- 1Key Laboratory of Mollisols Agroecology, Northeast Institute of Geography and Agroecology, Chinese Academy of Sciences, Harbin, China
- 2Division of Plant Science, Research School of Biology, Australian National University, Canberra, ACT, Australia
- 3Centre for AgriBioscience, La Trobe University, Bundoora, VIC, Australia
- 4Key Laboratory of Soybean Cultivation of Ministry of Agriculture, Soybean Research Institute, Heilongjiang Academy of Agricultural Sciences, Harbin, China
Nitrogen deficiency limits crop performance under elevated CO2 (eCO2), depending on the ability of plant N uptake. However, the dynamics and redistribution of N2 fixation, and fertilizer and soil N use in legumes under eCO2 have been little studied. Such an investigation is essential to improve the adaptability of legumes to climate change. We took advantage of genotype-specific responses of soybean to increased CO2 to test which N-uptake phenotypes are most strongly related to enhanced yield. Eight soybean cultivars were grown in open-top chambers with either 390 ppm (aCO2) or 550 ppm CO2 (eCO2). The plants were supplied with 100 mg N kg−1 soil as 15N-labeled calcium nitrate, and harvested at the initial seed-filling (R5) and full-mature (R8) stages. Increased yield in response to eCO2 correlated highly (r = 0.95) with an increase in symbiotically fixed N during the R5 to R8 stage. In contrast, eCO2 only led to small increases in the uptake of fertilizer-derived and soil-derived N during R5 to R8, and these increases did not correlate with enhanced yield. Elevated CO2 also decreased the proportion of seed N redistributed from shoot to seeds, and this decrease strongly correlated with increased yield. Moreover, the total N uptake was associated with increases in fixed-N per nodule in response to eCO2, but not with changes in nodule biomass, nodule density, or root length.
Introduction
Plant demand for nitrogen (N) likely increases under elevated atmospheric CO2 (eCO2). Nitrogen addition enhances CO2 effects on plant productivity. In ryegrass swards, compared to non-N control, N addition resulted in a greater yield response to eCO2 (Schneider et al., 2004). Moreover, eCO2 significantly increased N uptake of wheat (Butterly et al., 2016). It appears that sufficient N supply may lead to optimization of photosynthetic processes to favor the productivity under eCO2 (Ainsworth and Long, 2005; Luo et al., 2006; Langley and Megonigal, 2010).
Therefore, the magnitude of response in plant productivity largely depends on how plant N uptake is capable to keep pace with eCO2-induced stimulation of carbohydrate production and growth. Plants may positively regulate a series of physiological processes, such as secretion of enzymes and root growth, to increase the capacity of plant nutrient acquisition for optimal adaptability to eCO2 (Rogers et al., 2006; Sardans and Peñuelas, 2012). In legumes, symbiotic N2 fixation has been considered as the most influential factor affecting plant N uptake and productivity under eCO2 (Ainsworth et al., 2003). Elevated CO2 increased nodule size and number, specific nitrogenase activity and plant N content, and consequently increased biomass and/or seed yield in legumes such as Trifolium repens, Lupinus albus, Pisum sativum, and Glycine max (Zanetti et al., 1996, 1997; Lee et al., 2003; Rogers et al., 2009; Butterly et al., 2016). However, the responses of symbiotic N2 fixation to eCO2 may vary between legume species and even varieties within a given species. For example, Lam et al. (2012) reported that eCO2 (550 ppm) significantly increased the amount of symbiotic N2 fixation in the soybean (G. max) cultivar Zhonghuang 13 but had no effect in Zhonghuang 35.
Labile N in soil is an important source to satisfy plant N demand under eCO2 (Shimono and Bunce, 2009). Studies have shown that the increased root biomass of crops grown under eCO2 could increase N uptake from soil (Matamala and Schlesinger, 2000; Bertrand et al., 2007). Moreover, Matamala et al. (2003) reported that under eCO2, fine roots are more important for N uptake than total root biomass. However, to our knowledge, the extent of N originating from N2 fixation and soil/fertilizer among the soybean cultivars in response to eCO2 has not been quantified, especially in Mollisol regions where soybean is a major crop (Liu and Herbert, 2002; Yu et al., 2016). Investigating the cultivar variation in N uptake in response to eCO2 is essential to predict the adaptability of soybean cultivars and formulate the N fertilization strategy to increase N-use efficiency in the future.
Besides plant N uptake, the remobilization of N from vegetative to reproductive sinks during the reproductive stages of crop development is an important contributor to maximizing yield in soybean. Because N previously accumulated in vegetative organs can be remobilized to seeds when exogenous N cannot fulfill the N demand in seed filling (Salon et al., 2001; Schiltz et al., 2005), the effect of eCO2 on the dynamics of N accumulation might determine the pattern of N remobilization. It has been reported that the extent of the contribution of N remobilization to seed N varies from 80 to 90% in soybean cultivars (Warembourg and Fernandez, 1985; Kinugasa et al., 2012). However, few studies have investigated the N remobilization of soybean cultivars in response to eCO2.
Therefore, N uptake and its partitioning in plants under eCO2 are important characteristics of phenotypic plasticity in response to climate change. While most previous studies have focused on responses in single genotypes, or compared different unrelated species, our study utilized a group of soybean genotypes that differed in their plastic responses to eCO2. Using the 15N dilution method (Unkovich and Baldock, 2008), we aimed to assess the effect of eCO2 on the origins of plant N, i.e., symbiotically fixed-N, fertilizer N, and soil N, and the correspondent N remobilization during the seed-filling stage. We then correlated these changes with yield stimulation under eCO2. We hypothesized that eCO2 would increase N2 fixation and alter distribution of the fixed-N to seed to contribute to yield gain.
Materials and Methods
Research Site and Experimental Design
A pot experiment was conducted in open-top chambers (OTC) at the Northeast Institute of Geography and Agroecology (45°73′N, 126°61′E), Chinese Academy of Sciences, Harbin, China. The experiment had a random block design comprising two atmospheric CO2 concentration levels and eight soybean cultivars with three replications. The two CO2 levels were ambient CO2 (aCO2; 390 ppm) and eCO2 (550 ppm). Each couple of OTC (one per CO2 treatment) was considered as a block, and they were randomly located in the field site. The eight soybean cultivars were Xiaohuangjin (XHJ, released in 1951), Hejiao 6 (HJ6, released in 1962), Nenfeng 1 (NF1, released in 1972), Nenfeng 9 (NF9, released in 1980), Suinong 8 (SN8, released in 1989), Suinong 14 (SN14, released in 1996), Heinong 45 (HN45, released in 2003), Suinong 22 (SN22, released in 2005). These cultivars have been widely grown in northeast China with a growing area of more than 2 million ha (Jin et al., 2012).
Six octagonal OTC (three for each CO2 concentration) were constructed with a steel frame. The main body of each OTC is 3.5 m in diameter, 2.0 m high and with a 0.5-m high canopy, which formed a 45° angle with the plane (Zhang et al., 2014). The OTC were covered with polyethylene film (transparency ≥95%). This OTC design has been widely used in CO2-associated studies (e.g., Liu et al., 2016; Yu et al., 2016; Chaturvedi et al., 2017). A digital CO2-regulating system (Beijing VK2010, China) was installed to monitor the CO2 level in each OTC and automatically regulate the supply of CO2 gas (99.9%) to achieve CO2 concentrations of 550 ± 30 ppm for eCO2 and 390 ± 30 ppm for aCO2. There were 16 pots per OTC with two pots per cultivar for two harvest time points.
Plant Growth and 15N Labeling
The soil used in this study was classified as a Mollisol, and had an organic C content of 28.3 mg g−1 soil, total N of 2.24 mg g−1 soil, available N of 260 μg g−1 soil, and a pH of 6.97 (1:5 H2O). Nitrogen fertilizer was applied as Ca(NO3)2 with 5% of 15N atom excess at a rate of 100 mg N kg−1 soil. The procedure of 15N labeling is described in Li et al. (2016).
Before sowing, uniform seeds were selected and germinated at 25°C on moistened filter paper. After 2-day germination, six seeds were sown in each pot (20 cm diameter and 40 cm high) containing 9 L soil and thinned to 2 plants 10 days after emergence. Thus, there were six pots per cultivar grown in either aCO2 or eCO2 environment. The pot design was considered appropriate for precise isotope labeling and root sampling (Ainsworth et al., 2002). However, the pot size used in this experiment might limit, to some extent, the plant response to CO2 elevation as Arp (1991) stated that plants grown in pots of 3.5–12.5 L had intermediate responses to eCO2. Soil water content was maintained at 80 ± 5% of field capacity by weighing and watering. In addition, wheat (Triticum aestivum L. cv. Longmai 26) plants were grown under the same conditions as non-N2 fixing reference species (Rennie and Dubetz, 1986) due to lack of suitable non-nodulating isolines, and was harvested at physiological maturity. Although choosing wheat as non-fixing control exhibits some methodological limitations (Unkovich and Baldock, 2008), wheat has been widely used as a reference plant species in many studies to estimate legume N2 fixation (Rennie and Dubetz, 1986; Carranca et al., 1999; Lam et al., 2012).
Harvest and Measurements
Plants of three pots were harvested at the R5 (beginning seed formation, 81 days after sowing) and R8 stages (maturity, 120 days after sowing), respectively (Fehr et al., 1971). Shoots were cut at the cotyledon node level and separated into stems plus petioles, leaves and pods at R5, and additionally seeds at R8. The abscised leaves in each pot between R5 and R8 stages were collected for C and N measurements. The entire root system of each plant was carefully separated from soil, and then washed with tap water to remove soil particles adhering to the roots. Nodules were removed from the root system, counted and weighed. The root length and diameter classes of roots were then determined using WinRhizo 2004b (Régent Instruments Inc., Québec, Canada). According to their diameter, roots were classified as fine roots (<0.5 mm), intermediate roots (0.5–1.0 mm), and coarse roots (>1 mm) (Costa et al., 2002).
All plant samples were dried at 70°C for 72 h, and then finely ground in a ball mill (Retsol MM2000, Retsch, Haan, Germany). The 15N/14N ratio of all samples was measured with an isotope ratio mass spectrometer (Deltaplus, Finnigan MAT GmbH, Bremen, Germany). The C and N contents of plant samples were determined using an ELEMENTAR III analyzer (Hanau, Germany).
Calculations and Statistical Analysis
Atom% 15N excess was calculated with reference to the natural 15N abundance in the atmosphere (0.3663 atom% 15N; Mariotti et al., 1984). The percentage of plant N derived from N2 fixation (%Ndfa) was calculated as follows (Rennie and Dubetz, 1986):
%Ndfa = {1 − [atom% 15N excess (fs)/atom% 15N excess (nfs)]} × 100
where fs and nfs represented fixing and non-fixing (wheat) system, respectively.
N2 fixed was calculated as follows:
N2 fixed (mg plant−1) = (%Ndfa/100) × Nplant(mg plant−1)
where Nplant was the N content of each plant compartment.
The amounts of plant N derived from fertilizer (Ndffplant) and soil (Ndfsplant) were estimated (Martínez-Alcántara et al., 2012) as follows:
Ndffplant = Nplant(mg plant−1) × N atom% 15N excess in plant/N atom%
15N excess in fertilizer (19.83%)
Ndfsplant = Nplant(mg plant−1) − Ndffplant − N2 fixed
The amount of N remobilized from vegetative organs to seeds was estimated as N content in vegetative organs aboveground at R5 subtracted from that at R8 (Egli et al., 1978). Nodule density was calculated as nodule number divided by total root length. Two-way ANOVA on variables including yield components, parameters of plant N, root morphology, nodule number, and nodule fresh weight was performed with Genstat 13 (VSN International, Hemel Hemspstead, UK). Partial correlation analyses were used to evaluate the correlations of N assimilation indices with nodule characteristics, root morphology and yield gain in response to eCO2 (Peng et al., 2004). The least significance difference (LSD) was used to assess the differences among treatments at P = 0.05.
Results
Seed Yield and Seed N Origins
Compared to aCO2, eCO2 increased seed yield by an average of 40% (Figure 1A). The yield response to eCO2 varied among cultivars (P < 0.001), resulting in a 91% increase in XHJ in comparison to 12% in NF1, and leading to a significant CO2× cultivar interaction (P < 0.001). Interestingly, the cultivars showing the highest yield under eCO2 were not the ones showing the highest yield under aCO2, but exhibited the biggest increase in yield gain. In addition, the N content of the seed showed a shift in origin toward greater fixed N under eCO2 (Figure 1B). Overall, there was a strong (P < 0.001) correlation between the increase in fixed-N content of seeds and their yield increase under eCO2 (Figure 1C).
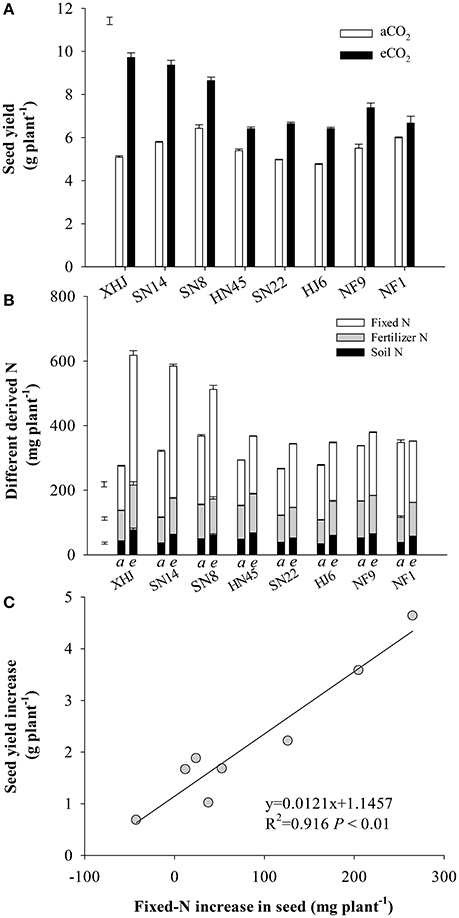
Figure 1. (A) Seed yield, (B) soil-derived N, fertilizer-derived N, and symbiotically fixed-N content in seed and (C) the relationship between increased fixed-N in seeds and yield increment of eight soybean cultivars under eCO2 relative to aCO2. Each data point represents one cultivar. The error bars represent standard error, and separate vertical bar(s) in (A) and (B) indicate the LSD (P < 0.05) for the CO2 × cultivar interaction.
Shoot Biomass and N Content
Shoot biomass at R8 also significantly increased (by 46% on average) under eCO2 compared with aCO2 (Figure S1) with a minimum increase of 22% for HN45 and a maximum of 87% for XHJ (P < 0.001). Compared with aCO2, eCO2 increased shoot N content by 11% at R5, and 41% at R8 (P < 0.05) (Table 1). Among cultivars, the largest increase in shoot N content at R8 in response to eCO2 was observed in XHJ (119%), and the smallest one (7%) in NF1.
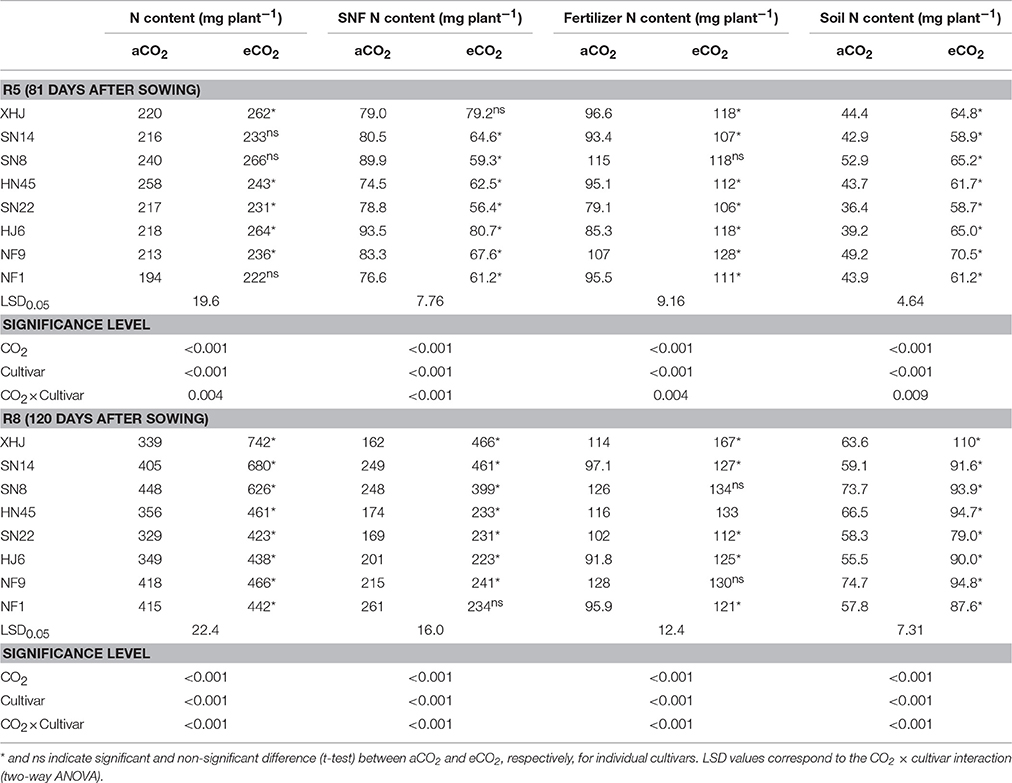
Table 1. Shoot N content, symbiotically fixed-N (SNF) content, fertilizer-derived, and soil-derived N content in shoot of eight soybean cultivars grown under aCO2 or eCO2 till R5 (81 days after sowing) and R8 (120 days after sowing).
Elevated CO2 decreased shoot N concentration (mg g−1) by an average of 30% at R5 (Figure S1). At R8, eCO2 did not affect shoot N concentration in SN8, SN14, HN45, and SN22 (Figure S1), but increased it by 17% in XHJ.
Shoot N Origins
Compared to aCO2, eCO2 decreased the fixed-N content (mg plant−1) of the shoot at R5 (P < 0.05), but significantly increased it at R8 (Table 1). The maximum increase was found in XHJ (188%) while no difference occurred in NF1 (P > 0.05) at R8 (Table 1).
Elevated CO2 increased the accumulation of the fertilizer-derived N in the shoot (mg plant−1) by 20 and 21% at R5 and R8, respectively (Table 1). The extent of increase of fertilizer-derived N under eCO2 differed among cultivars. At R5, the increase in fertilizer-derived N in HJ6 under eCO2 reached 38% compared to aCO2, while there was no CO2 effect in SN8. At R8, eCO2 increased fertilizer-derived N by 46% in XHJ but did not affect it in SN8 and NF9. A significant (P < 0.001) CO2× cultivar interaction was observed at R5 and R8 (Table 1).
Similarly, eCO2 increased the soil-derived N accumulation in the shoot by 45 and 47% at R5 and R8, respectively (Table 1). A significant CO2× cultivar interaction on soil-derived N content in the shoot was observed (Table 1). At R5, soil-derived N content increased by 66% in HJ6 under eCO2 in comparison to 23% in SN8. At R8, XHJ exhibited 73% increase for soil-derived N content, but only 27% increase in SN8 and NF1 was observed. However, overall, there was no significant correlation between yield gain and either soil-derived or fertilizer-derived N uptake under eCO2 (Figure S2).
Under eCO2, the proportion of fixed-N in the shoot at R5 decreased (P < 0.05) by 27% compared to aCO2 (Figure 2A). In contrast, the proportion of fertilizer- and soil-derived N in the shoot at R5 increased by 9.1 and 31%, respectively, under eCO2. At R8, however, eCO2 increased the proportion of fixed-N in the shoot of all cultivars except for HJ6 (−12%) and NF1 (−16%) (Figure 2B). Under eCO2, the proportion of fertilizer-derived N decreased in all cultivars. Elevated CO2 decreased the proportion of soil-derived N in the shoot of XHJ, SN8, and SN14, but increased it in HJ6, NF1, NF9, HN45, and SN22, leading to significant CO2× cultivar interactions (Figure 2B).
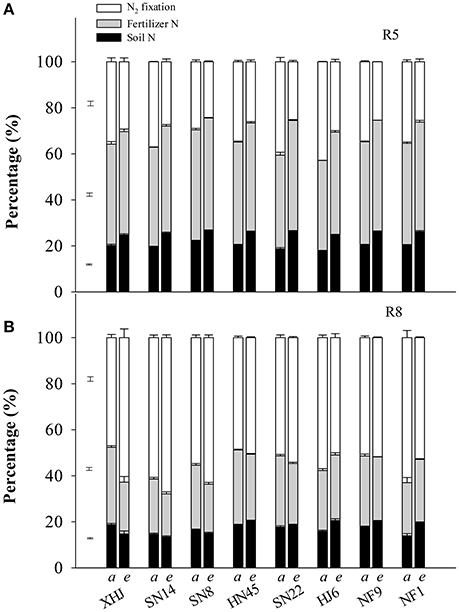
Figure 2. The effect of CO2 on the percentage of plant N derived from symbiotically fixed-, fertilizer-, and soil-N at (A) R5 and (B) R8 (81 and 120 days after sowing, respectively). The error bars represent standard error, and separate vertical bars indicate the LSD (P < 0.05) for the CO2 × cultivar interaction. The letters of a and e on the x-axis indicate aCO2 and eCO2, respectively.
N Remobilization
Elevated CO2 significantly decreased the proportion of the remobilized N in seeds, with the greatest decrease for XHJ and no significant response for HJ6, NF9, and NF1 (Figure 3A).
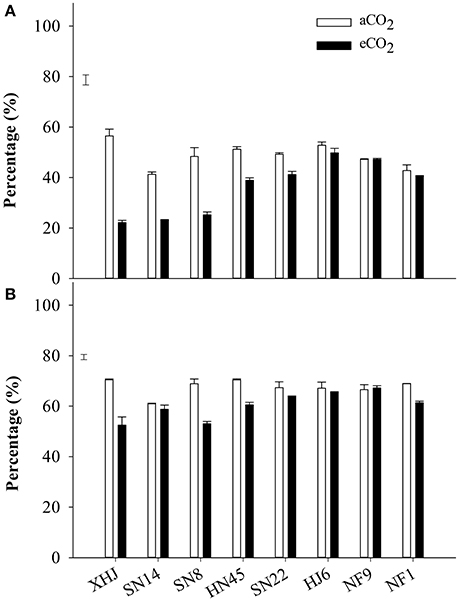
Figure 3. The percentage of (A) remobilized N in seed and (B) the percentage of N remobilized from vegetative organs to seeds of eight cultivars grown under aCO2 or eCO2. The error bars represent standard error, and the separate vertical bar in each panel indicates the LSD (P < 0.05) for the CO2 × cultivar interaction.
Approximately 68% of N was remobilized from vegetative organs to seeds at aCO2 in comparison to 60% under eCO2 (Figure 3B). Elevated CO2 significantly (P < 0.05) decreased the proportion of the N remobilization in XHJ, NF1, SN8, and HF45, but did not affect it in HJ6, NF9, SN14, and SN22, contributing to a significant CO2× cultivar interaction.
Relationship between Yield and N
The stimulation of fixed-N was significantly correlated with seed N increase (Figure 4A) and yield gain (Figure 4B), while the decrease of remobilized N to seed significantly correlated with the response of seed N to eCO2 (Figure 5A) and yield (Figure 5B). No significant correlation (P > 0.05) was found between the increase in fertilizer- or soil-derived N content and the increase of yield in response to eCO2 (Figure S3).
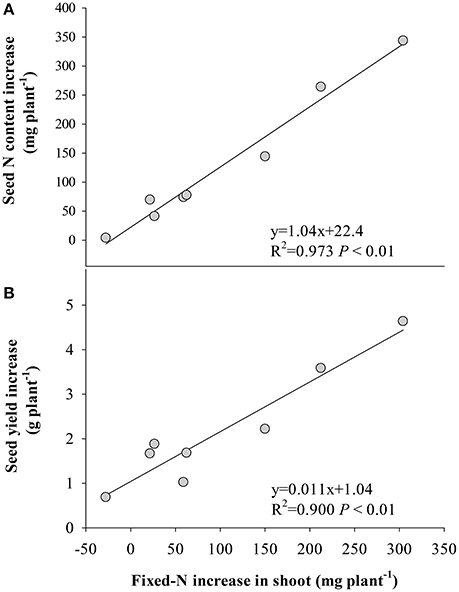
Figure 4. Relationships between the increase in the amount of fixed-N in shoot at R8 (120 days after sowing) and increases in (A) seed N and (B) seed yield of the eight soybean cultivars under eCO2 relative to aCO2. Each point represents one cultivar.
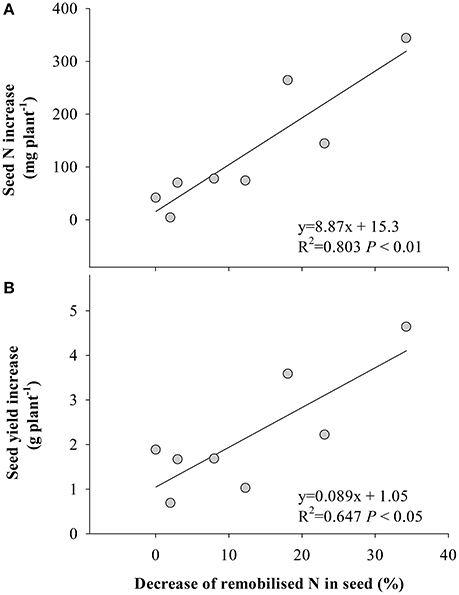
Figure 5. Relationships between the decrease of remobilized N in seed under eCO2 and (A) the seed N content increase, and (B) seed yield increase. Each point represents one soybean cultivar. The decrease of remobilized N was calculated as proportion of remobilized N in seed under aCO2 minus that under eCO2.
Root Morphology
Elevated CO2 increased total root length (P < 0.05) by an average of 19% (Table S1). The length of fine roots accounted for more than 85% of total root length, and fine roots (<0.5 mm) had a positive (P < 0.05) growth response to eCO2 in all cultivars except for SN22 (Table S1). Only the length of intermediate roots of XHJ, and the length of coarse roots of SN22 and NF1 were significantly higher under eCO2 than under aCO2 (P < 0.05).
Elevated CO2 significantly increased the N uptake per unit of root length in XHJ, SN14, HN45, SN22, and NF1 compared to aCO2 (P < 0.05), but did not in SN8, HJ6, and NF9 (Table S2). The fertilizer-derived N uptake per unit of root length did not significantly change in response to eCO2 except for NF1 (+15%) and NF9 (–12%) (P < 0.05). The soil-derived N uptake per unit of root increased by 26% (P < 0.05) across the cultivars under eCO2 compared to aCO2, with the maximum increase (44%) for XHJ and the minimum (9%) for SN8.
Although there were marked changes in root architecture in response to eCO2, these changes did not directly contribute to yield gain under eCO2. There was no correlation between seed yield increase with changes in total root length, fine, intermediate or coarse root length (P > 0.05, Figure S4).
Nodulation
Elevated CO2 significantly altered the nodule characteristics of soybean. Nodule numbers increased from 79 under aCO2 to 113 under eCO2 on average across cultivars (Table 2). Nodule number in response to eCO2 differed among soybean cultivars, with 96% of increase in HJ6 in comparison to only 3% in SN14. A significant (P < 0.001). A significant CO2× cultivars interaction was observed (P < 0.001; Table 2). Elevated CO2 resulted in a significant increase in nodule fresh weight (Table 2). The maximum increase (301%) was found in SN14 while the minimum increase was 93% in SN22. Elevated CO2 significantly increased nodule density of all cultivars but NF9 and SN14 (Table 2).
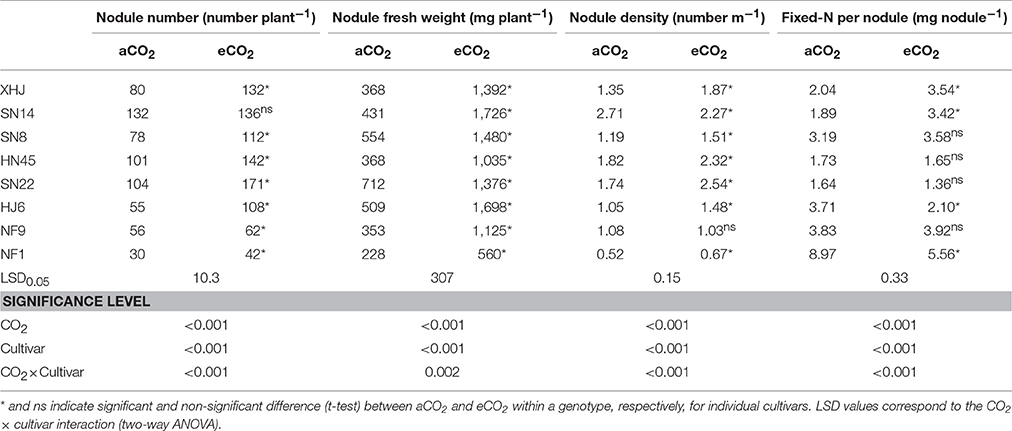
Table 2. Nodule number per plant, nodule fresh weight per plant, nodule density, and single nodule N fixation of eight soybean cultivars grown for 120 days (R8) under aCO2 or eCO2.
The amount of N fixed per nodule showed different responses to eCO2 among cultivars (Table 2), with 81 and 74% of increase in SN14 and XHJ in comparison to 43 and 38% of reduction in HJ6 and NF1, respectively, resulting in a significant CO2× cultivars interaction (P < 0.001).
Irrespective of cultivars, the increase in symbiotically fixed-N content in shoot correlated positively with the increase of fixed-N per nodule in response to eCO2 (P < 0.01; Figure 6), but did not correlate with nodule number, fresh weight, and density changes (P > 0.05; Figure S4).
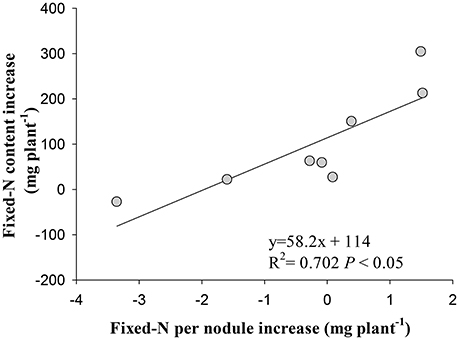
Figure 6. Relationship between the increase in fixed-N per nodule and the increase in fixed-N content in shoot of eight soybean cultivars at R8 (120 days after sowing) under eCO2 relative to aCO2. Each point represents one cultivar.
Discussion
This study demonstrated that eCO2 enhanced total N uptake in soybean, especially during the late reproductive stages. It was evident that the increase in the N content in shoots under eCO2 was greater at R8 than at R5 (Table 1). Moreover, irrespective of cultivars, the extent of the increase in N content derived from symbiotically fixed-N was greater than either fertilizer-derived N or soil-derived N during the period from R5 to R8 (Table 1). The fixed-N was the dominant source of plant N, but the proportion of fixed-N was greater under eCO2 than under aCO2 (Figure 2). The results are consistent with those of previous studies showing that eCO2 increased total N uptake in agricultural crops (Kimball et al., 2002; Leakey et al., 2009; Jin et al., 2012; Lam et al., 2012; Butterly et al., 2016).
Symbiotic N2 fixation during this reproductive period is critical for yield gain under eCO2. This was supported by the positive correlation (P < 0.05) between the amount of symbiotically fixed-N and seed yield (Figure 4), and the fixed-N being the major source of seed N (Figure 1). Furthermore, eCO2 decreased the proportion of remobilized N in seed (Figure 3), indicating that the eCO2-enhanced total N uptake during the late reproductive stage can largely satisfy N demand in seed development. Since the major source of N remobilization in soybean plants is from leaves (Schiltz et al., 2005; Li et al., 2016), the lesser amount of N removed from vegetative organs including leaves in response to eCO2 (Figure 3) was likely to maintain leaf photosynthetic capacity. Makino and Osmond (1991) also showed that leaf N correlated highly with the photosynthetic function of the leaf. Thus, the maintenance of adequate N in vegetative organs is likely to contribute to the increased biomass accumulation and seed yield under eCO2 (Figure 5).
The stimulation of N2 fixation during R5 to R8 under eCO2 was attributed to the increase in nodule N2 fixation efficiency, as evidenced by the positive correlation between the increase of fixed-N per nodule with the increase in fixed-N content in shoot under eCO2 (Figure 6). In previous studies, eCO2 enhanced N2 fixation through increasing specific nitrogenase activity (Saeki et al., 2008). The reason for the increased N2 fixation is that the enhanced photosynthesis under eCO2 (Ziska, 2008; Bishop et al., 2015) provides sufficient C sources for maintaining nodule function and N2 fixation (Li et al., 2016), resulting in the increase in shoot and root biomass (Figure S1). Another reason would be a change of rhizobium community in the rhizosphere of soybean under eCO2 (Yu et al., 2016), which might favor N2 fixation efficiency of nodules. This interaction between functional rhizobia and photosynthetic C supply under eCO2 warrants specific investigation.
A number of studies reported that eCO2 increased nodule number and biomass in chickpea, field pea (Jin et al., 2012), and common bean (Miyagi et al., 2007; Rogers et al., 2009). In the current study, a similar trend was observed for soybean, but neither the increase of nodule number nor biomass correlated with the increase of fixed-N content (Figure S4). This implies that the increase of fixed-N under eCO2 cannot be predominantly attributed to the number of nodules.
Elevated CO2 also changed root morphology with an increase in the proliferation of fine roots, which is likely to enhance plant nutrient absorption (Bentley et al., 2013; Beidler et al., 2015). Fine roots play a key role in N acquisition rather than root biomass (Matamala et al., 2003). In this study, the length of fine roots (<0.5 mm) significantly increased under eCO2 (Table S1), which helped to increase the uptake of soil and fertilizer N (Table 1). This is consistent with previous studies (Mikan et al., 2000; Zak et al., 2000; de Graaff et al., 2006; Beidler et al., 2015). Rogers et al. (1992) suggested that the greater proliferation of roots grown under eCO2 was a strategy to permit adequate nutrient acquisition under sub-optimal water supply. However, compared to fixed-N, the soil-, and fertilizer-derived N in the plant showed much less response to eCO2. The increase in fine root growth had no significant correlation with seed yield increase in response to eCO2 across genotypes (Figure S3), indicating that the contribution of root N uptake to yield gain is minimal under eCO2. In agreement with our observations, Butterly et al. (2015) also found that N fertilizer did not affect plant N concentration, and the proportion of fertilizer-derived N in field pea decreased under eCO2.
Nevertheless, eCO2 increased the uptake of soil N per unit of root length (Table S2). The enhancement of microbial activity and N mineralization in soil under eCO2 might be the main reason. The growth of fine roots leads to more rhizodeposition, which provides labile C for microorganisms to mineralize more soil organic N (Fischer and Kuzyakov, 2010; Fischer et al., 2010).
The capacity for total N uptake in response to eCO2 varied among soybean cultivars, XHJ had the greatest increase in N2 fixation under eCO2 (Figure 2), which supplied a large amount of N to seed during the reproductive stage (Figure 1B), and reduced the demand for N remobilization (Figure 3). In contrast, NF1 did not exhibit any increase in fixed-N during R5 to R8, and had the least increase in yield under eCO2 (Figure 1). The largest N2 fixation in XHJ would contribute to a high N2 fixation efficiency, since the amount of fixed-N per nodule was greatest in this cultivar (Table 2). As the dominant rhizobial strains in nodules greatly affected N2-fixing efficiency (Saeki et al., 2008) and soil microbial communities in the rhizosphere in response to eCO2 are dependent on soybean cultivars (Yu et al., 2016), the specific interaction between cultivar and rhizobial genera under eCO2 may influence soybean adaptability to eCO2. Therefore, the cultivar-specific rhizobia community in nodules may predominantly regulate the N2-fixing function in response to eCO2. This hypothesis deserves further research.
In summary, Figure 7 shows a conceptual diagram illustrating how eCO2 affects N uptake, and consequent yield gain in soybean. Elevated CO2 increased the plants' ability for N uptake. The N2 fixation during R5 to R8 became a major contributor to the increased N uptake and hence yield gain under eCO2. The enhanced N2 fixation under eCO2 might also lead to the decrease in remobilization of N from vegetative organs, increasing photosynthetic capacity and yield formation. Although eCO2 facilitated root proliferation and nodule growth, these variables were not correlated with yield gains. Cultivars with a greater N2-fixing efficiency during the late reproductive phase may exhibit a better adaptability to eCO2. The specific interaction between cultivar and rhizobia in the rhizosphere of soybean would be the key to this adaptability, and is worth further investigation.
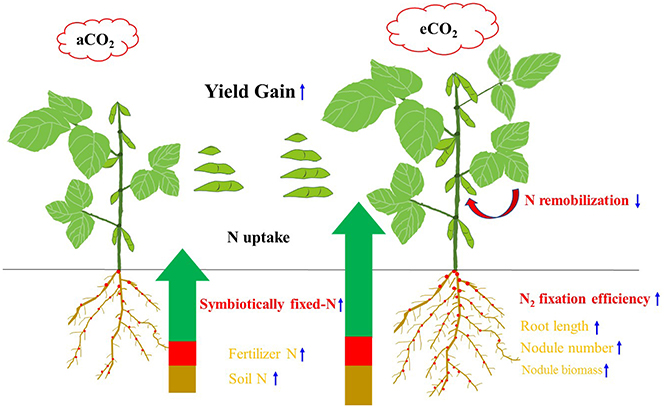
Figure 7. Diagram illustrating the N origins, root morphology, N remobilization, and yield gain of soybean in response to eCO2. The measurements that were significantly correlated with yield gain (P < 0.05) are indicated in red-bold, while the measurements responding to eCO2 but not correlated with yield gain are shown in orange. Upward and downward arrows indicate increase and decrease under the eCO2 condition, respectively.
Author Contributions
JJ and YL designed the experiments and managed the projects. YL, ZY, JL, SZ, and JW performed experiments. YL, JJ, UM, GW, and CT performed data analysis. JJ, UM, YL, XL, and CT wrote the manuscript.
Conflict of Interest Statement
The authors declare that the research was conducted in the absence of any commercial or financial relationships that could be construed as a potential conflict of interest.
Acknowledgments
The project was funded by the National Natural Science Foundation of China (31501259), Heilongjiang Provincial Funds for Distinguished Young Scientists (JC201413), Hundred Talents Program of Chinese Academy of Sciences, the Natural Science Foundation of Heilongjiang Province (QC2015045), and the Foundation of Key Laboratory for Agricultural Environment, Ministry of Agriculture of China.
Supplementary Material
The Supplementary Material for this article can be found online at: http://journal.frontiersin.org/article/10.3389/fpls.2017.01546/full#supplementary-material
References
Ainsworth, E. A., and Long, S. P. (2005). What have we learned from 15 years of free-air CO2 enrichment (FACE)? A meta-analytic review of the responses of photosynthesis, canopy. New Phytol. 165, 351–371. doi: 10.1111/j.1469-8137.2004.01224.x
Ainsworth, E. A., Davey, P. A., Bernacchi, C. J., Dermody, O. C., Heaton, E. A., Moore, D. J., et al. (2002). A meta-analysis of elevated CO2 effects on soybean (Glycine max) physiology, growth and yield. Global Change Biol. 8, 695–709. doi: 10.1046/j.1365-2486.2002.00498.x
Ainsworth, E. A., Rogers, A., Blum, H., Nosberger, J., and Long, S. P. (2003). Variation in acclimation of photosynthesis in Trifolium repens after eight years of exposure to Free Air CO2 Enrichment (FACE). J. Exp. Bot. 54, 2769–2774. doi: 10.1093/jxb/erg309
Arp, W. J. (1991). Effects of source-sink relations on photosynthetic acclimation to elevated CO2. Plant Cell Environ. 14, 869–875. doi: 10.1111/j.1365-3040.1991.tb01450.x
Beidler, K. V., Taylor, B. N., Strand, A. E., Cooper, E. R., Schonholz, M., and Pritchard, S. G. (2015). Changes in root architecture under elevated concentrations of CO2 and nitrogen reflect alternate soil exploration strategies. New Phytol. 205, 1153–1163. doi: 10.1111/nph.13123
Bentley, L. P., Stegen, J. C., Savage, V. M., Smith, D. D., von Allmen, E. I., Sperry, J. S., et al. (2013). An empirical assessment of tree branching networks and implications for plant allometric scaling models. Ecol. Lett. 16, 1069–1078. doi: 10.1111/ele.12127
Bertrand, A., Prevost, D., Bigras, F. J., Lalande, R., Tremblay, G. F., Castonguay, Y., et al. (2007). Alfalfa response to elevated atmospheric CO2 varies with the symbiotic rhizobial strain. Plant Soil 301, 173–187. doi: 10.1007/s11104-007-9436-9
Bishop, K. A., Betzelberger, A. M., Long, S. P., and Ainsworth, E. A. (2015). Is there potential to adapt soybean (Glycine max Merr.) to future CO2? An analysis of the yield response of 18 genotypes in free-air CO2 enrichment. Plant Cell Environ. 38, 1765–1774. doi: 10.1111/pce.12443
Butterly, C. R., Armstrong, R., Chen, D., and Tang, C. (2015). Carbon and nitrogen partitioning of wheat and field pea grown with two nitrogen levels under elevated CO2. Plant Soil 391, 367–382. doi: 10.1007/s11104-015-2441-5
Butterly, C. R., Armstrong, R., Chen, D., and Tang, C. (2016). Free-air CO2 enrichment (FACE) reduces the inhibitory effect of soil nitrate on N2 fixation of Pisum sativum. Ann. Bot. 117, 177–185. doi: 10.1093/aob/mcv140
Carranca, C., de Varennes, A., and Rolston, D. (1999). Biological nitrogen fixation by fababean, pea and chickpea, under field conditions, estimated by the 15N isotope dilution technique. Eur. J. Agron. 10, 49–56. doi: 10.1016/S1161-0301(98)00049-5
Chaturvedi, A., Bahuguna, R. N., Pal, M., Shah, D., Maurya, S., and Jagadish, K. S. V. (2017). Elevated CO2 and heat stress interactions affect grain yield, quality and mineral nutrient composition in rice under field conditions. Field Crops Res. 206, 149–157. doi: 10.1016/j.fcr.2017.02.018
Costa, C., Dwyer, L. M., Zhou, X. M., Dutilleul, P., Hamel, C., Reid, L. M., et al. (2002). Root morphology of contrasting maize genotypes. Agron. J. 94, 96–101. doi: 10.2134/agronj2002.0096
de Graaff, M. A., van Groenigen, K. J., Six, J., Hungate, B., and van Kessel, C. (2006). Interactions between plant growth and soil nutrient cycling under elevated CO2: a meta-analysis. Global Change Biol. 12, 2077–2091. doi: 10.1111/j.1365-2486.2006.01240.x
Egli, D. B., Leggett, J. E., and Duncan, W. G. (1978). Influence of N-stress on leaf senescence and N redistribution in soybeans. Agron. J. 70, 43–47. doi: 10.2134/agronj1978.00021962007000010011x
Fehr, W. R., Caviness, C. E., Burmood, D. T., and Pennington, J. S. (1971). Stage of development descriptions for soybeans, Glycine Max (L.) Merrill. Crop Sci. 11, 929–931. doi: 10.2135/cropsci1971.0011183X001100060051x
Fischer, H., and Kuzyakov, Y. (2010). Sorption, microbial uptake and decomposition of acetate in soil: transformations revealed by position-specific 14C labeling. Soil Biol. Biochem. 42, 186–192. doi: 10.1016/j.soilbio.2009.10.015
Fischer, H., Eckhardt, K. U., Meyer, A., Neumann, G., Leinweber, P., Fischer, K., et al. (2010). Rhizodeposition of maize: short-term carbon budget and composition. J. Plant Nutr. Soil Sci. 173, 67–79. doi: 10.1002/jpln.200800293
Jin, J., Tang, C. X., Armstrong, R., and Sale, P. (2012). Phosphorus supply enhances the response of legumes to elevated CO2 (FACE) in a phosphorus-deficient vertisol. Plant Soil 358, 86–99. doi: 10.1007/s11104-012-1270-z
Kimball, B. A., Kobayashi, K., and Bindi, M. (2002). Responses of agricultural crops to free-air CO2 enrichment. Adv. Agron. 77, 293–368. doi: 10.1016/S0065-2113(02)77017-X
Kinugasa, T., Sato, T., Oikawa, S., and Hirose, T. (2012). Demand and supply of N in seed production of soybean (Glycine max) at different N fertilization levels after flowering. J. Plant Res. 125, 275–281. doi: 10.1007/s10265-011-0439-5
Lam, S. K., Hao, X. Y., Lin, E. D., Han, X., Norton, R., Mosier, A. R., et al. (2012). Effect of elevated carbon dioxide on growth and nitrogen fixation of two soybean cultivars in northern China. Biol. Fert. Soils 48, 603–606. doi: 10.1007/s00374-011-0648-z
Langley, J. A., and Megonigal, J. P. (2010). Ecosystem response to elevated CO2 levels limited by nitrogen-induced plant species shift. Nature 466, 96–99. doi: 10.1038/nature09176
Leakey, A. D. B., Ainsworth, E. A., Bernacchi, C. J., Rogers, A., Long, S. P., and Ort, D. R. (2009). Elevated CO2 effects on plant carbon, nitrogen, and water relations: six important lessons from FACE. J. Exp. Bot. 60, 2859–2876. doi: 10.1093/jxb/erp096
Lee, T. D., Tjoelker, M. G., Reich, P. B., and Russelle, M. P. (2003). Contrasting growth response of an N2 fixing and non fixing forb to elevated CO2: dependence on soil N supply. Plant Soil 255, 475–486. doi: 10.1023/A:1026072130269
Li, Y. S., Liu, X. B., Wang, G. H., Yu, Z. H., Mathesius, U., Liu, J. D., et al. (2016). Shift in origin of plant nitrogen alters carbon and nitrogen assimilation during reproductive stages of soybean grown in a Mollisol. Crop Pasture Sci. 67, 872–880. doi: 10.1071/CP15184
Liu, N., Tian, Q., and Zhang, W. H. (2016). Artemisia frigida and Stipa krylovii, two dominant species in Inner Mongolia steppe, differed in their responses to elevated atmospheric CO2 concentration. Plant Soil 409, 117–129. doi: 10.1007/s11104-016-2952-8
Liu, X. B., and Herbert, S. J. (2002). Fifteen years of research examining cultivation of continuous soybean in northeast China: a review. Field Crops Res. 79, 1–7. doi: 10.1016/S0378-4290(02)00042-4
Luo, Y. Q., Hui, D. F., and Zhang, D. Q. (2006). Elevated CO2 stimulates net accumulations of carbon and nitrogen in land ecosystems: a meta-analysis. Ecology 87, 53–63. doi: 10.1890/04-1724
Makino, A., and Osmond, B. (1991). Effects of nitrogen nutrition on nitrogen partitioning between chloroplasts and mitochondria in pea and wheat. Plant Physiol. 96, 355–362. doi: 10.1104/pp.96.2.355
Mariotti, A., Lancelot, C., and Billen, G. (1984). Natural isotopic composition of nitrogen as a tracer of origin for suspended organic-matter in the scheldt estuary. Geochim. Cosmochim. Acta 48, 549–555. doi: 10.1016/0016-7037(84)90283-7
Martínez-Alcántara, B., Quiñones, A., Legaz, F., and Primo-Millo, E. (2012). Nitrogen-use efficiency of young citrus trees as influenced by the timing of fertilizer application. J. Plant Nutr. Soil Sci. 175, 282–292. doi: 10.1002/jpln.201100223
Matamala, R., and Schlesinger, W. H. (2000). Effects of elevated atmospheric CO2 on fine root production and activity in an intact temperate forest ecosystem. Global Change Biol. 6, 967–979. doi: 10.1046/j.1365-2486.2000.00374.x
Matamala, R., Gonzalez-Meler, M. A., Jastrow, J. D., Norby, R. J., and Schlesinger, W. H. (2003). Impacts of fine root turnover on forest NPP and soil C sequestration potential. Science 302, 1385–1387. doi: 10.1126/science.1089543
Mikan, C. J., Zak, D. R., Kubiske, M. E., and Pregitzer, K. S. (2000). Combined effects of atmospheric CO2 and N availability on the belowground carbon and nitrogen dynamics of aspen mesocosms. Oecologia 124, 432–445. doi: 10.1007/PL00008869
Miyagi, K. M., Kinugasa, T., Hikosaka, K., and Hirose, T. (2007). Elevated CO2 concentration, nitrogen use, and seed production in annual plants. Global Change Biol. 13, 2161–2170. doi: 10.1111/j.1365-2486.2007.01429.x
Peng, S. B., Huang, J. L., Sheehy, J. E., Laza, R. C., Visperas, R. M., Zhong, X. H., et al. (2004). Rice yields decline with higher night temperature from global warming. Proc. Natl. Acad. Sci. U.S.A. 101, 9971–9975. doi: 10.1073/pnas.0403720101
Rennie, R. J., and Dubetz, S. (1986). N-15-determined nitrogen-fixation in field-grown chickpea, lentil, fababean, and field pea. Agron. J. 78, 654–660. doi: 10.2134/agronj1986.00021962007800040020x
Rogers, A., Ainsworth, E. A., and Leakey, A. D. B. (2009). Will elevated carbon dioxide concentration amplify the benefits of nitrogen fixation in legumes? Plant Physiol. 151, 1009–1016. doi: 10.1104/pp.109.144113
Rogers, A., Gibon, Y., Stitt, M., Morgan, P. B., Bernacchi, C. J., Ort, D. R., et al. (2006). Increased C availability at elevated carbon dioxide concentration improves N assimilation in a legume. Plant Cell Environ. 29, 1651–1658. doi: 10.1111/j.1365-3040.2006.01549.x
Rogers, H. H., Peterson, C. M., McCrimmon, J. N., and Cure, J. D. (1992). Response of plant-roots to elevated atmospheric carbon-dioxide. Plant Cell Environ. 15, 749–752. doi: 10.1111/j.1365-3040.1992.tb01018.x
Saeki, Y., Minami, M., Yamamoto, A., and Akao, S. (2008). Estimation of the bacterial community diversity of soybean-nodulating bradyrhizobia isolated from Rj-genotype soybeans. Soil Sci. Plant Nutr. 54, 718–724. doi: 10.1111/j.1747-0765.2008.00300.x
Salon, C., Munier-Jolain, N. G., Duc, G., Voisin, A. S., Grandgirard, D., Larmure, A., et al. (2001). Grain legume seed filling in relation to nitrogen acquisition: a review and prospects with particular reference to pea. Agronomie 21, 539–552. doi: 10.1051/agro:2001143
Sardans, J., and Peñuelas, J. (2012). The role of plants in the effects of global change on nutrient availability and stoichiometry in the plant-soil system. Plant Physiol. 160, 1741–1761. doi: 10.1104/pp.112.208785
Schiltz, S., Munier-Jolain, N., Jeudy, C., Burstin, J., and Salon, C. (2005). Dynamics of exogenous nitrogen partitioning and nitrogen remobilization from vegetative organs in pea revealed by 15N in vivo labeling throughout seed filling. Plant Physiol. 137, 1463–1473. doi: 10.1104/pp.104.056713
Schneider, M. K., Luscher, A., Richter, M., Aeschlimann, U., Hartwig, U. A., Blum, H., et al. (2004). Ten years of free-air CO2 enrichment altered the mobilization of N from soil in Lolium perenne L. swards. Global Change Biol. 10, 1377–1388. doi: 10.1111/j.1365-2486.2004.00803.x
Shimono, H., and Bunce, J. A. (2009). Acclimation of nitrogen uptake capacity of rice to elevated atmospheric CO2 concentration. Ann. Bot. 103, 87–94. doi: 10.1093/aob/mcn209
Unkovich, M., and Baldock, J. (2008). Measurement of symbiotic N2 fixation in Australian agriculture. Soil Biol. Biochem. 40, 2915–2921. doi: 10.1016/j.soilbio.2008.08.021
Warembourg, F. R., and Fernandez, M. P. (1985). Distribution and remobilization of symbiotically fixed nitrogen in soybean (Glycine max). Physiol. Plant. 65, 281–286. doi: 10.1111/j.1399-3054.1985.tb02396.x
Yu, Z. H., Li, Y. S., Wang, G. H., Liu, J. J., Liu, J. D., Liu, X. B., et al. (2016). Effectiveness of elevated CO2 mediating bacterial communities in the soybean rhizosphere depends on genotypes. Agr. Ecosyst. Environ. 231, 229–232. doi: 10.1016/j.agee.2016.06.043
Zak, D. R., Pregitzer, K. S., Curtis, P. S., and Holmes, W. E. (2000). Atmospheric CO2 and the composition and function of soil microbial communities. Ecol. Appl. 10, 47–59. doi: 10.1890/1051-0761(2000)010[0047:ACATCA]2.0.CO;2
Zanetti, S., Hartwig, U. A., Luscher, A., Hebeisen, T., Frehner, M., Fischer, B. U., et al. (1996). Stimulation of symbiotic N2 fixation in Trifolium repens L. under elevated atmospheric pCO2 in a grassland ecosystem. Plant Physiol. 112, 575–583. doi: 10.1104/pp.112.2.575
Zanetti, S., Hartwig, U. A., vanKessel, C., Luscher, A., Hebeisen, T., Frehner, M., et al. (1997). Does nitrogen nutrition restrict the CO2 response of fertile grassland lacking legumes? Oecologia 112, 17–25.
Zhang, W. W., Wang, G. G., Liu, X. B., and Feng, Z. Z. (2014). Effects of elevated O3 exposure on seed yield, N concentration and photosynthesis of nine soybean cultivars (Glycine max (L.) Merr.) in Northeast China. Plant Sci. 226, 172–181. doi: 10.1016/j.plantsci.2014.04.020
Keywords: open-top chamber, 15N labeling, nodule density, symbiotic N2 fixation, N remobilization, Glycine max L.
Citation: Li Y, Yu Z, Liu X, Mathesius U, Wang G, Tang C, Wu J, Liu J, Zhang S and Jin J (2017) Elevated CO2 Increases Nitrogen Fixation at the Reproductive Phase Contributing to Various Yield Responses of Soybean Cultivars. Front. Plant Sci. 8:1546. doi: 10.3389/fpls.2017.01546
Received: 06 May 2017; Accepted: 23 August 2017;
Published: 14 September 2017.
Edited by:
José Manuel Mirás-Avalos, Centro de Edafología y Biología Aplicada del Segura (CSIC), SpainReviewed by:
Mauro Centritto, Trees and Timber Institute (CNR), ItalyFernando José Cebola Lidon, Faculdade de Ciências e Tecnologia da Universidade Nova de Lisboa, Portugal
Copyright © 2017 Li, Yu, Liu, Mathesius, Wang, Tang, Wu, Liu, Zhang and Jin. This is an open-access article distributed under the terms of the Creative Commons Attribution License (CC BY). The use, distribution or reproduction in other forums is permitted, provided the original author(s) or licensor are credited and that the original publication in this journal is cited, in accordance with accepted academic practice. No use, distribution or reproduction is permitted which does not comply with these terms.
*Correspondence: Jian Jin, ai5qaW5AbGF0cm9iZS5lZHUuYXU=