- Department of Resources and Environment, Resources and Environment College, Henan Agricultural University, Zhengzhou, China
Nitrogen (N) is critical for zinc (Zn) absorption into plant roots; this in turn allows for Zn accumulation and biofortification of grain in winter wheat (Triticum aestivum L.), an important food crop. However, little is known about root morphology and subcellular Zn distribution in response to N treatment at different levels of Zn supply. In this study, two nutrient solution culture experiments were conducted to examine Zn accumulation, Zn absorption kinetics, root morphology, and Zn subcellular distribution in wheat seedlings pre-cultured with different N concentrations. The results showed positive correlations between N and Zn concentrations, and N and Zn accumulation, respectively. The findings suggested that an increase in N supply enhanced root absorption and the root-to-shoot transport of Zn. Nitrogen combined with the high Zn (Zn10) treatment increased the Zn concentration and consequently its accumulation in both shoots and roots. The maximum influx rate (Vmax), root length, surface area, and volume of 14-d-old seedlings, and root growth from 7 to 14 d in the medium N (N7.5) treatment were higher, but the Michaelis constant (Km) and minimum equilibrium concentrations (Cmin) in this treatment were lower than those in the low (N0.05) and high (N15) N treatments, when Zn was supplied at a high level (Zn10). Meanwhile, there were no pronounced differences in the above root traits between the N0.05Zn0 and N7.5Zn10 treatments. An increase in N supply decreased Zn in cell walls and cell organelles, while it increased Zn in the root soluble fraction. In leaves, an increase in N supply significantly decreased Zn in cell walls and the soluble fraction, while it increased Zn in cell organelles under Zn deficiency, but increased Zn distribution in the soluble fraction under medium and high Zn treatments. Therefore, a combination of medium N and high Zn treatments enhanced Zn absorption, apparently by enhancing Zn membrane transport and stimulating root development in winter wheat. An increase in N supply was beneficial in terms of achieving a balanced distribution of Zn subcellular fractions, thus enhancing Zn translocation to shoots, while maintaining normal metabolism.
Introduction
Zinc (Zn) deficiency is a common nutritional disorder in humans, affecting billions of people worldwide, particularly those in developing countries, where diets are often based on cereal grains with low Zn concentration (Welch and Graham, 2004; Cakmak et al., 2010). In China, Zn deficiency affects ~100 million people who live in rural areas (Ma et al., 2008).
In many countries, wheat is the main dietary component and the most important source of both calories and protein (Cakmak, 2008). In China, the Northern Winter Wheat Region contributes about 70% of national wheat production (Zhuang, 2003). However, Zn deficiency is common in the calcareous soils of Northern China, and this is becoming a limitation for the improvement of Zn content in wheat grain for many Chinese provinces (Liu, 1994). Thus, attempts to increase the Zn concentration of wheat grain, when crops are grown in Zn-deficient soils, is an important area of agricultural research (Bouis, 2003; White and Broadley, 2009). The breeding of novel genotypes with high grain Zn content and applying Zn-rich fertilizers are two applicable and sustainable strategies for the long-term improvement of grain Zn content (Bouis, 2003; Pfeiffer and McClafferty, 2007; Cakmak, 2008). Furthermore, studies report that an increase in nitrogen (N) supply to plants shows potential for agronomic Zn biofortification of wheat (Cakmak et al., 2010; Xue et al., 2012). Furthermore, N combined with Zn supply is more effective in increasing grain Zn content than single N or Zn supply, especially when wheat is cultured in Zn deficient soils. Kutman et al. (2010) pointed out that both soil and foliar N application improves Zn concentration in durum wheat grain when Zn supply is adequately high. Li et al. (2015) also report that foliar Zn combined with N increased Zn concentration and bioavailability in wheat grain during a 2-year field experiment. These studies suggest that a combined N/Zn application might be a promising strategy for increasing grain Zn concentrations to address dietary Zn deficiency.
Zinc concentration in wheat grain depends on physiological processes in plants, such as root uptake, root-to-shoot transport, phloem loading, remobilization of Zn from the source tissues into developing seeds, and deposition of Zn in the seed (Kutman et al., 2010). Grain proteins are reported to be contributed for the accumulation of Zn as there are highly positive correlations between seed protein and seed Zn (Peleg et al., 2008). Increasing the levels of N nutrition has been reported to enhance root uptake, root-to-shoot translocation, and remobilization of Zn in wheat (Cakmak et al., 2010; Erenoglu et al., 2011), while also increasing protein concentration in the grain. Meanwhile, a high N supply leads to the long term uptake of Zn resulting in increased Zn accumulation in grain, due to delayed senescence and thus an extended grain-filling period (Yang and Zhang, 2006).
The distribution of Zn at subcellular levels in plant tissues has received significant attention (Rathore et al., 1972; Chardonnens et al., 1999; Li et al., 2006; Pan et al., 2016), due to the importance of the subcellular localization of Zn relative to its functional role in cellular activities (Whatley et al., 1951). However, all these studies were carried out under conditions of excessive Zn. Plants possess a range of detoxifying cellular mechanisms, such as storing Zn within cell walls or leaf vacuoles, which result intolerance to Zn stress (Li et al., 2006). Meanwhile, little information is currently available on the subcellular distribution of Zn in plants that are cultured under conditions of deficient or sufficient Zn supply, especially combined with a varied N supply.
Excessive N fertilization in intensive agricultural areas results in severe environmental problems such as eutrophication, increased greenhouse gas emissions, and soil acidification, and has recently received greater attention in China (Zheng et al., 2004; Guo et al., 2010; Le et al., 2010). Policies related to increased levels of N application have been highlighted by the Department of Agriculture of China, in relation to the key target of balancing crop production and environmental protection.
Our previous studies showed that adequate N supply increases grain yield, total Zn accumulation, and Zn concentration in the different physiological parts of winter wheat under field conditions; of particular interest is the greater accumulation of Zn in grains as compared with other parts (Zhao et al., 2013, 2016). The aim of this study was to: (i) re-examine the influence of different levels of N and Zn application on Zn accumulation; (ii) to investigate Zn absorption kinetics influenced by different levels of N supply; and (iii) to investigate root morphology and Zn subcellular distribution in response to different levels of N supply, combined with Zn supply during hydroponic trials under greenhouse conditions.
Materials and Methods
Greenhouse Conditions
All experiments were performed in a greenhouse under controlled environmental conditions, using a light/dark regime of 14/10 h, corresponding air temperatures of 22/18°C, a photon flux density of ~500 μmol m−2 s−1, and a relative humidity of ~65%.
Solution Culture
Winter wheat (Triticum aestivum. cv Yunong202) seeds were disinfected in a solution of 0.5% NaClO before being germinated in deionized water (resistivity >18.25 MΩ.cm at 25°C) at 25°C for 5 d. After germination, the seedlings were transferred to 4 L plastic containers of nutrient solutions consisting of 2.5 mM K2SO4, 1 mM KH2PO4, 2 mM MgSO4·7H2O, 100 μM ethylene diamine tetraacetic acid (EDTA)-Fe, 46 μM H3BO3, 9 μM MnCl2·4H2O, 0.3 μM CuSO4·5H2O, and 0.02 μM (NH4)6Mo7O24·4H2O. The final pH of the solution was adjusted to 6.0. Different amounts of Zn were added in the form of ZnSO4·7H2O, depending on the experimental or treatment group, and different N supplies were established through the addition of Ca(NO3)2·4H2O. Low N and medium N pots were supplemented with CaCl2·2H2O to ensure adequate Ca concentrations. Quarter-strength and half-strength nutrient solutions were used during the first and second weeks, respectively. Subsequently, full-strength solutions were used until all seedlings were sampled. The solutions were refreshed every 3 d. All vessels used in the experiment were dipped in 5% HCl for 1 week prior to use and were then washed with deionized water a minimum of three times. The water used for the preparation of nutrient solutions was deionized, and all chemical reagents were of analytical grade.
Absorption Kinetics and Subcellular Fractionation Experiments
In the first experiment, 20 seedlings per pot were cultured in a nutrient solution with 0.5 mM (N0.5, low), 7.5 mM (N7.5, medium), or 15 mM (N15, high) N as well as 0 μM (Zn0, without), 1 μM (Zn1, medium), or 10 μM (Zn10, high) Zn supply. The roots of two seedlings were sampled and analyzed for morphological characteristics after cultivation for 7 and 14 d, respectively. The shoots and roots of 14 plants were separately harvested after cultivation for 21 d, oven-dried at 65°C and analyzed for dry weights and elemental concentrations. The separated parts of other wheat plants, comprising leaves, stems, and roots, were immediately frozen in liquid nitrogen and stored at -20°C for further subcellular fractionation analysis.
The second experiment was a time course depletion experiment in which seedlings were pre-cultured in a nutrient solution for 21 d without Zn but with N7.5. To study the effects of varied N supply on the depletion of Zn from the nutrient solution, plants were supplied with N0.5, N7.5, or N15 for the next 24 h. To start the depletion experiment, the roots of the seedlings were washed in a solution containing 0.5 mM CaSO4 and 2 mM 2- (N- morpholine) ethanesulfonic acid (MES) for 30 min and then transferred to flasks containing 300 mL of the absorption solution, which contained macronutrients, micronutrients, and 10 μM ZnSO4·7H2O. The Zn concentration in 3 mL of nutrient solution was measured at 10 different time points (0, 0.5, 1, 2, 4, 6, 8, 10, 12, and 24 h). Throughout the experiment, the volume of solution in the pots was kept constant by adding the respective Zn-free nutrient solutions.
Analysis of Root Morphological Characteristics
Root length, root surface area, root volume, and average root diameter were determined from root images, using the root imaging analysis software WinRHIZO Version 2009 PRO (Regent Instruments, Quebec City, Canada).
Tissue Fractionation
Frozen materials were pretreated according to the method described by Weigel and Jäger (1980) and Li et al. (2006). A 2-g portion of frozen leaf, stem, or root was placed into 50-mL polypropylene centrifuge tubes and homogenized in 20 mL extraction buffer (50 mM Tris-HCl (pH 7.5), 250 mM sucrose, and 1.0 mM dithioerythritol). The homogenate was centrifuged at 300 × g for 30 s and the residue constituted the cell wall fraction. The supernatant was then centrifuged at 10,000 × g for 30 min and the retained pellet formed the cell organelle fraction. The resultant supernatant solution (referred to as the soluble fraction, consisting mostly of vacuoles) was used in subsequent characterization studies as described. All steps were performed at 4°C.
Mineral Analysis
For the Zn analysis, dry samples were ground and digested with 5 mL of HNO3: HClO4 (4:1, v/v). The cell wall and cell organelle fractions of tissues were transferred to 100 mL Erlenmeyer conical flasks containing deionized water, evaporated to dryness, and subjected to acid digestion as described above. The digested samples were diluted to 25 mL with deionized water and concentrations of Zn were determined by flame atomic absorption spectrophotometer (ZEEnit 700, Analytik Jena AG, Germany). The soluble fraction and solutions sampled from the depletion experiment were acidified with HNO3, and then determined using a flame atomic absorption spectrophotometer. For N analysis, dried and ground samples were digested with H2SO4 and H2O2, and the sample solution was determined for total N concentrations according to the Kjeldahl method, using a nitrogen autoanalyzer (BRAN LUEBBE AA3 Autoanalyzer, Germany). Measurements were checked for accuracy of Zn and N determination using certified standard reference materials, purchased from the National Center of Standard Material in China.
Calculations and Statistical Analyses
Zinc and N accumulation (μg plant−1 and mg plant−1, respectively) were calculated from Zn and N concentrations, respectively, multiplied by the dry weight.
Zinc absorption kinetics were estimated using a modified Michaelis–Menten model (Barber, 1979):
where: In is the inflow rate at substrate concentration Cs; Cs is the substrate concentration in the root medium; Vmax is the maximum influx rate at saturating substrate concentration; Cmin is the minimum equilibrium concentration, meaning the substrate concentration in solution at which there is no net inflow (In = 0); and Km is the Michaelis constant, equaling Cs-Cmin, where In is ½ of Vmax. The kinetic parameters of Zn including Vmax (μmol g−1 root fresh weight [FW]h−1), Km (μmol L−1), and Cmin (μmol L−1) were calculated based on the Zn depletion curve according to the method used by Jiang et al. (1995). Firstly, a quadratic curve between times and Zn concentration in solution is fitted using the non-linear regression procedure of Excel 2007:
where: Y represents Zn concentrations in solution at each time; and X represents the depletion time. A negative derivative equation is then obtained through Equation (2):
When X = 0, Y′ equals -b, meaning the maximum change rate of the Zn concentrations in solution. Thus, Vmax is obtained through calculation:
where: v is the volume of absorption solution; and m is the weight of dry root. When Y′ equals –b/2 in Equation (3), then X1 equals –b/4c. Km is calculated by substituting the above X1 into the Equation (2). Finally, when Y′ equals zero in Equation (3), then X2 equals –b/2c. Cmin is also calculated by substituting the above X2 into the Equation (2).
The subcellular fractions of Zn were calculated as a percentage of total Zn in all fractions.
The significance of the effects of treatment and their interaction on the reported traits was evaluated using one-way or two-way analysis of variance (ANOVA), depending on the experimental design. The data are presented as the average of three replicates. Significant differences among means were determined, using Fisher's protected least significant difference (LSD) test at a 5% level (P < 0.05).
Results
Dry Weight of Shoots and Roots and Root-to-Shoot Ratios
According to the results of two-way ANOVA, not only the N and Zn treatments, but also their interaction exerted significant effects (P < 0.05) on the dry weight of shoots and roots (Table S1). An increase in N supply significantly increased shoot and root dry weights at each Zn treatment, with the highest value in N15 (Table 1). However, the root-to-shoot ratio significantly decreased with increasing N supply. Increasing Zn supply significantly increased shoot dry weight in the N7.5 and N15 treatments, and root dry weight in the N15 treatment. When N was supplied at rates of N0.5 or N7.5, the root dry weights also increased with the Zn1 treatment. Root-to-shoot ratios were significantly increased by the N0.5Zn1 treatment, while the N7.5Zn10 treatment significantly decreased root-to-shoot ratios.

Table 1. Shoot and root dry weights and root-to-shoot ratios of winter wheat (Triticum aestivum cv Yunong202) seedlings, pre-cultured with 0.5, 7.5, or 15 mmol N L−1 in a nutrient solution with 0, 1, and 10 μmol Zn L−1 supply for 21 d.
Zn and N Concentrations and Their Accumulation
Two-way ANOVA revealed significant interactive effects of N and Zn treatment on Zn concentrations and its accumulation in shoots and roots (P < 0.01; Table S1). For each N treatment, increasing the Zn supply led to higher Zn concentrations and accumulation in shoots and roots. However, under Zn deficiency, increasing the N supply had no apparent effect on Zn concentration and accumulation in shoots and roots (Figure 1). In both Zn1 and Zn10, Zn concentration and accumulation in shoots were significantly increased by an increase in N supply (Figures 1A,C). Only in the Zn10 treatment did an increase in N supply significantly increase Zn concentration and accumulation in roots (Figures 1B,C).
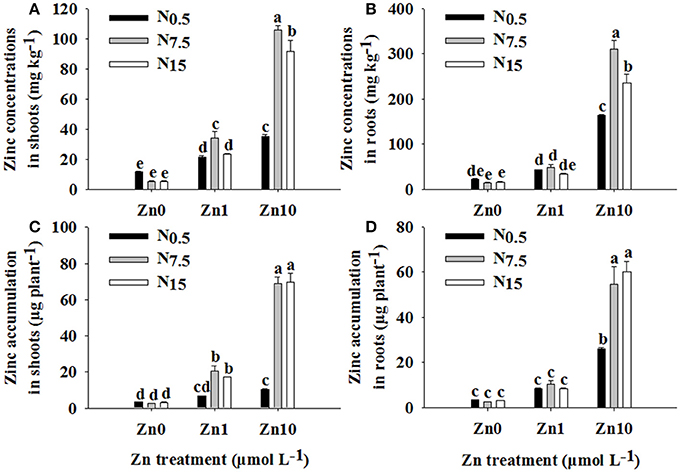
Figure 1. Zinc concentrations in shoots (A) and roots (B) as well as Zn accumulation in shoots (C) and roots (D) of winter wheat (Triticum aestivum cv Yunong202) seedlings grown at 0.5, 7.5, or 15 mmol N L−1 in a nutrient solution with 0, 1, and 10 μmol Zn L−1 supply. Values are means of three independent replicates. Error bars represent 1 SE. For each trait, means followed by different letters are significantly different from each other according to two-way ANOVA followed by least significant difference (LSD) multiple comparison (P < 0.05).
The N and Zn treatments interacted significantly in their effects on N concentration and accumulation in shoots and roots (P < 0.01; Table S1). An increase in N supply resulted in marked increases in N concentration and accumulation in shoots and roots (Figure 2). At N0.5, only the Zn10 treatment significantly increased shoot N concentration. At N7.5, an increase in Zn supply significantly increased shoot N concentration and accumulation, and only the Zn1 treatment significantly increased root N concentration and accumulation. In N15, the Zn10 treatment resulted in higher shoot and root N concentrations and accumulation, while the Zn1 treatment significantly increased shoot N accumulation but decreased root N concentration.
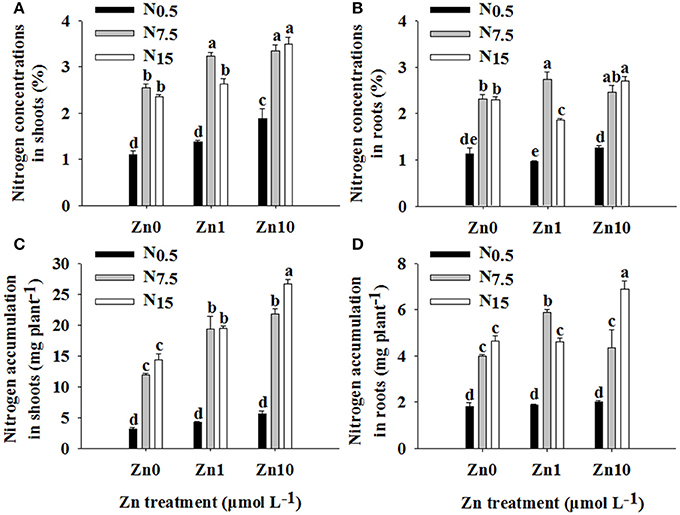
Figure 2. Nitrogen concentrations in shoots (A) and roots (B) and N accumulation in shoots (C) and roots (D) of winter wheat (Triticum aestivum cv Yunong202) seedlings grown at 0.5, 7.5, or 15 mmol N L−1 in a nutrient solution with 0, 1, and 10 μmol Zn L−1 supply. Values are means of three independent replicates. Error bars represent 1 SE. For each trait, means followed by different letters are significantly different from each other according to two-way ANOVA followed by least significant difference (LSD) multiple comparison (P < 0.05).
There were significant positive relationships between shoot N and Zn concentration (r = 0.647**), shoot N and Zn accumulation (r = 0.761**), root N and Zn accumulation (r = 0.445*), and plant N and Zn accumulation (r = 0.674**), respectively.
Zn Absorption Kinetics
In the time-course experiment, the depletion of Zn by plants was followed for 24 h under the three N treatments. N7.5 resulted in the highest level of Zn exhaustion in the solution after 1 h, followed by N15; N0.5 was the slowest to deplete Zn in the solution (Figure 3A). The values of Vmax, Km, and Cmin were strongly dependent on the level of N supply (P < 0.05; Table S2). The value of Vmax was higher, and the values of Km and Cmin were lower in N7.5, compared to N0.5 and N15 (Figures 3B,C).
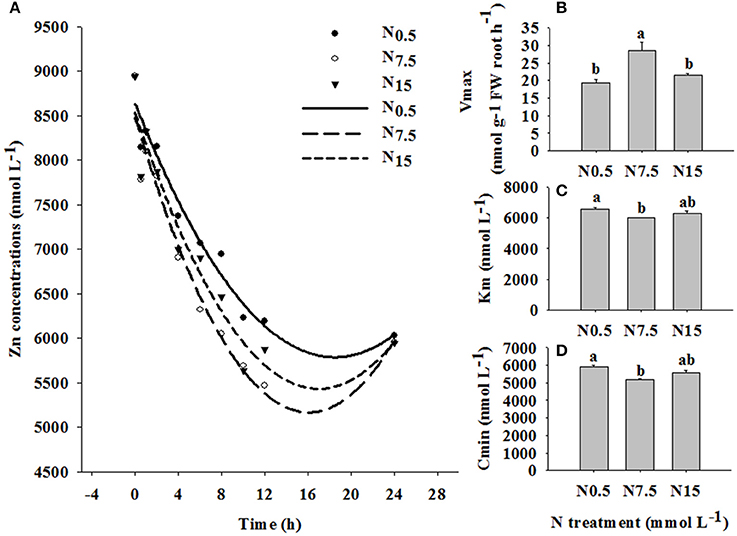
Figure 3. Zinc depletion in solutions containing three winter wheat (Triticum aestivum cv Yunong202) seedlings, grown without Zn and with medium (7.5 mmol L−1) N supply for 21 d and then transferred to solutions supplemented with 10 μmol Zn L−1 and low (0.5 mmol L−1; filled circles), medium (7.5 mmol L−1; open circles), or high (15 mmol L−1; triangles) N supply for varying durations (0–24 h). (A): Zn depletion curve, (B): maximum influx rate Vmax, (C): Michaelis constant Km, (D): minimum equilibrium concentration Cmin. Values are means of three independent replicates. Error bars represent 1 SE. Means followed by different letters are significantly different from each other according to one-way ANOVA followed by least significant difference (LSD) multiple comparison (P < 0.05).
Root Morphology
As revealed by two-way ANOVA, the root length, root surface area, root volume, and root average diameter of 7- and 14-d-old seedlings were significantly affected by N and Zn treatments, and N × Zn interaction (P < 0.05 or P < 0.01; Table S3). In the 7-d-old seedlings, the N7.5 and N15 treatments exhibited a significantly decreased root length for each Zn treatment (Table 2). The root surface area was also significantly decreased by N7.5 and N15 in the Zn1 treatment, and decreased by N15 in the Zn10 treatment. The N15 treatment exhibited a larger root volume under Zn deficiency, but had a smaller root volume than N0.5 and N7.5 under the Zn1 and Zn10 applications. The N7.5 and N15 treatments significantly increased root average diameter with the Zn0 and Zn10 applications, compared to the N0.5 treatment. The Zn10 treatment significantly increased root length, root surface area, and root volume in N0.5 and N7.5. The root surface area, volume, and average diameters were significantly increased by Zn1 with the N0.5 application, but decreased by Zn1 and Zn10 in the N15 application. In the 14-d-old seedlings, when compared to N0.5, the N7.5 treatment significantly increased length, surface area, and volume of roots at Zn10, while no pronounced effects appeared on the above root morphology traits in Zn0 and Zn1 (Table 2). The length, surface area, and volume of roots in N15 were lower than those in N0.5 and N7.5 at Zn0 or Zn1. Increasing Zn supply significantly decreased root length, surface area, and volume at N0.5 or N15. However, N or Zn treatment had no significant effect on the average root diameters of winter wheat.
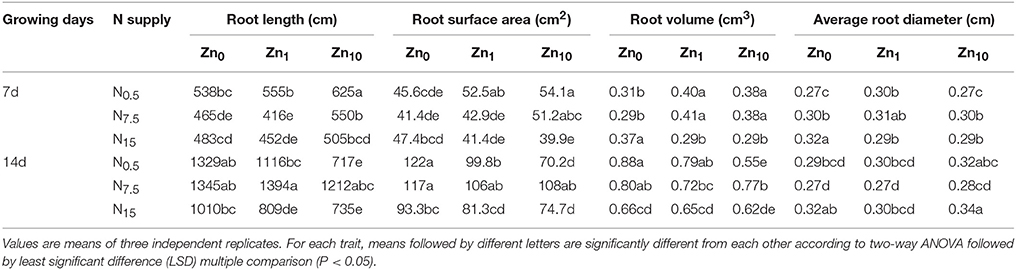
Table 2. Root morphology parameters of winter wheat (Triticum aestivum cv Yunong202) seedlings after 7 and 14 d, at 0.5, 7.5, or 15 mmol N L−1 in a nutrient solution with 0, 1, and 10 μmol Zn L−1 supply.
Zn Subcellular Fraction and Distribution
Zinc concentrations of each tissue fraction were positively affected by both the N and Zn treatments, according to the results of two-way ANOVA (P < 0.05 or P < 0.01; Table S4). Zinc concentration in each fraction of tissue showed a strong increase with increasing Zn supply, with the highest in the Zn10 treatment. Zinc concentration in cell walls and cell organelles of roots were significantly raised by increasing N supply at the Zn10 level. At Zn0 and Zn1, Zn concentrations in root cell walls and cell organelles increased when N supply increased from N0.5 to N7.5, but decreased when N supply increased from N7.5 to N15. The N7.5 treatment had the highest concentrations of Zn in root cell walls and cell organelles (Table 3). An increase in N supply resulted in significant increases in Zn concentration in the soluble fractions of roots for each Zn treatment. For stems of winter wheat, the Zn concentration in cell walls and cell organelles showed a strong decrease in Zn0 and Zn1 treatments with an increase in N supply. However, Zn concentration in cell walls showed a strong increase in Zn10 treatments with an increase in N supply; the N7.5 treatment exhibited higher Zn concentrations in cell organelles than the N0.5 and N15 treatments with the Zn10 application. An increase in N supply led to higher Zn concentrations in the soluble fraction in the Zn1 treatment. Zinc concentration in leaf cell walls and cell organelles were increased by an increase in N supply for each Zn treatment. An increase in N supply decreased Zn concentration in the soluble fraction with Zn0; however, concentration was increased in Zn10.
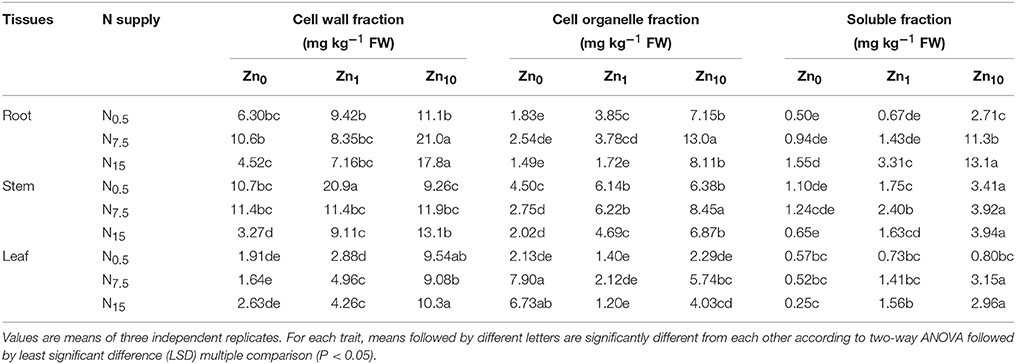
Table 3. Subcellular fractionation of Zn in tissues of winter wheat (Triticum aestivum cv Yunong202) seedlings, grown at 0.5, 7.5, or 15 mmol N L−1 in a nutrient solution with 0, 1, and 10 μmol Zn L−1 supply for 21 d.
The proportion of Zn in the tissues of winter wheat was higher for cell walls than for cell organelles or the soluble fraction, except for a major portion of Zn that was found in leaf cell organelles in the Zn0 treatment (Figure 4). Two-way ANOVA revealed significant effects of N and Zn treatment, and N × Zn interaction on the proportion of Zn in some factions of tissues (P < 0.05 or P < 0.01; Table S5). For each plant part of winter wheat, a decreased proportion of Zn in cell organelles and an increased proportion of Zn in cell walls and soluble fractions were found with increasing Zn supply for each N treatment (Figure 4). The proportion of Zn in root cell organelles showed a strong decrease with an increase in N supply; however, an increased response was observed in root soluble fractions at both Zn1 and Zn10 (Figure 4A). At Zn0 and Zn1, an increase in N supply significantly increased the proportion of Zn in stem soluble fractions (Figure 4B). Zinc proportion in leaf cell walls in the N0.5 treatment was higher than that in the N15 or N7.5 treatments at Zn0 and Zn10 (Figure 4C). An inconsistent result was found for the proportion of Zn in leaf cell organelles.
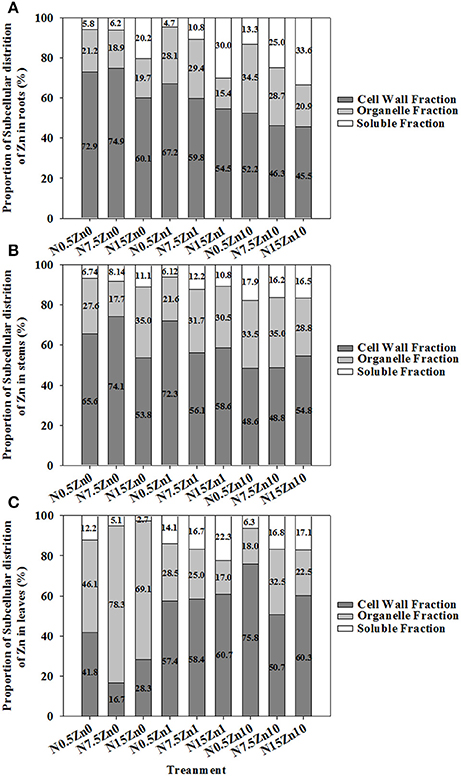
Figure 4. Subcellular distribution of Zn as a percent of total Zn in roots (A), stems (B), and leaves (C) of winter wheat (Triticum aestivum cv Yunong202) seedlings, grown at 0.5, 7.5, or 15 mmol N L−1 in a nutrient solution with 0, 1, and 10 μmol Zn L−1 supply for 21 d. Subcellular distribution of Zn (%) was calculated via dividing the fraction by the total of fractions in each tissue and multiplying the quotient by 100. Values are means of three independent replicates.
Discussion
N7.5 Combined with Zn10 Increased Zn Absorption Through Enhancing Root Growth in Winter Wheat
An increase in N supply positively contributed to root absorption and root-to-shoot transport of Zn; these findings concur with the results of Xue et al. (2012). However, the extent of influence of N supply on Zn concentration and accumulation in both shoots and roots was determined through differing levels of Zn application. Under Zn0, an increase in N supply decreased Zn concentrations in the shoots and roots of winter wheat (Figures 1A,B), which was attributed to a dilution effect caused by an increase in growth as a result of increased N availability (Alloway, 2008). Furthermore, N supply did not significantly contribute to Zn accumulation without an additional Zn supply (Figures 1C,D), which might be due to low concentrations of Zn in the solution, which in turn limited Zn absorption into the roots. For the Zn1 treatment, an increase in N supply increased Zn concentration and accumulation in shoots; however, it had no obvious effect in roots (Figure 1). This suggests that an increase in N supply positively impacted on the root-to-shoot transport of Zn and further Zn accumulation in shoots in Zn1. Enhancement of Zn concentration following an increase in N supply has been suggested due to the growth enhancing effect of N (Aciksoz et al., 2011). However, Xue et al. (2012) report that an increase in shoot growth caused by increased N supply cannot be the major reason for an increase in shoot Zn content. Our results suggested that the increased Zn concentration in shoots might be partially related to the enhanced growth that took place following an increase in N supply. On the one hand, Zn concentration and accumulation in roots were not affected by the N15 treatment (Figures 1B,D), though it significantly increased root dry weight (Table 1). On the other hand, dry weight and Zn concentrations of shoots were increased, and then Zn accumulation was enhanced by N supply (Table 1; Figures 1A,C). This might be because N supply enhanced Zn transfer from roots to shoots, even when a trace amount of Zn was in the solution. For Zn10, an increase in N supply increased Zn concentration and accumulation in both shoots and roots of winter wheat (Figure 1). These results showed that the positive effect of increasing N supply on Zn absorption and root-to-shoot transport became more and more remarkable as Zn application increased up to a high level. The results revealed that the combination of adequate or near-adequate N supply (N7.5 and N15) with high Zn supply (Zn10) was most beneficial to absorption and root-to-shoot transport of Zn than applications of N or Zn alone. Our previous study showed that N combined with a high Zn (30 kg Zn ha−1) treatment enhanced Zn absorption and translocation, and increased Zn accumulation in winter wheat grain that was grown in Zn-deficient soil (Zhao et al., 2016). Thus, in order to increase Zn concentration in wheat grain cultured on Zn-deficient soils, a combination of adequate N with high Zn application is a better choice.
The time-course depletion experiment also revealed the enhanced effect of increasing N supply on the Zn absorption kinetics in winter wheat. In the Zn10 treatment, the depletion of Zn from solution was significantly increased by N7.5 (Figure 3A). This suggests that the N7.5 treatment enhanced Zn removal from solution, and absorption through at root surface. The value of Vmax was higher, and the values of Km and Cmin were lower for the N7.5 treatment, compared to the N0.5 and N15 treatments (Figures 3B–D). Therefore, enhanced Zn absorption in the N7.5 treatment might be related to the membrane transport systems regulating Zn absorption and transport processes. Erenoglu et al. (2011) also report that increasing N supply significantly enhances root Zn uptake, which is due to the N-enhanced abundance of transporters, including ZIPs such as iron-regulated transporter (IRT). Generally, the expression of transporters related to nutrient uptake is quick in response to the change of environmental factors (Nie et al., 2014), which explains why N supply over only 24 h was able to change Zn absorption in our study. Thus, investigating the effect of N supply on the transporter expression related to Zn absorption and translocation in winter wheat is deserving of further research.
In the current study, when N increased from N7.5 to N15, no further increase in Zn accumulation in shoots or roots was observed. Moreover, the N7.5 treatment significantly increased Zn concentrations in shoots and roots under Zn1 (shoots: N7.5 34.11 mg kg−1 > N15 23.40 mg kg−1; roots: N7.5 48.26 mg kg−1 > N15 33.71 mg kg−1) and Zn10 treatments (shoot: N7.5 105.51 mg kg−1 > N15 91.37 mg kg−1; root: N7.5 310.61 mg kg−1 > N15 236.07 mg kg−1; Figure 1). Similar results were obtained for differences in Zn depletion in solution and the values of Vmax, Km, and Cmin between the N7.5 and N15 treatments (Figure 3). These results might be because the plants already had sufficient Zn under the N7.5 treatment.
Root morphology (root length, surface area, and volume) can reflect the growth conditions of plant roots as it is influenced by environmental factors (Fageria and Moreira, 2011). Our results showed that N7.5 could promote root development by increasing root length, surface area and volume at the Zn10 treatment. Xue et al. (2014) pointed that greater root lengths and surface area due to N application might contribute to the increase in Zn uptake of roots in winter wheat. Therefore, co-supply of N and Zn could stimulate roots development might be one reason why Zn absorption was enhanced. With a further increase in N supply to N15, root development of 7- and 14-d-old seedlings was inhibited, compared to the N0.5 treatment. However, compared to N0.5, N15 inhibited the growth of roots but still enhanced Zn absorption and accumulation in winter wheat, suggesting that interference with the Zn membrane transport process might be another reason for increasing Zn absorption following high rates of N application (Erenoglu et al., 2011).
In short, adequate N combined with high Zn supply increased Vmax and decreased Km and Cmin, and affected Zn membrane transport to enhance plant ability to absorb Zn. On the other hand, it increased root length, root surface area and root volume, and promoted root growth and development to enhance the absorption of Zn from soil solution.
An Increase in N Supply Was Beneficial to a Balanced Distribution of Zn between Subcellular Fractions
In our study, the subcellular distribution of Zn was higher in cell walls than in cell organelles or soluble fractions in most treatments, except that a greater amount of Zn was present in the cell organelles of leaves in Zn0 (Table 3; Figure 4). It was speculated that winter wheat could transfer more Zn into cell organelles to maintain normal metabolism under Zn deficiency. Our results were consistent with results of Pan et al. (2016), who noted that, at toxic levels, the majority of Zn was localized in cell walls. This indicated that the cell wall was the primary site of Zn storage, whether Zn was present at sufficient or excessive levels in the soil.
An increase in N supply had diverse effects on the subcellular distribution of Zn in different tissues or at different levels of Zn application. In the Zn0 and Zn1 treatments, Zn distribution in root cell walls and cell organelles were significantly decreased by an increase in N supply (Table 3; Figure 4A). In contrast, an increase in N supply enhanced Zn distribution in the root soluble fraction. A decreased distribution of Zn in cell walls of roots and stems through an increase in N supply was beneficial to Zn translocation to shoots, as the accumulation of trace metals in cell walls would inhibit migration into the protoplast (Krzesłowska, 2011). An increase in N supply significantly increased Zn concentrations in each subcellular fraction in the Zn10 treatment (Table 3), which was related to the increased Zn concentrations seen with the high Zn treatment. However, an increase in N supply still decreased Zn distribution in root cell walls and cell organelles, while an increase occurred in root soluble fractions (Figure 4A). In leaves under the Zn0 treatment, an increase in N supply decreased the portion of Zn in cell walls and in the soluble fraction, and increased the portion of Zn in cell organelles (Figure 4C). This suggested that an increase in N supply could enhance Zn transport to cell organelles, thus maintaining a normal exchange of materials and signals between cells and the external environment when Zn is deficient (Hacisalihoglu and Kochian, 2003; Alloway, 2008). In Zn1 and Zn10, Zn concentrations, and distribution in soluble fractions were increased by an increase in N supply, indicating that N could enhance the compartmentalization of Zn in vacuoles, which are the main compartment for the soluble fraction (Weigel and Jäger, 1980).
Conclusions
This study demonstrated that an adequate N supply enhanced Zn absorption and Zn accumulation in shoots. We found significant and positive correlations between N and Zn concentrations and accumulation. The results suggested that increased N supply positively contributed to root absorption and root-to-shoot transport of Zn. The N7.5 treatment significantly increased the value of Vmax, while it decreased the values of Km and Cmin. A combination of N7.5 and Zn10 treatments stimulated root development by increasing root length, surface area, and volume of 14-d-old seedlings, and led to a change in root size from 7 to 14 d. This indicated that the positive effects of a combination of N7.5 and Zn10 on high affinity between the Zn uptake transporters and stimulated root development might contribute to Zn absorption and its accumulation in winter wheat. In roots, Zn distribution in cell walls and cell organelles was significantly decreased, and that in soluble fractions was increased by an increase in N supply. By contrast, in leaves, an increase in N supply significantly decreased Zn in cell walls and soluble fractions, and increased Zn in cell organelles under Zn0; however, it increased Zn distribution in the soluble fractions of Zn1 and Zn10. These results suggest that an increase in N supply enhanced Zn translocation to shoots, thus maintaining normal metabolism in winter wheat.
Author Contributions
HL conceived and designed the experiments. JW and JL performed the experiments. PZ analyzed the data. ZN wrote the paper.
Conflict of Interest Statement
The authors declare that the research was conducted in the absence of any commercial or financial relationships that could be construed as a potential conflict of interest.
Acknowledgments
This study was supported by the National Natural Science Foundation of China (Program Nos. 41501311; 41201286) and the Key Scientific Research Project for the Universities of Henan Province (Program No. 17A210002).
Supplementary Material
The Supplementary Material for this article can be found online at: http://journal.frontiersin.org/article/10.3389/fpls.2017.01435/full#supplementary-material
Abbreviations
Vmax, maximum influx rate; Km, Michaelis constant; Cmin, minimum equilibrium concentrations; N nitrogen; Zn, zinc.
References
Aciksoz, S., Yazici, A., Ozturk, L., and Cakmak, I. (2011). Biofortification of wheat with iron through soil and foliar application of nitrogen and iron fertilizers. Plant Soil 349, 215–225. doi: 10.1007/s11104-011-0863-2
Barber, S. A. (1979). “Growth requirements for nutrients in relation to demand at the root surface,” in The Soil-Root Interface, eds J. L. Harley and R. S. Russell (London; New York, NY; San Francisco, CA: Academic Press), 5–20.
Bouis, H. E. (2003). Micronutrient fortification of plants through plant breeding: can it improve nutrition in man at low cost? Proc. Nutr. Soc. 62, 403–411. doi: 10.1079/PNS2003262
Cakmak, I. (2008). Enrichment of cereal grains with zinc: agronomic or genetic biofortification? Plant Soil 302, 1–17. doi: 10.1007/s11104-007-9466-3
Cakmak, I., Pfeiffer, W. H., and McClafferty, B. (2010). Review: biofortification of durum wheat with zinc and iron. Cereal Chem. 87, 10–20. doi: 10.1094/CCHEM-87-1-0010
Chardonnens, A. N., Wilma, M., Vellinga, S., Schat, H., Verkleij, J. A., and Ernst, W. H. (1999). Allocation patterns of zinc and cadmium in heavy metal tolerant and sensitive Silene vulgaris. J. Plant Physiol. 155, 778–787. doi: 10.1016/S0176-1617(99)80096-0
Erenoglu, E. B., Kutman, U. B., Ceylan, Y., Yildiz, B., and Cakmak, I. (2011). Improved nitrogen nutrition enhances root uptake, root-to-shoot translocation and remobilization of zinc (65Zn) in wheat. New Phytol. 189, 438–448. doi: 10.1111/j.1469-8137.2010.03488.x
Fageria, N., and Moreira, A. (2011). The role of mineral nutrition on root growth of crop plants. Adv. Agron. 110, 251–331. doi: 10.1016/B978-0-12-385531-2.00004-9
Guo, J., Liu, X., Zhang, Y., Shen, J., Han, W., Zhang, W., et al. (2010). Significant acidification in major Chinese croplands. Science 327, 1008–1010. doi: 10.1126/science.1182570
Hacisalihoglu, G., and Kochian, L. V. (2003). How do some plants tolerate low levels of soil zinc? Mechanisms of zinc efficiency in crop plants. New Phytol. 159, 341–350. doi: 10.1046/j.1469-8137.2003.00826.x
Jiang, T. H., Zheng, S. J., Shi, J. Q., Hu, A. T., and Shi, R. H. (1995). Several considerations in kinetic research on nutrition uptake by plants. Plant Nutr. Ferti. Sci. 1, 11–17 (in Chinese with English abstract).
Krzesłowska, M. (2011). The cell wall in plant cell response to trace metals: polysaccharide remodeling and its role in defense strategy. Acta Physiol. Plant. 33, 35–51. doi: 10.1007/s11738-010-0581-z
Kutman, U. B., Yildiz, B., Ozturk, L., and Cakmak, I. (2010). Biofortification of durum wheat with zinc through soil and foliar applications of nitrogen. Cereal Chem. 87, 1–9. doi: 10.1094/CCHEM-87-1-0001
Le, C., Zha, Y., Li, Y., Sun, D., Lu, H., and Yin, B. (2010). Eutrophication of lake waters in China: cost, causes, and control. Environ. Manage. 45, 662–668. doi: 10.1007/s00267-010-9440-3
Li, M., Wang, S. X., Tian, X. H., Zhao, J. H., Li, H. Y., Guo, C. H., et al. (2015). Zn distribution and bioavailability in whole grain and grain fractions of winter wheat as affected by applications of soil N and foliar Zn combined with N or P. J. Cereal Sci. 61, 26–32. doi: 10.1016/j.jcs.2014.09.009
Li, T. Q., Yang, X. E., Yang, J. Y., and He, Z. L. (2006). Zn accumulation and subcellular distribution in the Zn hyperaccumulator Sedum alfredii Hance. Pedosphere 16, 616–623. doi: 10.1016/S1002-0160(06)60095-7
Liu, Z. (1994). Regularities of content and distribution of zinc in soils of China. Sci. Agric. Sin. 27, 30–37 (in Chinese with English abstract).
Ma, G., Jin, Y., Li, Y., Zhai, F., Kok, F. J., Jacobsen, E., et al. (2008). Iron and zinc deficiencies in China: what is a feasible and cost-effective strategy? Public Health Nutr. 11, 632–638. doi: 10.1017/S1368980007001085
Nie, Z. J., Hu, C. X., Liu, H. E., Tan, Q. L., and Sun, X. C. (2014). Differential expression of molybdenum transport and assimilation genes between two winter wheat cultivars (Triticum aestivum). Plant Physiol. Biochem. 82, 27–33. doi: 10.1016/j.plaphy.2014.05.002
Pan, X., Chen, G., Shi, C., Chai, M., Liu, J., Cheng, S., et al. (2016). Effects of Zn stress on growth, Zn accumulation, translocation, and subcellular distribution of Spartina alterniflora Loisel. Clean Soil Air Water 44, 579–585. doi: 10.1002/clen.201400288
Peleg, Z., Saranga, Y., Yazici, A., Fahima, T., Ozturk, L., and Cakmak, I. (2008). Grain zinc, iron and protein concentrations and zinc-efficiency in wild emmer wheat under contrasting irrigation regimes. Plant Soil 306, 57–67. doi: 10.1007/s11104-007-9417-z
Pfeiffer, W. H., and McClafferty, B. (2007). Harvest Plus: breeding crops for better nutrition. Crop Sci. 47(Suppl. 3), S88–S105. doi: 10.2135/cropsci2007.09.0020IPBS
Rathore, V., Bajaj, Y., and Wittwer, S. (1972). Subcellular localization of zinc and calcium in bean (Phaseolus vulgaris L.) tissues. Plant Physiol. 49, 207–211. doi: 10.1104/pp.49.2.207
Weigel, H. J., and Jäger, H. J. (1980). Subcellular distribution and chemical form of cadmium in bean plants. Plant Physiol. 65, 480–482. doi: 10.1104/pp.65.3.480
Welch, R. M., and Graham, R. D. (2004). Breeding for micronutrients in staple food crops from a human nutrition perspective. J. Exp. Bot. 55, 353–364. doi: 10.1093/jxb/erh064
Whatley, F., Ordin, L., and Arnon, D. I. (1951). Distribution of micronutrient metals in leaves and chloroplast fragments. Plant Physiol. 26:414. doi: 10.1104/pp.26.2.414
White, P. J., and Broadley, M. R. (2009). Biofortification of crops with seven mineral elements often lacking in human diets–iron, zinc, copper, calcium, magnesium, selenium and iodine. New Phytol. 182, 49–84. doi: 10.1111/j.1469-8137.2008.02738.x
Xue, Y. F., Yue, S. C., Zhang, Y. Q., Cui, Z. L., Chen, X. P., Yang, F. C., et al. (2012). Grain and shoot zinc accumulation in winter wheat affected by nitrogen management. Plant Soil 361, 153–163. doi: 10.1007/s11104-012-1510-2
Xue, Y. F., Zhang, W., Liu, D. Y., Yue, S. C., Cui, Z. L., Chen, X. P., et al. (2014). Effects of nitrogen management on root morphology and zinc translocation from root to shoot of winter wheat in the field. Field Crop. Res. 161, 38–45. doi: 10.1016/j.fcr.2014.01.009
Yang, J., and Zhang, J. (2006). Grain filling of cereals under soil drying. New Phytol. 169, 223–236. doi: 10.1111/j.1469-8137.2005.01597.x
Zhao, P., Yang, F., Sui, F., and Wang, Q. (2013). Effect of combined application of Zn and N fertilizers on nitrogen use, grain yield and protein content in winter wheat. J. China Agric. Univ. 18, 28–33. doi: 10.3321/j.issn:1007-4333.2013.03.004. (Chinese with English abstract).
Zhao, P., Yang, F., Sui, F., Wang, Q., and Liu, H. (2016). Effect of nitrogen fertilizers on zinc absorption and translocation in winter wheat. J. Plant Nutr. 39, 1311–1318. doi: 10.1080/01904167.2015.1106560
Keywords: absorption kinetics, nitrogen, root morphology, subcellular fractionation, zinc
Citation: Nie Z, Zhao P, Wang J, Li J and Liu H (2017) Absorption Kinetics and Subcellular Fractionation of Zinc in Winter Wheat in Response to Nitrogen Supply. Front. Plant Sci. 8:1435. doi: 10.3389/fpls.2017.01435
Received: 20 February 2017; Accepted: 03 August 2017;
Published: 18 August 2017.
Edited by:
Richard William Bell, Murdoch University, AustraliaReviewed by:
Susan Tandy, ETH Zurich, SwitzerlandBrian M. Waters, University of Nebraska Lincoln, United States
Copyright © 2017 Nie, Zhao, Wang, Li and Liu. This is an open-access article distributed under the terms of the Creative Commons Attribution License (CC BY). The use, distribution or reproduction in other forums is permitted, provided the original author(s) or licensor are credited and that the original publication in this journal is cited, in accordance with accepted academic practice. No use, distribution or reproduction is permitted which does not comply with these terms.
*Correspondence: Hongen Liu, bGl1aG9uZ2VuNzE3OEAxMjYuY29t