- 1Laboratory of Molecular Biology, Department of Plant Sciences, Graduate School Experimental Plant Sciences, Wageningen University, Wageningen, Netherlands
- 2Sainsbury Laboratory, University of Cambridge, Cambridge, UK
Symbiotic bacteria (rhizobia) are maintained and conditioned to fix atmospheric nitrogen in infected cells of legume root nodules. Rhizobia are confined to the asymmetrical protrusions of plasma membrane (PM): infection threads (IT), cell wall-free unwalled droplets and symbiosomes. These compartments rapidly increase in surface and volume due to the microsymbiont expansion, and remarkably, the membrane resources of the host cells are targeted to interface membrane quite precisely. We hypothesized that the change in the membrane tension around the expanding microsymbionts creates a vector for membrane traffic toward the symbiotic interface. To test this hypothesis, we selected calcium sensors from the group of synaptotagmins: MtSyt1, Medicago truncatula homolog of AtSYT1 from Arabidopsis thaliana known to be involved in membrane repair, and two other homologs expressed in root nodules: MtSyt2 and MtSyt3. Here we show that MtSyt1, MtSyt2, and MtSyt3 are expressed in the expanding cells of the meristem, zone of infection and proximal cell layers of zone of nitrogen fixation (MtSyt1, MtSyt3). All three GFP-tagged proteins delineate the interface membrane of IT and unwalled droplets and create a subcompartments of PM surrounding these structures. The localization of MtSyt1 by EM immunogold labeling has shown the signal on symbiosome membrane and endoplasmic reticulum (ER). To specify the role of synaptotagmins in interface membrane formation, we compared the localization of MtSyt1, MtSyt3 and exocyst subunit EXO70i, involved in the tethering of post-Golgi secretory vesicles and operational in tip growth. The localization of EXO70i in root nodules and arbusculated roots was strictly associated with the tips of IT and the tips of arbuscular fine branches, but the distribution of synaptotagmins on membrane subcompartments was broader and includes lateral parts of IT, the membrane of unwalled droplets as well as the symbiosomes. The double silencing of synaptotagmins caused a delay in rhizobia release and blocks symbiosome maturation confirming the functional role of synaptotagmins. In conclusion: synaptotagmin-dependent membrane fusion along with tip-targeted exocytosis is operational in the formation of symbiotic interface.
Introduction
The legume-rhizobium and plant-arbuscular mycorrhizal symbioses are rare examples in the plant kingdom of intracellular microbes are being tolerated by the host cell for the long periods up to several weeks. Accommodation of microsymbionts causes profound morphological changes of host cell. During the entrance of infection thread to the host cell the division of bacterial cells within a physically confined space provides a “push” mechanism for entry, which is able to counteracting the turgor pressure of the host plant cell (Brewin, 2004). The entrance of microsymbionts triggers the formation of symbiosis-specific asymmetric protrusions of plasma membrane (PM). In legume root nodules such protrusions are enveloping tubular structures called infection threads (IT), infection droplets (unwalled extensions of ITs) and symbiosomes (released bacteria surrounded by a host cell-derived membrane; Roth and Stacey, 1989; Brewin, 2004; Gibson et al., 2008; Kondorosi et al., 2013). In symbiosis with arbuscular mycorrhiza PM protrusions envelop the intracellular branched hyphae called arbuscules (Parniske, 2000; Gutjahr and Parniske, 2013). It is remarkable that the membrane resources of the host cells are targeted to interface membrane surrounding these symbiotic compartments quite precisely in time and space, ensuring, for example, the tip growth of ITs and arbuscules and isodiametric expansion for symbiosomes and unwalled droplets. Till now the mechanisms of such meticulously correct delivery are not known.
The PM is known to be inelastic, unable to stretch more than 3% (Apodaca, 2002). Exocytosis of new membrane material is therefore crucial for the increase in membrane surface area (Grefen et al., 2011). In animal cells, a local increase in membrane tension induces membrane repair mechanisms in that specific region of the membrane (Morris and Homann, 2001). The response of the cells to mechanical stress involves Ca2+ spiking, as well as phospholipase signaling, rapid remodeling of the actin skeleton and quick retargeting of membrane resources to reduce the membrane tension (Jaffe et al., 2002; Telewski, 2006). The membrane fusion is achieved by the action of N-ethylmaleimide sensitive factor attachment protein receptors (t-SNAREs), as well as to the vesicle-associated membrane protein (VAMP or v-SNAREs) forming a SNARE complex in a Ca2+ dependent process (Adolfsen et al., 2004; Maximov et al., 2009; Falkowski et al., 2011). Local higher concentration of cytoplasmic calcium is required for the targeted vesicular fusion, but the SNARE complex lacks specific Ca2+-binding sites. This highlights the crucial role of calcium sensors like synaptotagmins in the process of locally targeted membrane fusion (Reddy et al., 2001; McNeil and Kirchhausen, 2005; Idone et al., 2008; Gauthier et al., 2009). Synaptotagmins are able to bind to t-SNAREs as well as to v-SNAREs hence the association of SNAREs with synaptotagmin may provide Ca2+ sensitivity to direct the rapid response that is needed for the fusion of the membrane vesicles with the region of the overstretched (Draeger et al., 2011).
Synaptotagmins have been found in plants (Craxton, 2004; Nakagawa et al., 2007; Schapire et al., 2008; Yamazaki et al., 2008, 2010). In Arabidopsis thaliana AtSYT1 is involved in membrane repair in the case of osmotic misbalance or cold stress (Schapire et al., 2008; Yamazaki et al., 2008, 2010). Recently is was found that AtSYT1 is a plant homolog of the mammalian extended synaptotagmins and on the membrane it is enriched on the ER-PM contact sites (Manford et al., 2012; Levy et al., 2015; Pérez-Sancho et al., 2015). AtSYT1 is preferentially localized to ER–PM contact sites also modulating the abundance of PEN1, a component of SNARE membrane fusion complex of PM (Kim et al., 2016).
The expansion of PM in plants depends on targeted exocytosis; the classical examples are the tip growth of root hairs and pollen tubes (Hepler and Winship, 2010; Qin and Dong, 2015) and the formation of the cell plate during cell division (El Kasmi et al., 2013). Tip growth is concomitant with the establishment of a tip-focused Ca2+ gradient, as well as with actin microfilament reorganization and redirection of small GTPases of the Rab, Rop and ARF families involved in membrane fusion (Ketelaar et al., 2003; Šamaj et al., 2006). The tethering of post-Golgi vesicles to the fast growing membrane subcompartments on the tip is regulated by the PM protein complex exocyst (He and Guo, 2009; Zárský et al., 2013; Zhang et al., 2015; Vukašinović et al., 2016).
The growth of symbiotic structures in infected cells is anisodiametric for infection threads and arbuscules, and isodiametric for unwalled droplets and symbiosomes. That means that the membrane resources have to be targeted to support the expansion indiscriminately of the type of growth. During endosymbiosis establishment the mechanical pressure over the host PM considered to be a potential signal monitored by plants (Hardham et al., 2008; Chehab et al., 2009; Jayaraman et al., 2014). We hypothesized that the membrane tension caused by expanding microsymbionts creates a spatial vector for membrane fusion to the interface membrane ensuring the enlargement of the symbiotic interface.
To test this hypothesis, we studied MtSyt1, M. truncatula homolog of AtSYT1 shown to be involved in membrane repair (Schapire et al., 2008; Yamazaki et al., 2008) and two other homologs expressed in root nodules: MtSyt2 and MtSyt3. The expression analysis, the localization of GFP-tagged synaptotagmins and functional analysis of the nodules from the roots carrying double silencing constructs shows that synaptotagmins MtSyt1, MtSyt2, and MtSyt3 are co-opted into the process of intracellular accommodation of microsymbionts and involved in the formation of interface membrane. Thereafter the synaptotagmin-dependent membrane fusion and putative membrane repair pathway along with tip-targeted exocytosis is operational in the formation of symbiotic interface.
Materials and Methods
Plant Materials, Transformation, and Inoculation
The Medicago truncatula accession Jemalong A17 was grown in perlite saturated with Färhaeus medium without nitrate in a growth chamber at 21°C and 16/8-h light/darkness cycle. These plants were inoculated with Sinorhizobium meliloti strain Sm2011 (OD600 0.1, 2 mL per plant). Root nodules were collected for analysis 14 days post inoculation (dpi). Agrobacterium rhizogenes MSU440-mediated hairy root transformation was performed according to Limpens et al. (2005).
Cloning
DNA sequences of M. truncatula synaptotagmins named MtSyt1, MtSyt2 and MtSyt3 were retrieved by BLAST based on homology with Arabidopsis SYT1 (Schapire et al., 2008). MtSyt1, MtSyt2 and MtSyt3 genes and their putative promoters were amplified by PCR on M. truncatula cDNA genomic DNA, respectively, using Phusion High-Fidelity DNA Polymerase (Finnzymes) and specific primers (Supplementary Table 1). PCR fragments were introduced in pENTR-D-TOPO (Invitrogen) and sequenced. Each promoter was further re-cloned into two different pENTR 4-1 vectors (Invitrogen); one is with GFP and the other without GFP (Invitrogen). To create N- or C- terminal GFP fusion these vectors together with pENTR-TOPO containing MtSyts genes and pENTR 2–3 (with or without GFP) were subsequently recombined into the modified pKGW-UBQ10::DsRED destination vector (Limpens et al., 2004) using Gateway technology (Invitrogen), pEnter clone of Exo70i was recombined to the plasmid of UBQ3-pK7WGF2-R (Limpens et al., 2009) creating N-terminal GFP fusion under Ubiquitin promoter (ProUbiq3:GFP-Exo70i).
The double RNAi constructs were created by fusing MtSyt1, MtSyt2 and MtSyt3 PCR-generated sequences using protocol adopted from Franssen et al. (2015). In a first round of PCR the short overlaps (15 bp) were introduced to PCR products by using specific primers. A mixture of two obtained PCR fragments diluted in 1:500 was used as a template in second PCR to create a single DNA fragment. The primers are listed in Supplementary Table 1.
Quantitative PCR Analysis
Total RNA was extracted from roots and 14-dpi root nodules using the E.Z.N.A. The extraction was made from two transformations. Plant RNA Mini Kit (Omega Bio-Tek) and transcribed into cDNA using the iScript cDNA synthesis kit (Bio-Rad). Real-time PCR was set up in a 20 μl reaction system using iQ SYBR Green Supermix (Bio-Rad). Gene-specific primers were designed with Primer-3-Plus software (Untergasser et al., 2007). Gene expression profiles were normalized against the transcription level of the reference gene UBQ10. The primers are listed in Supplementary Table 1. Results were compared with M. truncatula Gene Expression Atlas1 and Symbimics2 data. According to Symbimix database (Roux et al., 2014; INRA2). MtSyt1, MtSyt2 and MtSyt3 were expressed in the meristem, zone of infection and zone of nitrogen fixation; MtSyt1 and MtSyt3 have higher expression than MtSyt2 (Supplementary Figure 2B).
GUS Staining
Transgenic roots and nodules were collected and washed twice in 0.1M sodium phosphate buffer, pH 7.2, incubated in β-glucuronidase (GUS) buffer under vacuum at room temperature for 30 min to allow the buffer to replace oxygen in the tissue, incubated at 37°C for 2 h. Hand-cut sections of processed nodules were analyzed using Leica DM 5500 Flu microscopes.
Confocal Laser-Scanning Microscopy
GFP-fused proteins were visualized on transgenic roots and hand-sectioned nodules. Imaging was done on a Zeiss LSM 5 Meta confocal laser-scanning microscope (Carl Zeiss) and Leica TCS SP8 HyD confocal microscope (Leica) with 63 oil immersion objective. Polyclonal rabbit anti-GFP antibody (Molecular Probes) at a dilution of 1:100 and secondary anti-rabbit Alexa 488 antibody (Molecular Probes), (excitation max 490, emission max 525 nm) at a dilution of 1:200 were used for signal enhancement. The mixture of Goat serum (50%) with 2% (vol/wt) BSA was used as the blocking agent. Sections were counterstained with FM4-64 (30 μg/mL) or propidium iodide (0.001%).
Sample Preparation for Light and Electron Microscopy (EM)
Tissue preparation was performed as described previously (Limpens et al., 2009). Semi-thin (0.6 μm) sections were cut using a Leica Ultracut microtome (Leica) and examined using a Leica FL light microscope. For EM immunogold analysis the tissue was fixed by high-pressure freezing method as described before by Limpens et al. (2009). The nickel grids with the sections were blocked in normal goat serum with 1% of skimmed milk or 2% BSA in PBS and incubated with the primary antibody at the dilutions given above. Goat anti-rabbit coupled with 10-nm or with 15 nm gold particles (BioCell) (1:50 dilution) were used as secondary antibody. Sections were examined using a JEOL JEM 2100 transmission electron microscope equipped with a Gatan US4000 4K × 4K camera.
Western Blot Analysis
The proteins were extracted from root nodules in 0.025M Tris-HCl buffer containing 1 mM EDTA, 1 mM DTT and protease inhibitors cocktail (Roche). The probes loaded to the gel: 45 μg/well of root tips extracts of transgenic roots containing GFP-tagged synaptotagmins. The proteins were separated by 12.5% SDS-PAGE and blotted to nitrocellulose (Bio-Rad). The membrane was incubated in 3% BSA as a blocking agent followed by primary anti-GFP rabbit specific antibody, 1:1000 dilution; followed by secondary antibody, anti-rabbit AP antibody produced in goat (Sigma), 1:5000 dilution. The immunosignal was revealed by NBT/BCIP staining.
Accession Numbers
MtSyt1: Medtr4g073400. Sequence ID: XM_003607150.1.
MtSyt2: Medtr1g025550. Sequence ID: XM_003589467.1.
MtSyt3: Medtr1g094810. Sequence ID: XM_003591831.1.
MtExo 70i: Medtr1g017910. Sequence ID: XM_003589006.1.
Results
The Selection of Medicago truncatula Homologs of Synaptotagmin1
Five homologs of synaptotagmin1 genes were retrieved from M. truncatula databases by BLAST analysis using Arabidopsis thaliana synaptotagmin1 sequence. Phylogenetic analysis shows that the MtSyt1 and MtSyt2 genes and A. thaliana AtSYT1, AtSYT2 belong to the same group, and annotated in NCBI as synaptotagmins7 of M. truncatula, further sub-grouping is not clearly defined (Supplementary Figure 1). MtSyt1 is a closest homolog of AtSYT1. MtSyt3 is an ortholog of AtSyt3, forming a separate group (Yamazaki et al., 2010). MtSyt4 and MtSyt5 are grouped with AtSyt4 and AtSyt5.
For this study we selected MtSyt1, MtSyt2, and MtSyt3 genes. The expression of MtSyt1, MtSyt2, and MtSyt3 in roots and nodules at 14 dpi was analyzed by qRT-PCR (Supplementary Figure 2). MtSyt1 and MtSyt2 were expressed less in nodules than in the roots, whereas MtSyt3 expression in nodules was higher. According to Symbimix database (Roux et al., 2014; INRA3) MtSyt1, MtSyt2 and MtSyt3 are expressed in the meristem, zone of infection and zone of nitrogen fixation; the expression of MtSyt1 and MtSyt3 is higher than one of MtSyt2 (Supplementary Figure 2B). To clarify the role of synaptotagmins during symbiosis development, we studied the expression, localization, and functional role of MtSyt1, MtSyt2 and MtSyt3.
Expression Analyses of MtSyt1, MtSyt2, and MtSyt3
For the analysis, we created constructs containing a region 2.5 kb upstream of the translational start of MtSyt1, MtSyt2 and MtSyt3 fused to GUS. Transgenic roots were obtained using Agrobacterium rhizogenes-mediated transformation. In the roots ProMtSyt1:GUS, ProMtSyt2:GUS and ProMtSyt:GUS were expressed in all cells and most strongly in root primordia, meristem, epidermis of the zone of elongation, including the root hairs (Figures 1A–B). To see whether the expression pattern may be affected by membrane tension, we performed a root bending assay. Transgenic roots were selected from the plants grown in vertically oriented agar plates, bent and fixed using the tooth sticks. Bent roots were harvested after 48 h. Bending the root shifted the GUS signal to the site of curvature (Figures 1D,E). We concluded that the expression of synaptotagmins follows the region of the root with high membrane tension caused by the bending, hence the created constructs: ProMtSyt1:GUS, ProMtSyt2:GUS, and ProMtSyt3:GUS give a functional response for mechanical stimulation. In the nodules, ProMtSyt1:GUS, ProMtSyt2:GUS, and ProMtSyt3:GUS were strongly expressed in cells undergoing rapid increase of volume: in the cells of the meristem, of distal cell layers of the infection zone (Figures 1F–H) and distal layers of the zone of fixation (Figures 1F,H) (ProMtSyt1:GUS and ProMtSyt3:GUS).
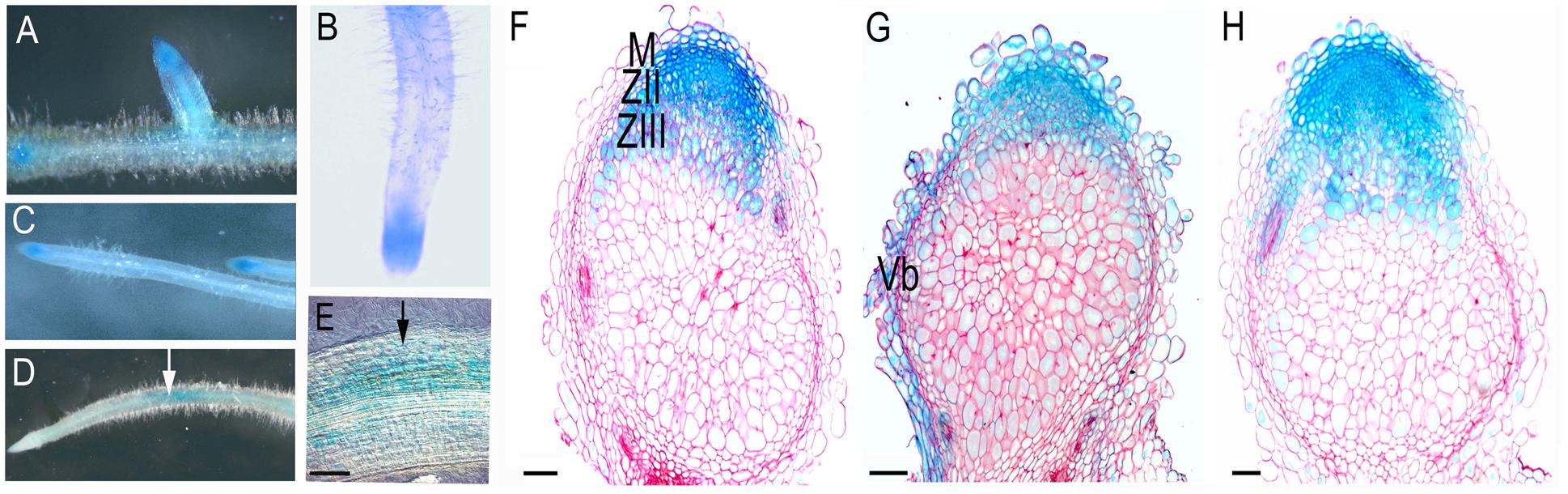
FIGURE 1. Promoter-GUS expression analysis of ProMtSyt1:GUS, ProMtSyt2:GUS, and ProMtSyt3:GUS in roots and nodules. (A) Expression pattern of ProMtSyt1:GUS;, (B) ProMtSyt2:GUS;(C) ProMtSyt3:GUS in roots. Synaptotagmins have a low ubiquitous expression in all cells, GUS staining is most intense in the meristems and vascular bundles. (D) ProMtSyt1: GUS in the root after bending. (E) The magnification of (D) shows the topography of GUS staining. The bending (arrow) is causing the repositioning of the GUS expression to the place of curvature. Expression pattern of ProMtSyt1:GUS (F), ProMtSyt2:GUS (G) and ProMtSyt3:GUS (H) in nodules, show the strong expression in the nodule meristem, vascular bundles, zone of infection and proximal layers of the fixation zone. M, meristem; ZII, zone of infection; ZIII, zone of fixation; Vb, vascular bundle. Bars: (E–H) = 100 μm.
Cellular Localization of MtSyt1, MtSyt2, and MtSyt3
To investigate the localization of synaptotagmins in nodules and roots, constructs expressing GFP translational fusions at the C-terminal position of these genes under the control of their respective 2.5 kb native 5′ regulatory sequences were created. The size of the proteins has been analyzed by Western blot with anti-GFP antibody (Supplementary Figure 3). For confocal imaging, roots and root nodules at 14–21 dpi were hand sectioned and fixed in 1% paraformaldehyde in a phosphate buffer as previously described (Gavrin et al., 2014). The GFP signal was weak and has to be enhanced by anti-GFP antibody coupled with secondary antibody tagged by ALEXA488. Confocal microscopy of the tissue reveals that GFP-tagged proteins ProMtSyt1:MtSyt1-GFP, ProMtSyt2:MtSyt2-GFP and ProMtSyt3:MtSyt3-GFP locally accumulate in the meristem of roots, the strongest signal was found in the developing cell walls in freshly divided cells (Figure 2A). In the nodules, the immunosignal of ProMtSyt1:MtSyt1-GFP, ProMtSyt2:MtSyt2-GFP and ProMtSyt3:MtSyt3-GFP was present in apical part of the nodule: in the meristem, zone of infection and proximal cell layers of zone of fixation and in vascular bundles (Figures 2B–D). On the cellular level the signal was found in the ER-rich central areas of the cell with an enrichment of the signal over the PM of freshly divided meristematic cells and around infection threads and unwalled droplets (Figures 2E,G,H). The symbiosomes were specifically outlined by the GFP signal only in the nodules carrying ProMtSyt1:MtSyt1-GFP (Figure 2F). The nodule tissue from the negative control where the primary (anti-GFP) antibody was omitted during the labeling process does not display labeling pattern (Supplementary Figure 4A). Synaptotagmins were therefore localized in the cells with expanding membranes. The special role in symbiosome membrane expansion, according to the localization study, belongs to MtSyt1 which is localizes on symbiosome membrane. Electron microscopy (EM) immunogold analysis of the transgenic nodules carrying GFP-tagged synaptotagmin shows the signal over the PM of freshly divided cells, in ER and the symbiosome membrane in the transgenic nodules carrying ProMtSyt1:MtSyt1-GFP (Figures 3A–C). The control without primary (anti-GFP) antibody is shown on Supplementary Figure 4B.
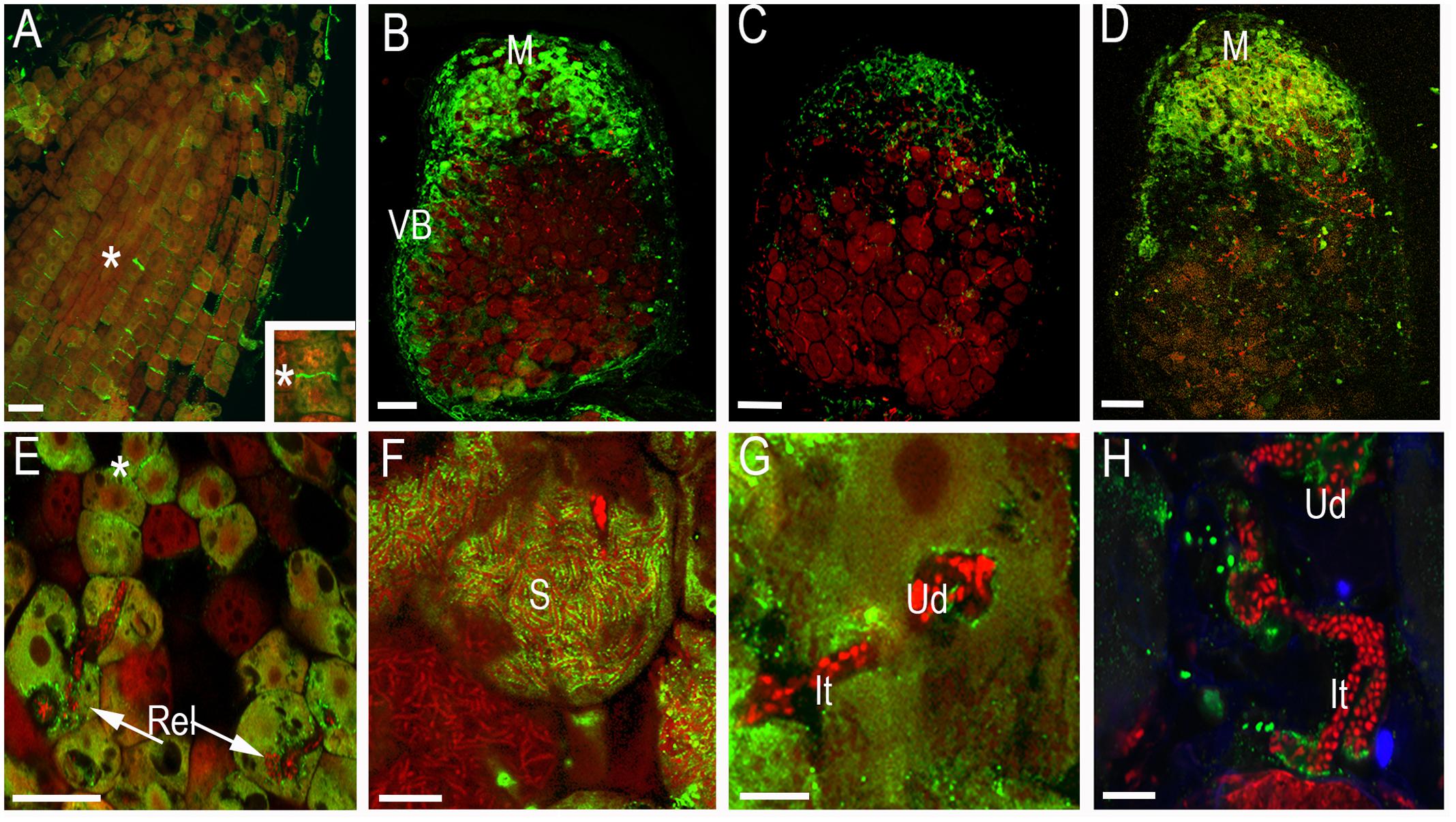
FIGURE 2. The localization of GFP-tagged MtSyt1, MtSyt2 and MtSyt3 by confocal microscopy immunolabeling with anti-GFP antibody. In root meristem the synaptotagmin signal is quite intense over developing cell walls in freshly divided meristematic cells (∗) (ProMtSyt2:MtSyt2-GFP) (A). The localization of synaptotagmins in apical part of root nodules: ProMtSyt1:MtSyt1-GFP (B), ProMtSyt2:MtSyt2-GFP (C), ProMtSyt3:MtSyt3-GFP (D). On the cellular level the signal of synaptotagmins is associated with newly formed cell walls in nodule meristem, with infection threads/unwalled droplets on the place of bacteria release. ProMtSyt1:MtSyt1-GFP (E); symbiosomes labeled by ProMtSyt1:MtSyt1-GFP (F). ProMtSyt2:MtSyt2-GFP (G), ProMtSyt3:MtSyt3-GFP (H). Vb, vascular bundle; Rel, release of bacteria; It, infection thread; Ud, unwalled droplet; M, meristem; S, symbiosomes; PM, plasma membrane; asterisk(∗) developing cell wall. Bars: (A–D) = 100 μm, (E) = 25 μm, (H,G) = 5 μm, (H) = 10 μm. Color codes: synaptotagmins: green fluorescence, rhizobia and nuclei of host cell: red fluorescence. Single optical sections.
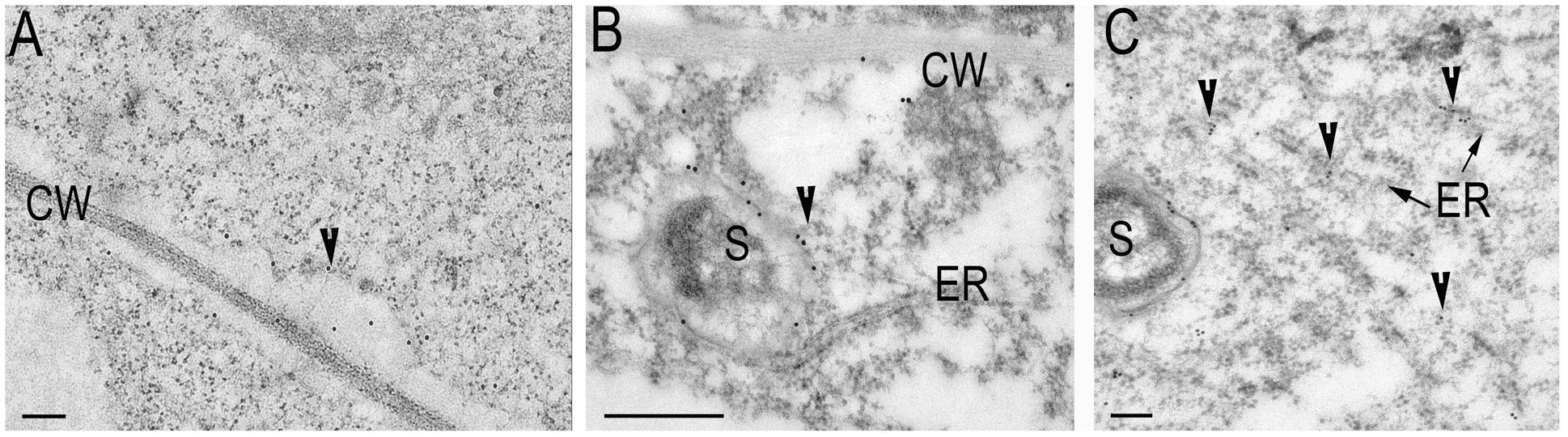
FIGURE 3. Electron microscopy (EM) immunogold labeling of Syt1 (A–C): The signal over the plasma membrane (PM) of freshly divided cells (A); the labeling on symbiosome membrane, note the contact with ER (B); (C) The signal on symbiosome membrane and ER. Gold granules are pointed by arrowheads. CW, cell wall; ER, endoplasmic reticulum; S, symbiosome. Bars: (A) = 200 nm, (B) = 500 nm, (C) = 200 nm
Endoplasmic reticulum is extremely abundant in young, freshly infected cells. ER contact sites with the membranes of infection threads, unwalled droplets, and symbiosomes represented as local dilations of tubular extensions and vesicles are ubiquitously present in young infected cells. The images obtained by transmission EM show numerous fusion events with the membrane vesicles and close contacts with ER (Supplementary Figures 5A,B).
To check the functional status of GFP-tagged constructs, we compared the localization pattern of the construct with GFP at the C-terminal position against the construct containing N-terminal (transmembrane domain) GFP (ProMtSyt3-GFP: MtSyt3). The GFP positioning on the transmembrane domain resulted in the protein becoming mis-localized. According to the localization pattern, the GFP signal was not targeted to the PM and was retained in the cytoplasm forming a dot-like pattern (Supplementary Figure 6A). EM immunogold analysis has shown that the protein was mainly present over the ER and in Golgi bodies (Supplementary Figure 6B). Therefore the C-terminal constructs which were used for the localization study maintained the correct positioning in the PM of the host cell.
Functional Analysis Using Double Silencing of MtSyt1/MtSyt3 and MtSyt2/ MtSyt3
We believe that the null mutations in case of housekeeping genes like synaptotagmins negatively affect the development of the whole host plant and indiscriminately damage the nodules. Hereafter, to specify the role of synaptotagmins in the infected symbiotic cells and avoid the negative effects for the shoots, vascular system and non-infected nodule cells we used RNAi silencing under the control of Enod12 promoter. This promoter is active in the nodules in zone of infection (Limpens et al., 2005), this approach permits to silence genes only in the young infected cells without affecting the other cells of the nodule.
We have created the double silencing constructs of MtSyt1/MtSyt3 and MtSyt2/MtSyt3 to induce a simultaneous silencing under the Enod12 promoter. The level of silencing of MtSyt1/MtSyt3 and MtSyt2/MtSyt3 in transgenic nodules of ProENOD12:MtSyt1/MtSyt3 and ProENOD12:MtSyt2/MtSyt3 is shown in Supplementary Figure 7.
Analysis of the transgenic roots from 10 plants showed that transgenic nodules were smaller and less numerous: 2.81 ± 1.65 per plant versus 5.32 ± 1.91 in the controls. Transgenic nodules shown a distinct phenotype: a short meristem, extended zone of infection and diminished or aborted zone of fixation (Figures 4A–D versus Figures 4E,F). Zones of infection have increased numbers of cell layers (Table 1), delayed rhizobia release and symbiosome maturation. The double-silencing phenotype was observed in 65% of the nodules (n = 20). The analysis was performed on 20 nodules randomly collected from 10 plants. In the majority of affected nodules, the symbiosomes were not able to differentiate beyond stage 2/3 according to the classification of Vasse et al. (1990) and undergo premature senescence (Figure 4A). EM analysis of nodules with double silencing (ProENOD12:MtSyt1/MtSyt3 and ProENOD12:MtSyt2/MtSyt3) shown that infected cells in the extended zone of infection contain unwalled droplets without apparent bacteria release (Figures 4G,H). However, the release was not completely inhibited and the cells were finally colonized, but the maturation of the symbiosomes was restricted. The colonization of infected cells was quite slow and was taking up to 12 cell layers (cf. Figures 4A,B with Figure 4E) comparing with 3–5 in control. The number of cell layers in different nodule zones in control and RNAi nodules and the statistical analysis are presented in Table 1. The T-test was used to estimate a difference between control and RNAi nodules (P < 0.05).We have concluded that the double silencing of MtSyt1/MtSyt3 and MtSyt2/MtSyt3 negatively affects nodule meristem development, growth of infected cells, caused the reduction of bacteria release from unwalled droplets, and hampers growth and maturation of symbiosomes.
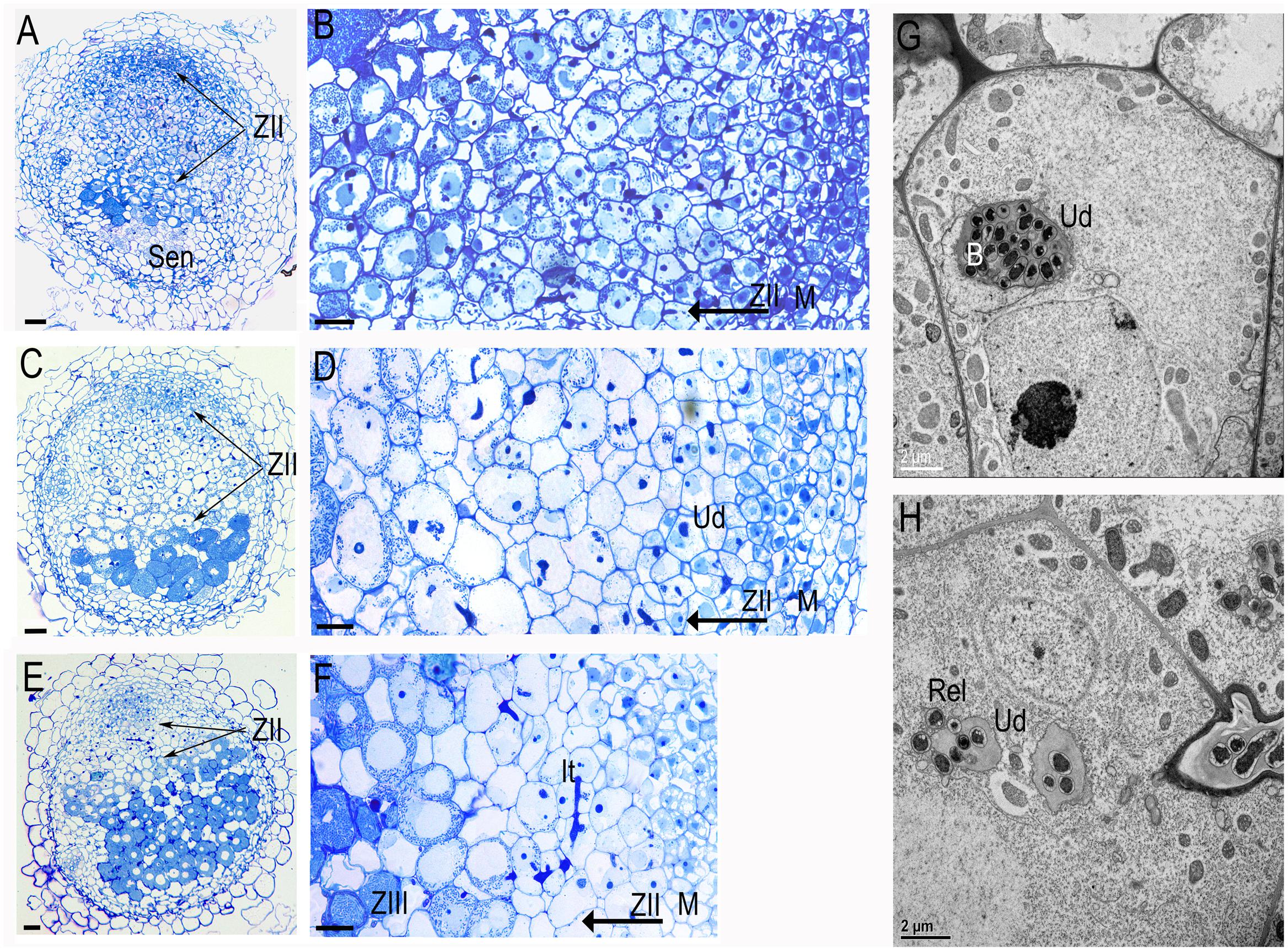
FIGURE 4. Functional analysis of synaptotagmins using double silencing constructs ProENOD12:MtSyt1/MtSyt3 and ProENOD12:MtSyt2/MtSyt3 analyzed by light (A–F) and electron microscopy (G,H). Nodules elicited on the transgenic roots: ProENOD12:MtSyt1/MtSyt3 (A,B), ProENOD12:MtSyt2/MtSyt3 (C,D). Note the extended zone of infection (zoneII) (arrows) and the senescent cells on the basal part of the nodules. (B) The magnification of (A,D) the magnification of (C) showing the extended zone of infection, note the small immature bacteroides populating the infected cells of zoneII. (E,F) Control nodule. Note the short zone of infection followed by zone of nitrogen fixation (zoneIII) containing mature infected cells with large developed bacteroides and the starch granules. (G) EM image displays the cells containing the unwalled droplet without the bacteria release from the transgenic nodules of ProENOD12:MtSyt1/MtSyt3, (H) control nodules of the same stage of development as (G) with unwalled droplets and releasing rhizobia. ZII, zone of infection; ZIII, zone of nitrogen fixation; Sen, zone of senescence; It, infection thread; Ud, unwalled droplet; B, bacteria; Rel, release of rhizobia. Bars: (A,C,E) = 50 μm; (B,D,F) = 25 μm, (G,H): as indicated.
The Localization of MtSyt1, MtSyt3 in Arbusculated Root Cells in Comparison with Exo70i
To specify the role of synaptotagmins in the formation of interface membrane in other type of symbiosis we have studied the localization of MtSyt3 in the roots inoculated by R. intraradices. The formation of arbuscules, the symbiotic intracellular extensions of hyphae is always accompanied by the rapid increase of interface membrane (Genre et al., 2009; Gutjahr and Parniske, 2013). The distribution of GFP signal of ProMtSyt1:MtSyt1-GFP and ProMtSyt3:MtSyt3-GFP was similar, it was clearly delineating the membranes of arbuscules fine branches, but not the trunks (Supplementary Figures 8A,B). The GFP signal was enhanced by anti-GFP antibody coupled with secondary antibody tagged by ALEXA488 equally as it was done for the nodule tissue.
To specify the role of synaptotagmin in tip growth we have compared the localization of synaptotagmin with the localization of Exo70i, the subunit of tethering complex exocyst in root nodule infected cells and in the cells containing arbuscules. For the study we have cloned the Exo70 subunit which is highly expressed in arbusculated roots and in the nodules. The same gene have been cloned and characterized by Zhang et al. (2015), due to this we are using the abbreviation used by Zhang et al. (2015): Exo70i, despite that this gene was cloned by us independently.
In arbusculated root cells Exo70i was strictly localized near the tip of fine branches of arbuscules in contrast to the signal of synaptotagmin which was found over the whole surface of the branches (Supplementary Figure 8C versus Supplementary Figures 8A,B). The localization pattern of this subunit over the arbuscules in our work and in the paper of Zhang et al. (2015) shows the identical pattern.
The localization of Exo70i in infected cells of the nodules was specific, it has dot-like pattern and forms a small clusters near the tips of infection threads and also was marking unwalled droplets, but was not delineating the membrane of infection thread or unwalled droplet like synaptotagmins do, neither it was labeling the symbiosome membrane (Supplementary Figure 8D).
Therefore we concluded that the distribution of synaptotagmins on the membranes of infection threads and fine branches of arbuscules was spatially overlapping on the regions of infection threads tips and the tips of arbuscular fine branches, but was not overlaying with the signal of Exo70i on the whole interface membrane.
Discussion
The intracellular accommodation of rhizobia triggers the reformation of endomembrane system, redirection of vesicular traffic and reorganization of the cytoskeleton and vacuole of a host cell (Roth and Stacey, 1989; Parniske, 2000; Gibson et al., 2008; Ivanov et al., 2012; Kondorosi et al., 2013; Gavrin et al., 2014, 2015, 2016; Gavrin, 2015). As a result of these events the symbiotic interface membrane is formed. We hypothesized that the membrane tension created by expanding microsymbiont provides the vector for targeted endomembrane traffic toward the symbiotic interface. To address this hypothesis, we performed promoter-GUS analyses, the localization study, and functional analysis of synaptotagmins MtSyt1, MtSyt2 and MtSyt3. As it was expected synaptotagmins, involved in housekeeping functions of membrane fusion, gave markedly enhanced signal over the “symbiotic” unsymmetrical protrusions of PM in zone of infection. We believe that the local accumulation of synaptotagmins over the interface enveloping expanding microbes represents functional membrane subcompartmentalization (Jarsch et al., 2014; Konrad and Ott, 2015) and may be causal for enhanced fusion capacity of these subcompartments. Synaptotagmins in this situation are serving as a “beacons” for a vectorial membrane transport. We do not consider it to be a “symbiotic” function, but rather a house keeping function of the host cell used for urgent membrane proliferation. For example, the localization pattern of Nicotiana benthamiana synaptotagmin homolog on the membrane enveloping the haustorium formed by Phytophthora infestans was quite similar to the pattern observed in our experiments with infection threads and arbuscular thin branches (Lu et al., 2012; Bozkurt et al., 2014).
The localization of MtSyt1 on symbiosome membrane is especially interesting. Symbiosomes are detached from PM, however, the surrounding symbiosome membrane surface reach up to several folds of PM of host cell (Roth and Stacey, 1989). The complete maturation of symbiosomes takes 5–7 cell layers, with most rapid growth in 1–2 cell layers proximal to zone of nitrogen fixation (Gavrin et al., 2014). This speedy growth brings under the consideration the putative membrane resources for the symbiosome membrane. The most obvious source is an exocytotic pathway with post-Golgi vesicles (Van de Velde et al., 2010; Wang et al., 2010; Ivanov et al., 2012; Sinharoy et al., 2016). However, since long ER has been considered to be one of the sources of membrane for symbiosomes (Roth and Stacey, 1989; Clarke et al., 2015), it is extremely abundant in young infected cells (Ivanov et al., 2012). Recently it was reported that ER is the main membrane source for biogenesis of the lytic vacuole in Arabidopsis meristem Viotti et al. (2013). As an organelle ER consists of discrete functional domains, it is quite dynamic and change a tubular to a vesicular morphology (Sparkes et al., 2009; Raeymaekers and Larivière, 2011). We speculate that the synaptotagmins may create a “reaper points” on symbiosome membrane for the fusion with ER. The putative SNARE complexes in infected cells may include the homologs of SNARE NPSN11, SNARE SYP71, and VAMP721, 722 which are forming tetrameric SNARE complex on the cell plates during cytokinesis in Arabidopsis (El Kasmi et al., 2013). In the previous work we have found that v-SNAREs MtVAMP721d and MtVAMP721a are essential for the intracellular accommodation of microsymbionts in nodules and arbusculated roots of M. truncatula. These SNAREs localize on symbiosome membrane and on the membranes of fine branches of arbuscules, as well as on the cell plate (Ivanov et al., 2012).
The comparison of localization pattern of MtSyt1, MtSyt2, and MtSyt3 showed also some unexpected differences. The localization on the symbiosome membrane is a result of retargeting from the default targeting to host cell PM. The retargeting toward symbiotic interface depends on the level of expression (Pumplin et al., 2012) as well as on other factors including the reformation of actin network around the symbiosomes (Gavrin et al., 2015). However, MtSyt3, the homolog of AtSyt3, which has similar expression pattern with MtSyt1 and localizes in apical part of the nodule and in proximal cell layers of zone of nitrogen fixation was not localized on the symbiosome membrane in contrast to MtSyt1. The functional features of Syt3 in plants are not defined, however, for the explanation we can point to the features of animal cells Syt3 (SytIII). Apart of being on PM (Bhalla et al., 2008) it colocalizes with early endosomal markers (Grimberg et al., 2003). In previous work we have found that symbiosomes do not accept early endosome markers (Limpens et al., 2009), so it may be one of the reasons why MtSyt3 is not retargeted toward symbiosome membrane.
The functional analysis of MtSyt1, MtSyt2, and MtSyt3 using double RNAi of MtSyt1/MtSyt2 and MtSyt2/MtSyt3 confirms the role of synaptotagmins in formation of symbiotic membrane interface and its growth. The silencing causes a delay in bacteria release and maturation and consequent widening of the infection zone. Such type of the deviations in symbiosomes development reflects the defect in colonization by rhizobia (Sinharoy et al., 2016). This phenotype resembles the phenotype of nodules with silencing of vesicle-associated membrane protein VAMP72d/a, the key player in targeted membrane fusion to PM and to symbiotic interface in nodules and micorriza (Ivanov et al., 2012). The restriction of symbiosomes development to stage 1–2 was also observed in the mutant dnf1 (Wang et al., 2010). Dnf1 encodes a symbiosis-specific subunit of the signal-peptidase complex (Van de Velde et al., 2010; Wang et al., 2010), a component of the protein secretory pathway in the ER.
With the aim of determining the role of synaptotagmins in interface membrane formation, we compared the localization of MtSyt1, MtSyt3 and exocyst subunit EXO70i in root nodules and arbusculated roots. The localization patterns of MtSyt1, MtSyt3 and EXO70i has shown that subcompartments labeled by synaptotagmins are broader and include lateral parts of infection threads and the membranes of unwalled droplets, which are the structures with an isodiametric type of growth, MtSyt1 also labels symbiosomes. This suggests that not only tip-targeted post-Golgi vesicles but also other membrane resources like, for instance, ER may be involved in the growth of interface membrane.
Conclusion
The synaptotagmin-dependent membrane fusion activated by the change in the membrane tension around the expanding microsymbionts along with tip-targeted exocytosis is operational in the formation of symbiotic interface.
Author Contributions
EF and AG designed the research. AG, EF, and OK performed experiments and analyzed data. AG and EF wrote the manuscript. EF, AG, OK, and TB edited the manuscript.
Conflict of Interest Statement
The authors declare that the research was conducted in the absence of any commercial or financial relationships that could be construed as a potential conflict of interest.
Acknowledgments
AG received a Ph.D. fellowship from EPS School of Biological Sciences (Wageningen University). Presented paper contains part of the data from chapter 5 of Dr. Gavrin’s Ph.D. thesis. EF and TB are supported by the European Research Council (ERC- 2011-AdG294790) awarded to TB Authors are very grateful for Dr. H. Franssen for his valuable comments on the draft. Electron microscopy analysis was performed in Wageningen University Electron Microscopy Center (WEMC). English spellcheck: http://www.tessera-trans.com.
Supplementary Material
The Supplementary Material for this article can be found online at: http://journal.frontiersin.org/article/10.3389/fpls.2017.00201/full#supplementary-material
Footnotes
- ^ http://mtgea.noble.org/v2/
- ^ https://iant.toulouse.inra.fr/symbimics/
- ^ https://iant.toulouse.inra.fr/symbimics/
References
Adolfsen, B., Saraswati, S., Yoshihara, M., and Littleton, J. T. (2004). Synaptotagmins are trafficked to distinct subcellular domains including the postsynaptic compartment. J. Cell Biol. 166, 249–260.
Apodaca, G. (2002). Modulation of membrane traffic by mechanical stimuli. Am. J. Physiol. Renal Physiol. 282, F179–F190.
Bhalla, A., Chicka, M. C., and Chapman, E. R. (2008). Analysis of the synaptotagmin family during reconstituted membrane fusion. Uncovering a class of inhibitory isoforms. J. Biol. Chem. 283, 21799–21807. doi: 10.1074/jbc.M709628200
Bozkurt, T. O., Richardson, A., Dagdas, Y. F., Mongrand, S., Kamoun, S., and Raffaele, S. (2014). The plant membrane-associated REMORIN1.3 accumulates in discrete perihaustorial domains and enhances susceptibility to Phytophthora infestans. Plant Physiol. 165, 1005–1018.
Brewin, N. J. (2004). Plant cell wall remodelling in the Rhizobium-legume symbiosis. Crit. Rev. Plant Sci. 23, 293–316.
Chehab, E. W., Eich, E., and Braam, J. (2009). Thigmomorphogenesis: a complex plant response to mechano-stimulation. J. Exp. Bot. 60, 43–56. doi: 10.1093/jxb/ern315
Clarke, V. C., Loughlin, P. C., Gavrin, A., Chen, C., Brear, E. M., Day, D. A., et al. (2015). Proteomic analysis of the soybean symbiosome identifies new symbiotic proteins. Mol. Cell. Proteomics 4, 1301–1322. doi: 10.1074/mcp.M114.043166
Draeger, A., Monastyrskaya, K., and Babiychuk, E. B. (2011). Plasma membrane repair and cellular damage control: the annexin survival kit. Biochem. Pharmacol. 81, 703–712. doi: 10.1016/j.bcp.2010.12.027
El Kasmi, F., Krause, C., Hiller, U., Stierhof, Y. D., Mayer, U., Conner, L., et al. (2013). SNARE complexes of different composition jointly mediate membrane fusion in Arabidopsis cytokinesis. Mol. Biol. Cell 24, 1593–1601. doi: 10.1091/mbc.E13-02-0074
Falkowski, M. A., Thomas, D. D. H., Messenger, S. W., Martin, T. F., and Groblewski, G. E. (2011). Expression, localization, and functional role for synaptotagmins in pancreatic acinar cells. Am. J. Physiol. Gastrointest. Liver Physiol. 301, G306–G316. doi: 10.1152/ajpgi.00108.2011
Franssen, H. J., Xiao, T. T., Kulikova, O., Wan, X., Bisseling, T., Scheres, B., et al. (2015). Root developmental programs shape the Medicago truncatula nodule meristem. Development 142, 2941–2950. doi: 10.1242/dev.120774
Gauthier, N. C., Rossier, O. M., Mathur, A., Hone, J. C., and Sheetz, M. P. (2009). Plasma membrane area increases with spread area by exocytosis of a GPI-anchored protein compartment. Mol. Biol. Cell 20, 3261–3272. doi: 10.1091/mbc.E09-01-0071
Gavrin, A. (2015). Intracellular Accommodation of Rhizobia in Legume Host Cwll: The Fine-Tuning of the Endomembrane System. Ph.D. thesis, Wageningen University, Wageningen.
Gavrin, A., Chiasson, D., Ovchinnikova, E., Kaiser, B. N., Bisseling, T., and Fedorova, E. E. (2016). VAMP721a and VAMP721d are important for pectin dynamics and release of bacteria in soybean nodules. New Phytol. 210, 1011–1021. doi: 10.1111/nph.13837
Gavrin, A., Jansen, V., Ivanov, S., Bisseling, T., and Fedorova, E. (2015). ARP2/3-mediated actin nucleation associated with symbiosome membrane is essential for the development of symbiosomes in infected cells of Medicago truncatula root nodules. Mol. Plant Microbe Interact. 28, 605–614. doi: 10.1094/MPMI-12-14-0402-R
Gavrin, A., Kaiser, B. N., Geiger, D., Tyerman, S. D., Wen, Z., Bisseling, T., et al. (2014). Adjustment of host cells for accommodation of symbiotic bacteria: vacuole defunctionalization, HOPS suppression, and TIP1g retargeting in Medicago. Plant Cell 26, 3809–3822. doi: 10.1105/tpc.114.128736
Genre, A., Ortu, G., Bertoldo, C., Martino, E., and Bonfante, P. (2009). Biotic and abiotic stimulation of root epidermal cells reveals common and specific responses to arbuscular mycorrhizal fungi. Plant Physiol. 149, 1424–1434. doi: 10.1104/pp.108.132225
Gibson, K. E., Kobayashi, H., and Walker, G. C. (2008). Molecular determinants of a symbiotic chronic infection. Annu. Rev. Genet. 42, 413–441. doi: 10.1146/annurev.genet.42.110807.091427
Grefen, C., Honsbein, A., and Blatt, M. R. (2011). Ion transport, membrane traffic and cellular volume control. Curr. Opin. Plant Biol. 14, 332–339. doi: 10.1016/j.pbi.2011.03.017
Grimberg, E., Peng, Z., Hammel, I., Sagi-Eisenberg, R. (2003). Synaptotagmin III is a critical factor for the formation of the perinuclear endocytic recycling compartment and determination of secretory granules size. J. Cell Sci. 116, 145–154. doi: 10.1242/jcs.00186
Gutjahr, C., and Parniske, M. (2013). Cell and developmental biology of arbuscular mycorrhiza symbiosis. Annu. Rev. Cell Dev. Biol. 29, 593–617. doi: 10.1146/annurev-cellbio-101512-122413
Hardham, A. R., Takemoto, D., and White, R. G. (2008). Rapid and dynamic subcellular reorganization following mechanical stimulation of Arabidopsis epidermal cells mimics responses to fungal and oomycete attack. BMC Plant Biol. 8:63. doi: 10.1186/1471-2229-8-63
He, B., and Guo, W. (2009). The exocyst complex in polarized exocytosis. Curr. Opin. Plant Biol. 21, 537–542.
Hepler, P., and Winship, L. (2010). Calcium at the cell wall-cytoplast interface. J. Integr. Plant Biol. 52, 147–160. doi: 10.1111/j.1744-7909.2010.00923.x
Idone, V., Tam, C., and Andrews, N. W. (2008). Two-way traffic on the road to membrane repair. Trends Cell Biol. 18, 552–559. doi: 10.1016/j.tcb.2008.09.001
Ivanov, S., Fedorova, E. E., Limpens, E., De Mita, S., Genre, A., Bonfante, P., et al. (2012). Rhizobium-legume symbiosis shares an exocytotic pathway required for arbuscule formation. Proc. Natl. Acad. Sci. U.S.A. 109, 8316–8321. doi: 10.1073/pnas.1200407109
Jaffe, M. J., Leopold, A. C., and Staples, R. C. (2002). Thigmo responses in plants and fungi. Am. J. Bot. 89, 375–382. doi: 10.3732/ajb.89.3.375
Jarsch, I. K., Konrad, S. S., Stratil, T. F., Urbanus, S. L., Szymanski, W., Braun, P., et al. (2014). Plasma membranes are subcompartmentalized into a plethora of coexisting and diverse microdomains in Arabidopsis and Nicotiana benthamiana. Plant Cell 26, 1698–1711.
Jayaraman, D., Gilroy, S., and Ané, J. M. (2014). Staying in touch: mechanical signals in plant-microbe interactions. Curr. Opin. Plant Biol. 20, 104–109. doi: 10.1016/j.pbi.2014.05.003
Ketelaar, T., de Ruijter, N. C. A., and Emons, A. M. C. (2003). Unstable F-actin specifies the area and microtubule direction of cell expansion in Arabidopsis root hairs. Plant Cell 15, 285–292.
Kim, H., Kwon, H., Kim, S., Kim, M. K., Botella, M. A., Yun, H. S., et al. (2016). Synaptotagmin 1 negatively controls the two distinct immune secretory pathways to powdery mildew fungi in Arabidopsis. Plant Cell Physiol. 57, 1133–1141. doi: 10.1093/pcp/pcw061
Kondorosi, E., Mergaert, P., and Kereszt, A. (2013). A paradigm for endosymbiotic life: cell differentiation of rhizobium bacteria provoked by host plant factors. Annu. Rev. Microbiol. 67, 611–628. doi: 10.1146/annurev-micro-092412-155630
Konrad, S. S., and Ott, T. (2015). Molecular principles of membrane microdomain targeting in plants. Trends Plant Sci. 20, 351–361. doi: 10.1016/j.tplants.2015.03.016
Levy, A., Zheng, J. Y., and Lazarowitz, S. G. (2015). Synaptotagmin SYTA forms ER-plasma membrane junctions that are recruited to plasmodesmata for plant virus movement. Curr. Biol. 3, 2018–2025. doi: 10.1016/j.cub.2015.06.015
Limpens, E., Ivanov, S., van Esse, W., Voets, G., Fedorova, E., and Bisseling, T. (2009). Medicago N2-fixing symbiosomes acquire the endocytic identity marker Rab7 but delay the acquisition of vacuolar identity. Plant Cell 21, 2811–2828. doi: 10.1105/tpc.108.064410
Limpens, E., Mirabella, R., Fedorova, E., Franken, C., Franssen, H., Bisseling, T., et al. (2005). Formation of organelle-like N2-fixing symbiosomes in legume root nodules is controlled by DMI2. Proc. Natl. Acad. Sci. U.S.A. 102, 10375–10380.
Limpens, E., Ramos, J., Franken, C., Raz, V., Compaan, B., Franssen, H., et al. (2004). RNA interference in Agrobacterium rhizogenes-transformed roots of Arabidopsis and Medicago truncatula. J. Exp. Bot. 55, 983–992.
Lu, Y. J., Schornack, S., Spallek, T., Geldner, N., Chory, J., Schellmann, S., et al. (2012). Patterns of plant subcellular responses to successful oomycete infections reveal differences in host cell reprogramming and endocytic trafficking. Cell Microbiol. 14, 682–697. doi: 10.1111/j.1462-5822.2012.01751.x
Manford, A. G., Stefan, C. J., Yuan, H. L., Macgurn, J. A., and Emr, S. D. (2012). ER-to-plasma membrane tethering proteins regulate cell signaling and ER morphology. Dev. Cell 23, 1129–1140. doi: 10.1016/j.devcel.2012.11.004
Maximov, A., Tang, J., Yang, X., Pang, Z. P., and Südhof, T. C. (2009). Complexin controls the force transfer from SNARE complexes to membranes in fusion. Science 323, 516–521. doi: 10.1126/science.1166505
McNeil, P. L., and Kirchhausen, T. (2005). An emergency response team for membrane repair. Nat. Rev. Mol. Cell Biol. 6, 499–505.
Morris, C. E., and Homann, U. (2001). Cell surface area regulation and membrane tension. J. Membr. Biol. 179, 79–102.
Nakagawa, Y., Katagiri, T., Shinozaki, K., Qi, Z., Tatsumi, H., Furuichi, T., et al. (2007). Arabidopsis plasma membrane protein crucial for Ca(2+) influx and touch sensing in roots. Proc. Natl. Acad. Sci. U.S.A. 104, 3639–3644.
Parniske, M. (2000). Intracellular accommodation of microbes by plants: a common developmental program for symbiosis and disease? Curr. Opin. Plant Biol. 3, 320–328.
Pérez-Sancho, J., Vanneste, S., Lee, E., McFarlane, H. E., Esteban, Del Valle A, Valpuesta, V., et al. (2015). The Arabidopsis synaptotagmin1 is enriched in endoplasmic reticulum-plasma membrane contact sites and confers cellular resistance to mechanical stresses. Plant Physiol. 168, 132–143. doi: 10.1104/pp.15.00260
Pumplin, N., Zhang, X., Noar, R. D., and Harrison, M. J. (2012). Polar localization of a symbiosis-specific phosphate transporter is mediated by a transient reorientation of secretion. Proc. Natl. Acad. Sci. U.S.A. 109, E665–E672. doi: 10.1073/pnas.1110215109
Qin, Y., and Dong, J. (2015). Focusing on the focus: What else beyond the master switches for polar cell growth? Mol. Plant. 8, 582–594. doi: 10.1016/j.molp.2014.12.023
Raeymaekers, L., and Larivière, E. (2011). Vesicularization of the endoplasmic reticulum is a fast response to plasma membrane injury. Biochem. Biophys. Res. Commun. 414, 246–251. doi: 10.1016/j.bbrc.2011.09.065
Reddy, A., Caler, E. V., and Andrews, N. W. (2001). Plasma membrane repair is mediated by Ca2+–regulated exocytosis of lysosomes. Cell 106, 157–169.
Roth, I. E., and Stacey, G. (1989). Bacterium release into host cells of nitrogen-fixing soybean nodules: the symbiosome membrane comes from three sources. Eur. J. Cell Biol. 49, 13–23.
Roux, B., Rodde, N., Jardinaud, M.-F., Timmers, T., Sauviac, L., Cottret, L., et al. (2014). An integrated analysis of plant and bacterial gene expression in symbiotic root nodules using laser-capture microdissection coupled to RNA sequencing. Plant J. 77, 817–837. doi: 10.1111/tpj.12442
Šamaj, J., Müller, J., Beck, M., Böhm, N., and Menzel, D. (2006). Vesicular trafficking, cytoskeleton and signalling in root hairs and pollen tubes. Trends Plant Sci. 11, 594–600.
Schapire, A. L., Voigt, B., Jasik, J., Rosado, A., Lopez-Cobollo, R., Menzel, D., et al. (2008). Arabidopsis synaptotagmin 1 is required for the maintenance of plasma membrane integrity and cell viability. Plant Cell 20, 3374–3388. doi: 10.1105/tpc.108.063859
Sinharoy, S., Liu, C., Breakspear, A., Guan, D., Shailes, S., Nakashima, J., et al. (2016). A Medicago truncatula cystathionine-b-synthase-like domain-containing protein is required for rhizobial infection and symbiotic nitrogen fixation. Plant Physiol. 170, 2204–2217. doi: 10.1104/pp.15.01853
Sparkes, I., Runions, J., Hawes, C., and Griffing, L. (2009). Movement and remodeling of the endoplasmic reticulum in nondividing cells of tobacco leaves. Plant Cell 21, 3937–3949. doi: 10.1105/tpc.109.072249
Telewski, F. W. (2006). A unified hypothesis of mechanoperception in plants. Am. J. Bot. 93, 1466–1476. doi: 10.3732/ajb.93.10.1466
Untergasser, A., Nijveen, H., Rao, X., Bisseling, T., Geurts, R., and Leunissen, J. A. M. (2007). Primer3Plus, an enhanced web interface to Primer3. Nucleic Acids Res. 35(Suppl. 2), W71–W74.
Van de Velde, W., Zehirov, G., Szatmari, A., Debreczeny, M., Ishihara, H., Kevei, Z., et al. (2010). Plant peptides govern terminal differentiation of bacteria in symbiosis. Science 327, 1122–1126. doi: 10.1126/science.1184057
Vasse, J., de Billy, F., Camut, S., and Truchet, G. (1990). Correlation between ultrastructural differentiation of bacteroids and nitrogen fixation in alfalfa nodules. J. Bacteriol. 172, 4295–4306.
Viotti, C., Krüger, F., Krebs, M., Neubert, C., Fink, F., Lupanga, U., et al. (2013). The endoplasmic reticulum is the main membrane source for biogenesis of the lytic vacuole in Arabidopsis. Plant Cell 25, 3434–3449. doi: 10.1105/tpc.113.114827
Vukašinović, N., and Žárský, V. (2016). Tethering complexes in the Arabidopsis endomembrane system. Front. Cell Dev. Biol. 19:46.
Wang, D., Griffitts, J., Starker, C., Fedorova, E., Limpens, E., Ivanov, S., et al. (2010). A nodule-specific protein secretory pathway required for nitrogen-fixing symbiosis. Science 327, 1126–1129. doi: 10.1126/science.1184096
Yamazaki, T., Kawamura, Y., Minami, A., and Uemura, M. (2008). Calcium-dependent freezing tolerance in Arabidopsis involves membrane resealing via synaptotagmin SYT1. Plant Cell 20, 3389–3404. doi: 10.1105/tpc.108.062679
Yamazaki, T., Takata, N., Uemura, M., and Kawamura, Y. (2010). Arabidopsis synaptotagmin SYT1, a type I signal-anchor protein, requires tandem C2 domains for delivery to the plasma membrane. J. Biol. Chem. 285, 23165–23176. doi: 10.1074/jbc.M109.084046
Zárský, V., Kulich, I., Fendrych, M., and Pečenková, T. (2013). Exocyst complexes multiple functions in plant cells secretory pathways. Curr. Opin. Plant Biol. 16, 726–733. doi: 10.1016/j.pbi.2013.10.013
Keywords: symbiosis, synaptotagmin1, membrane tension/repair, interface membrane, root nodule, arbuscular mycorrhiza, Medicago truncatula
Citation: Gavrin A, Kulikova O, Bisseling T and Fedorova EE (2017) Interface Symbiotic Membrane Formation in Root Nodules of Medicago truncatula: the Role of Synaptotagmins MtSyt1, MtSyt2 and MtSyt3. Front. Plant Sci. 8:201. doi: 10.3389/fpls.2017.00201
Received: 07 December 2016; Accepted: 02 February 2017;
Published: 20 February 2017.
Edited by:
Andrea Genre, University of Turin, ItalyReviewed by:
Viktor Zarsky, Charles University, CzechiaChristian Staehelin, Sun Yat-sen University, China
Copyright © 2017 Gavrin, Kulikova, Bisseling and Fedorova. This is an open-access article distributed under the terms of the Creative Commons Attribution License (CC BY). The use, distribution or reproduction in other forums is permitted, provided the original author(s) or licensor are credited and that the original publication in this journal is cited, in accordance with accepted academic practice. No use, distribution or reproduction is permitted which does not comply with these terms.
*Correspondence: Elena E. Fedorova, elena.fedorova@wur.nl