- 1Division of Integrative Bioscience and Biotechnology, College of Life Sciences, Sejong University, Seoul, South Korea
- 2Laboratory of Molecular Plant Pathology, College of Life Sciences and Biotechnology, Korea University, Seoul, South Korea
Microbial pathogens have evolved protein effectors to promote virulence and cause disease in host plants. Pathogen effectors delivered into plant cells suppress plant immune responses and modulate host metabolism to support the infection processes of pathogens. Reactive oxygen species (ROS) act as cellular signaling molecules to trigger plant immune responses, such as pathogen-associated molecular pattern (PAMP)-triggered immunity (PTI) and effector-triggered immunity. In this review, we discuss recent insights into the molecular functions of pathogen effectors that target multiple steps in the ROS signaling pathway in plants. The perception of PAMPs by pattern recognition receptors leads to the rapid and strong production of ROS through activation of NADPH oxidase Respiratory Burst Oxidase Homologs (RBOHs) as well as peroxidases. Specific pathogen effectors directly or indirectly interact with plant nucleotide-binding leucine-rich repeat receptors to induce ROS production and the hypersensitive response in plant cells. By contrast, virulent pathogens possess effectors capable of suppressing plant ROS bursts in different ways during infection. PAMP-triggered ROS bursts are suppressed by pathogen effectors that target mitogen-activated protein kinase cascades. Moreover, pathogen effectors target vesicle trafficking or metabolic priming, leading to the suppression of ROS production. Secreted pathogen effectors block the metabolic coenzyme NADP-malic enzyme, inhibiting the transfer of electrons to the NADPH oxidases (RBOHs) responsible for ROS generation. Collectively, pathogen effectors may have evolved to converge on a common host protein network to suppress the common plant immune system, including the ROS burst and cell death response in plants.
Introduction
Plants have evolved sophisticated defense mechanisms to resist potential attacks by microbial pathogens (Grant and Loake, 2000). The first line of defense is triggered in plants by the perception of microbe- or pathogen-associated molecular patterns (MAMPs or PAMPs) via membrane-bound pattern recognition receptors (PRRs), leading to basal immunity, known as PAMP-triggered immunity (PTI) (Gómez-Gómez and Boller, 2000; Jones and Dangl, 2006). PAMPs include conserved cell surface structures including bacterial flagellin, lipopolysaccharides, and peptidoglycan, or fungal cell wall components such as glucan or chitin (Zipfel et al., 2004; Torres, 2010). Plants may disrupt numerous non-host or host pathogen attacks via PTI; however, adapted pathogens can overcome the PTI-dependent defense response to cause disease on their host plants (Collins et al., 2003; He et al., 2006). PTI requires signal transduction from receptors to downstream components via mitogen-activated protein kinase (MAPK) cascade pathways (Pitzschke et al., 2009). Known PAMPs activate MAP kinases in plant cells. In the second line of defense, plants have acquired a cell-based surveillance system using intracellular nucleotide-binding leucine-rich repeat (NLR) receptors to recognize specific pathogen effectors, leading to resistance (R) gene-mediated effector-triggered immunity (ETI) (Jones and Dangl, 2006; Dangl et al., 2013). The two phases of plant immunity may be spatiotemporally distinct but are intimately related to the reactive oxygen species (ROS) burst (Grant and Loake, 2000; Torres et al., 2006; Kadota et al., 2015). Production of ROS in plant cells is a hallmark of successful recognition of plant pathogens and activation of plant defenses (Lamb and Dixon, 1997; Torres, 2010). Pathogen-induced apoplastic ROS production was first demonstrated in potato tuber tissues by Doke (1983) and indeed ROS play important roles in plant immune responses as signaling molecules (Torres, 2010; Mittler et al., 2011; O’Brien et al., 2012a; Frederickson Matika and Loake, 2014; Lehmann et al., 2015). In plant cells, ROS also occur in response to many physiological stimuli (Mori and Schroeder, 2004; Mittler et al., 2011; Baxter et al., 2014).
Reactive oxygen species are highly reactive reduced oxygen molecules, such as superoxide (⋅O2-), hydrogen peroxide (H2O2), and hydroxyl radical (⋅OH) (Grant and Loake, 2000; Mori and Schroeder, 2004; Sagi and Fluhr, 2006). There is comprehensive evidence that ROS can act as cellular signaling molecules, mediating various important responses of plant cells to different physiological stimuli, including pathogen attack, abiotic stress, hormone signaling, and polar growth (Mori and Schroeder, 2004; Torres and Dangl, 2005). ROS are formed intracellularly during certain redox reactions in the cell membranes, cytoplasm, nuclei, mitochondria, chloroplasts, peroxisomes, and endoplasmic reticulum (Ashtamker et al., 2007; Liu et al., 2007; Torres, 2010; La and López-Huertas, 2016). The endomembrane and nuclear compartments are likely targets or sources for ROS signaling (Ashtamker et al., 2007). Pathogen-induced ROS generation in chloroplasts is known to play a crucial role in the signaling for and/or execution of hypersensitive response (HR) cell death in plants (Liu et al., 2007). In addition, mitochondrial ROS associated with alteration in respiration are likely to activate defense responses, but could not be directly involved in plant cell death (Vidal et al., 2007). The generation of extracellular ROS, including H2O2, requires the extracellular activities of cell wall peroxidases and plasma membrane NADPH oxidases in plant cells (Bienert and Chaumont, 2014). NADPH oxidase-dependent ROS generation with electron supply provided by NADP-malic enzyme (ME) is presented in Figure 1. H2O2 is one of the most abundant and stable ROS in plants, and ROS generated inside cells can move apoplastically as H2O2 into neighboring cells (Allan and Fluhr, 1997). ROS signal transduction activates Ca2+ channels in the plasma membrane and may be a central step in many ROS-mediated processes regulating the physiology of plant cells (Mori and Schroeder, 2004). It has also been proposed that biomembrane channels (aquaporins) mediate H2O2 transport across biological membranes to control ROS signaling in plant cells (Bienert and Chaumont, 2014; Tian et al., 2016). The primary ROS bursts after perception of pathogens occur in the apoplasts; however, ROS produced in different compartments inside the plant cell may function in plant defenses to pathogen invasion (Torres, 2010).
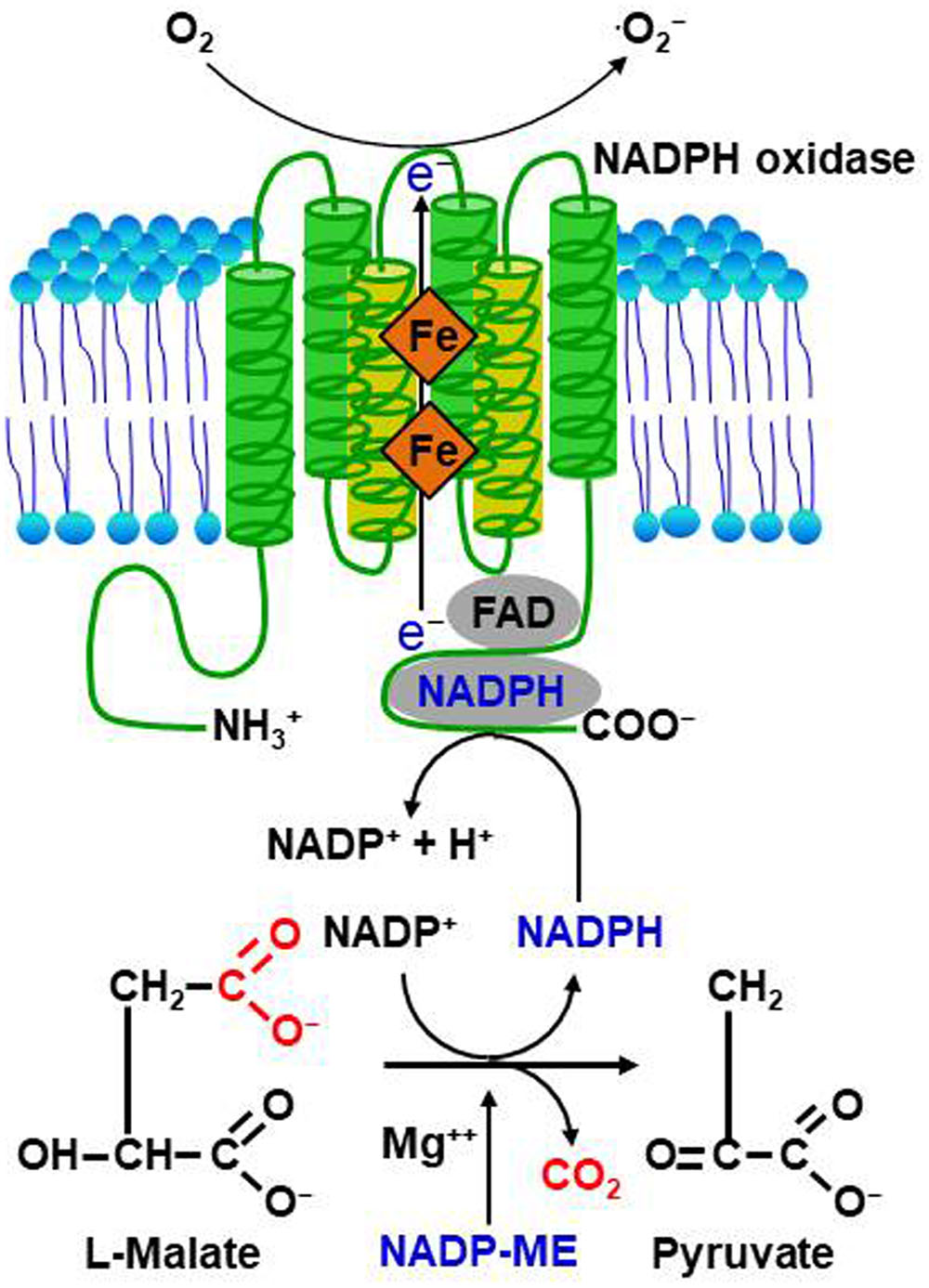
FIGURE 1. Proposed model for NADPH oxidase-dependent ROS generation via NADP-malic enzyme (ME)-mediated electron supply. NADP-malic enzyme (ME) serves as a source of NADPH and pyruvate in the cytosol of various plant tissues. It catalyzes the oxidative decarboxylation of L-malate to yield pyruvate, CO2, and NADPH in the presence of a bivalent cation, such as Mg++. NADPH oxidase, known as the Respiratory Burst Oxidase Homolog (RBOH), catalyzes the generation of superoxide (⋅O2-) by the one-electron reduction of molecular oxygen using NADPH as an electron donor. Superoxide can spontaneously form hydrogen peroxide (H2O2) that will undergo further reactions to generate reactive oxygen species (ROS).
Reactive oxygen species are an effective weapon that can be produced rapidly and utilized against pathogen infection (Levine et al., 1994). H2O2 and O2- are mainly produced at the site of attempted pathogen invasion in plant cells (Apostol et al., 1989). They are secreted from the cell within 3 min of recognition of MAMPs or PAMPs (Chinchilla et al., 2007; Nühse et al., 2007; Hedrich, 2012). The function of H2O2 in inhibiting pathogen growth is fully understood (Chi et al., 2009; Huang et al., 2011; Park et al., 2013). Plant-derived ROS act as a powerful weapon against pathogen invasion. By contrast, pathogens have evolved strategies to reduce plant ROS bursts in different ways during infection. For example, pathogens may avoid the risk of the ROS burst by using effectors. However, in the presence of effector-aware and defensible plants, pathogens are often faced with mutation pressure for virulence (Jones and Dangl, 2006). The competition between pathogens and host plants, called ‘arms races’ (Boller and He, 2009), may lead to the creation of numerous types of effectors in microbial pathogens and also R- or defense-related proteins in plants. Effectors from these evolutionarily diverse pathogens are predicted to converge on common host plant proteins, which are characterized by a high degree of interaction in host plant protein networks (Weßling et al., 2014; Rovenich et al., 2016). Here, we review and discuss recent advances in understanding how microbial pathogen effectors have evolved toward the suppression of plant apoplastic ROS bursts during infection.
Pamp-Mediated ROS Burst that Triggers Basal Immune Responses
Perception of PAMPs by plants via PRRs triggers ROS production through activation of NADPH oxidases as well as peroxidases, leading to PTI-dependent basal defenses that inhibit invading pathogens (Figure 2). The apoplastic ROS bursts generated in elicited plant cells are sufficiently cytotoxic to kill invading pathogens (Legendre et al., 1993; Chi et al., 2009; Park et al., 2013). ROS also act as signaling molecules, triggering plant immune and cell death responses (Tenhaken et al., 1995; Jabs, 1999; Torres, 2010). Thus, pathogens need to take steps to avoid exposure to toxic ROS. NADPH oxidases, also known as Respiratory Burst Oxidase Homologs (RBOHs), are responsible for production of ROS in plants during pathogen infection (Torres and Dangl, 2005; Torres, 2010). NADPH oxidase (RBOHD) phosphorylation by the PRR-associated kinase BIK1 has been proposed to be essential for PAMP-triggered ROS production (Kadota et al., 2014). In addition, the apoplastic peroxidase-dependent ROS burst plays an important role in Arabidopsis PTI mediated by the recognition of PAMPs (Daudi et al., 2012). Antisense expression of a heterologous French bean (Phaseolus vulgaris) peroxidase (FBP1) cDNA in Arabidopsis diminishes the expression of Arabidopsis peroxidases PRX33 and PRX34, blocking the ROS burst in response to a Fusarium oxysporum cell wall elicitor, and leading to enhanced susceptibility to fungal and bacterial pathogens (Bindschedler et al., 2006; Daudi et al., 2012; O’Brien et al., 2012b). Similarly, pepper extracellular peroxidase CaPO2 generates ROS bursts, activating local and systemic cell death and defense response to bacterial pathogens (Choi et al., 2007). Recently, the plant aquaporin AtPIP;4 has been demonstrated to trigger cytoplasmic import of apoplastic H2O2 into plant cells, activating systemic acquired resistance (SAR) and PTI pathways in response to Pseudomonas syringae pv. tomato DC3000 and two typical PAMPs (flagellin and chitin), respectively (Tian et al., 2016). This suggests a pivotal role for aquaporins in apocytoplastic ROS signal transduction in disease immunity pathways.
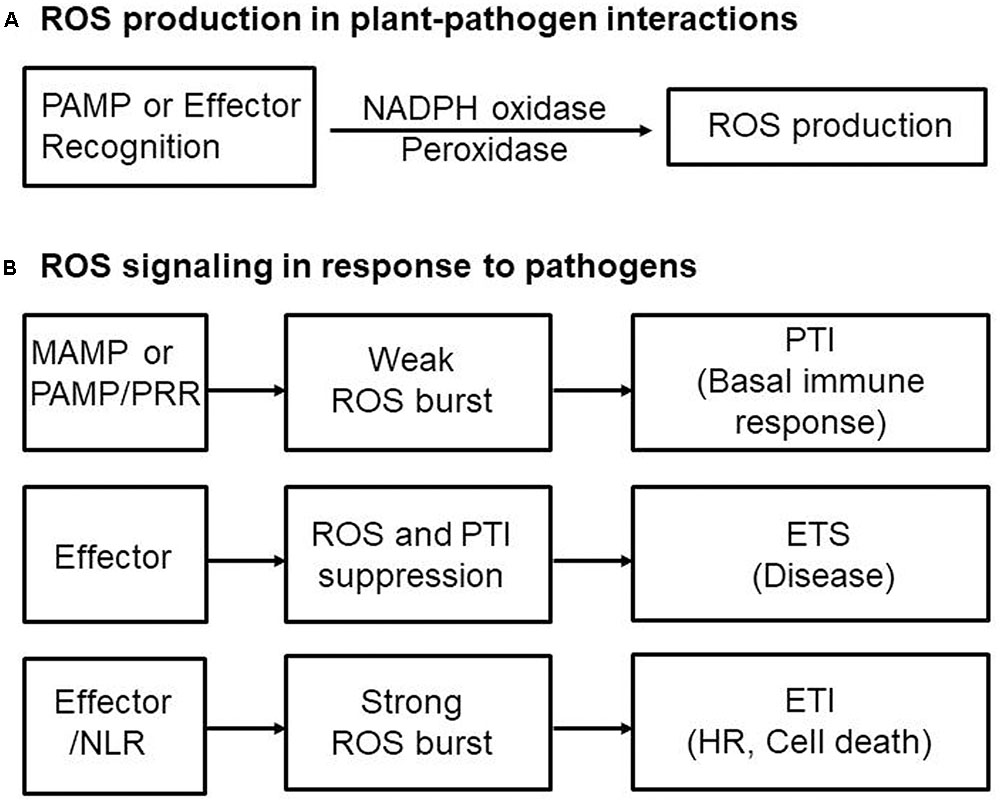
FIGURE 2. Reactive oxygen species (ROS) production and signaling in plant-pathogen interactions. (A) Perception of microbe- or pathogen-associated molecular patterns (MAMPs or PAMPs) or effectors by plants via membrane-bound pattern recognition receptors (PRRs) or intracellular nucleotide-binding leucine-rich repeat (NLR) receptors, respectively, activates NADPH oxidases as well as peroxidases, triggering ROS production and the ROS burst. (B) Perception of MAMPs or PAMPs by PRRs induces weak ROS bursts, leading to PAMP-triggered immunity (PTI)-dependent basal defense responses. Adapted pathogens secrete virulent effector proteins into plant cells to suppress the ROS burst and PTI, resulting in effector-triggered susceptibility (ETS) to cause disease in their respective host plants. Pathogen avirulence (Avr) effectors interact directly or indirectly with intracellular NLR proteins, leading to a strong ROS burst and HR cell death response, key components of effector-triggered immunity (ETI). ETI is an accelerated and amplified PTI response, resulting in disease resistance and, usually, a resistance (R) gene-mediated HR cell death response at the infection site in plants.
Plants defend themselves against invading pathogens through cell wall reinforcement. Cell wall fortifications are facilitated by an apoplastic H2O2 burst, cell wall cross-linking, and callose deposition at the site of infection (Bradley et al., 1992; Deepak et al., 2007; Luna et al., 2011; Ellinger et al., 2013). Effector (Elicitor)-induced oxidative cross-linking of plant cell wall structural proteins is essential for cell maturation and toughening of cell walls in the initial stages of plant defense (Bradley et al., 1992). Callose, a (1,3)-β-glucan, is a major component of cell wall thickening at sites of fungal penetration in plants (Ellinger et al., 2013). The Arabidopsis GTPase RabA4c physically interacts with its effector PMR4 to enhance PMR4-dependent callose biosynthesis, which ultimately results in complete penetration resistance to powdery mildew (Golovinomyces cichoracearum) (Ellinger et al., 2014). Hydroxyproline-rich glycoproteins (HRGPs) are involved in cell wall strengthening by formation of intra- and intermolecular cross-links (Deepak et al., 2007). Exogenous application of H2O2 rescues the callose deposition-deficient phenotype of peroxidase knockdown Arabidopsis lines treated with the bacterial flagellin, Flg22 (Daudi et al., 2012). This suggests that cell wall peroxidase-dependent H2O2 production is required for PAMP-triggered immune responses, such as callose deposition.
Effector-Mediated ROS Burst that Induces HR and Cell Death Responses
Reactive oxygen species bursts are monitored during infection by avirulent pathogens (Grant and Loake, 2000). Pathogen avirulence (Avr) effectors interact directly or indirectly with NLR proteins, leading to a strong ROS burst and the HR cell death response, both key components of ETI (Figure 2; Gabriel and Rolfe, 1990; McHale et al., 2006; van der Hoorn and Kamoun, 2008; Spoel and Dong, 2012; Cesari et al., 2014; Han and Hwang, 2017). However, whether NLR-mediated ROS themselves activate HR cell death and immune responses is not fully understood. Rice resistance to incompatible rice blast fungus (Magnaporthe oryzae) isolates is suppressed by inhibiting the accumulation of apoplastic ROS, even in the presence of the R gene (Singh et al., 2016). The resistant rice cultivar Hwayeonbyeo carrying Pib exhibits a compatible response to M. oryzae INA168 carrying AvrPii and AvrPib via the deletion of OsNADP-ME2. In the absence of normal production of ROS, cell death and immune responses are severely suppressed, although the Avr effector and cognate NLR proteins are not impaired (Singh et al., 2016). Increased ROS production during infection is essential for NLR-mediated cell death and immunity as well as disease-associated cell death (Greenberg and Yao, 2004; Choi et al., 2013). Perception of plant NLR receptors by specific pathogen effectors triggers a strong ROS burst through activation of RBOHs receiving an adequate supply of NADPH via the activity of NADP-ME (Figure 1; Singh et al., 2016).
Host cysteine proteases targeted by the Ustilago maydis effector Pit2 are likely to be crucial determinants in apoplastic maize immune responses such as the ROS burst (Mueller et al., 2013). The Cladosporium fulvum effector Avr2 binds and inhibits Rcr3, a extracellular tomato cysteine protease, which is required for Cf-2-dependent disease resistance (Rooney et al., 2005). It has been proposed that the Rcr3-Avr2 complex enables the Cf-2 protein to activate a HR, including the ROS burst and cell death (Table 1). The defense-related protease Rcr3 may act as a decoy for Avr2 perception in tomato plants carrying the Cf-2 resistance gene (Shabab et al., 2008).
The Xanthomonas campestris pv. vesicatoria effector AvrBsT induces a H2O2 burst and HR cell death in pepper (Capsicum annuum) (Kim et al., 2013). The AvrBsT-triggered HR cell death response is similar to the resistance (R) gene-mediated defense response in plants (Eitas and Dangl, 2010; Kim et al., 2010). AvrBsT physically binds to pepper arginine decarboxylase (CaADC1) (Kim et al., 2013), pepper aldehyde dehydrogenase (CaALDH1) (Kim and Hwang, 2015a), pepper heat shock protein 70a (CaHSP70a) (Kim and Hwang, 2015b), and pepper suppressor of the G2 allele of skp1 (CaSGT1) (Kim et al., 2014) in planta to promote the ROS burst, defense gene expression, cell death, and defense responses (Han and Hwang, 2017). AvrBsT and CaPIK1 directly bind to CaSGT1 in yeast and in planta. AvrBsT is subsequently phosphorylated by CaPIK1 and forms the active AvrBsT–SGT1–SGT1-PIK1 complex, which promotes the ROS burst, HR cell death, and defense responses in plants (Kim et al., 2014).
Effector-Mediated ROS Suppression that Causes Disease on Host Plants
Adapted microbial pathogens have evolved their effector proteins as virulence factors to suppress the ROS burst and PTI, causing disease on their respective host plants (Figure 2). Secreted effector proteins are delivered into host cells to protect pathogen cell walls against plant-derived hydrolytic enzymes and suppress PAMP-triggered host immunity, leading ultimately to the successful colonization of host plants (de Jonge et al., 2010; Mentlak et al., 2012; Lee et al., 2014; Sánchez-Vallet et al., 2014; Wawra et al., 2016). Various components of fungal cell walls such as glucans, chitin, and proteins, acting as PAMPs to trigger basal immune responses, are degraded by plant-derived hydrolytic enzymes, such as β-1,3-glucanases, chitinases, and serine and cysteine proteases (Jones and Dangl, 2006; van den Burg et al., 2006).
The C. fulvum effector Avr4, a chitin-binding lectin, binds to its own cell walls to protect chitin against hydrolysis by plant chitinases during infection of tomato, suggesting that Avr4 is a virulence factor (Table 1; van den Burg et al., 2006). The LysM domain-containing effector protein Ecp6 of C. fulvum mediates virulence through suppression of chitin-triggered immunity in plants (de Jonge et al., 2010). C. fulvum Ecp6 (CfEcp6) is secreted at high levels during plant infection and binds chitin, thereby blocking chitin-triggered immunity responses through sequestering chitin fragments and preventing their recognition by plant chitin receptors. By contrast to C. fulvum Ecp6, both Mg1LysM and Mg3LysM from the fungus Mycosphaerella graminicola protect fungal hyphae against plant-derived hydrolytic enzymes, such as chitinases (Table 1; Marshall et al., 2011). As a virulence determinant in the rice blast fungus M. oryzae, the secreted LysM effector (Slp1) binds to chitin inside the fungal cell wall, suppressing chitin-triggered plant immune responses, including the ROS burst and plant defense gene expression (Mentlak et al., 2012). The effector Slp1 inhibits the chitin-induced ROS burst in rice suspension cells. The lectin FGB1 (Fungal Glucan Binding 1), secreted from the root endophyte Piriformospora indica, specifically interacts with β-1, 6-linked glucan, altering cell wall composition and suppressing glucan-triggered ROS production in plants (Wawra et al., 2016). The presence of P. indica in the roots of barley inhibits laminarin-induced ROS production. Laminarin-triggered ROS production is also delayed when compared with that observed following chitin elicitation.
Some pathogen effectors specifically bind to plant proteases and may activate downstream signaling components (van der Hoorn and Jones, 2004). Apoplast-localized plant proteases can play an important role in defense responses to microbial pathogens. The U. maydis effector Pit2 physically interacts with and inhibits apoplastic maize cysteine proteases, suppressing host immunity (Table 1; Mueller et al., 2013). The secreted effector protein Pit2 is essential for maintenance of biotrophy and induction of tumors in maize. The biotrophic interaction of maize with U. maydis depends on inhibition of apoplastic cysteine proteases by the effector Pit2 with a conserved inhibitor domain. The U. maydis effector Pep1 (Protein essential during penetration-1) interferes with maize apoplastic peroxidases at the plant–pathogen interface to scavenge ROS (Table 1; Hemetsberger et al., 2012). As an inhibitor of plant peroxidases, Pep1 effectively inhibits the peroxidase-triggered ROS burst, which thereby suppresses the early immune response of maize leading to the establishment of a biotrophic interaction. Pep1 localizes to the plant apoplast, where it accumulates at sites of cell-to-cell passage of biotrophic U. maydis hyphae (Doehlemann et al., 2009). The obligate biotrophic fungal pathogen of barley, Blumeria graminis f. sp. hordei, secretes an extracellular catalase B to scavenge H2O2 at sites of fungal germ tube invasion during infection (Table 1; Zhang et al., 2004). A large number of catB transcripts accumulate during the mature primary germ tube and appressorium germ tube stages of fungal development on the susceptible barley plant, suggesting the upregulation of an extracellular catalase gene early during fungal invasion.
Effector-Mediated ROS Suppression that Targets MAPK Signaling Pathways
During pathogen infection, perception of PRRs by PAMPs leads to PTI by causing a rapid ROS burst via receptor-like cytoplasmic kinases (RLCKs) such as PBL1 and BIK1 (Feng et al., 2012; Shi et al., 2013; Ranf et al., 2014) or through MAPK cascades (Zhou et al., 2014). Pathogens overcome basal immune responses through inactivation of PAMP-induced signaling pathways that target MAPK cascade components (Pitzschke et al., 2009; Bi and Zhou, 2017). MAPKs are major targets for inactivation by pathogen effector proteins (Figure 3). MAPK cascades are highly conserved modules and are implicated in pathogen signaling during multiple defense responses against pathogen invasion in plants (Yang et al., 2001; Colcombet and Hirt, 2008; Tena et al., 2011; Singh et al., 2012, 2014, 2016). In particular, the MAPK cascade regulates transcriptional reprogramming via the WRKY transcription factor in the early signaling events following PAMP recognition in plants (Adachi et al., 2015). MAPK signaling is involved in the expression of genes required for apoplastic ROS production in plant defense responses. For example, WRKY transcription factors phosphorylated by MAPKs upregulate the RBOH, an NADPH oxidase, inducing pathogen-responsive ROS bursts in Nicotiana benthamiana (Adachi et al., 2015). However, because of the complicated nature of the downstream MAPK cascade, how MAPK signaling promotes ROS generation is not fully understood.
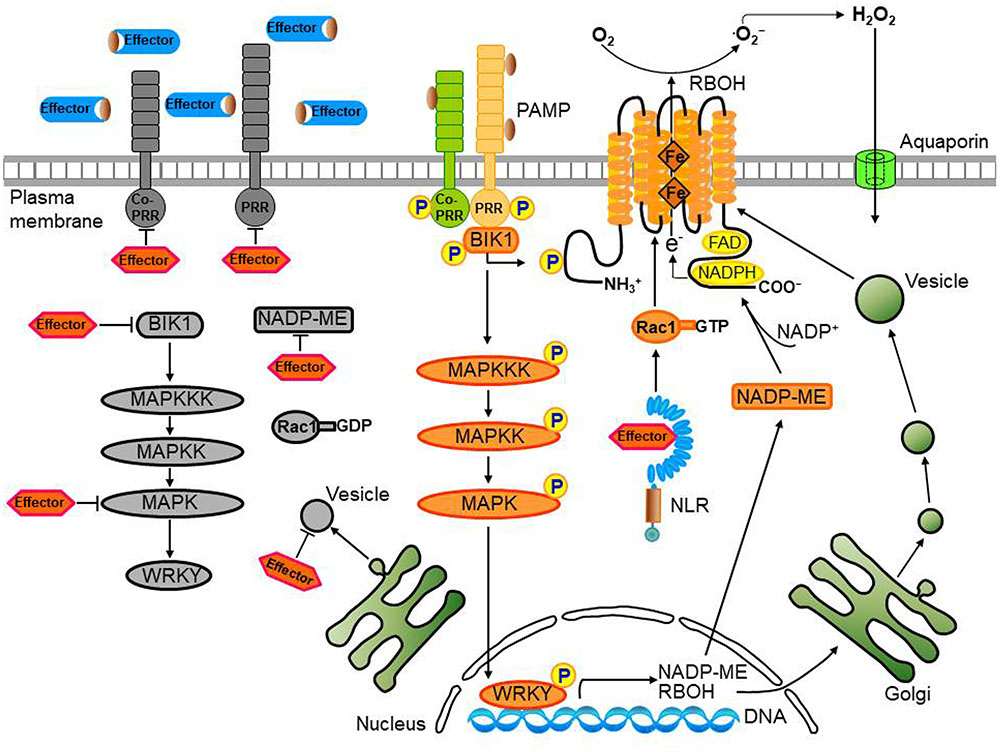
FIGURE 3. Convergent effector targeting of ROS signaling networks in plant cells. Plant pathogens secrete effectors into the apoplastic area and cytoplasm of plant cells. These apoplastic effectors interfere with PRR-mediated PAMP recognition, ultimately leading to the inactivation of plasma membrane-bound RBOHs. Cytoplasmic effectors target the kinase domains of PRRs, the receptor-like cytoplasmic kinase (RLCK) BIK1, MAPK cascades, and WRKY transcription factors, inhibiting the transcription of RBOHs and NADP-ME, both essential for robust ROS generation. A bacterial type III effector inhibits BIK1 downstream of the PRR (BAK1), enhancing virulence. Some cytoplasmic effectors target vesicle trafficking, suppressing the transport of ROS-producing RBOH enzymes to the plasma membrane during infection. Secreted pathogen effectors block the metabolic coenzyme NADP-ME, inhibiting the transfer of electrons to the NADPH oxidases (RBOHs) responsible for ROS generation. The biomembrane channels, aquaporins, mediate H2O2 transport across biological membranes. However, when specific pathogen effectors recognize intracellular NLR proteins, the effector no longer functions as a virulence factor; here, the effector-NLR complex leads to a strong apoplastic ROS burst and HR cell death response. The PRR-associated kinase BIK1 directly phosphorylates the NADPH oxidase RBOHD to enhance RBOHD-mediated ROS production. Rac1 directly interacts with both NLR and RBOH to activate RBOH. Apoplastic ROS are toxic to pathogens and also activate MAPK cascades and RBOH enzymes in their role as immune signal molecules.
Pathogen effectors act on plant host target proteins, interfering with PTI-mediated defense signaling cascades, such as ROS and MAPK cascades, ultimately causing disease in host cells (Jones and Dangl, 2006). For example, the P. syringae effector HopAO1 targets the Arabidopsis receptor kinase EF-TU RECEPTOR (EFR), reducing EFR phosphorylation, thereby preventing subsequent early immune responses, such as the ROS burst and MAPK activation (Table 1; Macho et al., 2014). The P. syringae effector HopF2 interacts directly with the plasma membrane-localized receptor-like kinase (RLK) BAK1 and suppresses early signaling events triggered by multiple PAMPs, including BIK1 phosphorylation, MAPK activation, and defense gene expression (Table 1; Wu et al., 2011; Zhou et al., 2014). In fact, BAK1 can directly phosphorylate the plasma membrane-localized RLCK BIK1 (Lu et al., 2010). FLAGELLIN SENSING2 (FLS2)/BAK1-induced MAPK signaling (Zhou et al., 2014) enhances gene expression for plant immune responses via recognition of specific target genes by WRKY transcription factors (Chi et al., 2013). Multiple WRKYs bind to and activate the NADPH oxidase RBOHB promoter, followed by enhanced RBOHB expression, which subsequently leads to the RBOHB-dependent ROS burst (Adachi et al., 2015). Type III effectors also target specific host plant proteins, such as major MAPK and WRKY modules (Feng and Zhou, 2012; Le Roux et al., 2015). The P. syringae effector HopAI1, for example, inactivates MAPKs by removing the phosphate group from phosphothreonine through a unique phosphothreonine lyase activity (Table 1; Zhang et al., 2007, 2012). Another type III effector, HopF2, interacts with Arabidopsis MAP Kinase Kinase 5 (MKK5), and likely other MAPKKs (MKK1, MKK3, MKK4, MKK6, and MKK10), suppressing MAPKs and PAMP-triggered defenses (Table 1; Wang et al., 2010). The Ralstonia solanacearum acetyltransferase effector PopP2 localizes to the plant cell nucleus and acetylates lysine residues of WRKY transcription factors, blocking DNA binding (Table 1; Deslandes et al., 2003;Le Roux et al., 2015). The X. campestris uridylyl transferase effector AvrAC targets and inhibits BIK1 downstream of BAK1, enhancing virulence (Table 1; Feng et al., 2012). In particular, AvrAC acts upstream of MAPK cascades and ROS production to suppress PTI signaling. The PRR-associated kinase BIK1 directly phosphorylates the NADPH oxidase RBOHD, enhancing RBOHD-mediated ROS production (Figure 3; Kadota et al., 2014; Li et al., 2014). The Phytophthora infestans RXLR effector PexRD2 interacts with a specific host MAPKKK, suppressing MAPKKK signaling-dependent cell death (King et al., 2014). In addition, multiple RXLR effectors from Hyaloperonospora arabidopsidis and P. infestans suppress the PAMP-elicited ROS burst (Fabro et al., 2011; Zheng et al., 2014). Functionally redundant effectors from different pathogen species may evolve virulence strategies to target PTI signal transduction processes, such as MAPK cascades, the ROS burst, and defense gene activation (Figure 3; Kvitko et al., 2009; Win et al., 2012; Zheng et al., 2014).
Effector-Mediated ROS Suppression that Targets Vesicle Trafficking and Metabolic Priming
Vesicle trafficking is an important cellular function in plants and is required for the transport of immune receptors and associated proteins, and for the extracellular secretion of immune-related molecules and antimicrobial compounds upon pathogen attack (Inada and Ueda, 2014; Macho and Zipfel, 2015). Visible vesicle-like bodies aggregate directly beneath sites of fungal attack in the barley-B. graminis f. sp. hordei pathosystem (An et al., 2006) and vesicle incidence is positively associated with levels of resistance to B. graminis f. sp. hordei penetration (Collins et al., 2003). Vesicles contain phytoalexins, phenolics, or ROS (Hückelhoven et al., 1999; Collins et al., 2003; An et al., 2006). The presence of ROS in the vesicles at penetration sites of B. graminis f. sp. hordei (Collins et al., 2003) suggests that the endomembrane-associated immune response is associated with ROS signaling. One constituent of the vesicles is H2O2, a plant defense compound involved in antimicrobial, cell wall cross-linking, and signaling functions (Lamb and Dixon, 1997; Collins et al., 2003).
Pathogen effectors target vesicle trafficking, suppressing PTI and potentially mediating immune-related ROS signaling (Figure 3; Macho and Zipfel, 2015). The Phytophthora cryptogea effector cryptogein induces an increase in abundance of the ROS-producing enzyme RBOHD, as well as ROS production at the plasma membrane of tobacco cells (Table 1; Noirot et al., 2014). Plant NADPH oxidases (RBOHDs) localize to the plasma membrane and endomembranes, and vesicle trafficking may contribute to the increase in RBOH abundance at the plasma membrane. The P. syringae pv. tomato virulence effector HopM1 interacts with and degrades an immunity-associated protein AtMIN7 via the host proteasome, suppressing PTI (Table 1; Nomura et al., 2006). AtMIN7 localizes to the trans-Golgi network/early endosome of plant cells and mediates immune-associated vesicle trafficking (Nomura et al., 2011). Some type III effector proteins from X. campestris pv. vesicatoria (Xcv) target plant protein secretion pathways, suppressing PTI (Macho and Zipfel, 2015). The Xcv type III effector XopJ, a member of the YopJ family of SUMO peptidases and acetyltransferases, is attached to the plasma membrane of plant cells through a myristoylation motif and interferes with host-cell protein secretion, inhibiting immune-associated callose deposition at the cell wall (Bartetzko et al., 2009; Üstün et al., 2013). In addition, XopB and XopS contribute to Xcv virulence, suppressing PAMP-triggered gene expression (Schulze et al., 2012). XopB localizes to the Golgi vesicles and cytoplasm and interferes with plant cell protein secretion (Schulze et al., 2012). However, specific PTI-related targets of these Xcv effectors are unknown. Overall, better understanding of the role of vesicle trafficking in promoting plant immune responses related to ROS signaling requires further experimental evidence.
Metabolic priming by secreted pathogen effectors has emerged as a common strategy for host manipulation (Figure 3). U. maydis secretes chorismate mutase (Cmu1) as a virulence factor into plant cells, suppressing plant defense responses associated with pathogen-induced salicylic acid biosynthesis (Djamei et al., 2011). During U. maydis infection in maize, Cmu1 is translocated into plant cells, spreads to neighboring cells, and can change the metabolic status of plant cells through metabolic priming. Pathogen effectors may target plant enzymes required for ROS production in the key metabolic pathways. For instance, the chloroplastic enzyme aspartate oxidase, which is involved in NAD metabolism, is required for the NADPH oxidase RBOHD-mediated ROS burst that is triggered by the perception of several unrelated PAMPs (Macho et al., 2012). Notably, inducible NAD overproduction in Arabidopsis transcriptionally up-regulates aspartate oxidase during the incompatible infection with P. syringae pv. tomato (avrRpm1) (Pétriacq et al., 2012). Intracellular NAD acts as an integral regulator of multiple defense layers to trigger the production of ROS and defense hormones (Pétriacq et al., 2016). Manipulation of abscisic acid (ABA) content in Arabidopsis modulates ROS production via the control of peroxidase activity in response to Dickeya dadantii infection (van Gijsegem et al., 2017). Increased ABA contents seem likely to correlate with reduced ROS production and with enhanced disease susceptibility. The M. oryzae effector AvrPii specifically interacts with rice NADP-malic enzyme2 (OsNADP-ME2) to suppress the host ROS burst (Table 1; Singh et al., 2016). Indeed, purified AvrPii proteins inhibit in vitro NADP-ME activity. NADP-ME, also known as a coenzyme, catalyzes the oxidative decarboxylation of malate and NADP+, and provides NADPH as an electron donor for plasma membrane-bound NADPH oxidase (Figure 1), which is essential for the apoplastic ROS burst at the infection site (Doubnerová and Ryšlavá, 2011). NADP-ME is involved in the production of ROS during early plant basal defense against the hemi-biotrophic fungal pathogen Colletotrichum higginsianum (Voll et al., 2012). The X. oryzae pv. oryzicola effector AvrRxo1 targets and phosphorylates the central metabolite and redox carrier NAD in planta, and this catalytic activity is required for suppression of the ROS burst (Shidore et al., 2017). The X. campestris pv. vesicatoria (Xcv) effector AvrBsT physically interacts with pepper arginine decarboxylase (CaADC1), mediating polyamine metabolism for ROS signaling, cell death, and defense responses in plants (Table 1; Kim et al., 2013). CaADC1 silencing in pepper plants greatly reduces ROS and nitric oxide (NO) bursts, as well as the cell death response during Xcv infection, suggesting that arginine decarboxylase is required for polyamine and ROS signaling in the HR cell death response.
Concluding Remarks
Microbial pathogens have evolved efficient strategies to overcome plant innate immunity for the establishment of compatible plant-pathogen interactions. Despite their evolution over a long period of time, pathogen effectors target a common host protein network, suppressing the common immune system of all plants (Weßling et al., 2014). In particular, adapted pathogens have developed effective weaponry to compete with the host plants and defeat evolving plant immunity (Jones and Dangl, 2006). In this arms race, the most frequent target of pathogen attack is a powerful plant weapon system that inflicts immense damage to invading pathogens in a short period of time (Doehlemann et al., 2014).
Effector targeting toward ROS signaling networks in plants is proposed in Figure 3. Adapted pathogens have evolved virulence effectors to inhibit both the generation and accumulation of ROS in the apoplastic space, ultimately leading to the inhibition of the intracellular signaling required for the powerful second ROS burst. Plant pathogens deliver virulence effectors into the apoplastic area and cytoplasm of plant cells. Apoplastic effectors interfere with the perception of PRRs by MAMPs or PAMPs, preventing activation of membrane-bound NADPH oxidase RBOHs. Removal of ROS from the apoplastic area reduces direct toxicity to pathogens and blocks plant cell wall reinforcement, which may be beneficial for the successful intracellular invasion and colonization of microbial pathogens. Cytoplasmic effectors target plant PRRs, the RLCK BIK1, MAPK cascades, and WRKY transcription factors, suppressing the expression of RBOHs and NADP-MEs that are essential for robust ROS generation (Figure 3; Singh et al., 2016). During pathogen infection, certain cytoplasmic effectors interfere with vesicle trafficking to suppress the transport of ROS-producing RBOH enzymes to the plasma membrane (Macho and Zipfel, 2015). Secreted pathogen effectors block the metabolic coenzyme NADP-ME, inhibiting the transfer of electrons to NADPH oxidases (RBOHs). However, when specific pathogen effectors interact directly or indirectly with intracellular NLR proteins, these effectors function as avirulence factors to trigger resistance (R) gene-mediated immunity, the so-called ETI (Dangl et al., 2013). The effector-NLR complex leads to a strong apoplastic ROS burst and HR cell death responses. Apoplastic ROS are toxic to pathogens and activate MAPK cascades and NADPH oxidase (RBOH) enzymes. The first phase of ROS production by PTI following PAMP recognition may eventually have a feedback effect on the second phase of ROS production, resulting in a more powerful ETI (Figure 2; Yoshioka et al., 2003; Ishihama et al., 2011; Adachi et al., 2015).
Pathogens have developed virulence effectors to circumvent the newly changed plant immune system during the coevolution of both pathogens and host plants. In host plants, effector-targeted host proteins inside the plant cell can be modified to restrict pathogen invasion. Virulence effectors interact with different target host proteins in the plant cell, which can ultimately result in the suppression of ROS production in the apoplastic area. Once NADP-ME fails to supply NADPH to the NADPH oxidase (RBOH) as a result of its mutation, apoplastic ROS production is inhibited and pathogens can overcome plant immunity (Singh et al., 2016). These results suggest that ROS contribute directly to plant immunity. PTI and ETI are closely related and a strong ROS burst is essential for ETI. The metabolic cellular processes related to ROS generation are required to sustain PTI and reinforce the immune response through ETI. The key proteins and/or enzymes involved in ROS production may be supplied by activation of MAPK signaling pathways (Ishihama et al., 2011; Adachi et al., 2015). The biomembrane channels, aquaporins, mediate H2O2 transport across biological membranes. However, when effectors interfere with MAPK cascades and WRKY transcription factors (Zhang et al., 2007, 2012; Wang et al., 2010; Feng and Zhou, 2012; Le Roux et al., 2015), the second phase ROS burst and accompanying ETI are severely inhibited (Adachi et al., 2015).
Microbial pathogens have evolved versatile effectors to target the cellular processes associated with plant ROS production. Despite recent advances in our knowledge of pathogen effector targets in ROS signaling networks in plants (Table 1), the key host factors directly linking ROS signaling to sites of attempted pathogen invasion are not fully understood. Further elucidation of the molecular and cellular functions of pathogen effectors and host factors underlying the ROS-mediated innate immune system will provide important clues to understand better how versatile effectors have evolved to converge on ROS signaling networks in plants.
Author Contributions
NSJ designed the outline of the manuscript and wrote major part of the story and BKH added his previous bacterial data and expertise to upgrade the whole text and Figures.
Funding
This research was supported by the Basic Science Research Program through the National Research Foundation of Korea (NRF) funded by the Ministry of Education (2016R1D1A1A09918756).
Conflict of Interest Statement
The authors declare that the research was conducted in the absence of any commercial or financial relationships that could be construed as a potential conflict of interest.
Acknowledgment
We thank Y. Kadota for valuable comments on the manuscript.
References
Adachi, H., Nakano, T., Miyagawa, N., Ishihama, N., Yoshioka, M., Katou, Y., et al. (2015). WRKY transcription factors phosphorylated by MAPK regulate a plant immune NADPH oxidase in Nicotiana benthamiana. Plant Cell 27, 2645–2663. doi: 10.1105/tpc.15.00213
Allan, A. C., and Fluhr, R. (1997). Two distinct sources of elicited reactive oxygen species in tobacco epidermal cells. Plant Cell 9, 1559–1572. doi: 10.1105/tpc.9.9.1559
An, Q., Hückelhoven, R., Kogel, K. H., and van Bel, A. J. E. (2006). Multivesicular bodies participate in a cell wall-associated defence response in barley leaves attacked by the pathogenic powdery mildew fungus. Cell Microbiol. 8, 1009–1019. doi: 10.1111/j.1462-5822.2006.00683
Apostol, I., Heinstein, P. F., and Low, P. S. (1989). Rapid stimulation of an oxidative burst during elicitation of cultured plant cells : role in defense and signal transduction. Plant Physiol. 90, 109–116.
Ashtamker, C., Kiss, V., Sagi, M., Davydov, O., and Fluhr, R. (2007). Diverse subcellular locations of cryptogein-induced reactive oxygen species production in tobacco bright yellow-2 cells. Plant Physiol. 143, 1817–1826. doi: 10.1104/pp.106.090902
Bartetzko, V., Sonnewald, S., Vogel, F., Hartner, K., Ruth Stadler, R., Hammes, U. Z., et al. (2009). The Xanthomonas campestris pv. vesicatoria type III effector protein XopJ inhibits protein secretion: evidence or interference with cell wall–associated defense responses. Mol. Plant Microbe Interact. 22, 655–664. doi: 10.1094/MPMI-22-6-0655
Baxter, A., Mittler, R., and Suzuki, N. (2014). ROS as key players in plant stress signalling. J. Exp. Bot. 65, 1229–1240. doi: 10.1093/jxb/ert375
Bi, G., and Zhou, J. M. (2017). MAP kinase signaling pathways: a hub of plant-microbe interactions. Cell Host Microbe 21, 270–273. doi: 10.1016/j.chom.2017.02.004
Bienert, G. P., and Chaumont, F. (2014). Aquaporin-facilitated transmembrane diffusion of hydrogen peroxide. Biochim. Biophys. Acta 1840, 1596–1604. doi: 10.1016/j.bbagen.2013.09.017
Bindschedler, L. V., Dewdney, J., Blee, K. A., Stone, J. M., Asai, T., Plotnikov, J., et al. (2006). Peroxidase-dependent apoplastic oxidative burst in Arabidopsis required for pathogen resistance. Plant J. 47, 851–863. doi: 10.1111/j.1365-313X.2006.02837
Boller, T., and He, S. Y. (2009). Innate immunity in plants: an arms race between pattern recognition receptors in plants and effectors in microbial pathogens. Science 324, 742–744. doi: 10.1126/science.1171647
Bradley, D. J., Kjellbom, P., and Lamb, C. J. (1992). Elicitor- and wound-induced oxidative cross-linking of a proline-rich plant cell wall protein: a novel, rapid defense response. Cell 70, 21–30.
Cesari, S., Bernoux, M., Moncuquet, P., Kroj, T., and Dodds, P. N. (2014). A novel conserved mechanism for plant NLR protein pairs: the “integrated decoy” hypothesis. Front. Plant Sci. 5:606. doi: 10.3389/fpls.2014.00606
Chi, M. H., Park, S. Y., Kim, S., and Lee, Y. H. (2009). A novel pathogenicity gene is required in the rice blast fungus to suppress the basal defenses of the host. PLOS Pathog. 5:e1000401. doi: 10.1371/journal.ppat.1000401
Chi, Y., Yang, Y., Zhou, Y., Zhou, J., Fan, B., Yu, J. Q., et al. (2013). Protein-protein interactions in the regulation of WRKY transcription factors. Mol. Plant 6, 287–300. doi: 10.1093/mp/sst026
Chinchilla, D., Zipfel, C., Robatzek, S., Kemmerling, B., Nürnberger, T., Jones, J. D., et al. (2007). A flagellin-induced complex of the receptor FLS2 and BAK1 initiates plant defence. Nature 448, 497–500. doi: 10.1038/nature05999
Choi, H. W., Kim, D. S., Kim, N. H., Jung, H. W., Ham, J. H., and Hwang, B. K. (2013). Xanthomonas filamentous hemagglutinin-like protein Fha1interacts with pepper hypersensitive-induced reaction protein CaHIR1 and functions as a virulence factor in host plants. Mol. Plant Microbe Interact. 26, 1441–1454. doi: 10.1094/MPMI-07-13-0204-R
Choi, H. W., Kim, Y. J., Lee, S. C., Hong, J. K., and Hwang, B. K. (2007). Hydrogen peroxide generation by the pepper extracellular peroxidase CaPO2 activates local and systemic cell death and defense response to bacterial pathogens. Plant Physiol. 145, 890–904. doi: 10.1104/pp.107.103325
Colcombet, J., and Hirt, H. (2008). Arabidopsis MAPKs: a complex signalling network involved in multiple biological processes. Biochem. J. 413, 217–226. doi: 10.1042/BJ20080625
Collins, N. C., Thordal-Christensen, H., Lipka, V., Bau, S., Kombrink, E., Qiu, J. L., et al. (2003). SNARE-protein-mediated disease resistance at the plant cell wall. Nature 425, 973–977. doi: 10.1038/nature02076
Dangl, J. L., Horvath, D. M., and Staskawicz, B. J. (2013). Pivoting the plant immune system from dissection to deployment. Science 341, 746–751. doi: 10.1126/science.1236011
Daudi, A., Cheng, Z., O’Brien, J. A., Mammarella, N., Khan, S., Ausubel, F. M., et al. (2012). The apoplastic oxidative burst peroxidase in Arabidopsis is a major component of pattern-triggered immunity. Plant Cell 24, 275–287. doi: 10.1105/tpc.111.093039
de Jonge, R., van Esse, H. P., Kombrink, A., Shinya, T., Desaki, Y., Bours, R., et al. (2010). Conserved fungal LysM effector Ecp6 prevents chitin-triggered immunity in plants. Science 329, 953–955. doi: 10.1126/science.ll90859
Deepak, S., Shailasree, S., Kini, R. K., Hause, B., Shetty, S. H., and Mithöfer, A. (2007). Role of hydroxyproline-rich glycoproteins in resistance of pearl millet against downy mildew pathogen Sclerospora graminicola. Planta 226, 323–333. doi: 10.1007/s00425-007-0484-4
Deslandes, L., Olivier, J., Peeters, N., Feng, D. X., Khounlotham, M., Boucher, C., et al. (2003). Physical interaction between RRS1-R, a protein conferring resistance to bacterial wilt, and PopP2, a type III effector targeted to the plant nucleus. Proc. Natl. Acad. Sci. U.S.A. 100, 8024–8029. doi: 10.1073/pnas.1230660100
Djamei, A., Schipper, K., Rabe, F., Ghosh, A., Vincon, V., Kahnt, J., et al. (2011). Metabolic priming by a secreted fungal effector. Nature 478, 395–398. doi: 10.1038/nature10454
Doehlemann, G., Requena, N., Schaefer, P., Brunner, F., O’Connell, R., and Parker, J. E. (2014). Reprogramming of plant cells by filamentous plant-colonizing microbes. New Phytol. 204, 803–814. doi: 10.1111/nph.12938
Doehlemann, G., van der Linde, K., Assmann, D., Schwammbach, D., Hof, A., Mohanty, A., et al. (2009). Pep1, a secreted effector protein of Ustilago maydis, is required for successful invasion of plant cells. PLOS Pathog. 5:e1000290. doi: 10.1371/journal.ppat.1000290
Doke, N. (1983). Involvement of superoxide anion generation in the hypersensitive response of potato-tuber tissues to infection with an incompatible race of Phytophthora-infestans and to the hyphal wall components. Physiol. Plant Pathol. 23, 345–357. doi: 10.1016/0048-4059(83)90019-X
Doubnerová, V., and Ryšlavá, H. (2011). What can enzymes of C4 photosynthesis do for C3 plants under stress? Plant Sci. 180, 575–583. doi: 10.1016/j.plantsci.2010.12.005
Eitas, T. K., and Dangl, J. L. (2010). NB-LRR proteins: pairs, pieces, perception, partners, and pathways. Curr. Opin. Plant Biol. 13, 472–477. doi: 10.1016/j.pbi.2010.04.007
Ellinger, D., Glöckner, A., Koch, J., Naumann, M., Stürtz, V., Schütt, K., et al. (2014). Interaction of the Arabidopsis GTPase RabA4c with its effector PMR4 results in complete penetration resistance to powdery mildew. Plant Cell 26, 3185–3200. doi: 10.1105/tpc.114.127779
Ellinger, D., Naumann, M., Falter, C., Zwikowics, C., Jamrow, T., Manisseri, C., et al. (2013). Elevated early callose deposition results in complete penetration resistance to powdery mildew in Arabidopsis. Plant Physiol. 161, 1433–1444. doi: 10.1104/pp.112.211011
Fabro, G., Steinbrenner, J., Coates, M., Ishaque, N., Baxter, L., Studholme, D. J., et al. (2011). Multiple candidate effectors from the oomycete pathogen Hyaloperonospora arabidopsidis suppress host plant immunity. PLOS Pathog. 7:e1002348. doi: 10.1371/journal.ppat.1002348
Feng, F., Yang, F., Rong, W., Wu, X., Zhang, J., Chen, S., et al. (2012). A Xanthomonas uridine 5’-monophosphate transferase inhibits plant immune kinases. Nature 485, 114–118. doi: 10.1038/nature10962
Feng, F., and Zhou, J. M. (2012). Plant-bacterial pathogen interactions mediated by type III effectors. Curr. Opin. Plant Biol. 15, 469–476. doi: 10.1016/j.pbi.2012.03.004
Frederickson Matika, D. E., and Loake, G. J. (2014). Redox regulation in plant immune function. Antioxid. Redox Signal. 21, 1373–1388. doi: 10.1089/ars.2013.5679
Gabriel, D. W., and Rolfe, B. G. (1990). Working models of specific recognition in plant-mirocbe interactions. Annu. Rev. Phytopathol. 28, 365–391.
Gimenez-Ibanez, S., Hann, D. R., Ntoukakis, V., Petutschnig, E., Lipka, V., and Rathjen, J. P. (2009). AvrPtoB targets the LysM receptor kinase CERK1 to promote bacterial virulence on plants. Curr. Biol. 19, 423–429. doi: 10.1016/j.cub.2009.01.054
Gómez-Gómez, L., and Boller, T. (2000). FLS2: an LRR receptor-like kinase involved in the perception of the bacterial elicitor flagellin in Arabidopsis. Mol. Cell 5, 1003–1011.
Grant, J. J., and Loake, G. J. (2000). Role of reactive oxygen intermediates and cognate redox signaling in disease resistance. Plant Physiol. 124, 21–29.
Greenberg, J. T., and Yao, N. (2004). The role and regulation of programmed cell death in plant-pathogen interactions. Cell Microbiol. 6, 201–211. doi: 10.1111/j.1462-5822.2004.00361
Han, S. W., and Hwang, B. K. (2017). Molecular functions of Xanthomonas type III effector AvrBsT and its plant interactors in cell death and defense signaling. Planta 245, 237–253. doi: 10.1007/s00425-016-2628-x
He, P., Shan, L., Lin, N. C., Martin, G. B., Kemmerling, B., Nürnberger, T., et al. (2006). Specific bacterial suppressors of MAMP signaling upstream of MAPKKK in Arabidopsis innate immunity. Cell 125, 563–575. doi: 10.1016/j.cell.2006.02.047
Hemetsberger, C., Herrberger, C., Zechmann, B., Hillmer, M., and Doehlemann, G. (2012). The Ustilago maydis effector Pep1 suppresses plant immunity by inhibition of host peroxidase activity. PLOS Pathog. 8:e1002684. doi: 10.1371/journal.ppat.1002684
Huang, K., Czymmek, K. J., Caplan, J. L., Sweigard, J. A., and Donofrio, N. M. (2011). HYR1-mediated detoxification of reactive oxygen species is required for full virulence in the rice blast fungus. PLOS Pathog. 7:e1001335. doi: 10.1371/journal.ppat.1001335
Hückelhoven, R., Fodor, J., Preis, C., and Kogel, K. H. (1999). Hypersensitive cell death and papilla formation in barley attacked by the powdery mildew fungus are associated with hydrogen peroxide but not with salicylic acid accumulation. Plant Physiol. 119, 1251–1260.
Inada, N., and Ueda, T. (2014). Membrane trafficking pathways and their roles in plant-microbe interactions. Plant Cell Physiol. 55, 672–686. doi: 10.1093/pcp/pcu046
Ishihama, N., Yamada, R., Yoshioka, M., Katou, S., and Yoshioka, H. (2011). Phosphorylation of the Nicotiana benthamiana WRKY8 transcription factor by MAPK functions in the defense response. Plant Cell 23, 1153–1170. doi: 10.1105/tpc.110.081794
Jabs, T. (1999). Reactive oxygen intermediates as mediators of programmed cell death in plants and animals. Biochem. Pharmacol. 57, 231–245.
Jones, J. D., and Dangl, J. L. (2006). The plant immune system. Nature 444, 323–329. doi: 10.1038/nature05286
Kadota, Y., Shirasu, K., and Zipfel, C. (2015). Regulation of the NADPH oxidase RBOHD during plant immunity. Plant Cell Physiol. 56, 1472–1480. doi: 10.1093/pcp/pcv063
Kadota, Y., Sklenar, J., Derbyshire, P., Stransfeld, L., Asai, S., Ntoukakis, V., et al. (2014). Direct regulation of the NADPH oxidase RBOHD by the PRR-associated kinase BIK1 during plant immunity. Mol. Cell 54, 43–55. doi: 10.1016/j.molcel.2014.02.021
Kim, N. H., Choi, H. W., and Hwang, B. K. (2010). Xanthomonas campestris pv. vesicatoria effector AvrBsT induces cell death in pepper, but suppresses defense responses in tomato. Mol. Plant Microbe Interact. 23, 1069–1082. doi: 10.1094/MPMI-23-8-1069
Kim, N. H., and Hwang, B. K. (2015a). Pepper aldehyde dehydrogenase CaALDH1 interacts with Xanthomonas effector AvrBsT and promotes effector-triggered cell death and defence responses. J. Exp. Bot. 66, 3367–3380. doi: 10.1093/jxb/erv147
Kim, N. H., and Hwang, B. K. (2015b). Pepper heat shock protein 70a interacts with the type III effector AvrBsT and triggers plant cell death and immunity. Plant Physiol. 167, 307–322. doi: 10.1104/pp.114.253898
Kim, N. H., Kim, B. S., and Hwang, B. K. (2013). Pepper arginine decarboxylase is required for polyamine and upgamma-aminobutyric acid signaling in cell death and defense response. Plant Physiol. 162, 2067–2083. doi: 10.1104/pp.113.217372
Kim, N. H., Kim, D. S., Chung, E. H., and Hwang, B. K. (2014). Pepper suppressor of the G2 allele of skp1 interacts with the receptor-like cytoplasmic kinase1 and type III effector AvrBsT and promotes the hypersensitive cell death response in a phosphorylation-dependent manner. Plant Physiol. 165, 76–91. doi: 10.1104/pp.114.238840
King, S. R., McLellan, H., Boevink, P. C., Armstrong, M. R., Bukharova, T., Sukarta, O., et al. (2014). Phytophthora infestans RXLR effector PexRD2 interacts with host MAPKKK ε to suppress plant immune signaling. Plant Cell 26, 1345–1359. doi: 10.1105/tpc.113.120055
Kvitko, B. H., Park, D. H., Velásquez, A. C., Wei, C. F., Russell, A. B., Martin, G. B., et al. (2009). Deletions in the repertoire of Pseudomonas syringae pv. tomato DC3000 type III secretion effector genes reveal functional overlap among effectors. PLOS Pathog. 5:e1000388. doi: 10.1371/journal.ppat.1000388
La, D. R., and López-Huertas, E. (2016). ROS generation in peroxisomes and its role in cell signaling. Plant Cell Physiol. 57, 1364–1376. doi: 10.1093/pcp/pcw076
Lamb, C., and Dixon, R. A. (1997). The oxidative burst in plant disease resistance. Annu. Rev. Plant Physiol. Plant Mol. Biol. 48, 251–275. doi: 10.1146/annurev.arplant.48.1.251
Le Roux, C., Huet, G., Jauneau, A., Camborde, L., Trémousaygue, D., Kraut, A., et al. (2015). A receptor pair with an integrated decoy converts pathogen disabling of transcription factors to immunity. Cell 161, 1074–1088. doi: 10.1016/j.cell.2015.04.025
Lee, W. S., Rudd, J. J., Hammond-Kosack, K. E., and Kanyuka, K. (2014). Mycosphaerella graminicola LysM effector-mediated stealth pathogenesis subverts recognition through both CERK1 and CEBiP homologues in wheat. Mol. Plant Microbe Interact. 27, 236–243. doi: 10.1094/MPMI-07-13-0201-R
Legendre, L., Rueter, S., Heinstein, P. F., and Low, P. S. (1993). Characterization of the oligogalacturonide-induced oxidative burst in cultured soybean (Glycine max) cells. Plant Physiol. 102, 233–240.
Lehmann, S., Serrano, M., L’Haridon, F., Tjamos, S. E., and Metraux, J. P. (2015). Reactive oxygen species and plant resistance to fungal pathogens. Phytochemistry 112, 54–62. doi: 10.1016/j.phytochem.2014.08.027
Levine, A., Tenhaken, R., Dixon, R., and Lamb, C. (1994). H2O2 from the oxidative burst orchestrates the plant hypersensitive disease resistance response. Cell 79, 583–593.
Li, L., Li, M., Yu, L., Zhou, Z., Liang, X., Liu, Z., et al. (2014). The FLS2- associated kinase BIK1 directly phosphorylates the NADPH oxidase RbohD to control plant immunity. Cell Host Microbe 15, 329–338. doi: 10.1016/j.chom.2014.02.009
Liu, Y., Ren, D., Pike, D., Pallardy, S., Gassmann, W., and Zhang, S. (2007). Chloroplast-generated reactive oxygen species are involved in hypersensitive response-like cell death mediated by a mitogen-activated protein kinase cascade. Plant J. 51, 941–954. doi: 10.1111/j.1365-313X.2007.03191
Lu, D., Wu, S., Gao, X., Zhang, Y., Shan, L., and He, P. (2010). A receptor-like cytoplasmic kinase, BIK1, associates with a flagellin receptor complex to initiate plant innate immunity. Proc. Natl. Acad. Sci. U.S.A. 107, 496–501. doi: 10.1073/pnas.0909705107
Luna, E., Pastor, V., Robert, J., Flors, V., Mauch-Mani, B., and Ton, J. (2011). Callose deposition: a multifaceted plant defense response. Mol. Plant Microbe Interact. 24, 183–193. doi: 10.1094/MPMI-07-10-0149
Macho, A. P., Boutrot, F., Rathjen, J. P., and Zipfel, C. (2012). Aspartate oxidase plays an important role in Arabidopsis stomatal immunity. Plant Physiol. 159, 1845–1856. doi: 10.1104/pp.112.199810
Macho, A. P., Schwessinger, B., Ntoukakis, V., Brutus, A., Segonzac, C., Roy, S., et al. (2014). A bacterial tyrosine phosphatase inhibits plant pattern recognition receptor activation. Science 343, 1509–1512. doi: 10.1126/science.1248849
Macho, A. P., and Zipfel, C. (2015). Targeting of plant pattern recognition receptor-triggered immunity by bacterial type-III secretion system effectors. Curr. Opin. Microbiol. 23, 14–22. doi: 10.1016/j.mib.2014.10.009
Marshall, R., Kombrink, A., Motteram, J., Loza-Reyes, E., Lucas, J., Hammond-Kosack, K. E., et al. (2011). Analysis of two in planta expressed LysM effector homologs from the fungus Mycosphaerella graminicola reveals novel functional properties and varying contributions to virulence on wheat. Plant Physiol. 156, 756–769. doi: 10.1104/pp.111.176347
McHale, L., Tan, X., Koehl, P., and Michelmore, R. W. (2006). Plant NBS-LRR proteins: adaptable guards. Genome Biol. 7:212. doi: 10.1186/gb-2006-7-4-212
Mentlak, T. A., Kombrink, A., Shinya, T., Ryder, L. S., Otomo, I., Saitoh, H., et al. (2012). Effector-mediated suppression of chitin-triggered immunity by Magnaporthe oryzae is necessary for rice blast disease. Plant Cell 24, 322–335. doi: 10.1105/tpc.111.092957
Mittler, R., Vanderauwera, S., Suzuki, N., Miller, G., Tognetti, V. B., Vandepoele, K., et al. (2011). ROS signaling: the new wave? Trends Plant Sci. 16, 300–309. doi: 10.1016/j.tplants.2011.03.007
Mori, I. C., and Schroeder, J. I. (2004). Reactive oxygen species activation of plant Ca2+ channels. A signaling mechanism in polar growth, hormone transduction, stress signaling, and hypothetically mechanotransduction. Plant Physiol. 135, 702–708. doi: 10.1104/pp.104.042069
Mueller, A. N., Ziemann, S., Treitschke, S., Aßmann, D., and Doehlemann, G. (2013). Compatibility in the Ustilago maydis-maize interaction requires inhibition of host cysteine proteases by the fungal effector Pit2. PLOS Pathog. 9:e1003177. doi: 10.1371/journal.ppat.1003177
Noirot, E., Der, C., Lherminier, J., Robert, F., Moricova, P., Kiêu, K., et al. (2014). Dynamic changes in the subcellular distribution of the tobacco ROS-producing enzyme RBOHD in response to the oomycete elicitor cryptogein. J. Exp. Bot. 65, 5011–5022. doi: 10.1093/jxb/eru265
Nomura, K., Debroy, S., Lee, Y. H., Pumplin, N., Jones, J., and He, S. Y. (2006). A bacterial virulence protein suppresses host innate immunity to cause plant disease. Science 313, 220–223. doi: 10.1126/science.1129523
Nomura, K., Mecey, C., Lee, Y. N., Imboden, L. A., Chang, J. H., and He, S. Y. (2011). Effector-triggered immunity blocks pathogen degradation of an immunity-associated vesicle traffic regulator in Arabidopsis. Proc. Natl. Acad. Sci. U.S.A. 108, 10774–10779. doi: 10.1073/pnas.1103338108
Nühse, T. S., Bottrill, A. R., Jones, A. M., and Peck, S. C. (2007). Quantitative phosphoproteomic analysis of plasma membrane proteins reveals regulatory mechanisms of plant innate immune responses. Plant J. 51, 931–940. doi: 10.1111/j.1365-313X.2007.03192
O’Brien, J. A., Daudi, A., Butt, V. S., and Bolwell, G. P. (2012a). Reactive oxygen species and their role in plant defence and cell wall metabolism. Planta 236, 765–779. doi: 10.1007/s00425-012-1696-9
O’Brien, J. A., Daudi, A., Finch, P., Butt, V. S., Whitelegge, J. P., Souda, P., et al. (2012b). A peroxidase-dependent apoplastic oxidative burst in cultured Arabidopsis cells functions in MAMP-elicited defense. Plant Physiol. 158, 2013–2027. doi: 10.1104/pp.111.190140
Park, S. Y., Choi, J., Lim, S. E., Lee, G. W., Park, J., Kim, Y., et al. (2013). Global expression profiling of transcription factor genes provides new insights into pathogenicity and stress responses in the rice blast fungus. PLOS Pathog. 9:e1003350. doi: 10.1371/journal.ppat.1003350
Pétriacq, P., de Bont, L., Hager, J., Didierlaurent, L., Mauve, C., Guérard, F., et al. (2012). Inducible NAD overproduction in Arabidopsis alters metabolic pools and gene expression correlated with increased salicylate content and resistance to Pst-AvrRpm1. Plant J. 70, 650–665. doi: 10.1111/j.1365-313X.2012.04920
Pétriacq, P., Ton, J., Patrit, O., Tcherkez, G., and Gakiere, B. (2016). NAD acts as an integral regulator of multiple defense layers. Plant Physiol. 172, 1465–1479.
Pitzschke, A., Schikora, A., and Hirt, H. (2009). MAPK cascade signalling networks in plant defence. Curr. Opin. Plant Biol. 12, 421–426. doi: 10.1016/j.pbi.2009.06.008
Ranf, S., Eschen-Lippold, L., Fröhlich, K., Westphal, L., Scheel, D., and Lee, J. (2014). Microbe-associated molecular pattern-induced calcium signaling requires the receptor-like cytoplasmic kinases, PBL1 and BIK1. BMC Plant Biol. 14:374. doi: 10.1186/s12870-014-0374-4
Rooney, H. C. E., van’t Klooster, J. W., van der Hoorn, R. A. L., Joosten, M. H. A. J., Jones, J. D. G., and de Wit, P. J. G. M. (2005). Cladosporium Avr2 inhibits tomato Rcr3 protease required for Cf-2-dependent disease resistance. Science 308, 1783–1786. doi: 10.1126/science.1111404
Rovenich, H., Zuccaro, A., and Thomma, B. P. (2016). Convergent evolution of filamentous microbes towards evasion of glycan-triggered immunity. New Phytol. 212, 896–901. doi: 10.1111/nph.14064
Sagi, M., and Fluhr, R. (2006). Production of reactive oxygen species by plant NADPH oxidases. Plant Physiol. 141, 336–340. doi: 10.1104/pp.106.078089
Sánchez-Vallet, A., Mesters, J. R., and Thomma, B. P. H. J. (2014). The battle for chitin recognition in plant–microbe interactions. FEMS Microbiol. Rev. 39, 171–183. doi: 10.1093/femsre/fuu003
Schulze, S., Kay, S., Büttner, D., Egler, M., Eschen-Lippold, L., Hause, G., et al. (2012). Analysis of new type III effectors from Xanthomonas uncovers XopB and XopS as suppressors of plant immunity. New Phytol. 195, 894–911. doi: 10.1111/j.1469-8137.2012.04210
Shabab, M., Shindo, T., Gu, C., Kaschani, F., Pansuriya, T., Chintha, R., et al. (2008). Fungal effector protein AVR2 targets diversifying defense-related cys proteases of tomato. Plant Cell 20, 1169–1183. doi: 10.1105/tpc.107.056325
Shan, L., He, P., Li, J., Heese, A., Peck, S. C., Nürnberger, T., et al. (2008). Bacterial effectors target the common signaling partner BAK1 to disrupt multiple MAMP receptor-signaling complexes and impede plant immunity. Cell Host Microbe 4, 17–27. doi: 10.1016/j.chom.2008.05.017
Shi, H., Shen, Q., Qi, Y., Yan, H., Nie, H., Chen, Y., et al. (2013). BR-SIGNALING KINASE1 physically associates with FLAGELLIN SENSING2 and regulates plant innate immunity in Arabidopsis. Plant Cell 25, 1143–1157. doi: 10.1105/tpc.112.107904
Shidore, T., Broeckling, C. D., Kirkwood, J. S., Long, J. J., Miao, J., Zhao, B., et al. (2017). The effector AvrRxo1 phosphorylates NAD in planta. PLOS Pathog. 13:e1006442. doi: 10.1371/journal.ppat.1006442
Singh, R., Dangol, S., Chen, Y., Choi, J., Cho, Y. S., Lee, J. E., et al. (2016). Magnaporthe oryzae effector AVR-Pii helps to establish compatibility by inhibition of the rice NADP-malic enzyme resulting in disruption of oxidative burst and host innate immunity. Mol. Cells 39, 426–438. doi: 10.14348/molcells.2016.0094
Singh, R., Lee, J. E., Dangol, S., Choi, J., Yoo, R. H., Moon, J. S., et al. (2014). Protein interactome analysis of 12 mitogen-activated protein kinase kinase kinase in rice using a yeast two-hybrid system. Proteomics 14, 105–115. doi: 10.1002/pmic.201300125
Singh, R., Lee, M. O., Lee, J. E., Choi, J., Park, J. H., Kim, E. H., et al. (2012). Rice mitogen-activated protein kinase interactome analysis using the yeast two-hybrid system. Plant Physiol. 160, 477–487. doi: 10.1104/pp.112.200071
Spoel, S. H., and Dong, X. (2012). How do plants achieve immunity? Defence without specialized immune cells. Nat. Rev. Immunol. 12, 89–100. doi: 10.1038/nri3141
Tena, G., Boudsocq, M., and Sheen, J. (2011). Protein kinase signaling networks in plant innate immunity. Curr. Opin. Plant Biol. 14, 519–529. doi: 10.1016/j.pbi.2011.05.006
Tenhaken, R., Levine, A., Brisson, L. F., Dixon, R. A., and Lamb, C. (1995). Function of the oxidative burst in hypersensitive disease resistance. Proc. Natl. Acad. Sci. U.S.A. 92, 4158–4163. doi: 10.1073/pnas.92.10.4158
Tian, S., Wang, X., Li, P., Wang, H., Ji, H., Xie, J., et al. (2016). Plant aquaporin AtPIP1;4 links apoplastic H2O2 induction to disease immunity pathways. Plant Physiol. 171, 1635–1650. doi: 10.1104/pp.15.01237
Torres, M. A. (2010). ROS in biotic interactions. Physiol. Plant. 138, 414–429. doi: 10.1111/j.1399-3054.2009.01326
Torres, M. A., and Dangl, J. L. (2005). Functions of the respiratory burst oxidase in biotic interactions, abiotic stress and development. Curr. Opin. Plant Biol. 8, 397–403. doi: 10.1016/j.pbi.2005.05.014
Torres, M. A., Jones, J. D. G., and Dangl, J. L. (2006). Reactive oxygen species signaling in response to pathogens. Plant Physiol. 141, 373–378. doi: 10.1104/pp.106.079467
Üstün, S., Bartetzko, V., and Börnke, F. (2013). The Xanthomonas campestris type III effector XopJ targets the host cell proteasome to suppress salicylic-acid mediated plant defence. PLOS Pathog. 9:e1003427. doi: 10.1371/journal.ppat.1003427
van den Burg, H. A., Harrison, S. J., Joosten, M. H., Vervoort, J., and de Wit, P. J. (2006). Cladosporium fulvum Avr4 protects fungal cell walls against hydrolysis by plant chitinases accumulating during infection. Mol. Plant Microbe Interact. 19, 1420–1430. doi: 10.1094/MPMI-19-1420
van der Hoorn, R. A., and Kamoun, S. (2008). From guard to decoy: a new model for perception of plant pathogen effectors. Plant Cell 20, 2009–2017. doi: 10.1105/tpc.108.060194
van der Hoorn, R. A. L., and Jones, J. D. G. (2004). The plant proteolytic machinery and its role in defence. Curr. Opin. Plant Biol. 7, 400–407. doi: 10.1016/j.pbi.2004.04.003
van Gijsegem, F., Pédron, J., Patrit, O., Simond-Côte, E., Maia-Grondard, A., Pétriacq, P., et al. (2017). Manipulation of ABA content in Arabidopsis thaliana modifies sensitivity and oxidative stress response to Dickeya dadantii and influences peroxidase activity. Front. Plant Sci. 8:456. doi: 10.3389/fpls.2017.00456
Vidal, G., Ribas-Carbo, M., Garmier, M., Dubertret, G., Rasmusson, A. G., Mathieu, C., et al. (2007). Lack of respiratory chain complex I impairs alternative oxidase engagement and modulates redox signaling during elicitor-induced cell death in tobacco. Plant Cell 19, 640–655.
Voll, L. M., Zell, M. B., Engelsdorf, T., Saur, A., Gerrard Wheeler, M. C., Drincovich, M. F., et al. (2012). Loss of cytosolic NADP-malic enzyme 2 in Arabidopsis is associated with enhanced susceptibility towards Colletotrichum higginsianum. New Phytol. 195, 189–202. doi: 10.1111/j.1469-8137.2012.04129
Wang, Y., Li, J., Hou, S., Wang, X., Li, Y., Ren, D., et al. (2010). A Pseudomonas syringae ADP-ribosyltransferase inhibits Arabidopsis mitogen-activated protein kinase kinases. Plant Cell 22, 2033–2044. doi: 10.1105/tpc.110.075697
Wawra, S., Fesel, P., Widmer, H., Timm, M., Seibel, J., Leson, L., et al. (2016). The fungal-specific β-glucan-binding lectin FGB1 alters cell-wall composition and suppresses glucan-triggered immunity in plants. Nat. Commun. 7:13188. doi: 10.1038/ncomms13188
Weßling, R., Epple, P., Altmann, S., He, Y., Yang, L., Henz, S. R., et al. (2014). Convergent targeting of a common host protein-network by pathogen effectors from three kingdoms of life. Cell Host Microbe 16, 364–375. doi: 10.1016/j.chom.2014.08.004
Win, J., Chaparro-Garcia, A., Belhaj, K., Saunders, D. G., Yoshida, K., Dong, S., et al. (2012). Effector biology of plant-associated organisms: concepts and perspectives. Cold Spring Harb. Symp. Quant. Biol. 77, 235–247. doi: 10.1101/sqb.2012.77.015933
Wu, S., Lu, D., Kabbage, M., Wei, H. L., Swingle, B., Records, A. R., et al. (2011). Bacterial effector HopF2 suppresses Arabidopsis innate immunity at the plasma membrane. Mol. Plant Microbe Interact. 24, 585–593. doi: 10.1094/MPMI-07-10-0150
Yang, K. Y., Liu, Y., and Zhang, S. (2001). Activation of a mitogen-activated protein kinase pathway is involved in disease resistance in tobacco. Proc. Natl. Acad. Sci. U.S.A. 98, 741–746. doi: 10.1073/pnas.98.2.741
Yoshioka, H., Numata, N., Nakajima, K., Katou, S., Kawakita, K., Rowland, O., et al. (2003). Nicotiana benthamiana gp91phox homologs NbrbohA and NbrbohB participate in H2O2 accumulation and resistance to Phytophthora infestans. Plant Cell 15, 706–718. doi: 10.1105/tpc.008680
Zhang, J., Shao, F., Li, Y., Cui, H., Chen, L., Li, H., et al. (2007). A Pseudomonas syringae effector inactivates MAPKs to suppress PAMP-induced immunity in plants. Cell Host Microbe 1, 175–185. doi: 10.1016/j.chom.2007.03.006
Zhang, Z., Henderson, C., and Gurr, S. J. (2004). Blumeria graminis secretes an extracellular catalase during infection of barley: potential role in suppression of host defence. Mol. Plant Pathol. 5, 537–547. doi: 10.1111/j.1364-3703.2004.00251
Zhang, Z., Wu, Y., Gao, M., Zhang, J., Kong, Q., Liu, Y., et al. (2012). Disruption of PAMP-induced MAP kinase cascade by a Pseudomonas syringae effector activates plant immunity mediated by the NB-LRR protein SUMM2. Cell Host Microbe 11, 253–263. doi: 10.1016/j.chom.2012.01.015
Zheng, X., McLellan, H., Fraiture, M., Liu, X., Boevink, P. C., Gilroy, E. M., et al. (2014). Functionally redundant RXLR effectors from Phytophthora infestans act at different steps to suppress early flg22-triggered immunity. PLOS Pathog. 10:e1004057. doi: 10.1371/journal.ppat.1004057
Zhou, J., Wu, S., Chen, X., Liu, C., Sheen, J., Shan, L., et al. (2014). The Pseudomonas syringae effector HopF2 suppresses Arabidopsis immunity by targeting BAK1. Plant J. 77, 235–245. doi: 10.1111/tpj.12381
Keywords: pathogen effector, reactive oxygen species, PAMP-triggered immunity, effector-triggered immunity, respiratory burst oxidase homolog, mitogen-activated protein kinase
Citation: Jwa N-S and Hwang BK (2017) Convergent Evolution of Pathogen Effectors toward Reactive Oxygen Species Signaling Networks in Plants. Front. Plant Sci. 8:1687. doi: 10.3389/fpls.2017.01687
Received: 19 June 2017; Accepted: 13 September 2017;
Published: 29 September 2017.
Edited by:
Tatiana Matveeva, Saint Petersburg State University, RussiaReviewed by:
Jin-Long Qiu, Institute of Microbiology (CAS), ChinaPierre Pétriacq, University of Sheffield, United Kingdom
Copyright © 2017 Jwa and Hwang. This is an open-access article distributed under the terms of the Creative Commons Attribution License (CC BY). The use, distribution or reproduction in other forums is permitted, provided the original author(s) or licensor are credited and that the original publication in this journal is cited, in accordance with accepted academic practice. No use, distribution or reproduction is permitted which does not comply with these terms.
*Correspondence: Nam-Soo Jwa, bnNqd2FAc2Vqb25nLmFjLmty