- 1United States Department of Agriculture-Agricultural Research Service, Plant Germplasm Introduction and Testing Research, Prosser, WA, United States
- 2College of Animal Science and Veterinary Medicine, Heilongjiang Bayi Agricultural University, Daqing, China
Salinity tolerance is highly desirable to sustain alfalfa production in marginal lands that have been rendered saline. In this study, we used a diverse panel of 198 alfalfa accessions for mapping loci associated with plant growth and forage production under salt stress using genome-wide association studies (GWAS). The plants were genotyped using genotyping-by-sequencing (GBS). A greenhouse procedure was used for phenotyping four agronomic and physiological traits affected by salt stress, including dry weight (DW), plant height (PH), leaf chlorophyll content (LCC), and stomatal conductance (SC). For each trait, a stress susceptibility index (SSI) was used to evaluate plant performance under stressed and non-stressed conditions. Marker-trait association identified a total of 42 markers significantly associated with salt tolerance. They were located on all chromosomes except chromosome 2 based on the alignment of their flanking sequences to the reference genome (Medicago truncatula). Of those identified, 13 were associated with multiple traits. Several loci identified in the present study were also identified in previous reports. BLAST search revealed that 19 putative candidate genes linked to 24 significant markers. Among them, B3 DNA-binding protein, Thiaminepyrophosphokinase and IQ calmodulin-binding motif protein were identified among multiple traits in the present and previous studies. With further investigation, these markers and candidates would be useful for developing markers for marker-assisted selection in breeding programs to improve alfalfa cultivars with enhanced tolerance to salt stress.
Introduction
Salinity affects crop production in approximately 830 million hectares worldwide and the acreage is increasing in the arid and semi-arid regions where saline water is used for irrigation (Rengasamy, 2006). Alfalfa (Medicago sativa L.) is the most widely grown forage crop with a high yield and nutritive value. Soil salinity causes a significant reduction in alfalfa yield. Alfalfa production decreases when soil salinity is above a threshold of 2.0 ds m−1 (~22 mM NaCl) (Johnson et al., 1992). The development of salt-tolerant alfalfa varieties is highly desirable to sustain alfalfa production in marginal lands that have been rendered saline.
Physiological responses of plants to salinity can be partitioned to two phases: a rapid, osmotic phase that reduces or inhibits growth of young leaves and a slower, ionic phase that accelerates senescence of mature leaves (Munns and Tester, 2008). Affenzeller et al. (2009) found that the ionic of salt stress led to the activation of the endonuclease resulting in programmed cell death that plays an important role in mediating plant adaptive response to salinity. Numerous reports have shown that the chlorophyll content declined under salt stress in leaves (Hernandez et al., 1999; Agastian et al., 2000; Wang and Nil, 2000). It has been reported that stomatal conductance under salt stress is reduced due to changes in cell anatomy (James et al., 2002). Genetic variability of salt tolerance exists within populations and it has been used for selection of salt-tolerant alfalfa (Kapulnik et al., 1989; Johnson et al., 1992). Physiological traits related to salt tolerance have also been investigated and used as factors for improving alfalfa salt tolerance (Ashraf et al., 1986; Monirifar and Barghi, 2009). Although efforts have been made on the selection of salt-tolerant alfalfa using traditional breeding (Smith et al., 1994; Scasta et al., 2012) and genetic engineering (Liu et al., 2011; Zhang and Wang, 2015), few salt-tolerant cultivars have been released.
The traditional breeding strategy for improving alfalfa with salt tolerance is slow due to the genetic complexity of this autotetraploid species. One way to improve breeding selection efficiency is to identify genetic loci associated with salt tolerance and developing diagnostic markers closely linked to the tolerance loci for marker-assisted selection (Lande and Thompson, 1990). Genetic linkage maps have been constructed in both diploid (M. truncatula) and tetraploid alfalfa (M. sativa) using various marker platforms including restriction fragment length polymorphism (RFLP), simple sequence repeat (SSR), random amplified polymorphic DNA (RAPD), and amplified fragment length polymorphism (AFLP) markers. QTLs associated with biomass (Robins et al., 2007b), morphological traits (Robins et al., 2007a), winter hardiness, persistence (Brouwer et al., 2000; Alarcón-Zúñiga et al., 2004), self-fertility (Robins and Brummer, 2010), Stagonospora root and crown rot resistance (Musial et al., 2007), anthracnose resistance (Mackie et al., 2007) and aluminum tolerance (Khu et al., 2010) have been identified in alfalfa. QTLs associated with salt tolerance have been identified in the model legume, M. truncatula and mapped to all chromosomes except chromosomes 5 and 6 (Arraouadi et al., 2011, 2012). In addition, a number of salt-responsive genes have recently been identified and characterized in alfalfa (Jin et al., 2010; Soto et al., 2011).
Yield under abiotic stresses such as drought and high salinity is most likely under the control of multiple genes and interact with environmental factors. Identification of loci that contribute to variation in such complex traits is a primary challenge in plant breeding and population genetics. Genome-wide association studies (GWAS) provide an integrated framework that merges a QTL mapping approach with high-throughput next-generation sequencing methodology to map stress tolerance loci. This framework provides a statistical basis for analyzing marker-trait association using linkage disequilibrium. It has been used with success in both humans (Cardon and Abecasis, 2003; Manolio et al., 2008) and plants. In plants, GWAS have been applied to Arabidopsis (Atwell et al., 2010), rice (Huang et al., 2010), wheat (Tadesse et al., 2015), soybean (Zhang J. et al., 2015; Patil et al., 2016), maize (Liu et al., 2016; Olukolu et al., 2016), and M. truncatula (Stanton-Geddes et al., 2013; Kang et al., 2015). In alfalfa, QTL related to disease resistance (Liu et al., 2014), drought tolerance (Zhang T. et al., 2015) and forage quality (Wang et al., 2016) have been identified using GWAS. Most recently, we used GWAS and identified salt tolerance loci during germination in alfalfa (Yu et al., 2016).
To better understand the genetic base of salt tolerance in alfalfa, in the present study, we performed GWAS on traits related to plant growth and forage production under salt stress in the same panel of alfalfa accessions previously used for mapping loci associated with drought tolerance (Zhang T. et al., 2015) and salt tolerance during germination (Yu et al., 2016). We then compared SNPs significantly associated with salt tolerance within this study and between the present and previous studies. Our goal is to identify loci linked to forage yield under salt stress and understand the genetic base by which alfalfa germination, growth and forage production are affected by salt stress.
Materials and Methods
Plant Materials and Experimental Conditions
A total of 198 accessions selected from the USDA-ARS National Plant Germplasm System (NPGS) alfalfa collection (http://www.ars-grin/npgs.gov) were used and previously described (Zhang J. et al., 2015; Yu et al., 2016). Single representative plants were grown and clonally propagated in the USDA greenhouse in Prosser, WA. The propagated plants were then transplanted individually into 4-inch square pots filled with commercial potting soil (Pro-Mix) and grown in a greenhouse at 22°C and 40% relative humidity. The light density was approximately 200 μmol m−2s−1 (16 h/day). To ensure plants were approximately the same size, the plants were trimmed to 5 cm length once they began to flower. Fertilizer (Technigro® 20-20-20 All Purpose, Sun Gro Horticulture Canada Ltd.) was applied as needed.
The clonally propagated plants from each accession were divided into two groups: Group 1 for salt stress and group 2 for non-treatment control. Each group contained 3 replications with a complete randomized design. The plants in both groups were watered regularly with tap water until 21 days after transplanting. After that, group 1 for salt treatment were watered with saline water containing 4.68 g/L (equivalent to 80 mM) NaCl 4 weeks until harvesting. Group 2 for control were continuously watered with tape-water throughout the experiment.
Measurement of Traits
Before harvesting, the plant height (PH) of each plant was measured from the soil surface to the top of the main plant stem using a centimeter ruler.
The above-ground biomass was harvested and the dry weight (DW) was measured after drying at 80°C for 2 days in the oven.
The stomatal conductance (SC) of the leaf was measured using an SC-1 Leaf Porometer (Decagon Devices, Inc., Pullman, WA) on the leaf lamina of fully expanded leaves (avoiding the mid-rib and edges). Two measurements were performed on different leaves of the same plant. The mean value was used for evaluating the stomatal conductance of each plant.
Leaf chlorophyll content (LCC) was measured using a Leaf Chl Meter (FT Green LLC, Wilmington, DE) on the leaf lamina of fully expanded leaves (avoiding the mid-rib and edges). The measurements were performed on two different leaves of the same plant. The mean value was used for evaluating LCC of each plant.
The soil electrical conductivity (Soil EC) was measured with a ProCheck (Decagon Devices, Inc., Pullman, WA) to control the actual salt concentration imposed on each plant. The measurements were performed on two different positions within the soil of the same pot.
Phenotypic Data Analysis
To evaluate plant response to salt stress, a stress susceptibility index (SSI) was used for all traits tested. It is calculated according to Fischer and Maurer (1978) as follows:
Where, Ys = performance of a specific accession under stress; Yn = performance of the same accession without stress; Ms and Mn are the mean values of performance overall of accessions in the given test under stress and non-stress conditions, respectively.
A statistical analysis was conducted for each trait. The equality of variance and means were analyzed by using ANOVA. Meanwhile, a normality test was performed for each trait using the Kolmogorov-Smirnov tests. Pearson correlation was calculated between traits using trait means. All analyses were conducted using SPSS 19 (http://www.ibm.com/software/analytics/spss/).
Broad-sense heritability (H2) was calculated using the formula H2 = VG/(VG+VE), where VG and VE were genotypic variance and environmental variance, respectively. The genotypic coefficient of variation (GCV) was calculated using the formula of
Where, X is average of each trait of the whole association panel (Ahsan et al., 2015).
SNP Genotyping
The same SNP data as described in our previous report (Yu et al., 2016) were used in the present study. Briefly, DNA was extracted from young leaves of the original plants of 198 genotypes using the Qiagen DNeasy 96 Plant kit (Qiagen, CA), according to the manufacturer's protocol. A Nanodrop 1000 spectrophotometer was used for quantifying DNA at 260 nm absorbance (Thermo Scientific, http://www.thermoscientific.com). GBS libraries were prepared by digestion of DNA with the EcoT221 restriction enzyme and ligated to barcode and common adaptors as described by Elshire et al. (2011). They were placed in two lanes of an Illumina Hi-Seq2000 instrument for sequencing using 100-base single-end at Cornell University Sequencing facility (Ithaca, NY).
A GBS pipeline consisting of a sequence of software tools was used for genotype calling according to Glaubitz et al. (2014). The detailed procedure was described in Zhang T. et al. (2015).
The GBS sequences were submitted to the NCBI Sequence Read Archive with bioproject ID: PRJNA287263 and biosample accession numbers: AMN03779142–SAMN03779330.
Genome-Wide Association Study
SNPs were further filtered with a 5% cutoff value for minor allele frequency and 25% for missing data. The remaining 4,653 SNPs were subjected for GWAS. The phenotypic data of plant height, leaf chlorophyll content, stomatal conductance, and dry weight were calculated with SSI and the resulting values were used for GWAS. A mixed linear model (MLM, Q+K model) was used to analyze the marker-trait association using TASSEL (http://www.maizegenetics.net/tassel). A false discovery rate (FDR) of 0.05 was used for multiple test correction to determine significant association according to Benjamini and Hochberg (1995). The FDR has similar function as Bonferroni correction and has been used as the threshold for determining the significance in association mapping.
Results
Phenotypic Variation and Correlation Between Characterized Traits
To evaluate phenotypic variation, we performed a statistical analysis for the four traits using ANOVA. Significant difference was found in each trait among the 198 accessions under both salt stress and control conditions (Table 1). A wide range of coefficient of variation (CV) was observed. The highest CV (82.2%) was found in the dry weight of stressed plants while the LCC of control plants showed lowest CV (18.2%). In addition, CV increased in dry weight, leaf chlorophyll content, stomatal conductance (SC)] and decreased in Soil EC (Table 1). The average values of all traits were much lower in salt stressed plants than those of control plants. The average dry weight and plant height of stressed plants decreased by 43.84 and 17.43%, respectively, compared to the control plants (Table 1, “DW and PH”). For the physiological traits, the average value of stomatal conductance of stressed plants decreased by more than half (57.68%) compared to the control plants (Table 1, “SC”), whereas relatively smaller effect was found on leaf chlorophyll content compared to other traits (Table 1, “LCC”). In contrast, the average value of the soil electrical conductivity was much higher in the stressed (2.08) than that in the control (0.2), indicating salt concentration was much higher in the stressed pots than that in the control (Table 1, “Soil EC”). The broad-sense heritability (H2) of the traits ranged from 8.37% (SC) to 46.08% (DW). A similar trend was observed for the GCV which ranged from 8.36% (SC) to 81.26% (DW).
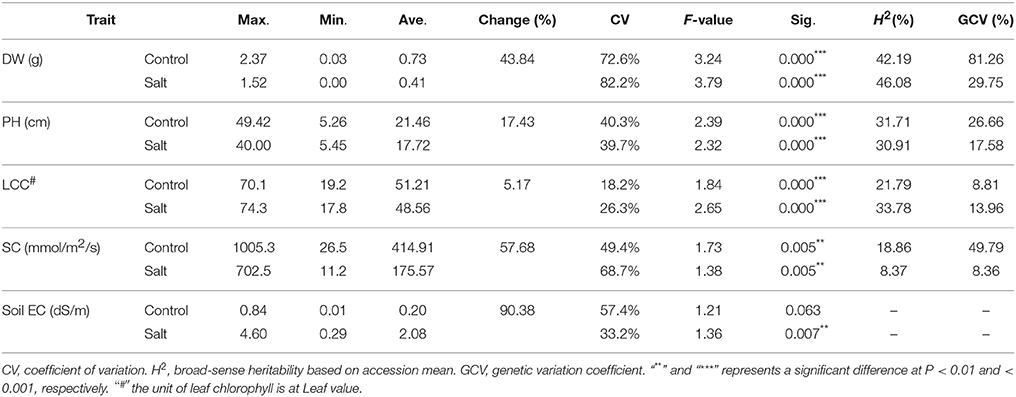
Table 1. Phenotypic variation for four traits including dry weight (DW), plant height (PH), leaf relative chlorophyll content (LCC), stomatal conductance (SC), and soil electrical conductivity (Soil EC) among 198 accessions.
As mentioned in the section of Materials and Methods, stress susceptibility index (SSI) calculates the ratio of yield reduction due to stress in a given genotype as compared to the mean reduction over all genotypes in a given test. The use of SSI is an improved measurement over the simple expression of a trait under stress as a percentage of the trait measured under non-stress conditions. The SSI presents not only phenotypic variation under stress condition but also takes into account of the genetic variability under non-stress condition. We therefore used the resulting SSI values for further analyses. Using these values, normal distributions were obtained for dry weight, plant height, leaf chlorophyll content, stomatal conductance and Soil EC (Figure 1). Correlations among the traits were also analyzed, and their correlation coefficients (r) were presented in Table 2. Positive correlations were obtained between all traits except Soil EC. Among those, the highest correlation was obtained between SSI-DW and SSI-PH (r = 0.604), while the lowest correlation was detected between SSI-DW and SSI-SC (r = 0.169). As expected, the soil electrical conductivity was negatively correlated with all other traits (Table 2).
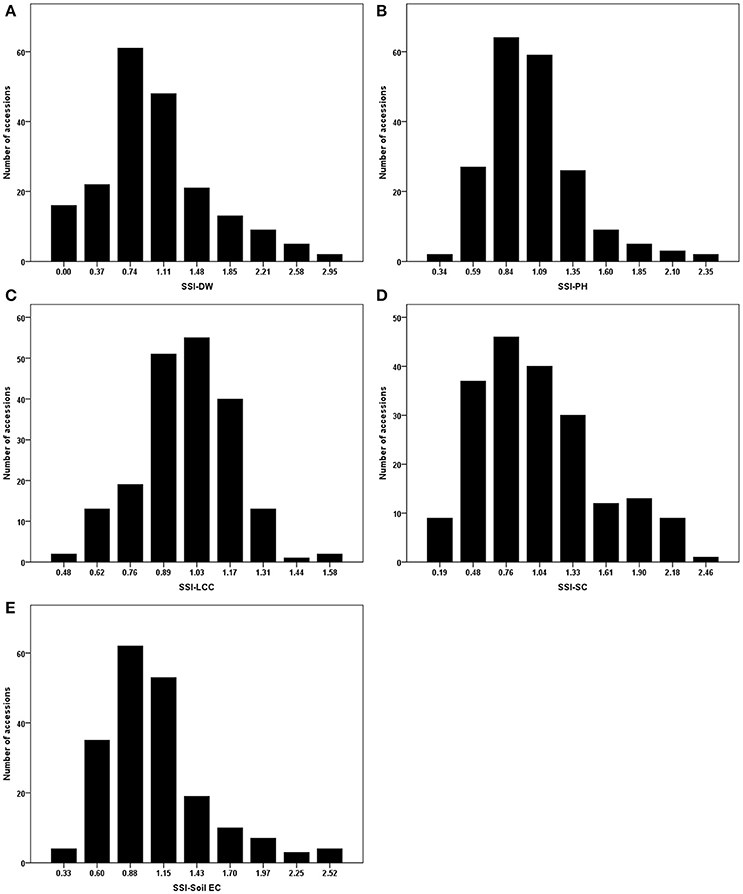
Figure 1. Phenotypic evaluations of stress susceptibility indexes (SSI) for measured traits. (A) SSI-DW, SSI for dry weight; (B) SSI-PH, SSI for plant height; (C) SSI-LCC, SSI for leaf relative chlorophyll content; (D) SSI-SC, SSI for stomatal conductance; (E) SSI-Soil EC, SSI for soil electrical conductivity.
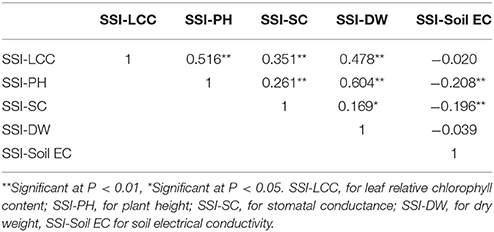
Table 2. Correlation coefficients (r) of SSI-DW, SSI-PH, SSI-LCC, and SSI-SC collected from 198 accessions.
A cluster analysis was also carried out using JMP13 (SAS, Cary, NC). Phenotypic values of all traits were used for hierarchical cluster analysis (Table S1). Two clusters were obtained according to the cluster analysis (Figure 2). Cluster 1 contained 114 accessions and they were tolerant (Figure 2, green) to moderate tolerant (Figure 2, blue) to salt stress based on the phenotypic evaluation (Table S1). Cluster 2 contained 85 genotypes and most of them were sensitive to salt stress (Figure 2, red). Most of accessions in the tolerance cluster were from US and Canada. Five additional accessions (PIs 211606, 211608, 211609, 212104, and 244674) from Afghanistan were also clustered in the resistance group.
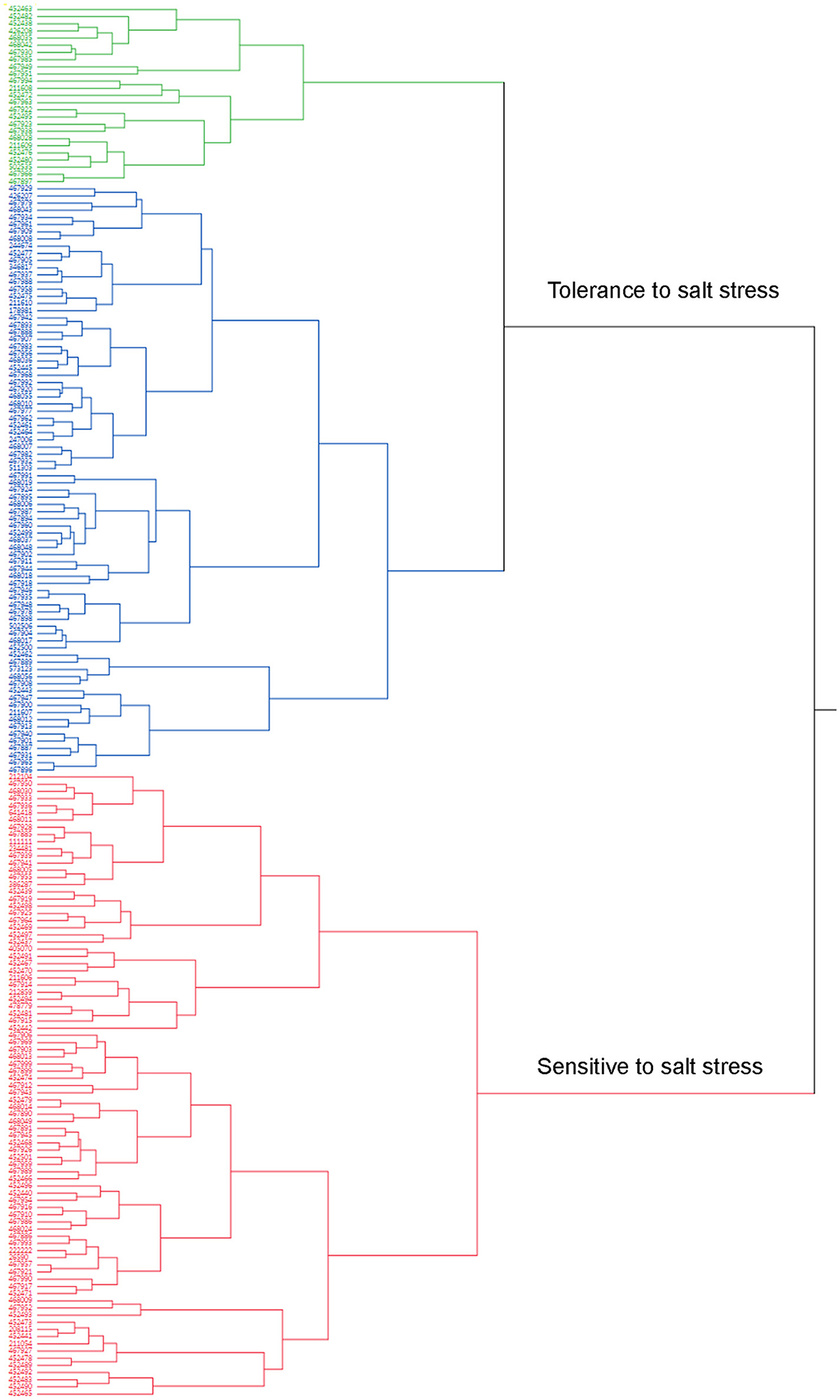
Figure 2. Dendrogram of phenotypic variations of salt tolerance in 198 accessions. Mean values of all traits evaluated in the present study were used for cluster analysis using the hierarchical cluster program built in the JMP13 software (SAS, Cary, NC). Accessions (PIs) were clustered into 2 clusters based on their responses to salt stress (Table S1). Cluster 1 contains 114 accessions (Blue and green colors) with resistant (Green colored) or moderate resistance (Blue) to salt stress. Cluster 2 contains 85 accessions (Red color) and most of them are sensitive to salt stress.
Marker-Trait Association
We first used phenotypic data without converting to SSI values for GWAS. However, the marker-trait association was very low. The use of SSI values helped in increasing significances of marker-trait association as it is not only measuring the plant response to stress but also considering the genotype variation under non-stress condition. So we choose to use the SSI value in the present analysis. Given that salt concentrations may vary from pot to pot, we added Soil EC as covariate to correct the possible variation in the association. However, there is no difference on the results of the association analysis with or without this cofactor. The marker's P-value was used to determine the significance of marker-trait association. The distribution of P-values (log transformed negatives) against chromosomal positions for each trait is presented in the manhattan plots (Figures 3A–D), and the observed P-values against expected P-values are shown in the quantile-quantile (QQ) plots (Figures 3E–H). Using the FDR of 0.05 as described in the Materials and Methods, a total of 42 SNPs were identified to be significantly associated with four traits (Table 3, Figure 3, above dot lines). Most of significant markers (66.67%) were located on Chromosomes 1, 3, 5, and 7. Marker's R2-values ranged from 0.08 to 0.38 with P-values of 1.39E-04 to 1.96E-16 (Table 3). The highest R2-value (R2 = 0.38) was found for marker S1_203399656 and it was significantly associated with dry weight under salt stress. Of those identified, 33, 14, 5, and 7 SNPs were significantly associated with dry weight, plant height, leaf chlorophyll content, and stomatal conductance, respectively. Among them, several markers were significantly associated with multiple traits. For example, Marker S1_6017132 and S1_213944479 were significantly associated with dry weight, chlorophyll content and stomatal conductance. Marker S1_5487587 and S1_5487588 located on chromosome 1 were significantly associated with dry weight, chlorophyll content and plant height. Six markers (S1_165051149, S1_238398605, S1_238398606, S1_238398607, S1_288752900, and S1_356690471) showed significant association with chlorophyll content and dry weight. On the same chromosome, three markers (S1_203399656, S1_90053345, and S1_330116900) were significantly associated with dry weight and plant height. The remaining markers were identified for only one trait.
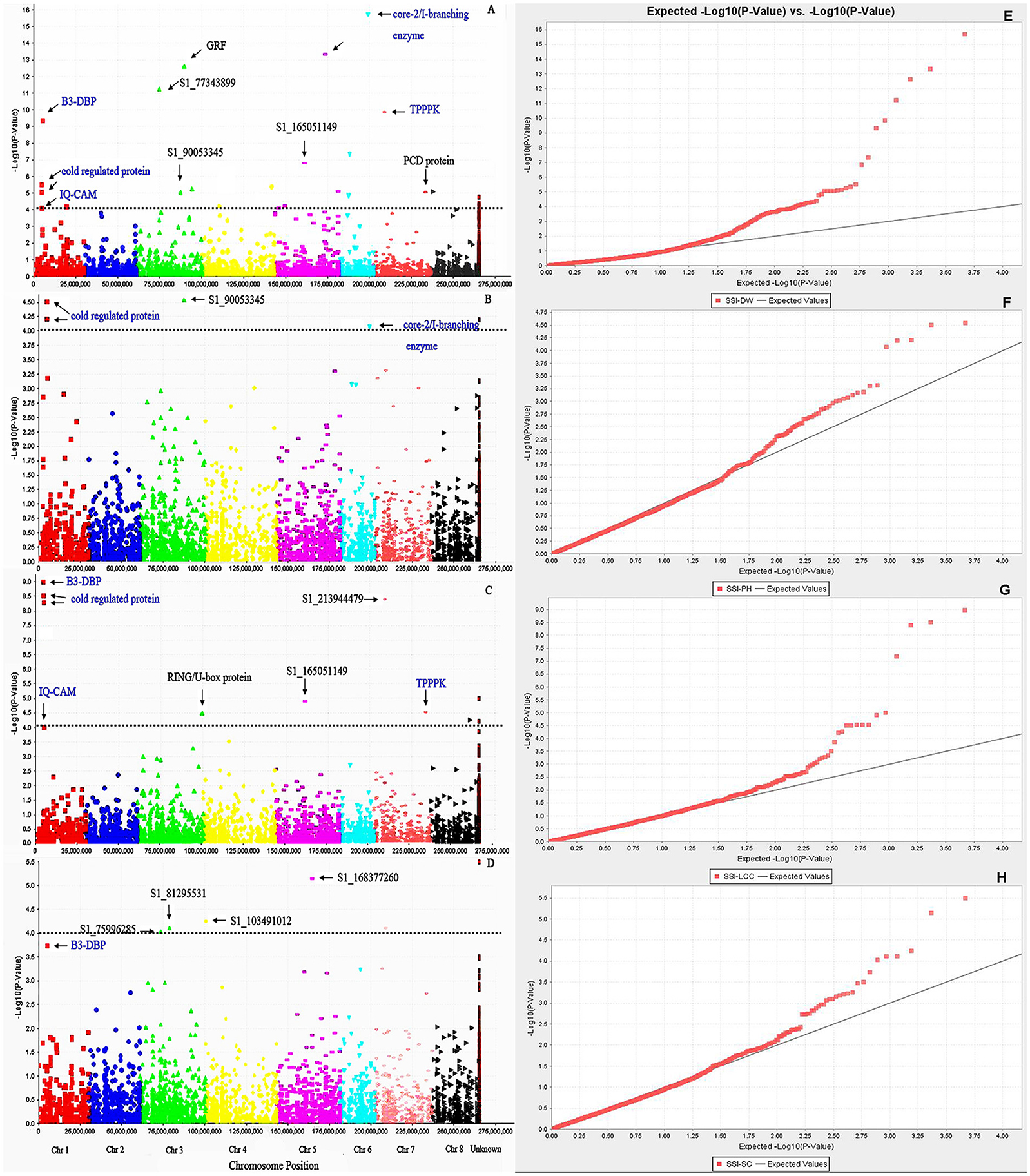
Figure 3. Manhattan plots of marker –trait association for salt tolerance traits: (A) SSI-DW, (B) SSI-LCC, (C) SSI-PH, (D) SSI-SC. Significant markers passed a cutoff –log (P-value) of 4 were above the dot lines. Putative candidate genes linked to significant markers were labeled and those identified in multiple traits were highlighted by blue color. (E–H) represent quantile-quantile (Q-Q) plots from GWAS of SSI-DW, SSI-LCC, SSI-PH, and SSI-SC, respectively. The color curves represent observed p-values (log transformed negatives) of marker-trait association. The black line represents expected p-values.
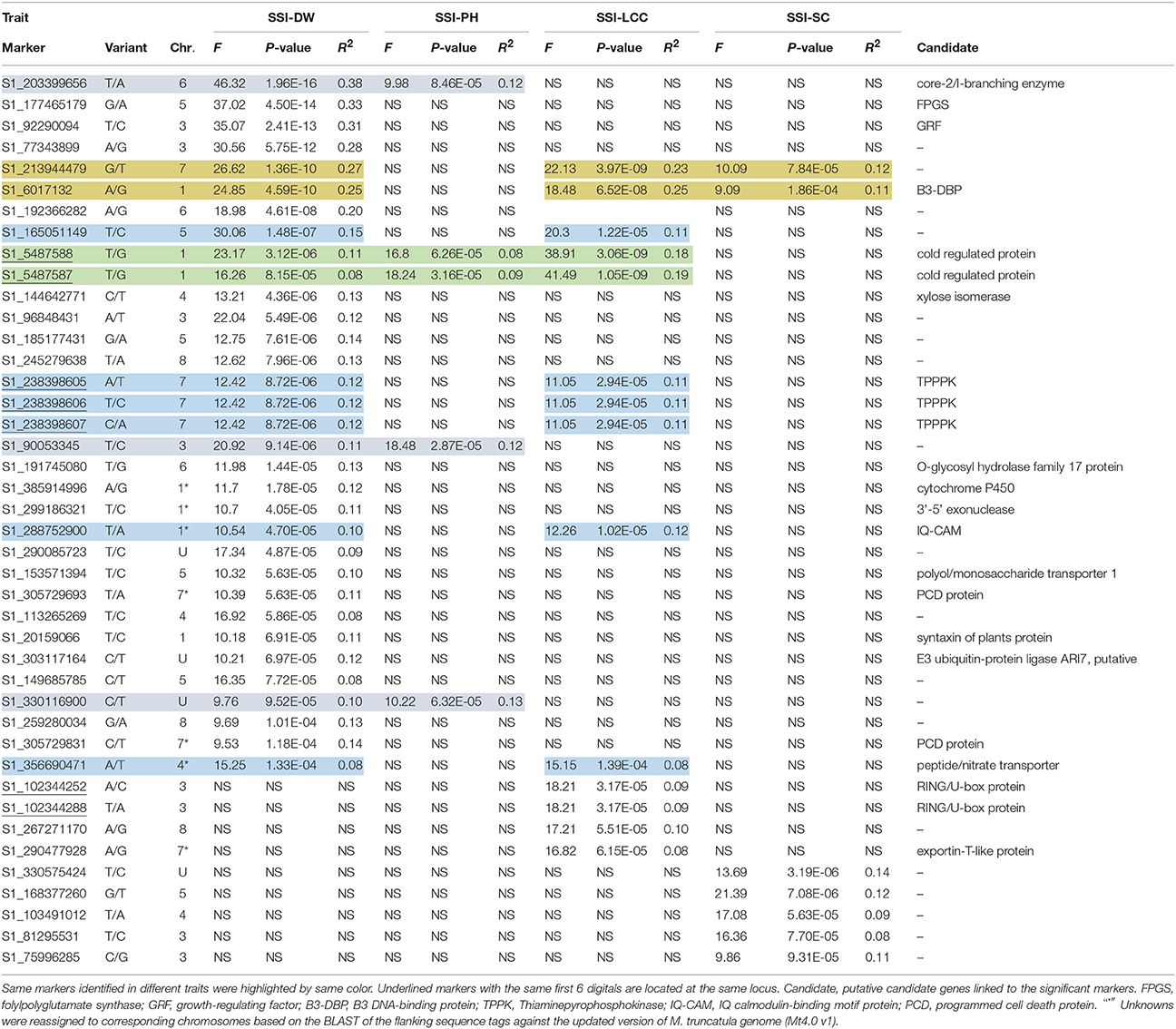
Table 3. Significant SNP markers associated with stress susceptibility indexes (SSI) for measured traits.
Assigning Significant Markers to Candidate Genes
To assess putative candidate genes underlying marker loci for salt tolerance, we performed a BLAST search using the flanking sequences of the significant SNPs against the M. truncatula Mt4.0v1 genome sequences in Phytozome (https://phytozome.jgi.doe.gov/). The BLAST search identified 19 homologs genes to 24 significant markers identified in this study (Figure 3, Table 3). Among them, B3 DNA-binding protein (B3-DBP) showed high homology to marker S1_6017132 on chromosome 1. On the same chromosome, markers S1_5487588 and S1_5487587 at the same locus showed high homology to a cold-regulated protein, and marker S1_288752900 showed homology to IQ calmodulin-binding motif protein (IQ-CAM). On chromosome 3, markers S1_102344252 and S1_102344288 at the same locus showed high homology to RING/U-box protein, and S1_92290094 showed homology to growth-regulating factor (GRF). Marker S1_177465179 on chromosome 5 showed homology to folylpolyglutamate synthase (FPGS). On chromosome 6, markers S1_299186321, S1_191745080, and S1_203399656 showed homology to 3'-5' exonuclease, O-glycosyl hydrolase family 17 protein core-2/I-branching enzyme, respectively. On chromosome 7, Thiaminepyrophosphokinase (TPPPK) showed homology to three markers (S1_238398605, S1_238398606, and S1_238398607) at the same locus. Peptide/nitrate transporter showed homology to S1_356690471 and cytochrome P450 homologs to S1_385914996. Marker S1_305729693 and S1_305729831 at the same locus homolog to a programmed cell death (PCD) protein.
Linkage Disequilibrium Analysis of Significant Markers
To identify the linkage of markers identified in the present study, we performed linkage disequilibrium (LD) analysis using their marker's data by HAPLOVIEW v.4.2 (http://www.broadinstitute.org/haploview/haploview). The D'- and r2-values between two markers were calculated and the result is presented in Figure 4. Among 42 markers identified, we observed 4 strong LDs between SNPs S1_102344252 and 102344288, S1_238398605, and S1_238398606, S1_238398606 and S1_238398607, S1_238398605 and S1_238398607, (Figure 4, red prisms). r2-values between these pairs were 1, suggesting they were tightly linked each other. This was expected as each pair is located at the same locus. Besides the markers with at the same location, high r2-values were also observed between markers S1_259280034 and S1_144642771 (r2 = 0.73), and S1_5487587 and S1_5487588 (r2 = 0.79). The former pair located on different chromosomes (Chromosomes 8 and 4, respectively) while the latter pair is closely located on the same chromosome (chromosome 1). For the remaining SNPs, we did not observe any pair with r2 > 0.4, suggesting that these loci are likely to contribute to phenotypic variations of the respective traits independently.
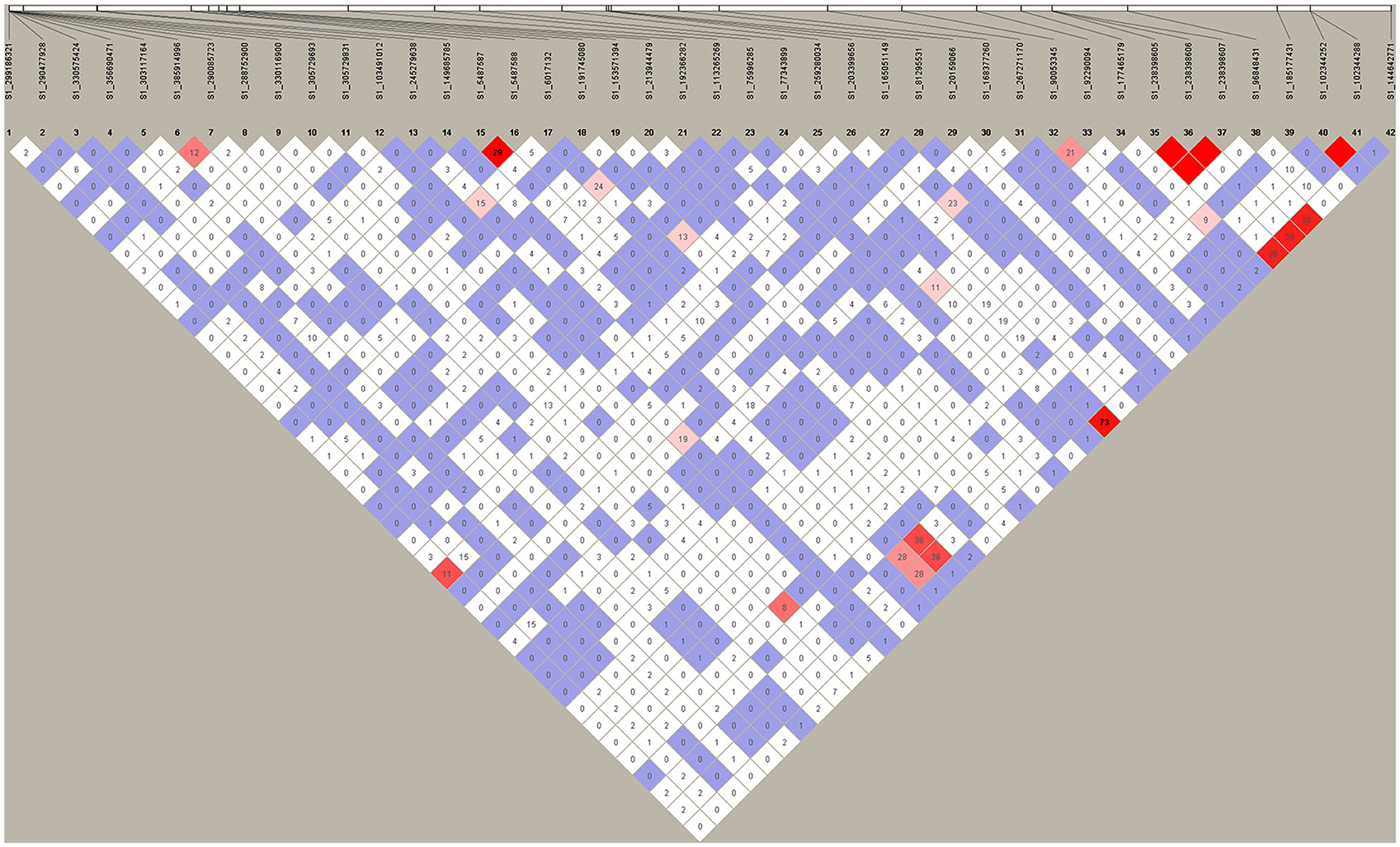
Figure 4. Linkage Disequilibrium (LD) amongst significant markers. HAPLOVIEW v.4.2 (http://www.broadinstitute.org/haploview/haploview) pairwise LD values (r2*100) for 42 SNPs based on genotypes of 198 individuals were used to test whether any of the SNPs significantly associated with salt tolerance were in strong LD with one another. Bright red coloring indicates D' = 1, LOD ≥ 2; Blue coloring indicates D' = 1, LOD < 2; White coloring indicates D' < 1, LOD < 2; Shades of pink/red coloring indicates D' < 1, LOD ≥ 2.
Discussion
Loci Associated with Salt Tolerance in Medicago
Abiotic stresses such as drought and salinity are severely affecting the yield of Medicago. Successful application of genomic tools requires both a good biological knowledge and the mechanisms underlying tolerance to these stresses. However, the complexity of the autotetraploid alfalfa (M. sativa) genome has hampered this goal. M. truncatula has been used as model plant to investigate the genetic bases of nodulation and other important processes such as tolerance to stresses as its small genome and diploid natures (2n = 16) (Dita et al., 2006). Numerous reports have been made on plant responses to salt stress and genes involved in salt tolerance have been identified and characterized in this species (See Rose, 2008 for review). QTLs for salt tolerance have been identified on chromosomes 1 and 8 in M. truncatula using recombinant inbred lines derived from a cross between the tolerant and susceptible parents (Arraouadi et al., 2011, 2012). However, limit report has been made on the genetics of the cultivated alfalfa. We have previously reported the identification of genetic loci associated with germination under salt stress in alfalfa (Yu et al., 2016). In the present study, we identified 42 marker loci associated with forage yield and plant growth (Table 3). Compared to the previous study (Yu et al., 2016), more loci and wider distribution on the genome were identified in the present analysis. This was not surprised since more traits were analyzed in the present than in the previous studies. Moreover, the yield-related traits such as dry weight and plant height analyzed in the present study are more complex and are likely polygenic inheritance. There was a trend toward most significant markers on chromosome 1 in both studies. Among them, the B3-DPB gene was consistently identified for all the traits analyzed in present analysis and germination under salt stress in the previous study. Comparable result was also obtained in M. truncatula (Arraouadi et al., 2012) where most of loci associated with salt related traits were located on chromosome 1. However, markers identified on chromosomes 5 and 6 in M. sativa by the present study were not reported in M. truncatula (Arraouadi et al., 2011, 2012).
Of significant markers identified by the present study, eight (S1_213944479, S1_6017132, S1_192366282, S1_238398607, S1_238398606, S1_238398605, S1_288752900, and S1_259280034) were also identified to be associated with germination under salt stress by our previous study (Yu et al., 2016), suggesting that these loci contribute to both germination and plant growth and yield under salt stress. Whereas, the rest were differentially associated with respective traits. Among markers identified in the present study, five (S1_203399656, S1_5487587, S1_5487588, S1_90053345, and S1_330116900) were identified in both dry weight and plant height suggest that they are likely play roles in forage yield as plant height is one of yield components. Additional markers associated with two or more traits identified in the present study are also critical for salt tolerance as all traits evaluated are related to salt tolerance. With further validation, these markers may be useful for maker-assisted breeding to improve salt tolerance in alfalfa.
Putative Candidate Genes Linked to Marker Loci for Salt Tolerance
Several functional genes linked to the lcoi identified in the present study provide putative underlying candidates for involvement in the plants' response to salt stress. For instance, SNP marker S1_177465179 on chromosome 5, linked to folylpolyglutamate synthase (FPGS), which plays a role in lignin biosynthesis in dicot species (Li et al., 2015). Lignin is a phenolic polymer that reinforces the secondary cell wall, confers structural integrity to the plant, aids in water transport and also plays an important role in the plant's responses to various environmental stresses (Holly and Baxter, 2013). A growth-regulating Factor (GRF) was found to be linked to marker S1_92290094 on chromosome 3. Recent studies have shown that GRF was regulated by osa-MIR396c, one member of the miR396 family that mediates plant salt-alkali stress responses in rice (Gao et al., 2010). On chromosome 1, SNP S1_385914996 was linked to cytochrome P450, which has been identified as a salt-responsive gene strongly induced in root after salt stress in Arabidopsis thaliana (Ma et al., 2006). Another marker, S1_299186321 on chromosome 1, is linked to 3'-5' exonuclease. It has been reported that Exonuclease transcripts accumulate at high levels in senescing leaves when stress is applied (Tang and Sakamoto, 2011). In addition, a programmed cell death protein (PCD) was found to be linked to markers S1_305729693 and S1_305729831. It has been reported that PCD was identified as a candidate gene responsible for plants to survive biotic (Greenberg and Yao, 2004) and abiotic stress such as salinity and cold stress (Kratsch and Wise, 2000; Huh et al., 2002).
A core-2/I-branching enzyme was found to be linked to marker S1_203399556. Core-2/I-branching beta-1, 6-N-acetylglucosaminyltransferase is a member of Core-2/I-Branching enzyme family that has been shown to be inhibited in response to a long exposure to low temperature in grapevine (Vitis spp.) (Kim et al., 2016). Markers S1_102344252 and S1_102344288 on chromosome 3 were linked to a RING/U-box protein. Many plant U-Box genes have been identified as up-regulated in response to salinity conditions (Banzai et al., 2002; Cho et al., 2008).
Cold-regulated protein is a gene linked to S1_5487587 and S1_5487588, which are associated with three traits, SSI-DW, SSI-LCC, and SSI-PH. Cold-regulated protein identified in M. truncatula belongs to the family of WRKY transcription factors (TFs) that play a role in biotic stress associated processes (Wang et al., 2006). It has been reported that the expression of WRKY TFs was significantly altered under salt stress in M. truncatula (Li et al., 2009). Marker S1_6017132 on chromosome 1, linked to B3-DBP, identified in the present study was also identified as one of the most significant markers in response to salt stress during germination in our previous study (Yu et al., 2016). Additionally, the same gene has been identified by screening a cDNA library induced by salt stress in alfalfa by another group (Jin et al., 2010). The identification of B3-DBP, TPPPK, and IQ-CAM in the present and previous investigations (Yu et al., 2016) suggests that they are likely to play roles in response to salt stress.
In conclusion, in the present study, we identified 42 SNP markers highly significant associated with salt-tolerance traits. They are located on all chromosomes except chromosome 2. Of those identified, 13 were associated with multiple traits. Eight loci identified in the present study were also identified in previous reports. BLAST search revealed that 19 putative candidate genes linked to 24 significant markers. Among them, B3 DNA-binding protein, Thiaminepyrophosphokinase, and IQ calmodulin-binding motif protein were identified among multiple traits in the present and previous (Yu et al., 2016) studies, suggesting that they may play critical roles in plant response to salt stress. Additional markers were associated independently to the respective traits. As different mechanisms exist among different traits, loci conferred to the salt tolerance may via different pathways. Understanding their specific roles of the loci identified in this study required additional investigation such as haplotype analysis of individuals. To this end, we will develop more advanced alfalfa populations for salt tolerance in collaboration with other scientists and will validate these loci in a wide-range of germplasm with known levels of resistance to salt stress. The haplotype analysis will be carried out for validation the markers as described by Patil et al. (2016). Once validated, the haplotypes co-segregated with traits for salt tolerance will be used for developing markers for MAS to improve alfalfa cultivars with enhanced salt tolerance, and the putative candidates underlying these loci can be used for further investigations such as gene cloning and functional characterization.
Author Contributions
Conceived and designed the experiments and reviewed the manuscript: LY. Performed the phenotyping association mapping and writing the manuscript: XL.
Funding
This work was supported by The United State Department of Agriculture NIFA_AFRP Grant Number 2015-70005-24071 and the Agriculture Research Service base fund.
Conflict of Interest Statement
The authors declare that the research was conducted in the absence of any commercial or financial relationships that could be construed as a potential conflict of interest.
Acknowledgments
We thank Dr. Charles Hawkins for his critical reading, editing and suggestions regarding the manuscript. We also thank Mr. William Boge and Mrs. Martha Rivera for their technical assistance.
Supplementary Material
The Supplementary Material for this article can be found online at: http://journal.frontiersin.org/article/10.3389/fpls.2017.00853/full#supplementary-material
Table S1. The mean SSI values of phenotypic evaluation of traits related to salt stress in the association panel. SSI-LCC, for leaf relative chlorophyll content; SSI-PH, for plant height; SSI-SC, for stomatal conductance; SSI-RWC, SSI for relative water content; SSI-DW, for dry weight.
References
Affenzeller, M. J., Dareshouri, A., Andosch, A., Lütz, C., and Lütz-Meindl, U. (2009). Salt stress-induced cell death in the unicellular green alga Micrasterias denticulata. J. Exp. Bot. 60, 939–954. doi: 10.1093/jxb/ern348
Agastian, P., Kingsley, S., and Vivekanandan, M. (2000). Effect of salinity on photosynthesis and biochemical characteristics in mulberry genotypes. Photosynthetica 38, 287–290. doi: 10.1023/A:1007266932623
Ahsan, M. Z., Majidano, M. S., Bhutto, H., Soomro, A. W., Panhwar, F. H., Channa, A. R., et al. (2015). Genetic variability, coefficient of variance, heritability and genetic advance of some Gossypium hirsutum L. accessions. J. Agric. Sci. 7, 147–151. doi: 10.5539/jas.v7n2p147
Alarcón-Zúñiga, B., Brummer, E. C., Scott, P., Moore, K., and Luth, D. (2004). “Quantitative trait loci mapping of winter hardiness metabolites in autotetraploid alfalfa,” in Molecular Breeding of Forage and Turf. Developments in Plant Breeding, Vol. 11, eds A. Hopkins, Z.-Y. Wang, R. Mian, M. Sledge and R. E. Barker (Dordreht: Kluwer Academia Publishers), 94–107.
Arraouadi, S., Badri, M., Abdelly, C., Huguet, T., and Aouani, M. E. (2012). QTL mapping of physiological traits associated with salt tolerance in Medicago truncatula recombinant inbred lines. Genomics 99, 118–125. doi: 10.1016/j.ygeno.2011.11.005
Arraouadi, S., Chardon, F., Huguet, T., Aouani, M. E., and Badri, M. (2011). QTLs mapping of morphological traits related to salt tolerance in Medicago truncatula. Acta Physiol. Plant. 33, 917–926. doi: 10.1007/s11738-010-0621-8
Ashraf, M., McNeilly, T., and Bradshaw, A. D. (1986). The response to NaCl and Ionic content of selected salt-tolerant and normal lines of three legume forage species in sand culture. New Phytol. 104, 463–471. doi: 10.1111/j.1469-8137.1986.tb02913.x
Atwell, S., Huang, Y. S., Vilhjalmsson, B. J., Willems, G., Horton, M., Li, Y., et al. (2010). Genome-wide association study of 107 phenotypes in Arabidopsis thaliana inbred lines. Nature 465, 627–631. doi: 10.1038/nature08800
Banzai, T., Hershkovits, G., Katcoff, D. J., Hanagata, N., Dubinsky, Z., and Karube, I. (2002). Identification and characterization of mRNA transcripts differentially expressed in response to high salinity by means of differential display in the mangrove, Bruguiera gymnorrhiza. Plant Sci. 162, 499–505. doi: 10.1016/S0168-9452(01)00601-X
Benjamini, Y., and Hochberg, Y. (1995). Controlling the false discovery rate: a practical and powerful approach to multiple testing. J. R. Stat. Soc. 57, 289–300.
Brouwer, D. J., Duke, S. H., and Osborn, T. C. (2000). Mapping genetic factors associated with winter hardiness, fall growth, and freezing injury in autotetraploid alfalfa Crop Sci. 40, 1387–1396. doi: 10.2135/cropsci2000.4051387x
Cardon, L. R., and Abecasis, G. R. (2003). Using haplotype blocks to map human complex trait loci. Trends Genet. 19, 135–140. doi: 10.1016/s0168-9525(03)00022-2
Cho, S. K., Ryu, M. Y., Song, C., Kwak, J. M., and Kim, W. T. (2008). Arabidopsis PUB22 and PUB23 are homologous U-Box E3 ubiquitin ligases that play combinatory roles in response to drought stress. Plant Cell 20, 1899–1914. doi: 10.1105/tpc.108.060699
Dita, M. A., Rispail, N., Prats, E., Rubiales, D., and Singh, K. B. (2006). Biotechnology approaches to overcome biotic and abiotic stress constraints in legumes. Euphytica 147, 1–24. doi: 10.1007/s10681-006-6156-9
Elshire, R. J., Glaubitz, J. C., Sun, Q., Poland, J. A., Kawamoto, K., Buckler, E. S., et al. (2011). A robust, simple genotyping-by-sequencing (GBS) approach for high diversity species. PLoS ONE 6:e19379. doi: 10.1371/journal.pone.0019379
Fischer, R., and Maurer, R. (1978). Drought resistance in spring wheat cultivars. I. Grain yield responses. Aust. J. Agric. Res. 29, 897–912. doi: 10.1071/AR9780897
Gao, P., Bai, X., Yang, L., Lv, D., Li, Y., Cai, H., et al. (2010). Over-expression of osa-MIR396c decreases salt and alkali stress tolerance. Planta 231, 991–1001. doi: 10.1007/s00425-010-1104-2
Glaubitz, J. C., Casstevens, T. M., Lu, F., Harriman, J., Elshire, R. J., Sun, Q., et al. (2014). TASSEL-GBS: a high capacity genotyping by sequencing analysis pipeline. PLoS ONE 9:e90346. doi: 10.1371/journal.pone.0090346
Greenberg, J. T., and Yao, N. (2004). The role and regulation of programmed cell death in plant-pathogen interactions. Cell. Microbiol. 6, 201–211. doi: 10.1111/j.1462-5822.2004.00361.x
Hernandez, J., Campillo, A., Jimenez, A., Alarcon, J., and Sevila, F. (1999). Response of antioxidant systemsand leaf water relations to NaCl stress in pea plants. New Phytol. 141, 241–251. doi: 10.1046/j.1469-8137.1999.00341.x
Holly, L., and Baxter, C. N. S. (2013). Effects of altered lignin biosynthesis on phenylpropanoid metabolism and plant stress. Biofuels 4, 635–650. doi: 10.4155/bfs.13.56
Huang, X., Wei, X., Sang, T., Zhao, Q., Feng, Q., Zhao, Y., et al. (2010). Genome-wide association studies of 14 agronomic traits in rice landraces. Nat. Genet. 42, 961–967. doi: 10.1038/ng.695
Huh, G.-H., Damsz, B., Matsumoto, T. K., Reddy, M. P., Rus, A. M., Ibeas, J. I., et al. (2002). Salt causes ion disequilibrium-induced programmed cell death in yeast and plants. Plant J. 29, 649–659.
James, R., Rivelli, A., Munns, R., and Von Caemmerer, S. (2002). Factors affecting CO2 assimilation, leaf injury and growth in salt-stressed durum wheat. Funct. Plant Biol. 29, 1393–1403. doi: 10.1071/FP02069
Jin, H., Sun, Y., Yang, Q., Chao, Y., Kang, J., Jin, H., et al. (2010). Screening of genes induced by salt stress from Alfalfa. Mol. Biol. Rep. 37, 745–753. doi: 10.1007/s11033-009-9590-7
Johnson, D. W., Smith, S. E., and Dobrenz, A. K. (1992). Selection for increased forage yield in alfalfa at different NaCl levels. Euphytica 60, 27–35. doi: 10.1007/BF00022255
Kang, Y., Sakiroglu, M., Krom, N., Stanton-Geddes, J., Wang, M., Lee, Y.-C., et al. (2015). Genome-wide association of drought-related and biomass traits with HapMap SNPs in Medicago truncatula. Plant Cell Environ. 38, 1997–2011. doi: 10.1111/pce.12520
Kapulnik, Y., Teuber, L., and Phillips, D. (1989). Lucerne (Medicago sativa L.) selected for vigor in a nonsaline environment maintained growth under salt stress. Aust. J. Agric. Res. 40, 1253–1259. doi: 10.1071/AR9891253
Khu, D. M., Reyno, R., Brummer, E. C., Bouton, J. H., and Monteros, M. J. (2010). “QTL mapping of aluminum tolerance in tetraploid alfalfa,” in Sustainable Use of Genetic Diversity in Forage and Turf Breeding, ed C. Huyghe (Dordrecht: Springer), 437–442.
Kim, S. A., Ahn, S. Y., and Yun, H. K. (2016). Transcriptome analysis of grapevine shoots exposed to chilling temperature for four weeks. Hortic. Environ. Biotechnol. 57, 161–172. doi: 10.1007/s13580-015-0118-5
Kratsch, H. A., and Wise, R. R. (2000). The ultrastructure of chilling stress. Plant Cell Environ. 23, 337–350. doi: 10.1046/j.1365-3040.2000.00560.x
Lande, R., and Thompson, R. (1990). Efficiency of marker-assisted selection in the improvement of quantitative traits. Genetics 124, 743.
Li, D., Su, Z., Dong, J., and Wang, T. (2009). An expression database for roots of the model legume Medicago truncatula under salt stress. BMC Genomics 10:517. doi: 10.1186/1471-2164-10-517
Li, L., Hill-Skinner, S., Liu, S., Beuchle, D., Tang, H. M., Yeh, C. T., et al. (2015). The maize brown midrib4 (bm4) gene encodes a functional folylpolyglutamate synthase. Plant J. 81, 493–504. doi: 10.1111/tpj.12745
Liu, C., Weng, J., Zhang, D., Zhang, X., Yang, X., Shi, L., et al. (2014). Genome-wide association study of resistance to rough dwarf disease in maize. Eur. J. Plant Pathol. 139, 205–216. doi: 10.1007/s10658-014-0383-z
Liu, N., Xue, Y., Guo, Z., Li, W., and Tang, J. (2016). Genome-wide association study identifies candidate genes for starch content regulation in maize kernels. Front. Plant Sci. 7:1046. doi: 10.3389/fpls.2016.01046
Liu, Z.-H., Zhang, H.-M., Li, G.-L., Guo, X.-L., Chen, S.-Y., Liu, G.-B., et al. (2011). Enhancement of salt tolerance in alfalfa transformed with the gene encoding for betaine aldehyde dehydrogenase. Euphytica 178, 363–372. doi: 10.1007/s10681-010-0316-7
Ma, S., Gong, Q., and Bohnert, H. J. (2006). Dissecting salt stress pathways. J. Exp. Bot. 57, 1097–1107. doi: 10.1093/jxb/erj098
Mackie, J. M., Musial, J. M., Armour, D. J., Phan, H. T. T., Ellwood, S. E., Aitken, K. S., et al. (2007). Identification of QTL for reaction to three races of Colletotrichum trifolii and further analysis of inheritance of resistance in autotetraploid lucerne. Theor. Appl. Genet. 114, 1417–1426. doi: 10.1007/s00122-007-0527-z
Manolio, T. A., Brooks, L. D., and Collins, F. S. (2008). A HapMap harvest of insights into the genetics of common disease. J. Clin. Invest. 118, 1590–1605. doi: 10.1172/JCI34772
Monirifar, H., and Barghi, M. (2009). Identification and selection for salt tolerance in alfalfa (Medicago sativa L.) ecotypes via physiological traits. Notulae Sci. Biol. 1, 4. doi: 10.15835/nsb.1.1.3498
Munns, R., and Tester, M. (2008). Mechanisms of salinity tolerance. Ann. Rev. Plant Biol. 59, 651–681. doi: 10.1146/annurev.arplant.59.032607.092911
Musial, J. M., Mackie, J. M., Armour, D. J., Phan, H. T. T., Ellwood, S. E., Aitken, K. S., et al. (2007). Identification of QTL for resistance and susceptibility to Stagonospora meliloti in autotetraploid lucerne. Theor. Appl. Genet. 114, 1427–1435. doi: 10.1007/s00122-007-0528-y
Olukolu, B. A., Tracy, W. F., Wisser, R., De Vries, B., and Balint-Kurti, P. J. (2016). A genome-wide association study for partial resistance to maize common rust. Phytopathology 106, 745–751. doi: 10.1094/phyto-11-15-0305-r
Patil, G., Do, T., Vuong, T. D., Valliyodan, B., Jeong-Dong Lee, J.-D., et al. (2016). Genomic-assisted haplotype analysis and the development of highthroughput SNP markers for salinity tolerance in soybean. Sci. Rep. 6:19199. doi: 10.1038/srep19199
Rengasamy, P. (2006). World salinization with emphasis on Australia. J. Exp. Bot. 57, 1017–1023. doi: 10.1093/jxb/erj108
Robins, J. G., Bauchan, G. R., and Brummer, E. C. (2007a). Genetic mapping forage yield, plant height, and regrowth at multiple harvests in tetraploid alfalfa (Medicago sativa L.). Crop Sci. 47, 11–18. doi: 10.2135/cropsci2006.07.0447
Robins, J. G., and Brummer, E. C. (2010). QTL underlying self-fertility in tetraploid alfalfa. Crop Sci. 50, 143–149. doi: 10.2135/cropsci2009.02.0104
Robins, J. G., Luth, D., Campbell, T. A., Bauchan, G. R., He, C., Viands, D. R., et al. (2007b). Genetic mapping of biomass production in tetraploid alfalfa. Crop Sci. 47, 1–10. doi: 10.2135/cropsci2005.11.0401
Rose, R. (2008). Medicago truncatula as a model for understanding plant interactions with other organisms, plant development and stress biology: past, present and future. Funct. Plant Biol. 35, 253–264. doi: 10.1071/FP07297
Scasta, J. D., Trostle, C. L., and Foster, M. A. (2012). Evaluating alfalfa (Medicago sativa L.) cultivars for salt tolerance using laboratory, greenhouse and field methods. J. Agric. Sci. 4, 90–103. doi: 10.5539/jas.v4n6p90
Smith, S. E., Johnson, D. W., Conta, D. M., and Hotchkiss, J. R. (1994). Using climatological, geographical, and taxonomic information to identify sources of mature-plant salt tolerance in alfalfa. Crop Sci. 34, 690–694. doi: 10.2135/cropsci1994.0011183X003400030017x
Soto, G., Stritzler, M., Lisi, C., Alleva, K., Pagano, M. E., Ardila, F., et al. (2011). Acetoacetyl-CoA thiolase regulates the mevalonate pathway during abiotic stress adaptation. J. Exp. Bot. 62, 5699–5711. doi: 10.1093/jxb/err287
Stanton-Geddes, J., Paape, T., Epstein, B., Briskine, R., Yoder, J., Mudge, J., et al. (2013). Candidate genes and genetic architecture of symbiotic and agronomic traits revealed by whole-genome, sequence-based association genetics in Medicago truncatula. PLoS ONE 8:e65688. doi: 10.1371/journal.pone.0065688
Tadesse, W., Ogbonnaya, F. C., Jighly, A., Sanchez-Garcia, M., Sohail, Q., Rajaram, S., et al. (2015). Genome-wide association mapping of yield and grain quality traits in winter wheat genotypes. PLoS ONE 10:e0141339. doi: 10.1371/journal.pone.0141339
Tang, L. Y., and Sakamoto, W. (2011). Tissue-specific organelle DNA degradation mediated by DPD1 exonuclease. Plant Signal. Behav. 6, 1391–1393. doi: 10.4161/psb.6.9.16595
Wang, D., Amornsiripanitch, N., and Dong, X. (2006). A genomic approach to identify regulatory nodes in the transcriptional network of systemic acquired resistance in plants. PLoS Pathog. 2:e123. doi: 10.1371/journal.ppat.0020123
Wang, Y., and Nil, N. (2000). Changes in chlorophyll, ribulosebiphosphate carboxylase oxygenase, glycine betaine content, photosynthesis and transpiration in Amaranthus tricolor leaves during salt stress. J. Hort. Sci. Biotechnol. 75, 623–627. doi: 10.1080/14620316.2000.11511297
Wang, Z., Qiang, H., Zhao, H., Xu, R., Zhang, Z., Gao, H., et al. (2016). Association mapping for fiber-related traits and digestibility in alfalfa (Medicago sativa). Front. Plant Sci. 7:331. doi: 10.3389/fpls.2016.00331
Yu, L. X., Liu, X., Boge, W., and Liu, X. P. (2016). Genome-wide association study identifies loci for salt tolerance during germination in autotetraploid alfalfa (Medicago sativa L.) using genotyping-by-sequencing. Front. Plant Sci. 7:956. doi: 10.3389/fpls.2016.00956
Zhang, J., Song, Q., Cregan, P. B., Nelson, R. L., Wang, X., Wu, J., et al. (2015). Genome-wide association study for flowering time, maturity dates and plant height in early maturing soybean (Glycine max) germplasm. BMC Genomics 16, 217. doi: 10.1186/s12864-015-1441-4
Zhang, T., Yu, L. X., Zheng, P., Li, Y., Rivera, M., Main, D., et al. (2015). Identification of loci associated with drought resistance traits in heterozygous autotetraploid alfalfa (Medicago sativa L.) using genome-wide association studies with genotyping by sequencing. PLoS ONE 10:e0138931. doi: 10.1371/journal.pone.0138931
Keywords: GWAS, alfalfa, SNP, linkage disequilibrium, salt tolerance
Citation: Liu X-P and Yu L-X (2017) Genome-Wide Association Mapping of Loci Associated with Plant Growth and Forage Production under Salt Stress in Alfalfa (Medicago sativa L.). Front. Plant Sci. 8:853. doi: 10.3389/fpls.2017.00853
Received: 24 January 2017; Accepted: 08 May 2017;
Published: 24 May 2017.
Edited by:
Michael Eric Schranz, Wageningen University and Research Centre, NetherlandsReviewed by:
Magdalena Maria Julkowska, King Abdullah University of Science and Technology, Saudi ArabiaGunvant Baliram Patil, University of Minnesota, United States
Copyright © 2017 Liu and Yu. This is an open-access article distributed under the terms of the Creative Commons Attribution License (CC BY). The use, distribution or reproduction in other forums is permitted, provided the original author(s) or licensor are credited and that the original publication in this journal is cited, in accordance with accepted academic practice. No use, distribution or reproduction is permitted which does not comply with these terms.
*Correspondence: Long-Xi Yu, longxi.yu@ars.usda.gov