figure 1A). In a time course assay, the basal level of CbRCI35 mRNA was highest in roots, and was lower in stems and leaves. During cold application, CbRCI35 transcription was elevated at the time point of 4 h and reached its peak after 8 h in 4°C. As before, in roots it showed the highest expression level, while in leaves and stems the gene expression pattern had a similar trend but the transcript levels were lower (Figure 1B).
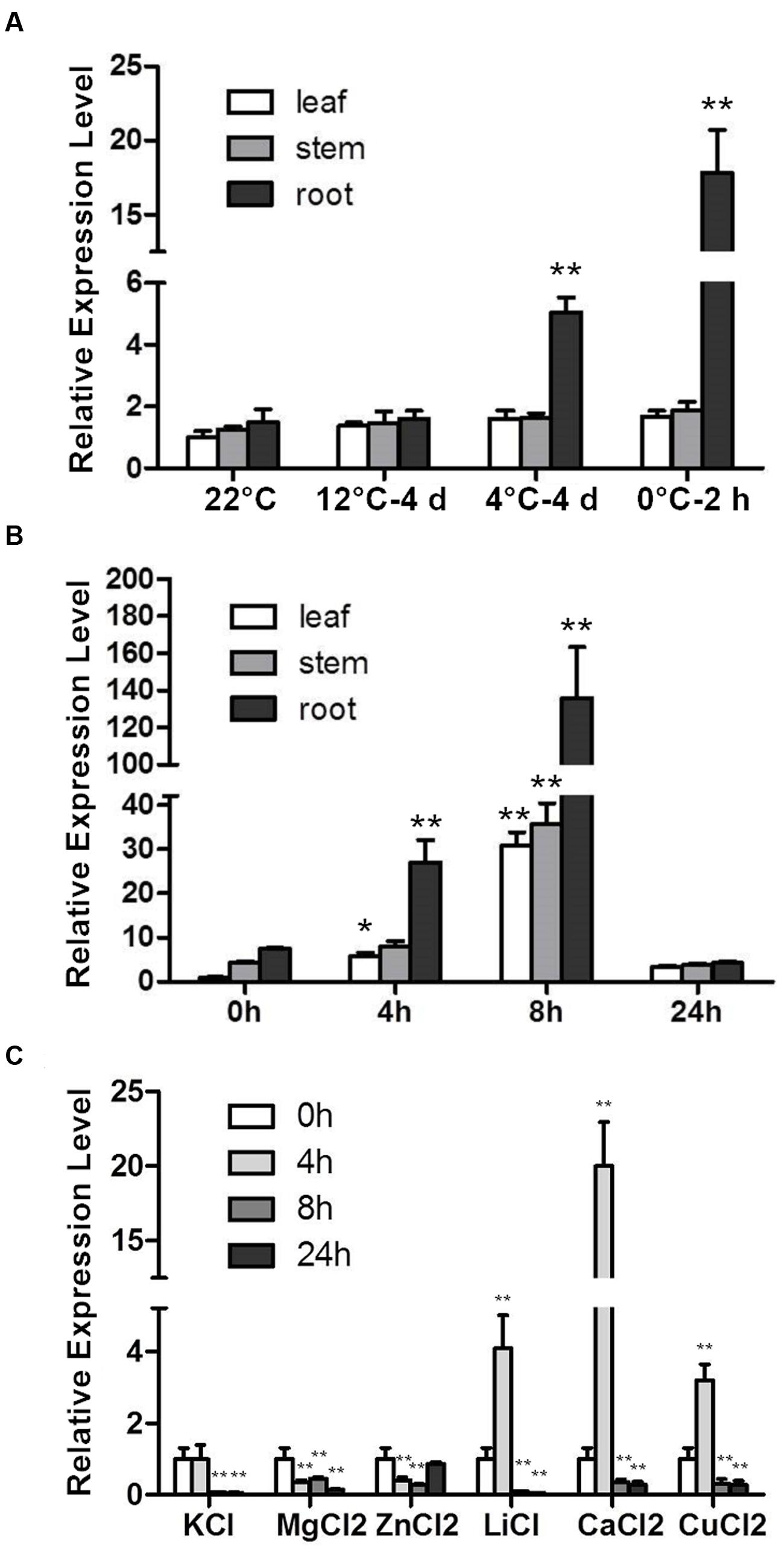
FIGURE 1. Induction of CbRCI35 in Capsella bursa-pastoris plants under different treatments. (A) The CbRCI35 expression was analyzed in leaves, roots and stem in 4-week-old plants growing at 22°C after shifting to 12°C for 4 days, 4°C for 4 days and 0°C for 2 h in turn. (B) The CbRCI35 expression in leaves, roots and stem in 4-week-old plants growing at 22°C was tested after treatments at 4°C for 0, 4, 8, and 24 h. (C) The CbRCI35 expression was examined in 2-week-old seedlings after treatments with different proton solutions at normal temperature for 0, 4, 8, and 24 h. CbActin gene was used as a housekeeping control. Expression levels were relative to control condition in each tissue. Data are means ± SD (n = 3, ∗P < 0.05, ∗∗P < 0.01).
The RCI genes coding peroxidases such as AtRCI3 are involved in K+ transportation (Kim et al., 2010) and the protein activity can be affected by ion concentration (Lai et al., 2006). We detected CbRCI35 expression in response to 80 mM KCl, 50 mM MgCl2, 5 mM ZnCl2, 30 mM LiCl, 0.1 mM CuCl2, 80 mM CaCl2 in young seedlings of C. bursa-pastoris. Consistent with the induced transcription of AtRCI3 under K+ deficiency, CbRCI35 expression was repressed by K+ application. Mg2+ also down-regulated CbRCI35 expression and Zn2+ caused a transient suppression. For Li+, Cu2+, and Ca2+, the transcription of CbRCI35 showed a significant transient elevation in the early stage, suggesting that CbRCI35 might play a role in Li+, Cu2+, and Ca2+ transportation (Figure 1C).
Cloning and Characterization of CbRCI35 Promoter from C. bursa-pastoris
A fragment of 1011 bp in the promoter region of CbRCI35 was isolated from the C. bursa-pastoris genome by DNA walking (Figure 2). Sequence analysis was performed using the PLANTCARE software2. Three cis-acting elements involved in gibberellin (GA), SA and MeJA responsiveness were identified, respectively. To further characterize the CbRCI35 promoter, a GUS reporter system driven by the 1011 bp pCbRCI35 promoter fragment was created (Figure 3A). Histochemical GUS staining indicated extremely low levels of GUS activity throughout the entire seedling at 22°C, and increased activity after 8 h exposure to 4°C in 2-week-old plants (Figure 3B). Correspondingly, a markedly increased level of GUS mRNA in response to cold was detected in 2-week-old seedlings (Figure 3C). For a more accurate, tissue-specific analysis, semi-thin sections of 4.5-week-old plant organs were dissected out after staining. In contrast to what is observed in younger seedlings, GUS accumulation was mainly in the roots, while comparatively lower GUS enhancement was observed in stems and leaves (Figure 3D). The GUS activity in roots was displayed in the cortex but not the epidermal or vascular tissues, implying the protective function of CbRCI35 for the cortical cells containing stored carbohydrates and other substances. These indicated that the cold-induced activity of CbRCI35 was in an age-dependent, tissue-specific manner. Considering the predicted cis-acting elements in the CbRCI35 promoter, phytohormone responsive activity was subsequently investigated using GA, SA, and MeJA treatments. Interestingly, GA and MeJA inhibited CbRCI35 transcription while SA conferred transient upregulation of gene expression in early stages and downregulation after 24 h of application (Figure 3E). The promoter characterization revealed a cold and phytohormone inducible expression pattern of CbRCI35.
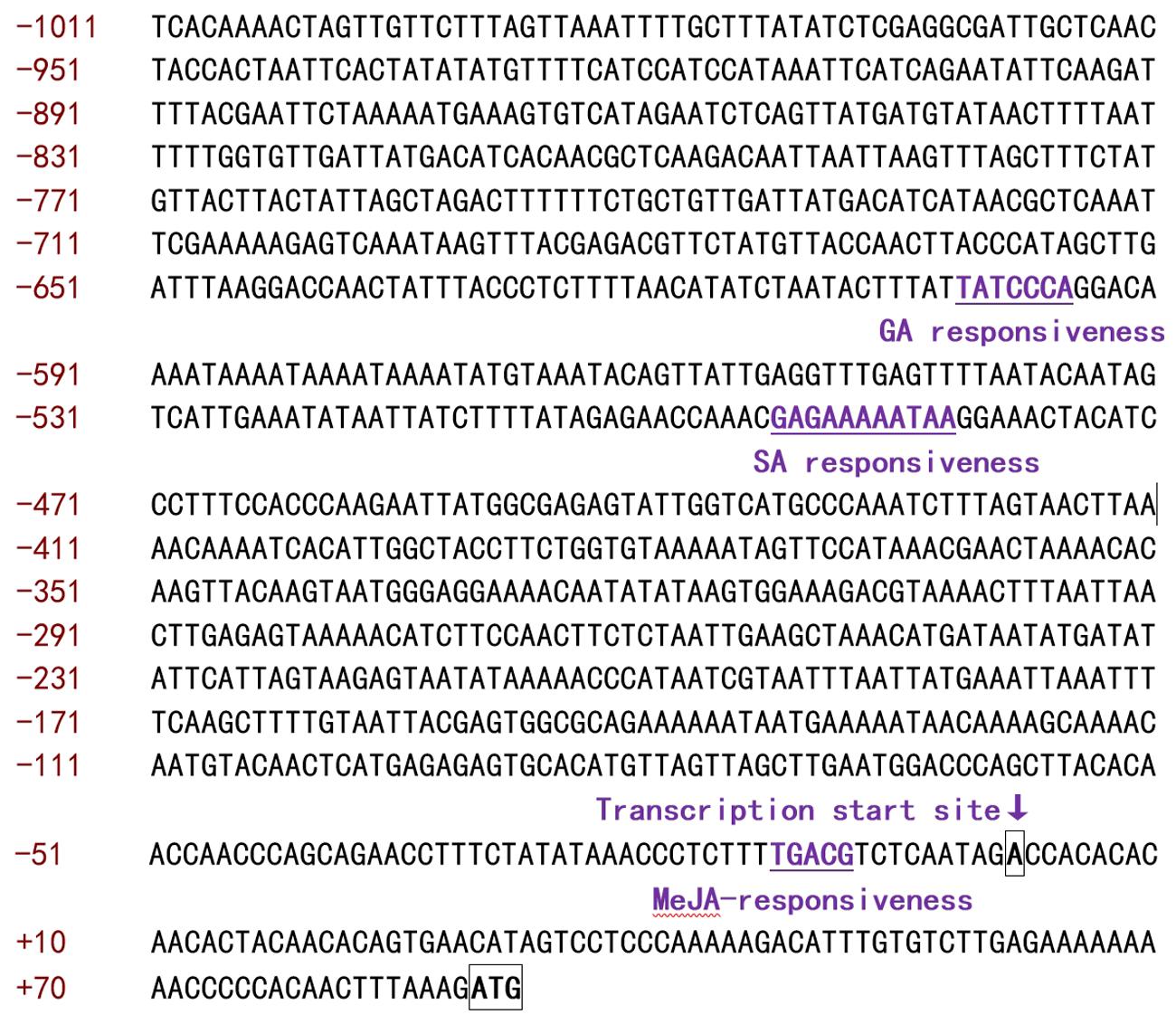
FIGURE 2. Sequences of the 5′ upstream flanking region of CbRCI35. Numbering is relative to the transcription start site which was designated +1 and indicated with an arrow. gibberellin (GA), salicylic acid (SA), and methyl jasmonate (MeJA) responsiveness associated elements were labeled and underlined.
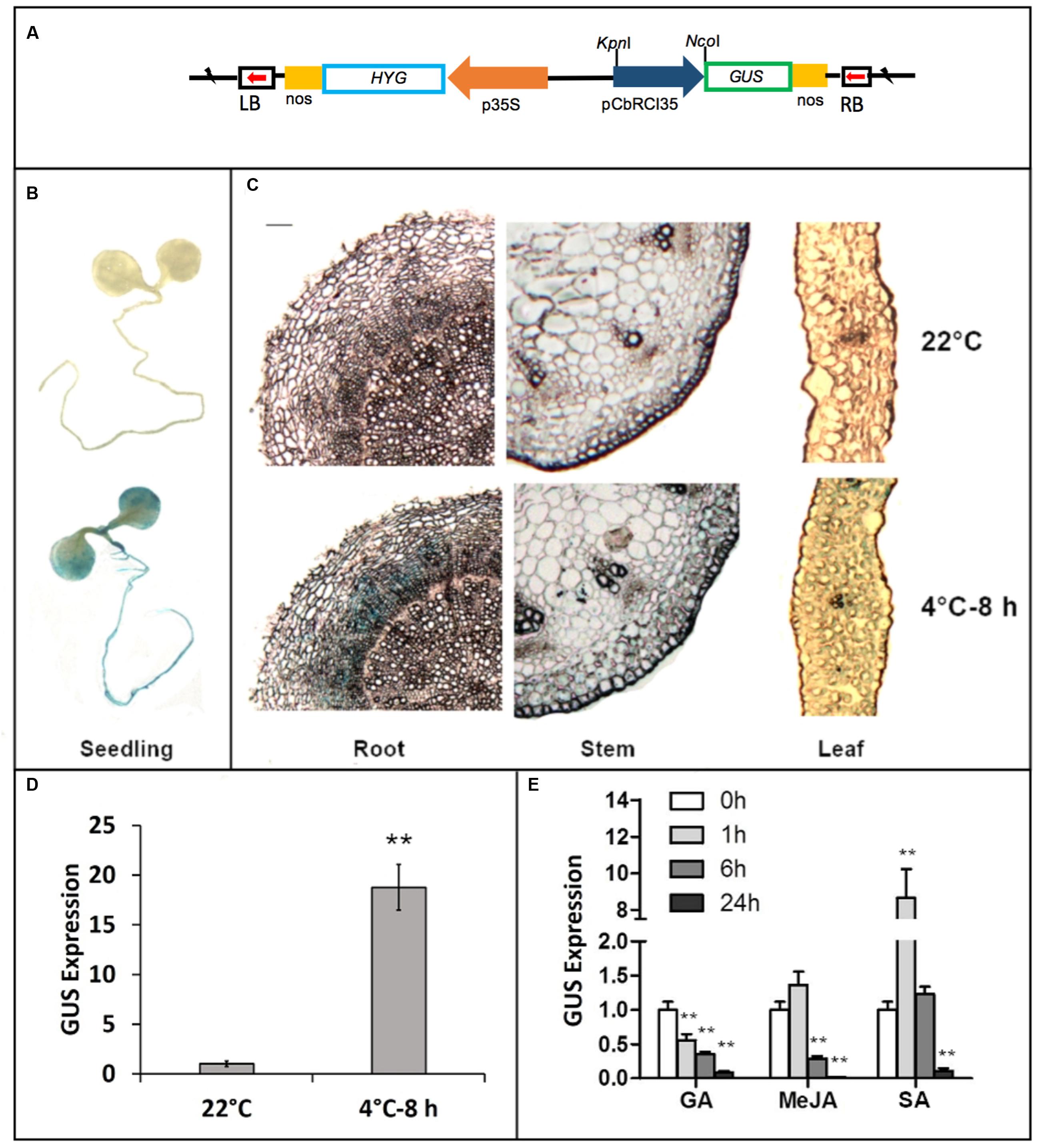
FIGURE 3. Characterization of CbRCI35 promoter activity using GUS report system and qPCR. (A) The GUS reporter gene was under the control of the CbRCI35 promoter in pCbRCI35::GUS plasmid. (B) GUS staining of 2.5-week-old transgenic Arabidopsis seedlings containing pCbRCI35::GUS fusion growing at 22°C or exposed to 4°C for 8 h. (C) Semi-thin section of leaf, stem and root tissues from 4.5-week-old transgenic seedlings containing pCbCOR15a::GUS fusion growing at 22°C or exposed to 4°C for 8 h after GUS staining. The bar represents 0.001 cm. Transcript level of GUS reporter gene driven by CbRCI35 in transgenic plants growing at 22°C was tested after exposed to 4°C for 8 h (D) or treated by GA, MeJA, or SA for time indicated (E). Actin2 gene was performed as an internal control. Data are means ± SD (n = 3, ∗∗P < 0.01).
Subcellular Localization of CbRCI35 Protein
Given the tissue-specific expression pattern of CbRCI35, it was relevant to study the subcellular localization of the CbRCI35 protein to further clarify its function. It was reported that AtRCI3, a highly homologous protein to CbRCI35, was a secreted protein and could be sorted to the cell wall (Kim et al., 2010). The online tool Plant-mPLoc3 suggested that CbRCI35 was localized in the cytoplasm. Using a transient expression assay, CbRCI35-GFP fusion protein driven by the 35S promoter was expressed in onion epidermal cells (Figure 4A). The CbRCI35-GFP promoter was distributed around the periphery of onion cells, while the signal of the GFP control was also detected in the nucleus (Figure 4B). After plasmolysis in 2 g/mL sucrose solution, the CbRCI35-GFP fusion signal was observed in the cytoplasm but not the cell wall (Figure 4C). These showed that unlike secretion of AtRCI3, CbRCI35 is localized in the cytoplasm, although the sequence similarity of these two proteins is as high as 95% (Lin et al., 2007).
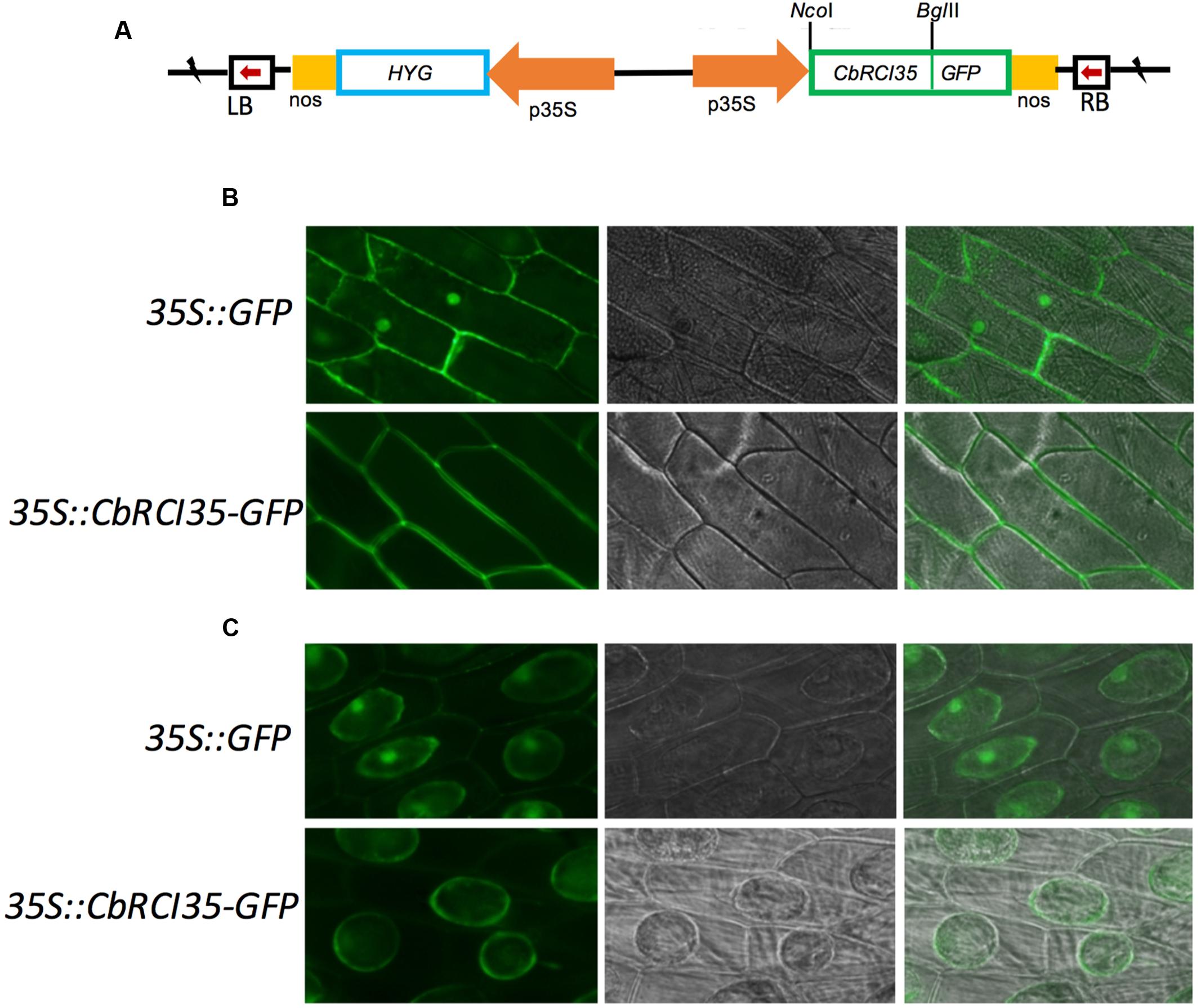
FIGURE 4. Subcellular localization of CbRCI35 protein. (A) The structure of 35S::CbRCI35-GFP fusion plasmid. (B) Subcellular localization of CbRCI35 in onion epidermis cells. (C) GFP signal in onion epidermis cells after plasmolysis in 2 g/ml sucrose solution. Photographs of cells expressing CbRCI35-GFP fusion protein and GFP only were taken in bright light to observe cell morphology (middle) and in dark-field to observe green fluorescence (left). The right ones are merged pictures.
Overexpression of CbRCI35 Enhanced Cold Tolerance in Tobacco
Next, the functional analysis of CbRCI35 was carried out in cold sensitive tobacco (N. tabacum). The CbRCI35 expression level was examined in transgenic tobacco lines. Two individual CbRCI35-ox lines (L1 and L4) exhibited high transcript levels and were therefore selected for subsequent experiments (Figure 5A). Compared with wild type (WT) and empty vector transformants (EV), L1 and L4 plants showed higher capacity of cold acclimation. WT and EV seedlings both had wilting leaves while L1 and L4 showed a robust status after 1 h of -4°C exposure followed by 2 days of recovery at 22°C (Figure 5B). Corresponding physiological analyses were performed using four indices: electrolyte leakage, malondialdehyde (MDA) content, relative water content, and glucose content – all representative indicators of cellular damage under low temperatures (Zhou et al., 2014). After freezing application, relative water contents and glucose content in L1 and L4 were much higher than that of the controls, indicating the protection of cellular water and bioactive components in transgenic plants. In addition, two CbRCI35-ox lines showed significantly lower electrolyte leakage and less MDA accumulation under both chilling and freezing stress, which was a symbol of the protection of plasma membrane integrity (Figure 5C). These phenotypically demonstrated that CbRCI35 overexpression increased freezing resistance in tobacco.
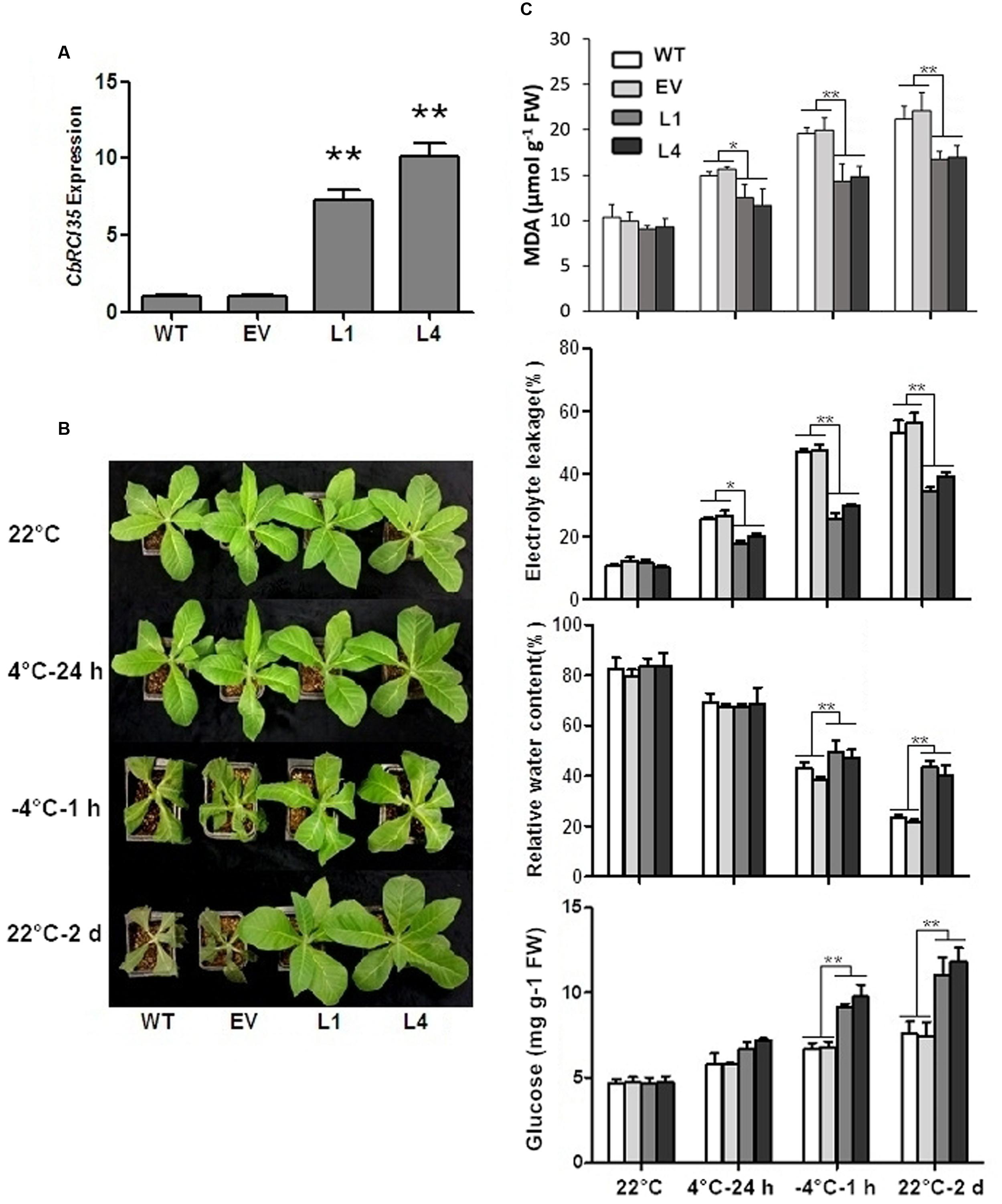
FIGURE 5. CbRCI35 enhanced cold tolerance of tobacco plants. (A) The relative expression of CbRCI35 in transgenic tobacco plants. The NtActin gene was performed as an internal control. (B) Wild type (WT), empty vector control (EV) and CbRCI35-ox lines L1, L2 were grown at 22°C. After treated at 4°C for 24 h, at -4°C for 1 h, and at 22°C for a 2-day recovery, plants phenotypes were shown. They were representatives of their respective types of tobacco plants. (C) The malondialdehyde (MDA) content, electrolyte leakage, relative water content and glucose content of tobacco leaves before and after cold application. Error bars indicate SD (n = 3, ∗P < 0.05, ∗∗P < 0.01).
Moreover, the impact of CbRCI35 on endogenous cold responsive signaling genes in tobacco was investigated. NtDREB1 and NtDREB3 belong to CBF/DREB transcription factor family, a group of key cold responsive regulators (Catala et al., 2003; Zhou et al., 2012). NtERD10a and NtERD10b are downstream CBF/DREB regulons modulated by DREBs (Kasuga et al., 2004). In normal temperatures, NtDREB3 of L1 and L4 exhibited significantly higher transcript levels than WT or EV. NtERD10a as well as NtERD10b were also upregulated (Figure 6). During treatment in 4°C, NtDREB3 expression levels of transformants were still much higher than the controls, although they remained similar compared to NtDREB3 expression at 22°C. NtERD10a and NtERD10b were remarkably induced by cold and showed much higher transcripts in L1 and L4. NtDREB1 did not exhibit obviously different expression levels from WT and EV. It can be concluded that CbRCI35 positively regulated NtDREB3. NtERD10a, and NtERD10b expression, and conferred enhanced cold induction of these two ERD10 genes in cold temperature.
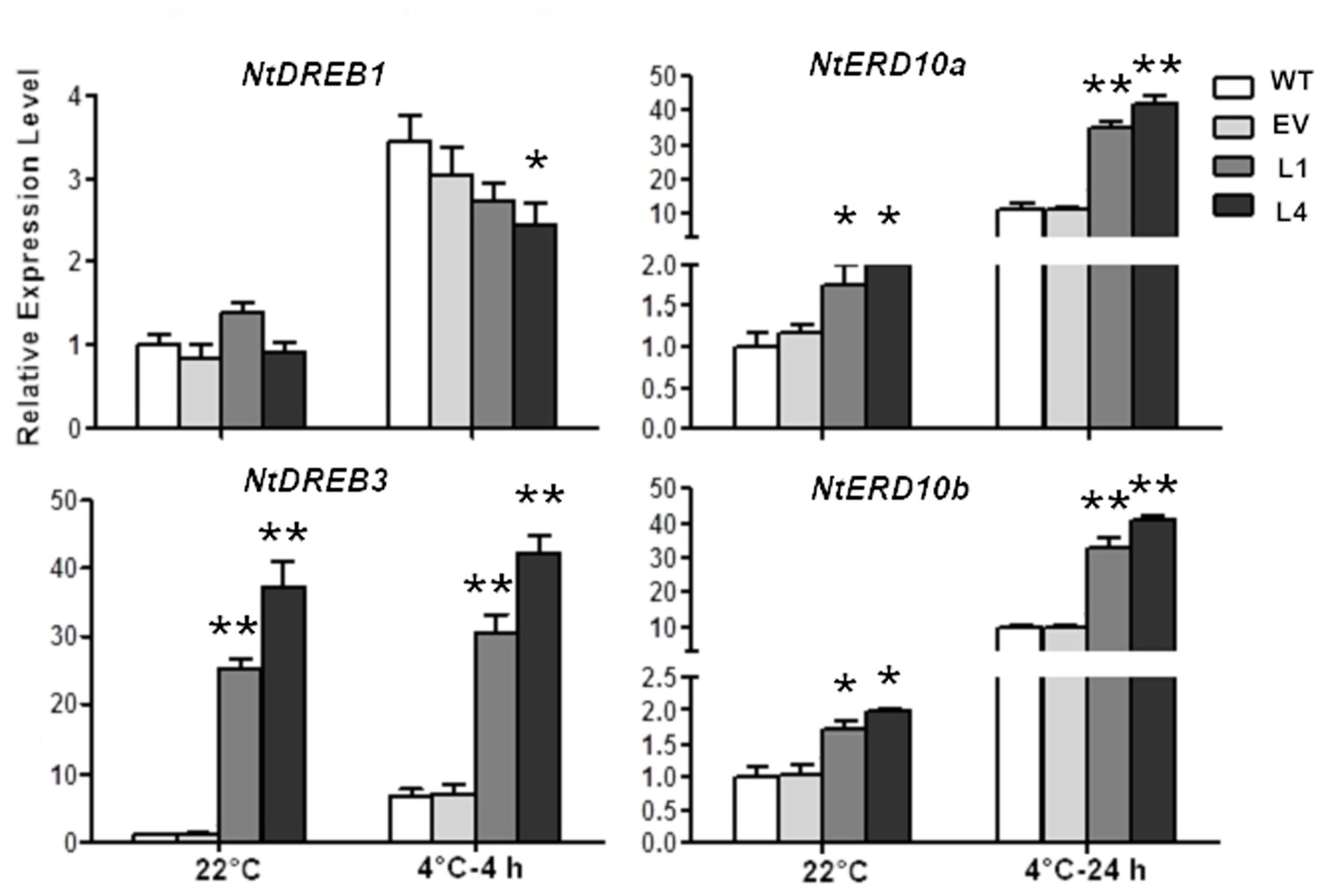
FIGURE 6. CbRCI35 activated cold responsive genes in tobacco plants. The relative expression of NtDREB1. NtDREB3. NtERD10a, and NtERD10b in CbRCI35-ox tobacco plants before and after cold treatments. The NtActin gene was performed as an internal control. Data are means ± SD (n = 3, ∗P < 0.05, ∗∗P < 0.01).
Overexpression of CbRCI35 Modulated ROS Homeostasis and Altered ROS Metabolic Gene Expression in Tobacco
Predicted as a peroxidase, CbRCI35 is supposed to regulate ROS levels in plants. Using the dichloro-dihydro-fluorescein diacetate (DCFH-DA) assay, we verified that two CbRCI35-ox tobacco lines exhibited moderately elevated ROS levels before exposure to cold temperatures compared to WT plants. After exposure to 4°C for 24 h, the ROS levels in each tobacco were similar (Figure 7A). As a representative component of ROS (Slesak et al., 2007), H2O2 showed content changes that were in line with DCFH-DA results (Figure 7B). We also detected the activity of SOD, a primary antioxidant enzyme in plants (Price et al., 1994). In both normal and chilling conditions, CbRCI35-ox tobacco showed higher SOD activity than WT, which can contribute to the protection of cell structure (Figure 7C). The increase of ROS at the basal level together with the stronger SOD activity after cold exposure indicated the effects of CbRCI35 in plant ROS regulation and cell protection. We further examined the expression of ROS metabolic genes in transgenic tobacco. Intriguingly, ROS scavenging and biosynthesis genes were both disrupted (Figure 8). In 22°C, transcripts of a key ROS scavenging gene NtAPX (Yan et al., 2014) were significantly lower in CbRCI35-ox plants, while that of NtSOD, another ROS detoxifying gene (Liu et al., 2016), was higher than controls. After 24 h of 4°C application, expression levels of the ROS scavenging genes NtAPX. NtCAT, and NtGST were noticeably lower in CbRCI35-ox plants. Meanwhile, ROS production modulators NtRBOHD1 and NtRBOHD2 were downregulated by CbRCI35 under normal temperature, and the cold induction of NtRBOHD1 was also significantly blocked in CbRCI35-ox tobacco (Figure 8). Taken cumulatively, these revealed that the overexpression of CbRCI35 regulates ROS homeostasis in tobacco.
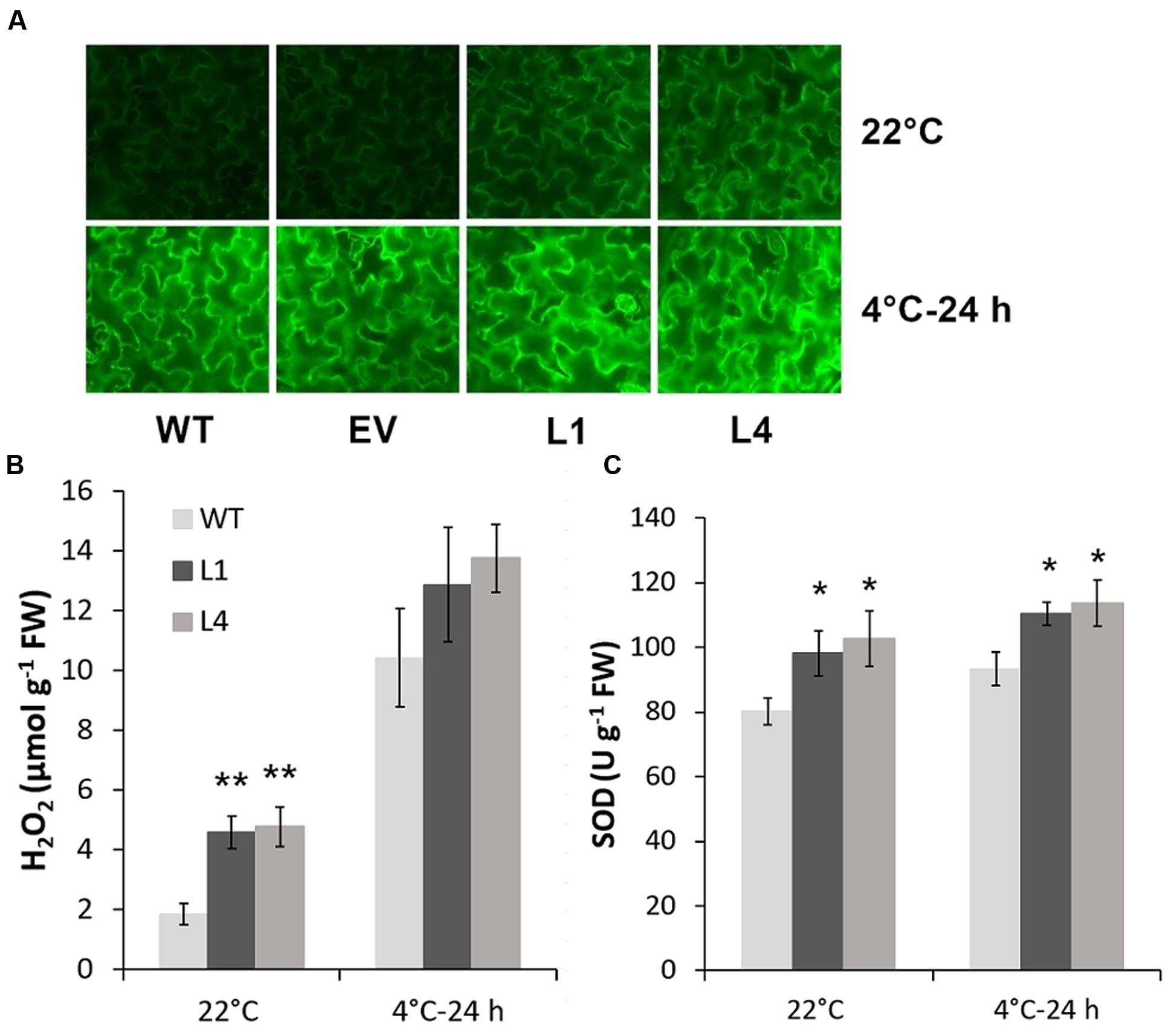
FIGURE 7. CbRCI35 elevated tobacco ROS level in normal condition and increased SOD activity after chilling treatment. (A) ROS levels detected by Dichloro-dihydro-fluorescein diacetate (DCFH-DA) assay in 4-week-old tobacco leaf disks with or without 4°C treatment. H2O2 contents and SOD activity in tobacco leaves of WT and CbRCI35-ox lines (L1 and L4) were exhibited in (B) and (C), respectively. Error bars indicate SD (n = 3, ∗P < 0.05, ∗∗P < 0.01).
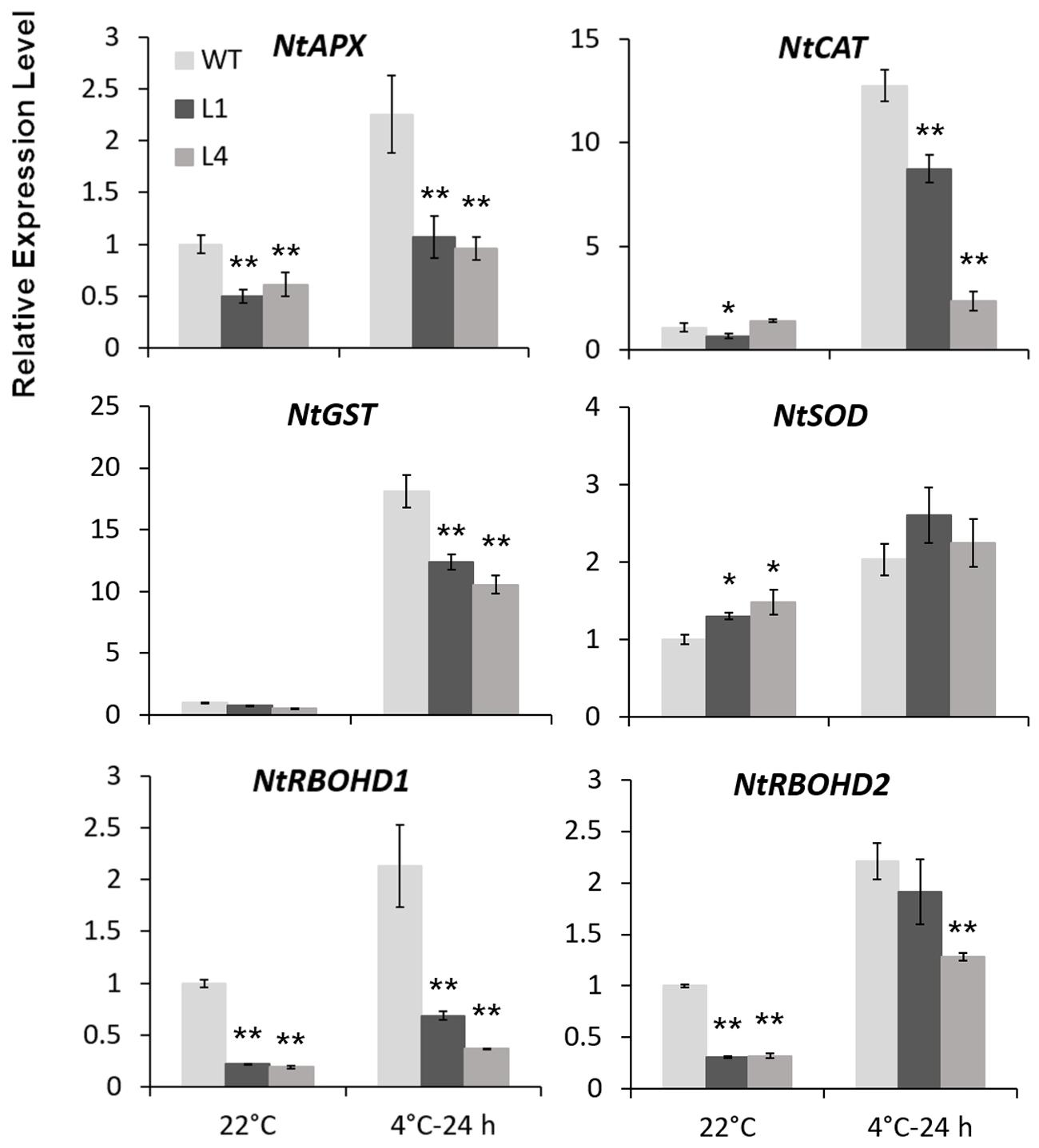
FIGURE 8. CbRCI35 altered ROS homeostasis related genes expression. Relative expression levels of ROS scavenging genes including NtAPX. NtCAT. NtGST, and NtSOD, as well as ROS biosynthesis genes NtRBOHD1 and NtRBOHD2 in CbRCI35-ox tobacco plants with or without 4°C treatment. The NtActin gene was performed as an internal control. Expression levels were relative to that of WT plants. Data are means ± SD (n = 3, ∗P < 0.05, ∗∗P < 0.01).
Discussion
The RCI genes are a group of cold responsive genes identified using Arabidopsis cDNA library screening (Jarillo et al., 1994). These genes consist of several different categories. Unlike AtRCI1A/B or AtRCI2A/B. AtRCI3 is a type III peroxidase gene in Arabidopsis (Medina et al., 2001; Sivankalyani et al., 2015). With a high similarity to AtRCI3. CbRCI35 also shows an obvious cold-inducible expression pattern and high transcription level in roots (Figures 1A,B). Interestingly, these two genes behave differently in multiple aspects. During cold response, AtRCI3 transcription is gradually elevated and attained the maximal level after 24 h of 4°C exposure (Llorente et al., 2002). CbRCI35 responds to the same temperature with a peak of expression levels at 8 h after treatment and returned to a low level at the 24 h time point (Figure 1B), indicating its faster activation and potential function in the earlier stage of response to low temperature. For organ dependent expression, AtRCI3 shows a root specific transcription while CbRCI35 has a high expression level in roots but can also be induced in leaves and stems (Figure 1B). Moreover, AtRCI3 is expressed not only in the cortex but also in the stele (Llorente et al., 2002), while CbRCI35 transcription is restricted to the cortex in roots (Figure 3C). These data reveal the diverse expressional regulation of type III peroxidase genes from different plant species. Coordinately, the AtRCI3 protein is detected in the endoplasmic reticulum (ER) and can be secreted to the cell wall (Kim et al., 2010), while the CbRCI35 protein is localized in the cytoplasm (Figures 4B,C). The distinct transcription pattern and protein localization implies that CbRCI35 from hardy plant species of C. bursa-pastoris may have unique functions distinct from AtRCI3. Our work provides a novel insight into the role of AtRCI3-like type III peroxidase in regulating cold tolerance of plants.
Using a cold sensitive species of tobacco, we characterized the molecular function of CbRCI35 and observed the moderately increased ROS level in transgenic plants at the normal temperature (Figures 7A,B). ROS production and scavenging plays a key role in plant cold acclimation (Del Rio, 2015). Plenty of regulators of ROS homeostasis jointly contribute to the control of ROS level and signal transduction (Mangano et al., 2016). Given the widely reported ROS scavengers during stress response in plants, few positive modulators of cold resistance that can augment ROS accumulation have been documented. In our case, overexpression of CbRCI35 participates in the increase of ROS under normal conditions, which is similar to AtRCI3 (Kim et al., 2010). The representative well-known genes regulating ROS biosynthesis (NtRBOHD1 and NtRBOHD2) and scavenging (NtAPX and NtCAT) were repressed, while NtSOD expression was slightly enhanced in CbRCI35-ox seedlings (Figure 8), suggesting that CbRCI35 may contribute to ROS accumulation through RBOHD1/2-independent pathways in tobacco. The increase of NtSOD transcripts can be due to a feedback activation mechanism. In response to chilling treatment, most of these genes were negatively regulated in transgenic tobacco compared with WT control (Figure 8). As a result, the ROS level was similar to the control (Figures 7A,B), revealing that overexpression of CbRCI35 reformed the transcriptional control of ROS metabolic genes to facilitate the homeostatic mechanism of ROS. In addition, the SOD activity was higher in transgenic plants in both the warm and chilling conditions, indicating that CbRCI35 might participate in the protection of bioactive enzymes under cold stress. Although the total ROS level was not lowered, the CbRCI35-ox tobacco plants showed alleviated membrane damage suggested by MDA content and electrolyte leakage in chilling temperatures (Figures 7A,B). Together with the significantly increased freezing resistance caused by CbRCI35, it can be concluded that CbRCI35 enhanced plant cold acclimation through controlling the ROS homeostasis and activating downstream cold responsive genes. Further, no growth retardation was observed in CbRCI35-ox transgenic plants, demonstrating the broad prospects of CbRCI35 application in plant breeding for crop improvements.
Author Contributions
JL, MZ, and WL were responsible for the overall experimental design and conduct of the experiments. MZ performed the cold induction test, promoter isolation, and tobacco transformation. JL and MZ took the lead on manuscript development. WL and YZ conducted the gene expressional analysis, promoter activity investigation, protein localization detection, and transgenic tobacco characterization. PL and XY contributed to experimental design and data analyses. All authors read and approved the final manuscript.
Conflict of Interest Statement
The authors declare that the research was conducted in the absence of any commercial or financial relationships that could be construed as a potential conflict of interest.
Acknowledgment
This study was supported by the Natural Science Foundation of China (31370346), the National Key Technology R&D Program (2009BADA8B04) and the National High Technology Research and Development Program of China (2008AA10Z105).
Footnotes
- ^http://bioinformatics.psb.ugent.be/webtools/plantcare/html/
- ^http://bioinformatics.psb.ugent.be/webtools/plantcare/html/
- ^http://www.csbio.sjtu.edu.cn/bioinf/plant-multi/
References
Abat, J. K., and Deswal, R. (2009). Differential modulation of S-nitrosoproteome of Brassica juncea by low temperature: change in S-nitrosylation of Rubisco is responsible for the inactivation of its carboxylase activity. Proteomics 9, 4368–4380. doi: 10.1002/pmic.200800985
Beike, A. K., Lang, D., Zimmer, A. D., Wust, F., Trautmann, D., Wiedemann, G., et al. (2015). Insights from the cold transcriptome of Physcomitrella patens: global specialization pattern of conserved transcriptional regulators and identification of orphan genes involved in cold acclimation. New Phytol. 205, 869–881. doi: 10.1111/nph.13004
Cantrel, C., Vazquez, T., Puyaubert, J., Reze, N., Lesch, M., Kaiser, W. M., et al. (2011). Nitric oxide participates in cold-responsive phosphosphingolipid formation and gene expression in Arabidopsis thaliana. New Phytol. 189, 415–427. doi: 10.1111/j.1469-8137.2010.03500.x
Catala, R., Santos, E., Alonso, J. M., Ecker, J. R., Martinez-Zapater, J. M., and Salinas, J. (2003). Mutations in the Ca2+/H+ transporter CAX1 increase CBF/DREB1 expression and the cold-acclimation response in Arabidopsis. Plant Cell 15, 2940–2951. doi: 10.1105/tpc.015248
Del Rio, L. A. (2015). ROS and RNS in plant physiology: an overview. J. Exp. Bot. 66, 2827–2837. doi: 10.1093/jxb/erv099
Draper, H. H., Squires, E. J., Mahmoodi, H., Wu, J., Agarwal, S., and Hadley, M. (1993). A comparative-evaluation of thiobarbituric acid methods for the determination of malondialdehyde in biological-materials. Free Radic. Biol. Med. 15, 353–363. doi: 10.1016/0891-5849(93)90035-S
Ezaki, B., Gardner, R. C., Ezaki, Y., and Matsumoto, H. (2000). Expression of aluminum-induced genes in transgenic Arabidopsis plants can ameliorate aluminum stress and/or oxidative stress. Plant Physiol. 122, 657–665. doi: 10.1104/pp.122.3.657
Farnese, F. S., Menezes-Silva, P. E., Gusman, G. S., and Oliveira, J. A. (2016). When bad guys become good ones: the key role of reactive oxygen species and nitric oxide in the plant responses to abiotic stress. Front. Plant Sci. 7:471. doi: 10.3389/fpls.2016.00471
Hossain, M. A., Bhattacharjee, S., Armin, S. M., Qian, P., Xin, W., Li, H. Y., et al. (2015). Hydrogen peroxide priming modulates abiotic oxidative stress tolerance: insights from ROS detoxification and scavenging. Front. Plant Sci. 6:420. doi: 10.3389/fpls.2015.00420
Hung, S. H., Wang, C. C., Ivanov, S. V., Alexieva, V., and Yu, C. W. (2007). Repetition of hydrogen peroxide treatment induces a chilling tolerance comparable to cold acclimation in mung bean. J. Am. Soc. Hortic. Sci. 132, 770–776.
Iseri, O. D., Korpe, D. A., Sahin, F. I., and Haberal, M. (2013). Hydrogen peroxide pretreatment of roots enhanced oxidative stress response of tomato under cold stress. Acta Physiol. Plant. 35, 1905–1913. doi: 10.1007/s11738-013-1228-7
Jarillo, J. A., Capel, J., Leyva, A., Martinezzapater, J. M., and Salinas, J. (1994). 2 Related low-temperature-inducible genes of Arabidopsis encode proteins showing high homology to 14-3-3-Proteins, a family of putative kinase regulators. Plant Mol. Biol. 25, 693–704. doi: 10.1007/BF00029607
Jiang, M., and Zhang, J. (2001). Effect of abscisic acid on active oxygen species, antioxidative defence system and oxidative damage in leaves of maize seedlings. Plant Cell Physiol. 42, 1265–1273. doi: 10.1093/pcp/pce162
Kasuga, M., Miura, S., Shinozaki, K., and Yamaguchi-Shinozaki, K. (2004). A combination of the Arabidopsis DREB1A gene and stress-inducible rd29A promoter improved drought- and low-temperature stress tolerance in tobacco by gene transfer. Plant Cell Physiol. 45, 346–350. doi: 10.1093/pcp/pch037
Kim, M. J., Ciani, S., and Schachtman, D. P. (2010). A peroxidase contributes to ROS production during Arabidopsis root response to potassium deficiency. Mol. Plant 3, 420–427. doi: 10.1093/mp/ssp121
Kvaratskhelia, M., Winkel, C., and Thorneley, R. N. F. (1997). Purification and characterization of a novel class III peroxidase isoenzyme from tea leaves. Plant Physiol. 114, 1237–1245. doi: 10.1104/pp.114.4.1237
Lai, L. S., Wang, D. J., Chang, C. T., and Wang, C. H. (2006). Catalytic characteristics of peroxidase from wheat grass. J. Agric. Food Chem. 54, 8611–8616. doi: 10.1021/jf060888w
Lamotte, O., Bertoldo, J. B., Besson-Bard, A., Rosnoblet, C., Aime, S., Hichami, S., et al. (2014). Protein S-nitrosylation: specificity and identification strategies in plants. Front. Chem. 2:114.
Lin, J., Zhang, W., Zhou, X., Wang, X., Shi, M., Sun, X., et al. (2007). Molecular cloning and characterization of cold-responsive gene Cbrci35 from Capsella bursa-pastoris. Biologia 62, 690–696. doi: 10.2478/s11756-007-0145-x
Liu, H., Wang, Y., Zhou, X., Wang, C., Fu, J., and Wei, T. (2016). Overexpression of a harpin-encoding gene popW from Ralstonia solanacearum primed antioxidant defenses with enhanced drought tolerance in tobacco plants. Plant Cell Rep. 35, 1333–1344. doi: 10.1007/s00299-016-1965-3
Llorente, F., Lopez-Cobollo, R. M., Catala, R., Martinez-Zapater, J. M., and Salinas, J. (2002). A novel cold-inducible gene from Arabidopsis, RCI3, encodes a peroxidase that constitutes a component for stress tolerance. Plant J. 32, 13–24. doi: 10.1046/j.1365-313X.2002.01398.x
Mangano, S., Juarez, S. P., and Estevez, J. M. (2016). ROS regulation of polar growth in plant cells. Plant Physiol. 171, 1593–1605.
Mckersie, B. D., Chen, Y. R., Debeus, M., Bowley, S. R., Bowler, C., Inze, D., et al. (1993). Superoxide-dismutase enhances tolerance of freezing stress in transgenic alfalfa (Medicago-Sativa L). Plant Physiol. 102, 85–85.
Medina, J., Catala, R., and Salinas, J. (2001). Developmental and stress regulation of RCI2A and RCI2B, two cold-inducible genes of Arabidopsis encoding highly conserved hydrophobic proteins. Plant Physiol. 125, 1655–1666. doi: 10.1104/pp.125.4.1655
Mengel, A., Chaki, M., Shekariesfahlan, A., and Lindermayr, C. (2013). Effect of nitric oxide on gene transcription - S-nitrosylation of nuclear proteins. Front. Plant Sci. 4:293. doi: 10.3389/fpls.2013.00293
Mishra, R. C., Ghosh, R., and Bae, H. (2016). Plant acoustics: in the search of a sound mechanism for sound signaling in plants. J. Exp. Bot. 67, 4483–4494. doi: 10.1093/jxb/erw235
Mittler, R. (2002). Oxidative stress, antioxidants and stress tolerance. Trends Plant Sci. 7, 405–410. doi: 10.1016/S1360-1385(02)02312-9
Mittler, R., Vanderauwera, S., Gollery, M., and Van Breusegem, F. (2004). Reactive oxygen gene network of plants. Trends Plant Sci. 9, 490–498. doi: 10.1016/j.tplants.2004.08.009
Narusaka, Y., Narusaka, M., Seki, M., Umezawa, T., Ishida, J., Nakajima, M., et al. (2004). Crosstalk in the responses to abiotic and biotic stresses in Arabidopsis: analysis of gene expression in cytochrome P450 gene superfamily by cDNA microarray. Plant Mol. Biol. 55, 327–342. doi: 10.1007/s11103-004-0685-1
Navabpour, S., Morris, K., Allen, R., Harrison, E., A-H-Mackerness, S., and Buchanan-Wollaston, V. (2003). Expression of senescence-enhanced genes in response to oxidative stress. J. Exp. Bot. 54, 2285–2292. doi: 10.1093/jxb/erg267
Neill, S. J., Desikan, R., Clarke, A., Hurst, R. D., and Hancock, J. T. (2002). Hydrogen peroxide and nitric oxide as signalling molecules in plants. J. Exp. Bot. 53, 1237–1247. doi: 10.1093/jexbot/53.372.1237
Petrov, V. D., and Van Breusegem, F. (2012). Hydrogen peroxide-a central hub for information flow in plant cells. AoB Plants 2012, ls014. doi: 10.1093/aobpla/pls014
Potters, G., Pasternak, T. P., Guisez, Y., Palme, K. J., and Jansen, M. A. (2007). Stress-induced morphogenic responses: growing out of trouble? Trends Plant Sci. 12, 98–105. doi: 10.5363/tits.12.12_98
Price, A. H., Taylor, A., Ripley, S. J., Griffiths, A., Trewavas, A. J., and Knight, M. R. (1994). Oxidative signals in tobacco increase cytosolic calcium. Plant Cell 6, 1301–1310. doi: 10.1105/tpc.6.9.1301
Shigeto, J., and Tsutsumi, Y. (2016). Diverse functions and reactions of class III peroxidases. New Phytol. 209, 1395–1402. doi: 10.1111/nph.13738
Sivankalyani, V., Geetha, M., Subramanyam, K., and Girija, S. (2015). Ectopic expression of Arabidopsis RCI2A gene contributes to cold tolerance in tomato. Transgenic Res. 24, 237–251. doi: 10.1007/s11248-014-9840-x
Slesak, I., Libik, M., Karpinska, B., Karpinski, S., and Miszalski, Z. (2007). The role of hydrogen peroxide in regulation of plant metabolism and cellular signalling in response to environmental stresses. Acta Biochim. Pol. 54, 39–50.
Thomashow, M. F. (1999). PLANT COLD ACCLIMATION: freezing tolerance genes and regulatory mechanisms. Annu. Rev. Plant Physiol. Plant Mol. Biol. 50, 571–599. doi: 10.1146/annurev.arplant.50.1.571
Tognolli, M., Penel, C., Greppin, H., and Simon, P. (2002). Analysis and expression of the class III peroxidase large gene family in Arabidopsis thaliana. Gene 288, 129–138. doi: 10.1016/S0378-1119(02)00465-1
Wang, Y., Li, J. L., Wang, J. Z., and Li, Z. K. (2010). Exogenous H2O2 improves the chilling tolerance of manilagrass and mascarenegrass by activating the antioxidative system. Plant Growth Regul. 61, 195–204. doi: 10.1007/s10725-010-9470-0
Wu, L., Zhou, M., Shen, C., Liang, J., and Lin, J. (2012). Transgenic tobacco plants over expressing cold regulated protein CbCOR15b from Capsella bursa-pastoris exhibit enhanced cold tolerance. J. Plant Physiol. 169, 1408–1416. doi: 10.1016/j.jplph.2012.05.016
Yan, H., Jia, H., Chen, X., Hao, L., An, H., and Guo, X. (2014). The cotton WRKY transcription factor GhWRKY17 functions in drought and salt stress in transgenic Nicotiana benthamiana through ABA signaling and the modulation of reactive oxygen species production. Plant Cell Physiol. 55, 2060–2076. doi: 10.1093/pcp/pcu133
Yu, C. W., Murphy, T. M., and Lin, C. H. (2003). Hydrogen peroxide-induced chilling tolerance in mung beans mediated through ABA-independent glutathione accumulation. Funct. Plant Biol. 30, 955–963. doi: 10.1071/FP03091
Yue, C., Cao, H. L., Wang, L., Zhou, Y. H., Huang, Y. T., Hao, X. Y., et al. (2015). Effects of cold acclimation on sugar metabolism and sugar-related gene expression in tea plant during the winter season. Plant Mol. Biol. 88, 591–608. doi: 10.1007/s11103-015-0345-7
Zhao, M. G., Chen, L., Zhang, L. L., and Zhang, W. H. (2009). Nitric reductase-dependent nitric oxide production is involved in cold acclimation and freezing tolerance in Arabidopsis. Plant Physiol. 151, 755–767. doi: 10.1104/pp.109.140996
Zhou, M., Wu, L., Liang, J., Shen, C., and Lin, J. (2012). Cold-induced modulation of CbICE53 gene activates endogenous genes to enhance acclimation in transgenic tobacco. Mol. Breed. 30, 1611–1620. doi: 10.1007/s11032-012-9744-5
Keywords: CbRCI35, cold tolerance, gene expression, ROS, tobacco
Citation: Zhou M, Li W, Zheng Y, Lin P, Yao X and Lin J (2016) CbRCI35, a Cold Responsive Peroxidase from Capsella bursa-pastoris Regulates Reactive Oxygen Species Homeostasis and Enhances Cold Tolerance in Tobacco. Front. Plant Sci. 7:1599. doi: 10.3389/fpls.2016.01599
Received: 16 August 2016; Accepted: 10 October 2016;
Published: 21 October 2016.
Edited by:
Chandrashekhar Pralhad Joshi, Michigan Technological University, USAReviewed by:
Dmitry A. Los, Timiryazev Institute of Plant Physiology (RAS), RussiaMohammad Anwar Hossain, Bangladesh Agricultural University, Bangladesh
Copyright © 2016 Zhou, Li, Zheng, Lin, Yao and Lin. This is an open-access article distributed under the terms of the Creative Commons Attribution License (CC BY). The use, distribution or reproduction in other forums is permitted, provided the original author(s) or licensor are credited and that the original publication in this journal is cited, in accordance with accepted academic practice. No use, distribution or reproduction is permitted which does not comply with these terms.
*Correspondence: Juan Lin, bGluanVhbkBmdWRhbi5lZHUuY24=
†These authors have contributed equally to this work.