- 1Aquatic Biology, Department of Bioscience, Aarhus University, Aarhus, Denmark
- 2Institute of Botany, The Czech Academy of Sciences, Průhonice, Czechia
- 3Department of Landscape Architecture and Horticulture, Temple University, Ambler, PA, United States
- 4Department of Entomology, Kansas State University, Manhattan, KS, United States
- 5Department of Natural Resources Science, University of Rhode Island, Kingston, RI, United States
- 6Department of Biological Sciences, Louisiana State University, Baton Rouge, LA, United States
- 7College of Landscape Architecture and Forestry, Qingdao Agricultural University, Qingdao, China
- 8Institute of Ecology and Biodiversity, School of Life Sciences, Shandong University, Jinan, China
- 9Department of Watershed Sciences and Ecology Center, Utah State University, Logan, UT, United States
- 10Department of Agricultural Sciences, University of Bologna, Bologna, Italy
- 11Smithsonian Environmental Research Center, Edgewater, MD, United States
- 12Department of Biology, Bryn Mawr College, Bryn Mawr, PA, United States
- 13Department of Ecology, Faculty of Science, Charles University, Prague, Czechia
Phragmites australis is a cosmopolitan grass and often the dominant species in the ecosystems it inhabits. Due to high intraspecific diversity and phenotypic plasticity, P. australis has an extensive ecological amplitude and a great capacity to acclimate to adverse environmental conditions; it can therefore offer valuable insights into plant responses to global change. Here we review the ecology and ecophysiology of prominent P. australis lineages and their responses to multiple forms of global change. Key findings of our review are that: (1) P. australis lineages are well-adapted to regions of their phylogeographic origin and therefore respond differently to changes in climatic conditions such as temperature or atmospheric CO2; (2) each lineage consists of populations that may occur in geographically different habitats and contain multiple genotypes; (3) the phenotypic plasticity of functional and fitness-related traits of a genotype determine the responses to global change factors; (4) genotypes with high plasticity to environmental drivers may acclimate or even vastly expand their ranges, genotypes of medium plasticity must acclimate or experience range-shifts, and those with low plasticity may face local extinction; (5) responses to ancillary types of global change, like shifting levels of soil salinity, flooding, and drought, are not consistent within lineages and depend on adaptation of individual genotypes. These patterns suggest that the diverse lineages of P. australis will undergo intense selective pressure in the face of global change such that the distributions and interactions of co-occurring lineages, as well as those of genotypes within-lineages, are very likely to be altered. We propose that the strong latitudinal clines within and between P. australis lineages can be a useful tool for predicting plant responses to climate change in general and present a conceptual framework for using P. australis lineages to predict plant responses to global change and its consequences.
Introduction
One of the greatest challenges in ecology is to understand, predict, and mitigate the consequences of climate change (IPCC, 2014). Climate change will affect species interactions, community structure, and biodiversity, and will induce major shifts in plant phenology and geographic ranges (e.g., Post, 2013; Visser, 2016). However, not all species will respond similarly to changing climatic conditions (Springate and Kover, 2014). In a highly variable and changing environment, globally distributed species will likely have the genetic variation needed to acclimate to a broad spectrum of environmental and climatic gradients (Jump and Peñuelas, 2005). So far, however, most efforts to assess species changes have focused on climate modeling (e.g., Thuiller et al., 2005; Munguia-Rosas et al., 2011; Niu et al., 2014) or experiments using plants that are unlikely to have widespread impacts on community diversity or ecosystem processes (e.g., Chapman et al., 2014; Springate and Kover, 2014).
Species with the high genetic diversity and heritable phenotypic variation typically seen in cosmopolitan species are likely to have more inherent flexibility to evolve in response to climate change than species with low intraspecific diversity and restricted geographic ranges (Lavergne and Molofsky, 2007). Moreover, genotypes with high phenotypic plasticity (i.e., a high capacity of a genotype to produce distinct phenotypes in response to environmental variation; Bradshaw, 1965) typically have a greater capacity to adapt to altered environmental conditions than species with low plasticity (Franks et al., 2014; Valladares et al., 2014). Despite the fact that intraspecific variation is the basis of evolutionary change (Hiesey et al., 1942), it has only recently gained notice in studies of species responses to global change (Violle et al., 2012; Aspinwall et al., 2013; Pauls et al., 2013; Meyerson et al., 2016a; Münzbergová et al., 2017). Widespread and genetically diverse species, including those that are invasive, may be buffered against the adverse effects of global change (Oney et al., 2013). Truly cosmopolitan species, such as Phragmites australis (Cav.) Trin. ex Steud. (common reed), have global distributions, high genetic and phenotypic variation, and occur in a wide range of environments. The high intraspecific diversity usually found within P. australis stands may provide the species with the ability to cope with and benefit from a rapidly changing climate (Jump and Peñuelas, 2005; Kettenring et al., 2010, 2011). However, some populations may experience decreased genetic diversity during the acclimation and adaptation processes (Almeida et al., 2013). At the community and ecosystem scales, local extinction (Bolnick et al., 2011) and the alteration of small-scale environmental conditions and species-interactions (Crutsinger et al., 2008; Schöb et al., 2013) may be the ultimate consequences of the loss of intraspecific diversity. Whilst it is highly unlikely that species with high intraspecific diversity could be threatened with total extinction, shifts in genetic composition, including the genetic impoverishment of a population, may occur (Franks et al., 2014; Valladares et al., 2014). Therefore, a key challenge awaiting future research is determining how intraspecific variation drives local species composition and mediates the effects of rapid environmental change.
Phragmites australis is a cosmopolitan species that has strong effects on the ecosystems it inhabits; it therefore can offer valuable insights into plant responses to global change (Den Hartog et al., 1989; Chambers et al., 1999; Koppitz, 1999; Engloner, 2009; Mozdzer and Megonigal, 2012; Caplan et al., 2015; Hughes et al., 2016). It is a robust and highly productive grass in the Poaceae family that occurs in a wide range of freshwater and brackish wetlands (Brix, 1999a; Meyerson et al., 2000) spanning temperate and tropical regions (Den Hartog et al., 1989). The success of P. australis as a cosmopolitan species is related to its high productivity, its rapid stand-scale expansion through both clonal and sexual reproduction, and its ability to evolve rapidly in new ranges (Kettenring et al., 2010, 2011, 2012, 2015; Douhovnikoff and Hazelton, 2014; Eller et al., 2014a; Saltonstall et al., 2014). Changes in the distribution and growth patterns of P. australis have strong socioeconomic and environmental impacts that may be influenced by, and also feedback on, changing climatic conditions (Kim et al., 1998; Dukes and Mooney, 1999; Brix et al., 2001; Windham and Meyerson, 2003). The species has undergone an almost exponential range-expansion in North America (Chambers et al., 1999), where it is considered one of the worst invasive species on the continent (Saltonstall, 2002; Hazelton et al., 2014). Its global distribution and ability to proliferate in a wide range of habitats, especially in areas where physical disturbances are abundant, appear to derive from its distinct ecophysiological strategies, broad ecological amplitude, high evolutionary potential, and high phenotypic plasticity (Eller and Brix, 2012; Kettenring and Mock, 2012; Mozdzer and Megonigal, 2012; Mozdzer et al., 2013; Guo et al., 2014; Kettenring et al., 2015, 2016; Bhattarai et al., 2017a; Packer et al., 2017b). Like other cosmopolitan invasive plant species (Lavergne and Molofsky, 2004), P. australis has recently been suggested as a model organism for studying plant invasions (Meyerson et al., 2016b; Packer et al., 2017a). Given its highly plastic physiological and morphological responses to interacting global change factors (Eller and Brix, 2012; Mozdzer and Megonigal, 2012; Eller et al., 2013, 2014a,b; Caplan et al., 2015), P. australis may also provide insights into global change responses of other plant species.
Despite the large body of knowledge generated by prior research on P. australis, it is perhaps surprising that there is no global synthesis of the genetic variability of P. australis, its functional traits, its ecophysiology, and how the performance of the species is expected to change in a rapidly changing environment, especially under the expected scenarios of global climate change. Our goal here is to provide a comprehensive review of the high intraspecific variation of the ecophysiological processes that allow P. australis, as a cosmopolitan species, to respond to global change factors such as temperature, atmospheric CO2 concentrations, drought, flooding, salinity, and eutrophication. We further aim to highlight the value of P. australis as a model species both for plant invasions, a widespread phenomenon with accelerating dynamics (van Kleunen et al., 2015; Pyšek et al., 2017) and also for cosmopolitan species’ responses to environmental change. Moreover, our review identifies and resolves knowledge gaps to further elucidate plant responses to global change.
Intraspecific Variation
Although P. australis is classified as one species, it is comprised of three main phylogeographic groups. These can be identified by their chloroplast DNA sequences (Lambertini et al., 2012c) and include: (i) the North American group, which contains Phragmites australis subsp. americanus (hereafter NAnat; Saltonstall, 2002), (ii) the East Asian/Australian group, and (iii) the Northern Hemisphere/African group (Figure 1). Phragmites australis of the latter region is known as European Phragmites (sensu Lambertini et al., 2012c) and is poised to benefit the most from global change. It has recently enlarged its geographic range via two invasive lineages. European Phragmites includes the lineages “EU” in temperate Europe and elsewhere, “Med” in the Mediterranean region of Europe and north and south Africa (Lambertini et al., 2012c; Guo et al., 2013), and their introduced lineages in North America. The introduced lineages are known as “Haplotype M” (hereafter NAint M), which occurs across the North American continent in sympatry with NAnat, and the “Delta-type” (NAint Delta), which occurs in the Mississippi River Delta and in isolated populations in Florida (Lambertini et al., 2012b). Populations of the invasive lineages are genetically and ecophysiologically distinct from their native populations in Europe (Saltonstall, 2002; Lambertini et al., 2012b,c; Tho et al., 2016). They are reported in the literature under these specific names, which is why they are referred to here as NAint M and NAint Delta. European Phragmites also occurs across the continents of Africa and Asia in sympatry with other Phragmites species and P. australis lineages of the East Asian/Australian phylogeographic group in East Asia. The ranges of the P. australis East Asian/Australian and North American groups have been more stable than the range of European Phragmites. However, this pattern might reflect isolation or a lower research effort rather than these genotypes having lower fitness to establish in new ranges. More lineages have been found outside of the three groups, but these are not well-described and consist of scattered observations, or are Phragmites species other than P. australis (Figure 1). In the absence of an updated revised systematics reflecting the genetic structure of the species, we use the above names to refer to the above described lineages and phylogeographic groups of P. australis.
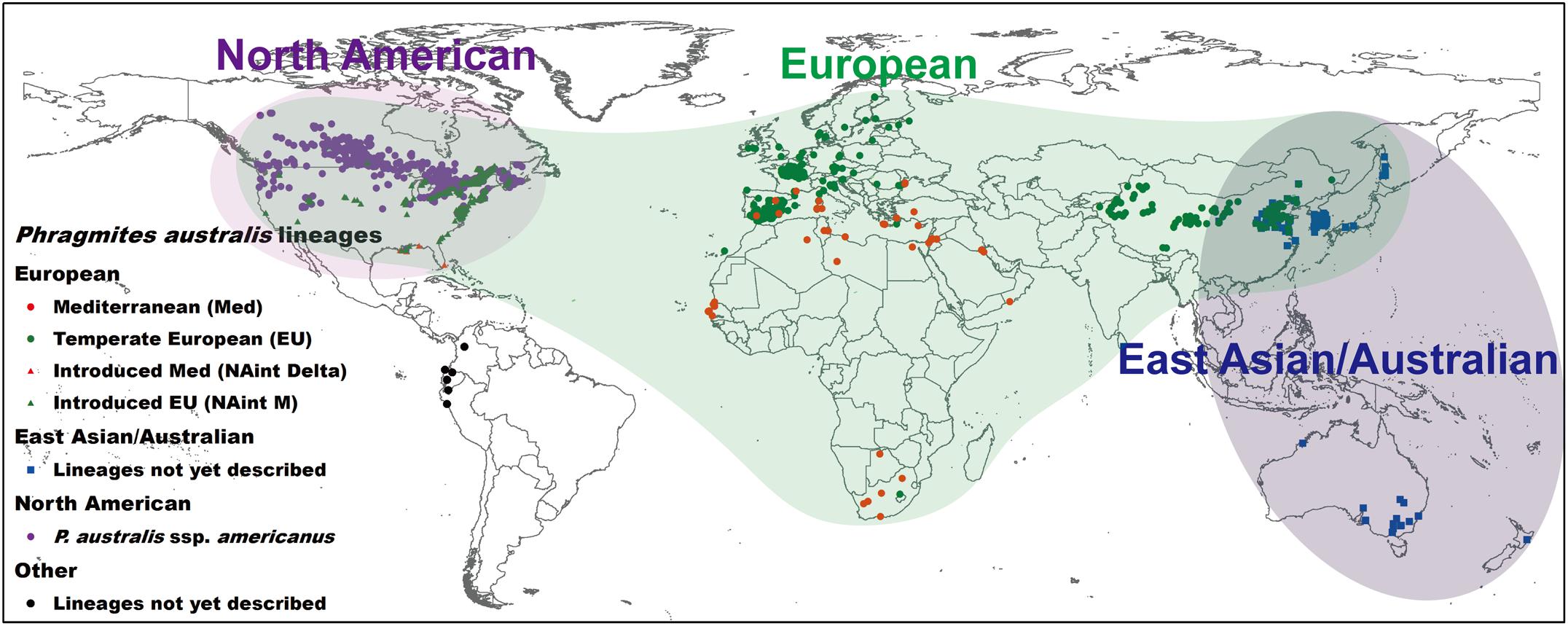
FIGURE 1. Global distribution of three main phylogeographic groups (North American, European, and East Asian/Australian) of the cosmopolitan wetland grass Phragmites australis, including several distinct lineages within the groups. More lineages or groups could possibly exist but have not been described yet. Points represent the collection locations of herbarium specimens analyzed by Lambertini et al. (2012c) and Guo et al. (2013) as well as the collection locations of several additional specimens at the Aarhus University herbarium.
Phragmites australis lineages and genotypes can be very diverse within and among populations, and genes from relatives in other phylogeographic regions or species can become incorporated into populations. This is due to a combination of inter- and intraspecific hybridization (McCormick et al., 2010a; Meyerson et al., 2010b; Chu et al., 2011; Paul et al., 2011; Lambertini et al., 2012b,c; Saltonstall et al., 2014; Saltonstall and Lambert, 2015; Wu et al., 2015), polyploidy (Clevering and Lissner, 1999; Meyerson et al., 2016a), genome size variability (Suda et al., 2015; Meyerson et al., 2016a), heteroplasmy (Lambertini, 2016), and long-distance dispersal.
Influences of Environmental Gradients and Phenotypic Plasticity on P. australis Phenotypic Diversity
The phenotypic diversity of globally dispersed species derives from adaptations to environmental factors such as climate or day length; phenotypes are therefore expected to vary over broad latitudinal ranges (Wilson, 1988; Coomes and Grubb, 2000; Poorter et al., 2009). Differences among distinct lineages of P. australis reflect adaptations to the environment of their geographic origin and include differences in plant traits, the degree of phenotypic plasticity, and the environmental drivers to which these traits respond (Eller and Brix, 2012; Mozdzer and Megonigal, 2012; Eller et al., 2013; Mozdzer et al., 2013, 2016a,b; Bhattarai et al., 2017a).
Phenotypic differences within P. australis are apparent along clines within lineages and phylogeographic groups (Bastlová et al., 2006; Reich and Oleksyn, 2008; Cronin et al., 2015; Mozdzer et al., 2016a; Allen et al., 2017; Bhattarai et al., 2017a). A general observation is that shoots increase in height with decreasing latitude and altitude (Haslam, 1973; Clevering et al., 2001; Hansen et al., 2007; Mozdzer et al., 2016a), but these trends are non-linear across broad latitudinal ranges (Mozdzer et al., 2016a). In the Mediterranean region P. australis can reach heights of up to 5 m, while temperate European Phragmites usually has stem heights of 2–3.5 m (Haslam, 1972; Eid et al., 2010; Packer et al., 2017b). European Phragmites populations from lower latitudes allocate relatively little biomass to leaves and more to stems; they also produce fewer shoots than populations originating from higher latitudes (Hansen et al., 2007; Eller and Brix, 2012). Also, northern populations have an earlier onset of flowering, a shorter growing season, and greater resistance to winter frosts, which is even more pronounced in populations from continental climates (Clevering et al., 2001; Bastlová et al., 2006; Lambertini et al., 2012c). On the local scale, water availability and soil properties such as salinity are important controls of P. australis morphology and biomass; this derives from the high phenotypic plasticity of the species (Vretare et al., 2001; Achenbach et al., 2013; Hughes et al., 2016; Mozdzer et al., 2016a). Plastic and genetically determined differences in P. australis below-ground structures yield considerable differences in seasonal shoot initiation, root organic acid content, rhizome construction costs, and rhizospheric microbial communities (Dykyjová et al., 1970; Moore et al., 2012; Zhai, 2013; Caplan et al., 2014).
Several ploidy levels have been identified in P. australis genotypes, specifically 2n = 3×, 4×, 6×, 8×, 10×, 12× (Gorenflot et al., 1983). Higher ploidy levels often result in larger plants (Stebbins, 1971, but see Meyerson et al., 2016b). However, only the octoploids from Romania, belonging to European Phragmites, have been found to have giant traits compared to the other ploidy levels (Hansen et al., 2007; Achenbach et al., 2012). In the Danube Delta, the octoploids have bigger leaves, are taller, and have thicker shoots than the tetraploids (Rodewald-Rudescu, 1974; Hanganu et al., 1999; Pauca-Comanescu et al., 1999; Clevering et al., 2001). However, gas exchange rates are not affected by differences in ploidy level (Hansen et al., 2007; Saltonstall and Stevenson, 2007), and neither are salt tolerance or a range of growth and ecophysiological traits (Achenbach et al., 2012, 2013). This suggests that ploidy level has a minor or still poorly understood role in determining phenotypic characteristics within the species, particularly when it interacts with genome size (Meyerson et al., 2016a).
Intraspecific Diversity Determines Responses to Global Change Drivers – The CRC (Cause-Response-Consequence)-Model
Dominant and invasive species can modify community traits and ecosystem processes (e.g., species richness or primary productivity), thereby affecting regional and biogeographic patterns of species distribution and interactions (Wright and Jones, 2004; Vilà et al., 2011; Pyšek et al., 2012; Hughes et al., 2016). High genetic diversity provides P. australis with a broad ecological amplitude, which may be especially important when it colonizes new habitat or faces environmental stresses (van der Putten, 1997; Clevering, 1999; Koppitz, 1999). The capacity of P. australis to acclimate and eventually adapt to environmental change depends not only on the degree and nature of the change, but also on the genetic composition of the lineage itself (Hiesey et al., 1942; Eller and Brix, 2012). A lineage can be described as an entity consisting of several genetically distinct genotypes, each of which shares a part of the genome with the genotypes of the same lineage, but is also comprised of different genes and phenotypic plasticity toward various environmental drivers (Bradshaw, 1965). Phenotypic plasticity is a genetically determined trait-set, and recent studies have shown that plastic responses are inheritable and determined by the climatic origin of a plant (Latzel and Klimesova, 2010; Münzbergová and Hadincová, 2017). The sum of all plastic responses of a geographic population within a lineage is determined by genotypes responding to a specific environmental factor (Figure 2). Genotypes with high or medium plasticity toward a specific driver of environmental change will be able to acclimate to that driver, meaning that they will thrive equally well before vs. after the change. Hence, a population consisting of mainly highly or moderately plastic genotypes will change in genetic composition and the resulting population will consist of genotypes able to thrive under the changed conditions. Genotypes with low plasticity toward that specific driver will be subject to local extinction or a range shift if a more suitable habitat without the change is accessible for establishment (Figure 2). Global drivers of spatially homogeneous impact, such as the concentration of atmospheric CO2, therefore pose a greater challenge than patchy changes such as soil salinity. Some P. australis lineages show predictable responses to climatic and environmental scenarios, and are therefore particularly suitable models for understanding and predicting adaptation processes and evolutionary dynamics in other plants and plant types. We describe below the ecophysiological responses to global change drivers and present a conceptual model (Figure 3) that predicts how each Phragmites lineage will evolve by acclimation and adaptation to the drivers. Some reed lineages have not been described well enough in the literature to be included in the model, such as NAint Delta and the Far East/Australian (FEAU) group. The FEAU group is likely to be a suitable model for highly productive species like tropical grasses, but needs further investigation, especially with respect to phenotypic plasticity.
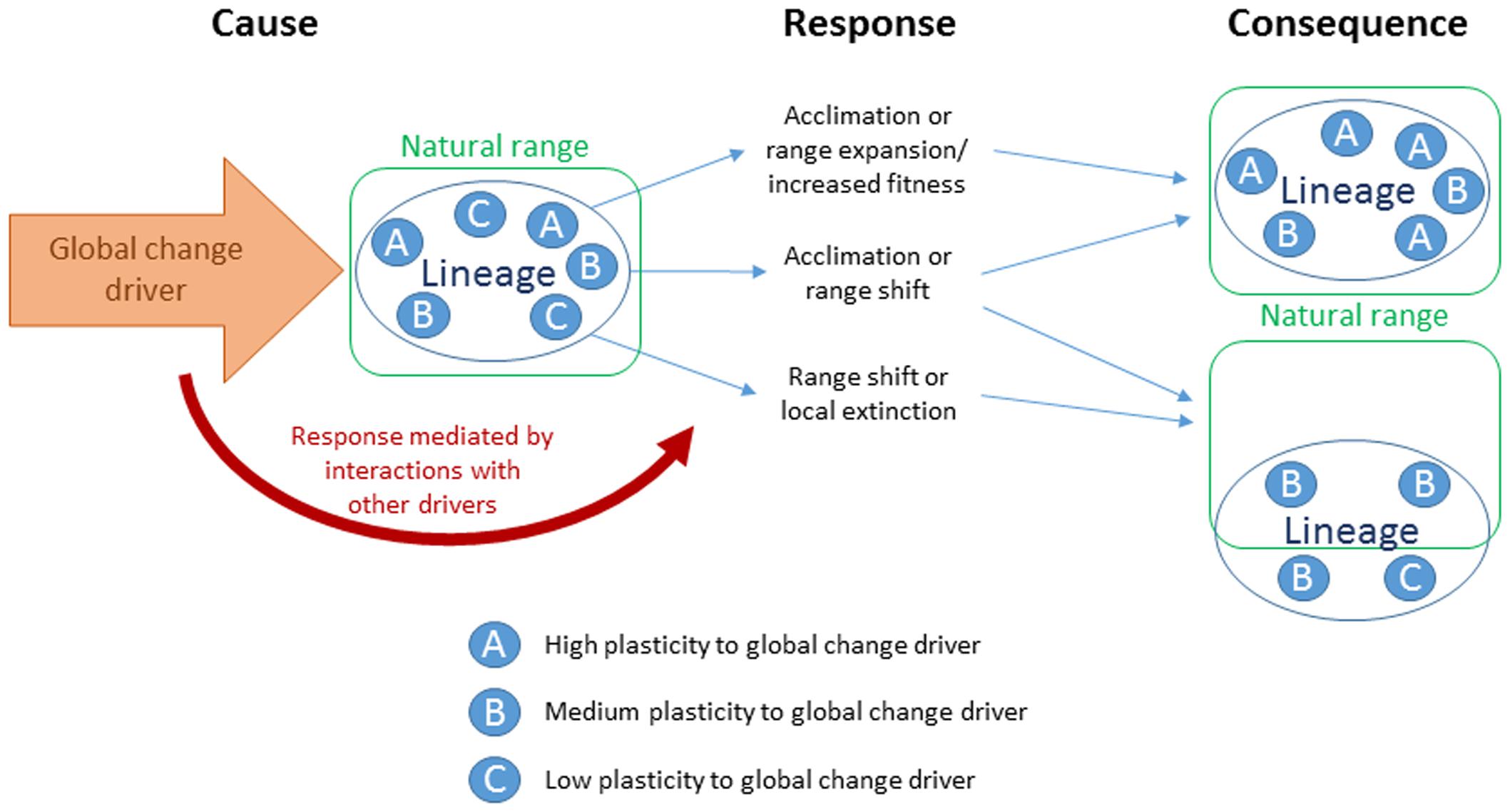
FIGURE 2. CRC (cause-response-consequence) model of global change driver acting upon lineages (or geographic populations within a lineage) composed of different genotypes. A global change driver affects the lineage which consists of highly plastic genotypes with respect to the driver (A), moderate plasticity with respect to the driver (B), and low plasticity with respect to the driver (C). Plasticity refers to phenotypic plasticity in fitness-related traits (reproduction and productivity), thus affecting the genotype’s acclimation and adaptation capacity. The genotypes respond differently to the driver depending on their phenotypic plasticity; likely responses are acclimation, increased fitness, range expansion, range shift, or local extinction. Acclimation is the response to the environmental driver that results in similar or increased fitness. This scenario will likely lead to range expansion. A range shift occurs from the natural range of occurrence, which is the current distribution range including the native range for native lineages and the presently invaded range for introduced lineages. The responses can be mediated by interacting environmental drivers. The ultimate consequence of the responses to the effect are impoverished genetic diversity, including lineages with lower phenotypic plasticity and fewer, but better adapted genotypes, or a lineage shifting into a new range less or differently affected by the global change driver.
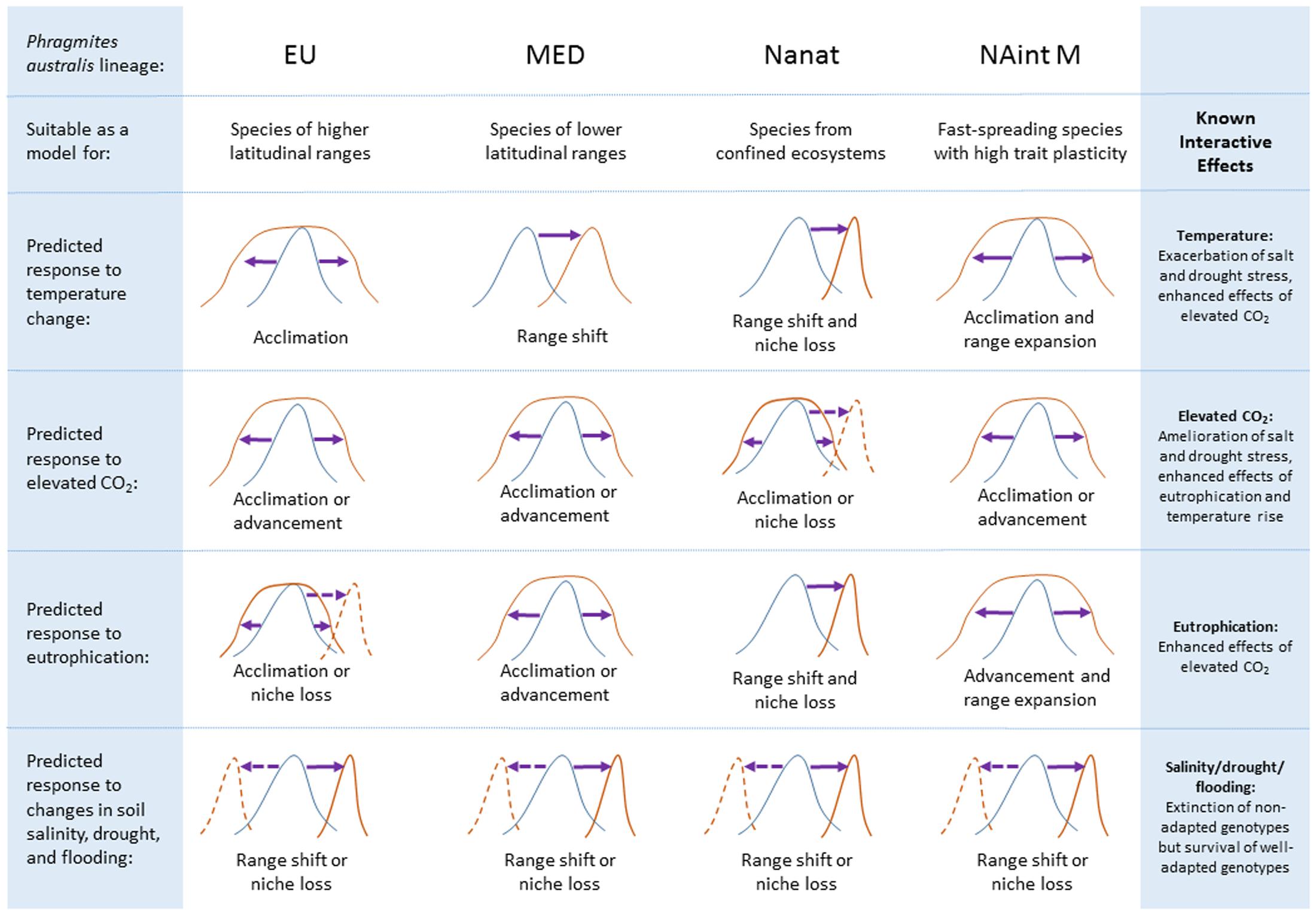
FIGURE 3. Specific effects of global change drivers on reed lineages. Lineage response is averaged, based on studies conducted on several genotypes from within these lineages. Well-established interactions with other global change drivers are specified. Curves show ecophysiological amplitude with specific niche-breadth and response strength to changes. Each lineage response can be extrapolated to different species with similar ecophysiological characteristics. Curves outline a relative normal distribution of fitness-related parameters of the population. A narrower curve means a narrower niche-breadth with respect to a global change factor (on x-axis). Advancement here means increased fitness. Blue curves show the current stage while orange curves result from the action of the specific global change factors. Either solid or dashed curve are expected to appear, but not both simultaneously.
The conceptual model presented in Figure 3 is based on the responses of P. australis lineages to factors associated with global change that act upon a lineage individually or in combination (Table 1). Overall, EU and NAint M are the lineages best adapted to withstand temperature changes and, together with the MED lineage, elevated CO2, while MED and NAint M will respond most positively to eutrophication (Figure 3). NAnat is the lineage with the least acclimation capacity. However, interactions with other environmental factors may change the above predictions (Figure 3). In the following sections, we review the main ecophysiological processes of P. australis to illustrate the diversity of these processes as a function of intraspecific variation and phenotypic plasticity, as well as the breadth of ecological niches that the species inhabits. We further describe ecophysiological responses to environmental factors to which the species is commonly exposed: temperature, atmospheric CO2 concentration, salinity, flooding, drought, and eutrophication. All of these factors are currently changing and are expected to change further in upcoming decades (IPCC, 2014). We also show how and why P. australis’ responses to global change can be extrapolated to predict those of other species.
Key Ecophysiological Processes
Gas Exchange
Like biomass production and morphology, gas exchange-related traits in P. australis are highly plastic. Within a phylogeographic region, the prevailing climatic conditions have the strongest effects on gas exchange rates (Lessmann et al., 2001; Hansen et al., 2007; Mozdzer et al., 2016a). Although the climate of the area of origin strongly affects physiological responses, there are also phylogeographic differences in potential responses to environmental change. For example, the NAint Delta lineage was less plastic in its ability to modify gas exchange parameters compared to the highly plastic NAint M lineage when grown across 14° of latitude (Mozdzer et al., 2016a). Furthermore, tropical and subtropical populations of P. australis have a higher photosynthetic capacity and photosynthetic pigment concentration than populations in the temperate zone (Nguyen et al., 2013). Similarly, NAint P. australis has a higher photosynthetic capacity and pigment concentrations than NAnat (Mozdzer and Zieman, 2010; Guo et al., 2014). Nguyen et al. (2013) proposed the existence of a diversified C3 pathway within P. australis that is modified to maintain high enzymatic efficiencies in tropical and Mediterranean climates but can be down-regulated to accommodate the lower temperature and irradiance of temperate regions.
Despite the typical C3-photosynthetic features displayed by P. australis, C4-like strategies have also been observed. A prominent sheath layer that is especially pronounced in young P. australis leaves surrounds the vascular bundles in the mesophyll, resembling the foliar Kranz anatomy of C4 plants (Henriques and Webb, 1989). However, due to the lack of chloroplasts in this layer, there is no functional correlation with true C4 plants (Henriques and Webb, 1989). Doubts about the photosynthetic pathway of P. australis have also emerged due to relatively high PEPcase activities, higher activities of the decarboxylating NADP-dependent malic enzyme (NADP-ME), and a possible C3–C4 intermediate pathway associated with ecotypes from arid or salt-affected habitats (Rintamaki and Aro, 1985; Zheng et al., 2000; Zhu et al., 2012). Most of the known C4 species occur in the Poaceae, in which C4-evolution has occurred independently several times and, thus, genes are present in P. australis that can rapidly develop C4 functions including the gene coding for NADP-ME (Christin et al., 2009).
Nevertheless, P. australis has, in most studies, been shown to possess characteristics typical of C3 plants, including a high Rubisco/PEPcarboxylase ratio, high photorespiration rates, and a high CO2 compensation point (Antonielli et al., 2002; Hansen et al., 2007; Eller and Brix, 2012). The photosynthetic pathway of P. australis therefore remains unresolved, as the range of the abovementioned studies suggests that the photosynthetic pathway may vary within the species. The distinct bundle sheath cells in P. australis leaves also raise the possibility of C2 photosynthesis, which is the evolutionary bridge between C3 and C4 photosynthesis (Sage, 2016); however, evidence of this possibility has yet to be found.
Nutrient Acquisition
By far the greatest number of scientific studies on P. australis have been concerned with the species’ tremendous potential for nutrient removal, which makes it an ideal candidate species for wastewater treatment in constructed wetlands (e.g., Brix and Schierup, 1989; Brix, 1997; Bragato et al., 2006; Vymazal, 2013; Hernández-Crespo et al., 2016). Genetically determined differences in nutrient uptake and assimilation capacity result in distinct reed ecotypes with differences in productivity (Tho et al., 2016). Some ecotypes sustain high nutrient assimilation rates and high allocation to aboveground biomass, while others have high nutrient translocation rates to rhizomes for storage and thus high belowground biomass allocation (Kühl et al., 1997; Tripathee and Schäfer, 2014). Reed genotypes with dissimilar nutrient demands and productivity can thus grow at similar nutrient levels in naturally adjacent stands. Such distinct ecophysiological strategies confer greater population plasticity and performance to a genetically diverse stand compared to a monoclonal stand (Rolletschek et al., 1999). Pronounced differences in the nitrate uptake kinetics of distinct reed genotypes are possibly caused by distinct transcript abundances of nitrate transporter genes, and a likely reason for the genotypic differences in nutrient acquisition strategies (Araki et al., 2005). In general, P. australis is well-adapted for growth in nutrient-rich habitats (Mozdzer et al., 2010; Caplan et al., 2015) but can also acclimate to low nutrient availability by increasing the affinity for ammonium uptake (Romero et al., 1999; Tylova-Munzarova et al., 2005; Mozdzer and Megonigal, 2012).
Gas Transport and Ventilation
Like almost all plants that can grow vigorously in habitats where soil saturation and flooding are common (Vartapetian and Jackson, 1997), P. australis aerates flooded tissues by transporting oxygen through a well-developed network of internal airspaces, or aerenchyma (Armstrong and Armstrong, 1991; Jackson and Armstrong, 1999). These internal airspaces are continuous from the leaf sheaths and culms, through the rhizomes, and into the root cortex, where aerenchyma are particularly well-developed through lysigeny (Armstrong et al., 1996a; White and Ganf, 2002). Rhizomes are segmented internally and have secondary aeration channels in the internode cortex, such that airflow is maintained even if rhizome cavities become damaged and filled with water (Soukup et al., 2000). More efficient root aeration also allows for greater respiration rates and, thus, sustained nutrient uptake capacity and root development, even in hypoxic soils (Nakamura et al., 2013).
Phragmites australis is also one of the few wetland species that does not rely solely on simple diffusion for gas transport; it supplements its aeration with convective gas flow (Brix, 1989; Brix et al., 1992, 1996; Armstrong et al., 1996a). Convection is induced by humidity gradients generated in lacunae (i.e., sub-stomatal cavities) in leaf sheaths of live culms (Armstrong et al., 1996a,c). The pressure that builds up in lacunae pushes air down through live culms and rhizomes; air is vented out of the plant through damaged or dead culms (Brix, 1989; Armstrong et al., 1996c; Afreen et al., 2007).
Little attention has been paid to potential intraspecific differences in gas transport among P. australis lineages. Tulbure et al. (2012) showed that the ventilation efficiency of the invasive NAint M lineage in North America was 300 times higher than that of native P. australis subsp. americanus, when differences in stem densities between lineages were accounted for. Since gas flux is a physically determined process and is strongly affected by internal anatomy (Rolletschek et al., 1999), different gas flow behavior can be expected in plants with genotype-specific morphological characteristics. Moreover, gas flow characteristics of wetland plants affect not only oxygen transport but also plant-mediated methane emission (Brix et al., 2001), and lineage-specific differences in factors controlling gas flow are known to affect methane fluxes (Armstrong et al., 1996b; Kim et al., 1998). For example, NAint M roots more deeply than other lineages and, through changes in soil organic matter dynamics, can lead to increased rates of CO2 losses to the atmosphere (Bernal et al., 2017). Differences in gas flow capacity and rhizosphere oxygenation among lineages are therefore very likely and deserve greater attention.
Effects of Major Drivers of Global Change on the Performance of P. australis
Contrasting responses to global change drivers have been reported in North American and European Phragmites. Phragmites australis of Asia and Australia has received limited attention, so their responses to such drivers remain poorly understood. From the 1970s to the 1990s, P. australis in Europe experienced a decrease in abundance termed ‘reed dieback,’ largely due to anthropogenic eutrophication and deeper flooding, especially in Eastern Europe (Ostendorp, 1989; van der Putten, 1997; Brix, 1999b). Increased salinity caused by land use changes may also have contributed to reed dieback in northern European brackish marshes, as it may have allowed halophytes like Spartina alterniflora to displace less salt-tolerant species like P. australis (Vasquez et al., 2006). Reductions in P. australis growth have also been associated with litter accumulation leading to the production of phytotoxins (Armstrong et al., 1996a; Čížková et al., 1999) and high rates of anaerobic mineralization stemming from excess organic matter and the associated increase in biological oxygen demand (Sorrell et al., 1997). Degraded reed stands have been shown to have an altered C/N metabolism due to higher rates of photorespiration and thus, lower carbon fixation (Erdei et al., 2001).
In contrast to the situation in Europe, the species has shown invasive behavior in North America over the last 50 years. The invasion is driven by a few lineages originating from European Phragmites (Hauber et al., 1991; Saltonstall, 2002; Hauber et al., 2011; Lambertini et al., 2012b) and may depend largely on the high genetic diversity of the species in its native range (Saltonstall, 2003; McCormick et al., 2010b; Pyšek et al., 2017).
Temperature Effects
Without considerable greenhouse gas reductions, the global rise in mean surface temperature of Earth is very likely to exceed 1.5–4°C by the end of the 21st century, with the greatest increases in the Northern Hemisphere (IPCC, 2014). Heatwaves and extreme precipitation events are expected to occur more frequently and with longer durations in many regions, but occasional cold temperature extremes can also be expected (IPCC, 2014).
Phragmites australis exhibits lineage-specific responses to temperature regimes in terms of morphology, growth, and to a certain extent, photosynthetic traits (Table 1; Clevering et al., 2001; Lessmann et al., 2001; Eller and Brix, 2012; Eller et al., 2013; Mozdzer et al., 2016a). Rates of P. australis growth (especially shoot height and length), as well as rates of transpiration and photosynthesis, are generally greater at lower latitudes due to warmer temperature regimes and longer day lengths (Haslam, 1975; Lissner et al., 1999a,b; Zemlin et al., 2000; Lessmann et al., 2001; Karunaratne et al., 2003; Mozdzer et al., 2016a). Reciprocal transplant experiments in common gardens have shown that, for lineages originating from lower latitudes, higher temperatures are needed to initiate growth and, after being transplanted to higher latitudes, panicles either emerge late or do not flower at all (Brix, 1999b; Clevering et al., 2001; Karunaratne et al., 2003; Lambertini et al., 2012c). Adaptation to the climate in the region of origin significantly affects plant species’ performance and plasticity (Franks et al., 2014; Molina-Montenegro et al., 2016; Allen et al., 2017; Bhattarai et al., 2017a,b; Münzbergová et al., 2017). Hence, P. australis belonging to the MED lineage can be a model for Mediterranean, subtropical, and even tropical plant species, while populations of the EU lineage can be a model for temperate species found at higher latitudes (Figure 3).
Some lineages seem to be more plastic to changes in temperature than others, as they show a large acclimation capacity to both increases and decreases in temperature (Figure 3; Lessmann et al., 2001; Eller and Brix, 2012). This is the case for NAint M in North America, for example, where temperature fluctuations have been shown to enhance its distribution (Guo et al., 2013). The North American invasion is therefore likely to accelerate with climate change. It has previously been suggested that lineages originating in areas with high fluctuating temperatures also have higher plasticity to temperature changes, and may therefore be better adapted to withstand climatic changes (Molina-Montenegro and Naya, 2012). The same has been shown for P. australis lineages; EU genotypes from higher latitudes in temperate areas have generally shown higher plasticity toward differences in growth temperature (Lessmann et al., 2001; Eller and Brix, 2012; Nguyen et al., 2013). It can be assumed that the high plasticity of NAint M derives from its origin in the highly plastic EU populations, emphasizing the potential model role of P. australis lineages from high-latitudes for temperate plant responses to temperature differences (Figure 3).
Other lineages appear to be pre-adapted to predicted future temperature regimes and are therefore likely to extend their range northward (Clevering et al., 2001; Lessmann et al., 2001; Eller et al., 2014a,b; Mozdzer et al., 2016a). It is possible that lineages originating from lower latitudes may expand their distributions northward in the warming world (Figures 2, 3; Guo et al., 2013; Mozdzer et al., 2016a), as frost and cool temperatures limit growth or sexual reproduction at mid and high-latitudes (Mozdzer et al., 2016a). Also, the expansion of the invasive NAint Delta lineage can be attributed, in part, to warmer temperatures in its invasive range than in its native range (Guo et al., 2013), as advancement of a population can be expected if a high phenotypic plasticity to temperatures is inherent (Figure 2). Alternatively, lower-latitudinal lineages may be unable to cope with the rapidity of temperature changes due to a narrow niche-breadth or acclimation-capacity, and may become genetically diminished (Figure 2). Using P. australis as model for global warming, a two-way scenario can be expected as the species responds to temperature increases. On the one hand, species with high phenotypic plasticity, and therefore greater niche breadths, will likely be able to cope with warming and thrive equally well or even extend their range northward. Another species in which this is likey to occur is Nothofagus pumilio (Mathiasen and Premoli, 2016). On the other hand, species with limited plasticity and narrower niche breadths may fail to acclimate, facing local extinction in the worst case (Figure 2). Some herbaceous alpine species occurring at high elevation provide a good example of narrow niche breadth leading to local extinction (Schmid et al., 2017). Indirect changes and interactions of temperature with other abiotic factors, such as increased drought and salinity, may impose additional challenges on P. australis populations in already warm areas, possibly resulting in more favorable growth conditions at higher latitudes (Figure 3; Brix, 1999b; Eller et al., 2014a).
CO2 Effects
Atmospheric CO2-equivalents are likely to exceed 720 ppm, and possibly reach 1000 ppm, by the late 21st century if greenhouse gas emissions are not restricted substantially (IPCC, 2014). As a C3 plant, P. australis will benefit from rising atmospheric CO2 concentrations, but there is growing evidence that the magnitude of its response may be lineage-specific due to differences in phenotypic and physiological plasticity (Figure 3). For example, in an experiment in which CO2 was elevated to ∼700 ppm, both the NAint M and NAnat lineage responded positively to CO2 elevation but the NAint M lineage had considerably greater plasticity in nearly every trait measured (Mozdzer and Megonigal, 2012; Caplan et al., 2014). In contrast, several studies focusing on other lineages of P. australis have found no significant effects of elevated CO2 on biomass or morphological parameters, though some photosynthetic enhancements have been reported (Scholefield et al., 2004; Milla et al., 2006; Kim and Kang, 2008; Eller et al., 2013). We note that these studies either did not measure below-ground biomass productivity or did not account for respiration rates, which may partly explain the lack of biomass stimulation by elevated CO2.
Based on the above, differential responses to elevated CO2 may result in lineage-specific shifts, increases in competitiveness and distribution changes. However, interactions with other abiotic factors such as salinity and nutrients make it more difficult to predict the effects of elevated CO2 in natural environments. For example, whilst shoot elongation rates are enhanced by elevated CO2 and temperature to similar degrees in both the invasive NAint Delta and the invasive NAint M lineages, the NAint Delta lineage outperforms the NAint M lineage when grown at 20‰ soil salinity (Eller et al., 2014a). The stronger growth response of NAint Delta is facilitated, in large part, by intrinsically greater photosynthetic rates (Table 1; Nguyen et al., 2013; Eller et al., 2014a). Overall, the strongest effects on growth and carbon assimilation rates are expected to result from changes in CO2 that are accompanied by increases in temperature or nutrient enrichment, especially nitrogen (N) (Figures 2, 3; Mozdzer and Megonigal, 2012; Eller et al., 2013, 2014a,b; Caplan et al., 2015), which has been shown for other C3 species (Ainsworth and Rogers, 2007).
Changes induced by elevated atmospheric CO2 concentrations may influence ecosystem services in P. australis dominated wetlands. For example, elevated CO2 increases the methane emission rate of both NAnat and NAint lineages (Mozdzer and Megonigal, 2013), which may offset the net carbon fixation of P. australis wetlands that would otherwise be greenhouse gas sinks (Brix et al., 2001). Moreover, elevated CO2 induces greater belowground productivity and rooting depths in the NAint M lineage (Mozdzer et al., 2016b), which are likely to increase rates of belowground biomass accumulation and surface elevation gain. Such effects could enhance the ability of P. australis dominated wetlands to keep pace with sea level rise (Rooth et al., 2003; Caplan et al., 2015; Mozdzer et al., 2016b). Responses to elevated CO2 have predominantly been investigated through short-term studies and have only investigated a few P. australis lineages (including NAnat, NAint M, NAint Delta, EU, and Med; Eller et al., 2013; Mozdzer and Megonigal, 2013; Eller et al., 2014a,b); more research is needed to determine if enhancement of growth and methane emission rates apply to the whole species. Due to its high plasticity to atmospheric CO2 concentration, the NAint M lineage can be used as a model for studying the responses of invasive C3 species to elevated CO2 (Figure 3), as high phenotypic plasticity is a common trait in invasive species (Drenovsky et al., 2012; Gioria and Osborne, 2014; Colautti et al., 2017).
Salinity Effects
Saltwater intrusion due to global sea level rise is becoming a major issue in both brackish saltmarshes and tidal freshwater wetlands (Beckett et al., 2016). Moreover, regions with high salinity and high evaporation rates are likely to become more saline, while regions of low salinity and high precipitation will become fresher, inducing greater extremes in salinity in wetlands globally (IPCC, 2014). Finally, some climate change models predict an increase in the intensity and frequency of tropical storms and hurricanes (e.g., Bender et al., 2010; Knutson et al., 2010), which may lead to flooding and salt intrusion in near-coastal habitats. Hence, soil salinity regimes are shifting such that salinity tolerance is of increasing importance to biotic communities in coastal ecosystems.
The ecological amplitude of P. australis extends from freshwater to saline tidal wetlands, with plants persisting at salinities as high as 65‰ (recorded in Delaware, eastern United States; Engloner, 2009), with tolerances of 22.5‰ (Lissner and Schierup, 1997) to 35‰ salinity reported for juveniles (Engloner, 2009) and a limit of 30‰ reported for seed germination (Yu et al., 2012). The mechanisms of salt tolerance in P. australis include Na+ exclusion or vacuolar compartmentalization, tissue dehydration or compatible osmotic solute accumulation, and increased gene expression of oxidative stress response enzymes (Matoh et al., 1988; Lissner and Schierup, 1997; Lissner et al., 1999a; Pagter et al., 2005; Vasquez et al., 2005; Achenbach and Brix, 2013; Achenbach et al., 2013; Eller et al., 2014b). Certain ecotypes of P. australis are more salt tolerant than others (Table 1), with higher salinities yielding greater germination rates and better developed root systems (Rechav, 1967; Van der Toorn, 1972). Several controlled experimental studies have shown that NAint Delta has higher salt tolerance than NAint M, though both perform better at higher salinities than Med or the NAnat lineages (Achenbach and Brix, 2013; Eller et al., 2014a). Since the NAnat lineage has considerably greater N uptake rates than the invasive NAint M lineage at salt concentrations up to 20‰ (Mozdzer et al., 2010), it is primarily limited to oligohaline and mesohaline wetlands in the mid-Atlantic United States (Vasquez et al., 2005; Packett and Chambers, 2006; Mozdzer et al., 2010). However, in other regions of the United States, like New England and the Midwest, native North American populations are not limited by salinity and occur in brackish river systems as well as in saltmarshes (e.g., Burdick et al., 2001; Kettenring and Whigham, 2009; Kettenring and Mock, 2012; Cronin et al., 2015). These results indicate that salt tolerance is genotype-specific rather than lineage-specific, and highly variable within the species. Plant responses to changes in soil salinity can therefore be elucidated by studying locally adapted genotypes rather than lineages; the genetic composition of a population exposed to changing salinity regimes will be altered according to its pre-adaptation for salt tolerance (Figure 2).
Locally adapted genotypes may, however, not be the only strategy P. australis employs to persist in saline environments. Salt avoidance by below-ground labor division may also play a significant role in acclimation to shifts in salinity regime, as has also been found in the clonal species Schoenoplectus americanus in a brackish tidal wetland (Ikegami et al., 2008). An important component of P. australis’ ability to grow in soils spanning a wide range of salinities is its extensive rhizome and root system. Thus, most of the belowground biomass of lineages occurring in North America has been found in the upper 70 cm of soil (Moore et al., 2012), though depths can exceed 3 m even at coastal sites (Mozdzer et al., 2016b). This morphology may grant the species access to freshwater resources at soil depths less affected by tides. The importance of belowground organs to salt tolerance has also been demonstrated in Asia in landscapes with patchy soil salinity, where the genetic variation of P. australis is closely correlated with habitat heterogeneity (Gao et al., 2012).
Despite being able to survive and grow in saline soil conditions, P. australis has historically been considered a fresh to brackish water species (Raunkiaer, 1893; Haslam, 1973; Matoh et al., 1988). Several studies have identified negative effects of greater salinity levels on various traits including biomass production, culm height, stand density, culm diameter, and rhizome carbohydrate content (Lissner et al., 1999a; Engloner, 2009; Achenbach et al., 2013; Tang et al., 2013; Eller et al., 2014a). Physiologically, P. australis responses to high salinity are associated with decreases in tissue water potential, stomatal conductance and transpiration rates, photosynthetic efficiency of PSII, and nitrogen uptake rates (Chambers et al., 1998; Lissner et al., 1999b; Naumann et al., 2007; Pagter et al., 2009; Mozdzer et al., 2010; Zhang and Deng, 2012). The photosynthetic recovery and re-opening of stomata after short-term exposure to high salinity differs between genotypes of different lineages, demonstrating that sensing and responding to osmotic stress is a genotype-specific feature (Achenbach and Brix, 2014). Also, high-affinity K+ transporters isolated from salt tolerant reed plants are more efficient in K+ uptake and less permeable to Na+ than transporters from salt-sensitive plants, offering an explanation for their difference in salt-sensitivity (Takahashi et al., 2007).
Salinity increases in freshwater wetlands are likely to affect the natural distribution of P. australis genotypes and to alter the competitive dynamics between less and more salt-resistant plants. Spread of P. australis into salt marshes might also be accelerated in El Niño years due to temporary decreases in salinity from heavy rains that open windows for seedling establishment (Minchinton, 2002) and also due to the expansion of patches that maintain access to less saline groundwater in other parts of the stand. Storm surge from tropical storms, cyclones, and hurricanes can flood near-coastal freshwater wetlands and greatly elevate salinity levels. In North America, the spread of invasive NAint M populations is strongly positively correlated with the frequency of these storms and it has been argued that NAint M lineage thrives because it is more salt tolerant than native wetland plants (Burdick and Konisky, 2003; Bhattarai and Cronin, 2014). Unlike climatic adaptations that can be attributed, in part, to the phylogeographic origins of P. australis lineages, salt tolerance cannot simply be ascribed to a specific phylogenetic background, but is rather a consequence of the single pre-adapted genotype (Gao et al., 2012; Achenbach et al., 2013). Locally adapted genotypes of different reed lineages may therefore serve as models for studying responses to changes in soil salinity. Depending on the lineage, however, the outcome of interactions with other global change drivers can be estimated. Overall, future changes in P. australis salt tolerance are very likely, as responses to salinity have been shown to interact with temperature and CO2, and may confer greater salt resistance on P. australis due to improved osmotic acclimation and higher assimilation rates (Lissner et al., 1999a; Eller et al., 2014a). A lineage with inherently high phenotypic plasticity, such as NAint M, can be expected to benefit more from interactive effects of salinity and elevated CO2 than NAnat.
More research is needed to determine which genetic factors underlie the high salt tolerance of genotypes within P. australis lineages, and how and why these factors arise in these genotypes. Due to their increased likelihood of including salt-resistant genotypes, populations and stands with high genetic variability will probably have the strongest prospects of adapting to changes in salinity. Moreover, individual stands of P. australis will likely face genetic impoverishment following the extinction of salt-sensitive genotypes under shifting soil salinity regimes (Figure 2).
Flooding Effects
Climate projections indicate that greater variability in precipitation will cause more frequent extremes in precipitation and discharge in many areas. This will increase the frequency and magnitude of inland and coastal floods, which will be compounded by larger storm surges and rising sea levels (IPCC, 2014).
Although P. australis seedlings are extremely vulnerable to flooding (Chambers et al., 2003; Mauchamp and Methy, 2004; Baldwin et al., 2010; Kettenring et al., 2015), once established, seedlings and adult plants are highly tolerant of inundation. Specifically, the survival, physiology, and growth of P. australis are less affected by submersion than are many other wetland plants (Gries et al., 1990; Brix et al., 1992; Armstrong et al., 1996b). Moreover, susceptibility to flooding decreases with ontogeny in the species (Bart and Hartman, 2003; Chambers et al., 2003; Whyte et al., 2008; Tulbure and Johnston, 2010). P. australis is also tolerant of greater amplitude fluctuations (±45 cm) in water level than other species, provided that its elevation is close to the mean water level (White et al., 2007). However, high water during extreme flooding years caused reed belts to decline along lakes in southern Germany and Austria; stands rejuvenated only in low-water years (Ostendorp, 1999; Ostendorp et al., 2003).
We found no studies that directly assessed genotypic differences in flooding tolerance. However, the number of genotypes represented in a lakeshore stand in Hungary decreased with water depth (Engloner and Major, 2011), which the authors attributed to genotype-specific flooding tolerance. Another study found that seasonal profiles of amino acids and carbohydrates differed by genotype and flooding regime in a German fen (Koppitz, 2004; Koppitz et al., 2004), though genotype by flooding regime interactions were not reported. These findings indicate that responses to flooding are a consequence of pre-adapted genotypes rather than adaptation at the lineage scale. Like soil salinity, plant responses to fluctuating water levels can best be studied in locally adapted genotypes, and the population response to be expected will be an alteration of its genetic composition (Figure 2).
Responses to flooding depend on the interaction of other drivers of global change in a way that is similar to soil salinity. For example, belowground, deeper water induces a shallower rhizome depth distribution (Weisner and Strand, 1996; Vretare et al., 2001; White and Ganf, 2002; Mozdzer et al., 2016b), but this rooting depth will deepen with rising CO2 concentrations (Mozdzer et al., 2016b). Vretare et al. (2001) suggested that P. australis increases allocation to stem height when growing in deeper water and simultaneously decreases stem density and belowground allocation. While stem density appears to be consistently lower in deeper water (Yamasaki and Tange, 1981; Vretare et al., 2001; Bodensteiner and Gabriel, 2003), stem height has been reported to both increase and decrease in response to deeper flooding (e.g., Hellings and Gallagher, 1992; Coops et al., 1996; Vretare et al., 2001; Engloner, 2004). Genotypic differences in the P. australis plants studied may contribute to the variation in outcomes from these studies, though this has not been assessed in the majority of cases. Facing more frequent flooding regimes with global change (IPCC, 2014), natural selection of flooding-resistant genotypes can be anticipated such that flooded populations may become genetically impoverished. Previous studies have often focused on the growth and morphological acclimation of P. australis to flooding, but there is a need to investigate the physiological consequences of inundation more thoroughly. Recent advances in research on photosynthesis in submerged shoots showed that elevated CO2 can alleviate flooding stress (Winkel et al., 2014). Although not investigated in P. australis, this capability would be especially relevant to determining seedling responses to inundation.
Drought Effects
Phragmites australis is well-adapted for life in flooded environments but is tolerant of the full range of wetland hydrological conditions, including drought (Pagter et al., 2005). Wetland hydrology can be highly variable, with relatively dry conditions being common or even extreme in times of drought (Mitsch and Gosselink, 2007). With climate change, drought is predicted to develop more quickly and increase in intensity in many regions of the world (IPCC, 2014; Trenberth et al., 2014). Phragmites australis deals with drought through both short-term tolerance mechanisms (i.e., by making physiological or biochemical adjustments) and longer-term avoidance strategies that affect morphological and developmental traits (Morgan, 1984; Chaves et al., 2002; Pagter et al., 2005; Touchette et al., 2007). Following extreme low-water conditions, reed stands employ a “guerilla strategy” to efficiently and quickly occupy new wet habitats; they produce tillers across the uninhabited littoral zone as well as “legehalme,” which are rapidly elongating, horizontal shoots from which new culms emerge at the nodes (Ostendorp and Dienst, 2012).
Under dry soil conditions (in situ), P. australis substantially decreases leaf osmotic potential and accumulates more soluble sugars, amino acids, protein metabolites, proline, and nutrient elements than under moist conditions (Elhaak et al., 1993). When subjected to mild water stress, P. australis reduces total leaf area and biomass, but severe water stress induces changes in osmolality, leaf proline concentration, leaf chlorophyll a content, stomatal conductance, and photosynthetic rates (Pagter et al., 2005). Similarly, terrestrial dryland ecotypes of P. australis from northwest China increase their capacity for osmotic adjustment, significantly decrease stomatal conductance, reduce net photosynthetic rate, and their cover and height declines (Cui et al., 2010). Compared to wetland ecotypes, they also exhibit greater water use efficiency, increased activity of C4 photosynthetic enzymes, protective down-regulation of photosynthetic enzyme activities, and greater antioxidant enzyme activity (contributing to oxidative stress protection; Wang et al., 1998; Zhu et al., 2001, 2003a,b; Gong et al., 2011; Xiang et al., 2012). With the onset of complete drought (in controlled experimental studies), P. australis showed signs of drought in leaf xylem pressure potentials by the second day, stomatal conductance and photosynthesis by days four to eight, and leaf rolling and wilting by day five (Saltmarsh et al., 2006; Naumann et al., 2007). Field-based phenological studies of P. australis in Great Britain showed that years with spring drought can induce later emergence and flowering, as well as shorter culms, compared to years with normally flooding patterns. Also, years with fall drought may lead to earlier senescence compared to years when the stand is flooded. Nonetheless, P. australis rhizomes can penetrate up to 2 m into the soil to access deeper groundwater (Haslam, 1970).
As with salinity tolerance, it is very likely that some P. australis genotypes are more drought tolerant than others, even within lineages (Figure 3). However, drought events are more tightly coupled to climate than are high salinity periods, with the implication that drought-resistance could be phylogeographically determined in the species. It remains to be determined if phylogenetically determined drought tolerant lineages are also salt tolerant. For example, it seems that the North American native lineage is more sensitive to drought in some regions, such as the southwestern United States, where it is often associated with small streams and springs, which are sensitive to small changes in water availability (Meyerson et al., 2010a; Kettenring and Mock, 2012; Kettenring et al., 2012). In contrast, short-term drought that leads to temporary drawdowns may benefit colonization of the NAint M lineage by fostering seedling recruitment (Alvarez et al., 2005; Tulbure et al., 2007; Whyte et al., 2008; Kettenring et al., 2015, 2016). These studies serve as examples for using P. australis as model to study, whether physiologically similar responses to different global change factors result from similar adaptations or are independent of the plants’ phylogeographic origin.
Eutrophication Effects
Increases in nutrients from atmospheric deposition, agriculture, and development are a well-known component of global change (Galloway et al., 2004). The ability of P. australis to efficiently take up nutrients, especially nitrogen (N; i.e., NO3-, NH4+, and dissolved organic nitrogen), suggests that increased eutrophication from human activities will have a positive impact on the spread of the species, particularly its invasive lineages. Wetland eutrophication is expected to increase in areas such as in agricultural and densely populated urban and suburban areas where nutrient loads continue to increase. However, the distribution of eutrophication under global change is likely to be spatially heterogeneous across regions. In some places, increased water resources due to glacier melting or increased precipitation may dilute N concentrations, whereas, in other places, evaporation and decreased precipitation could exacerbate the effects of pollutants and nutrients (IPCC, 2007, 2014).
Although P. australis grown under controlled experimental conditions generally responds positively to nutrient addition, e.g., displaying increased biomass production and a greater shoot density (Szczepanska and Szczepanski, 1976; Romero et al., 1999; Tho et al., 2016), eutrophication was a key factor responsible for reed die-back in Europe during the 1990s (van der Putten, 1997). However, the detrimental effects were predominantly indirect and caused by anoxic sediments, phytotoxin production from algal blooms or increased litter production, callus development and blockage of gas transport pathways in rhizomes and roots, and exacerbated by other human-induced impairments of natural reed habitats (Armstrong et al., 1996c; Brix, 1999b). In general, the high aeration capacity of the species allows for high root respiration rates throughout its large root systems, which, in turn, can facilitate high nutrient uptake rates (Nakamura et al., 2013). Phragmites australis lineages with inherently high biomass productivity and high belowground:aboveground ratios are therefore well-adapted for growth under increasingly eutrophic and anaerobic conditions, and appropriate models for investigating nutrient availability responses of highly productive and ruderal species (Figure 3). Increased nutrient availability is also likely to increase P. australis inflorescence and floret production (Kettenring et al., 2011) as well as seedling success given that seedlings will grow more rapidly beyond a vulnerable size (Saltonstall and Stevenson, 2007; Kettenring et al., 2015). Nutrient addition can also alter phenology, inducing culms to grow more rapidly early and late in the year, increasing their heights and annual carbon gains (Caplan et al., 2015). Relative to other wetland species, N affinity is very high for P. australis, but is usually its limiting nutrient (Chambers et al., 1998; Clevering, 1998; Romero et al., 1999; Saltonstall and Stevenson, 2007; Mozdzer et al., 2010). In contrast to phosphate, nitrate availability has been shown to result in altered aboveground:belowground biomass ratio of P. australis by favoring aboveground productivity with increasing N addition (Ulrich and Burton, 1985).
In North America, both native and introduced P. australis lineages have the capacity to rapidly take up and assimilate nutrients including inorganic N (Meyerson et al., 2000; Windham and Meyerson, 2003; Hazelton et al., 2010; Mozdzer and Zieman, 2010) and organic N (Mozdzer et al., 2010). However, most studies indicate that, in response to increased N availability, the NAint M lineage is competitively superior to many other wetland species (Chambers et al., 1998; Meyerson et al., 2000; Windham and Meyerson, 2003; Hazelton et al., 2010; Mozdzer et al., 2010, 2013) as well as to the native NAnat lineage (Saltonstall and Stevenson, 2007; Holdredge et al., 2010; Mozdzer et al., 2010, 2013; Mozdzer and Megonigal, 2012). This may be due to its ability to substantially increase carbon assimilation in response to greater N availability (Caplan et al., 2015). Nevertheless, NAint M is also able to regulate its N metabolism to outperform NAnat under low-N conditions (Mozdzer and Megonigal, 2012). This greater plasticity and ability to use available N in both eutrophic and oligotrophic ecosystems can enhance this lineage’s invasiveness by conferring traits such as shifts in phenology as well as increased height growth, leaf area, specific leaf area, leaf area ratio, root mass fraction, and foraging distance (Meadows, 2006; Holdredge et al., 2010; Mozdzer and Zieman, 2010; Mozdzer et al., 2010, 2013; Mozdzer and Megonigal, 2012).
In contrast to NAint M in North America, reeds from the East Asian/Australian group have lower plasticity, N uptake capacity and assimilation rates than co-occurring Spartina alterniflora, a C4 grass that displaces P. australis on the east coast of China (Zhao et al., 2010). Reed lineages adapted to nutrient-poor sites, which preferably translocate nutrients to storage organs rather than the assimilating tissue, may be outcompeted by stronger competitors for nutrients when in eutrophied settings. They may also respond by increasing productivity and culm height, which, due to their inherently lower tissue N allocation, may lead to poor culm stability and mechanical impairment (Kühl et al., 1997). Lineages that are capable of utilizing nutrients at higher concentrations, especially by allocating more biomass and N to their aboveground organs, may gain competitive advantages that contribute to invasive behavior in eutrophied habitats (Tho et al., 2016). The impacts of eutrophication on plants that are adapted to low vs. high nutrient availability can be studied by using NAnat and NAint M. Lineages EU and MED are also appropriate model systems to investigate eutrophication, although to a lesser extent than the North American lineages (Figure 3).
Conclusion and Future Outlook
Although P. australis has been intensely studied, gaps in knowledge remain with respect to the effects that global change will have on community- and ecosystem-level processes. For example, it is not clear how global change will affect Phragmites-herbivore interactions under increased N availability, rising temperatures, and in some regions, increasing salinity (Cronin et al., 2015). Also, the increasing availability of phosphorus may ameliorate the susceptibility of P. australis to physiological stress induced by increased N availability (Tylová et al., 2013) and deserves further investigation. Additional research on wetland soil biogeochemistry and potential changes in nutrient availability under global change are also critically needed. For example, deep rooting by P. australis primes soil carbon deep within the soil profile, accelerating N mineralization under elevated CO2 and N conditions (Mozdzer et al., 2016b) and inducing a loss of previously recalcitrant soil carbon (Bernal et al., 2017). This may offset the concomitant stimulation to P. australis’ gross primary productivity (Caplan et al., 2015), such that the net effects on carbon storage potential of wetlands under global change are unclear. Moreover, there is a need to investigate the suggested modified photosynthetic pathway to compare responses to climate change of C3 and C4-like lineages, including gene-expression patterns and the role of photorespiration under elevated atmospheric CO2 (Bräutigam and Gowik, 2016). Warmer temperatures may increase the impact of P. australis-specific pathogens (Nechwatal et al., 2008), highlighting that climatic effects on pathogenic and symbiotic organisms in the rhizosphere, as well as their effects on the performance of P. australis, deserve further attention. Field investigations addressing higher trophic levels and changing soil conditions would extend future projections of the viability and range distribution of P. australis especially in the plant’s role as an ecosystem engineer affecting the role of wetland habitats as carbon sinks (Mitsch et al., 2013; Caplan et al., 2015).
As a species, P. australis has a high phenotypic plasticity, an extensive ecological amplitude, and capacity to acclimate to adverse environmental conditions. As such, P. australis is unlikely to be threatened by the multiple effects of global change in most regions, but can be expected to benefit from them in many cases. Here, the occurrence of strong latitudinal clines within and between P. australis lineages can be a useful tool for predicting climate change responses, specifically using populations within the same lineage that are distributed over a large geographical gradient. Adaptation to the climate of origin will confer these populations phenotypic plasticity to climatic drivers, and allow comparisons of climate change effects. Reverse transplant experiments and common gardens are particularly amenable to investigations of functional trait responses to climate change. This is demonstrated by reed lineages with distinct phylogeographic origins growing in similar environments, which respond differently to changes in climatic conditions. As global change will place intense selective pressure on diverse P. australis lineages, the distribution and interactions of co-occurring lineages and their within-population variability is very likely to be altered (Figure 3). The globally high genetic (Saltonstall, 2002; Lambertini et al., 2006, 2012a,b,c; Meyerson et al., 2010a, 2012), genomic (Suda et al., 2015; Meyerson et al., 2016b), and phenotypic diversity within P. australis suggests that both lineage- and genotype-specific responses to global change are likely to occur, resulting either in acclimation, advancement or range-shifts. We have distinguished four lineages that can be suitable models for plant species from higher latitudinal ranges (EU), lower-latitudinal ranges (MED), confined ecosystems (NAnat), and fast-spreading species with high phenotypic plasticity (NAint M) (Figure 3).
Although some stress tolerance mechanisms are genetically determined (e.g., those against flooding or salinity), they do not seem to be consistent within lineages. Hence, selection and differentiation within reed populations will be affected by their interactions with local environmental factors. In the worst case, a directional shift in the environment may result in genetic impoverishment of those populations or lineages with a few pre-adapted genotypes and few genotypes with inherently high phenotypic plasticity toward the specific global change driver (Figure 2). Ultimately, reduced genetic diversity may even lead to diminished population viability and local extirpation (Pauls et al., 2013). It is important to note that locally adapted populations that would otherwise be maladapted for rapidly changing future conditions may experience expanded gene-flow due to hybridization between lineages and could eventually replenish populations with genetic diversity.
The rapid invasion of non-native P. australis lineages across North America proves that a selection of well-adapted, highly plastic genotypes in a novel environment is possible and may occur elsewhere. The consequences for ecosystem functioning may be drastic and impossible to reverse. The replacement of diverse genotypes with a few well-adapted genotypes or lineages may yield strong competitors with traits promoting invasion; this may be difficult to detect and control in a species with a cosmopolitan distribution. As we have shown, the ecophysiological responses of P. australis to global change depend on the lineage and genotypes within it. We suggest that the phylogeographic background has to be considered when estimating the future distribution of P. australis populations and populations of cosmopolitan species in general.
Author Contributions
All authors substantially contributed to the work. FE, DFW, and HB drafted the Introduction; CL drafted Intraspecific Variation; FE, JTC, and MKM drafted Influences of Environmental Gradients…; FE, BKS, TJM, and JSC drafted Intraspecific Diversity Determines Responses…; and FE, TJM, XG, W-YG, PP, HS, ELGH, JSC, BKS, KMK, LAM, JTC, MKB, and GPB drafted Key Ecophysiological Processes. All authors contributed to Effects of Major Drivers… and Conclusion and Future Outlook. FE, JSC, HS, CL, ELGH, HB, and BKS made final editorial adjustments. All authors commented on and edited the document and have approved the final submitted document.
Funding
FE was funded by the Carlsberg Foundation (grant CF15-0330). HB and BKS were funded by the Innovation Fund Denmark, FACCE ERA-NET, and FACCE Plus (CINDARELLA 4215-00003B). HB was also funded by the Danish Council for Independent Research – Natural Sciences (Project 4002-00333B). XG was funded by the Natural Science Foundation of Shandong Province, China (BS2015HZ020). GPB and JTC were funded by the US National Science Foundation (grant DEB-1050084), as was LAM (grant DEB-1049914). PP was supported by the Czech Science Foundation (PLADIAS Centre of Excellence, projects 14-36079G and 14-15414S) and the Czech Academy of Sciences (long-term research development project RVO 67985939). PP and HS were funded by the Czech Science Foundation (project 14-15414S) and the Czech Academy of Sciences (long-term research development project RVO 67985939). PP also appreciates support from a Praemium Academiae award through the Czech Academy of Sciences. DFW, KMK, MKM, and ELGH acknowledge funding from the NOAA/SCCOR Mid-Atlantic Shorelines Project (NA09NOS4780214). TJM was funded by Maryland Sea Grant (SA7528082, SA7528114-WW) and the National Science Foundation’s Long Term Research in Environmental Biology Program (DEB-0950080, DEB-1457100, and DEB-1557009).
Conflict of Interest Statement
The authors declare that the research was conducted in the absence of any commercial or financial relationships that could be construed as a potential conflict of interest.
References
Achenbach, L., and Brix, H. (2013). Can differences in salinity tolerance explain the distribution of four genetically distinct lineages of Phragmites australis in the Mississippi River Delta? Hydrobiologia 737, 5–23. doi: 10.1007/s10750-013-1601-y
Achenbach, L., and Brix, H. (2014). Monitoring the short-term response to salt exposure of two genetically distinct Phragmites australis clones with different salinity tolerance levels. Am. J. Plant Sci. 5, 1098–1109. doi: 10.4236/ajps.2014.58122
Achenbach, L., Eller, F., Nguyen, L. X., and Brix, H. (2013). Differences in salinity tolerance of genetically distinct Phragmites australis clones. AoB PLANTS 5, plt019. doi: 10.1093/aobpla/plt019
Achenbach, L., Lambertini, C., and Brix, H. (2012). Phenotypic traits of Phragmites australis clones are not related to ploidy level and distribution range. AoB PLANTS 2012:pls017. doi: 10.1093/aobpla/pls017
Afreen, F., Zobayed, S. M. A., Armstrong, J., and Armstrong, W. (2007). Pressure gradients along whole culms and leaf sheaths, and other aspects of humidity-induced gas transport in Phragmites australis. J. Exp. Bot. 58, 1651–1662. doi: 10.1093/jxb/erm017
Ainsworth, E. A., and Rogers, A. (2007). The response of photosynthesis and a stomatal conductance to rising CO2: mechanisms and environmental interactions. Plant Cell Environ. 30, 258–270. doi: 10.1111/j.1365-3040.2007.01641.x
Allen, W. J., Meyerson, L. A., Cummings, D., Anderson, J., Bhattarai, G. P., and Cronin, J. T. (2017). Biogeography of a plant invasion: drivers of latitudinal variation in enemy release. Glob. Ecol. Biogeogr. 26, 435–446. doi: 10.1111/geb.12550
Almeida, J. P., Montúfar, R., and Anthelme, F. (2013). Patterns and origin of intraspecific functional variability in a tropical alpine species along an altitudinal gradient. Plant Ecol. Divers. 6, 423–433. doi: 10.1080/17550874.2012.702137
Alvarez, M. G., Tron, F., and Mauchamp, A. (2005). Sexual versus asexual colonization by Phragmites australis: 25-year reed dynamics in a Mediterranean marsh, southern France. Wetlands 25, 639–647. doi: 10.1672/0277-5212(2005)025[0639:SVACBP]2.0.CO;2
Antonielli, M., Pasqualini, S., Batini, P., Ederli, L., Massacci, A., and Loreto, F. T. I. (2002). Physiological and anatomical characterisation of Phragmites australis leaves. Aquat. Bot. 72, 55–66. doi: 10.1016/S0304-3770(01)00220-0
Araki, R., Mori, M., Mori, M., and Hasegawa, H. (2005). Genetic differences in nitrate uptake in two clones of the common reed, Phragmites australis. Breed. Sci. 55, 297–302. doi: 10.1270/jsbbs.55.297
Armstrong, J., and Armstrong, W. (1991). A convective through-flow of gases in Phragmites australis (Cav) Trin Ex Steud. Aquat. Bot. 39, 75–88. doi: 10.1016/0304-3770(91)90023-X
Armstrong, J., Armstrong, W., Armstrong, I. B., and Pittaway, G. R. (1996a). Senescence, and phytotoxin, insect, fungal and mechanical damage: factors reducing convective gas-flows in Phragmites australis. Aquat. Bot. 54, 211–226. doi: 10.1016/0304-3770(96)82384-9
Armstrong, J., Armstrong, W., Beckett, P. M., Halder, J. E., Lythe, S., Holt, R., et al. (1996b). Pathways of aeration and the mechanisms and beneficial effects of humidity- and Venturi-induced convections in Phragmites australis (Cav) Trin ex Steud. Aquat. Bot. 54, 177–197. doi: 10.1016/0304-3770(96)01044-3
Armstrong, W., Armstrong, J., and Beckett, P. M. (1996c). Pressurized aeration in wetland macrophytes: some theoretical aspects of humidity-induced convection and thermal transpiration. Folia Geobot. 31, 25–36. doi: 10.1007/BF02803991
Aspinwall, M. J., Lowry, D. B., Taylor, S. H., Juenger, T. E., Hawkes, C. V., Johnson, M. V. V., et al. (2013). Genotypic variation in traits linked to climate and aboveground productivity in a widespread C4 grass: evidence for a functional trait syndrome. New Phytol. 199, 966–980. doi: 10.1111/nph.12341
Baldwin, A. H., Kettenring, K. M., and Whigham, D. F. (2010). Seed banks of Phragmites australis-dominated brackish wetlands: relationships to seed viability, inundation, and land cover. Aquat. Bot. 93, 163–169. doi: 10.1016/j.aquabot.2010.06.001
Bart, D., and Hartman, J. M. (2003). The role of large rhizome dispersal and low salinity windows in the establishment of common reed, Phragmites australis, in salt marshes: new links to human activities. Estuaries 26, 436–443. doi: 10.1007/BF02823720
Bastlová, D., Bastl, M., Cizkova, H., and Kvet, J. (2006). Plasticity of Lythrum salicaria and Phragmites australis growth characteristics across a European geographical gradient. Hydrobiologia 570, 237–242. doi: 10.1007/s10750-006-0186-0
Beckett, L. H., Baldwin, A. H., and Kearney, M. S. (2016). Tidal marshes across a chesapeake bay subestuary are not keeping up with sea-level rise. PLOS ONE 11:e0159753. doi: 10.1371/journal.pone.0159753.s001
Bender, M. A., Knutson, T. R., Tuleya, R. E., Sirutis, J. J., Vecchi, G. A., Garner, S. T., et al. (2010). Modeled impact of anthropogenic warming on the frequency of intense Atlantic hurricanes. Science 327, 454–458. doi: 10.1126/science.1180568
Bernal, B., Megonigal, J. P., and Mozdzer, T. J. (2017). An invasive wetland grass primes deep soil carbon pools. Glob. Change Biol. 23, 2104–2116. doi: 10.1111/gcb.13539
Bhattarai, G. P., and Cronin, J. T. (2014). Hurricane activity and the large-scale pattern of spread of an invasive plant species. PLOS ONE 9:e98478. doi: 10.1371/journal.pone.0098478
Bhattarai, G. P., Meyerson, L. A., Anderson, J., Cummings, D., Allen, W. J., and Cronin, J. T. (2017a). Biogeography of a plant invasion: genetic variation and plasticity in latitudinal clines for traits related to herbivory. Ecol. Monogr. 87, 57–75. doi: 10.1002/ecm.1233
Bhattarai, G. P., Meyerson, L. A., and Cronin, J. T. (2017b). Geographical variation in apparent competition between native and invasive Phragmites australis. Ecology 98, 349–358. doi: 10.1002/ecy.1646
Bodensteiner, L. R., and Gabriel, A. O. (2003). Response of mid-water common reed stands to water level variations and winter conditions in Lake Poygan, Wisconsin, United States. Aquat. Bot. 76, 49–64. doi: 10.1016/S0304-3770(03)00013-5
Bolnick, D. I., Amarasekare, P., Arau jo, M. S., Bürger, R., Levine, J. M., and Novak, M. (2011). Why intraspecific trait variation matters in community ecology. Trends Ecol. Evol. 26, 183–192. doi: 10.1016/j.tree.2011.01.009
Bradshaw, A. D. (1965). Evolutionary significance of phenotypic plasticity in plants. Adv. Genet. 13, 115–155. doi: 10.1016/S0065-2660(08)60048-6
Bragato, C., Brix, H., and Malagoli, M. (2006). Accumulation of nutrients and heavy metals in Phragmites australis (Cav.) Trin. ex Steudel and Bolboschoenus maritimus (L.) Palla in a constructed wetland of the Venice lagoon watershed. Environ. Pollut. 144, 967–975. doi: 10.1016/j.envpol.2006.01.046
Bräutigam, A., and Gowik, U. (2016). Photorespiration connects C3 and C4 photosynthesis. J. Exp. Bot. 67, 2953–2962. doi: 10.1093/jxb/erw056
Brisson, J., Paradis, E., and Bellavance, M. E. (2008). Evidence of sexual reproduction in the invasive common reed (Phragmites australis subsp. australis; Poaceae) in Eastern Canada: A possible consequence of global warming. Rhodora 110, 225–230. doi: 10.3119/07-15.1
Brix, H. (1989). Gas-Exchange through dead culms of reed, Phragmites australis (Cav) Trin Ex Steudel. Aquat. Bot. 35, 81–98. doi: 10.1016/0304-3770(89)90069-7
Brix, H. (1997). Do macrophytes play a role in constructed treatment wetlands? Water Sci. Technol. 35, 11–17.
Brix, H. (1999a). Genetic diversity, ecophysiology and growth dynamics of reed (Phragmites australis). Aquat. Bot. 64, 179–184.
Brix, H. (1999b). The European research project on reed die-back and progression (EUREED). Limnol. Ecol. Manag. Inland Waters 29, 5–10. doi: 10.1016/S0075-9511(99)80033-4
Brix, H., and Schierup, H. H. (1989). The use of aquatic macrophytes in water-pollution control. Ambio 18, 100–107.
Brix, H., Sorrell, B. K., and Lorenzen, B. (2001). Are Phragmites-dominated wetlands a net source or net sink of greenhouse gases? Aquat. Bot. 69, 313–324. doi: 10.1016/S0304-3770(01)00145-0
Brix, H., Sorrell, B. K., and Orr, P. T. (1992). Internal pressurization and convective gas flow in some emergent freshwater macrophytes. Limnol. Oceanogr. 37, 1420–1433. doi: 10.4319/lo.1992.37.7.1420
Brix, H., Sorrell, B. K., and Schierup, H. H. (1996). Gas fluxes achieved by in situ convective flow in Phragmites australis. Aquat. Bot. 54, 151–163. doi: 10.1016/0304-3770(96)01042-X
Burdick, D. M., Buchsbaum, R., and Holt, E. (2001). Variation in soil salinity associated with expansion of Phragmites australis in salt marshes. Environ. Exp. Bot. 46, 247–261. doi: 10.3389/fpls.2016.00432
Burdick, D. M., and Konisky, R. A. (2003). Determinants of expansion for Phragmites australis, common reed, in natural and impacted coastal marshes. Estuaries 26, 407–416. doi: 10.1007/BF02823717
Caplan, J. S., Hager, R. N., Megonigal, J. P., and Mozdzer, T. J. (2015). Global change accelerates carbon assimilation by a wetland ecosystem engineer. Environ. Res. Lett. 10, 115006. doi: 10.1088/1748-9326/10/11/115006
Caplan, J. S., Wheaton, C. N., and Mozdzer, T. J. (2014). Belowground advantages in construction cost facilitate a cryptic plant invasion. AoB PLANTS 6:plu020. doi: 10.1093/aobpla/plu020
Chambers, R., Osgood, D., Bart, D., and Montalto, F. (2003). Phragmites australis invasion and expansion in tidal wetlands: interactions among salinity, sulfide, and hydrology. Estuaries 26, 398–406. doi: 10.1007/BF02823716
Chambers, R. M., Meyerson, L. A., and Saltonstall, K. (1999). Expansion of Phragmites australis into tidal wetlands of North America. Aquat. Bot. 64, 261–273. doi: 10.1016/S0304-3770(99)00055-8
Chambers, R. M., Mozdzer, T. J., and Ambrose, J. C. (1998). Effects of salinity and sulfide on the distribution of Phragmites australis and Spartina alterniflora in a tidal saltmarsh. Aquat. Bot. 62, 161–169. doi: 10.1016/S0304-3770(98)00095-3
Chapman, D. S., Haynes, T., Beal, S., Essl, F., and Bullock, J. M. (2014). Phenology predicts the native and invasive range limits of common ragweed. Glob. Change Biol. 20, 192–202. doi: 10.1111/gcb.12380
Chaves, M. M., Pereira, J. S., Maroco, J., Rodrigues, M. L., Ricardo, C. P. P., Osório, M. L., et al. (2002). How plants cope with water stress in the field? Photosynthesis and growth. Ann. Bot. 89, 907–916. doi: 10.1093/aob/mcf105
Chen, K. M., Gong, H. J., Chen, G. C., Wang, S. M., and Zhang, C. L. (2003). Up-regulation of glutathione metabolism and changes in redox status involved in adaptation of reed (Phragmites communis) ecotypes to drought-prone and saline habitats. J. Plant Physiol. 160, 293–301. doi: 10.1078/0176-1617-00927
Christin, P. A., Salamin, N., Kellogg, E. A., Vicentini, A., and Besnard, G. (2009). Integrating phylogeny into studies of C4 variation in the grasses. Plant Physiol. 149, 82–87. doi: 10.1104/pp.108.128553
Chu, H., Cho, W. K., and Jo, Y. (2011). Identification of natural hybrids in Korean Phragmites using haplotype and genotype analyses. Plant Syst. Evol. 293, 247–253. doi: 10.1007/s00606-011-0423-5
Čížková, H., Brix, H., Kopecky, J., and Lukavska, J. (1999). Organic acids in the sediments of wetlands dominated by Phragmites australis: evidence of phytotoxic concentrations. Aquat. Bot. 64, 303–315. doi: 10.1016/S0304-3770(99)00058-3
Cizkova-Koncalova, H., Kvet, J., and Thompson, K. (1992). Carbon starvation: a key to reed decline in eutrophic lakes. Aquat. Bot. 43, 105–113. doi: 10.1016/0304-3770(92)90036-I
Clevering, O. A. (1998). Effects of litter accumulation and water table on morphology and productivity of Phragmites australis. Wetl. Ecol. Manag. 5, 275–287. doi: 10.1023/A:1008233912279
Clevering, O. A. (1999). Between-and within-population differences in Phragmites australis. Oecologia 121, 447–457. doi: 10.1007/s004420050951
Clevering, O. A., Brix, H., and Lukavská, J. (2001). Geographic variation in growth responses in Phragmites australis. Aquat. Bot. 69, 89–108. doi: 10.1111/gcb.12704
Clevering, O. A., and Lissner, J. (1999). Taxonomy, chromosome numbers, clonal diversity and population dynamics of Phragmites australis. Aquat. Bot. 64, 185–208. doi: 10.1016/S0304-3770(99)00059-5
Colautti, R. I., Alexander, J. M., Dlugosch, K. M., Keller, S. R., and Sultan, S. E. (2017). Invasions and extinctions through the looking glass of evolutionary ecology. Philos. Trans. R. Soc. B 372, 20160031. doi: 10.1098/rstb.2016.0031
Coomes, D. A., and Grubb, P. J. (2000). Impacts of root competition in forests and woodlands: a theoretical framework and review of experiments. Ecol. Monogr. 70, 171–207. doi: 10.1890/0012-9615(2000)070[0171:IORCIF]2.0.CO;2
Coops, H., van den Brink, F. W. B., and van der Velde, G. (1996). Growth and morphological responses of four helophyte species in an experimental water-depth gradient. Aquat. Bot. 54, 11–24. doi: 10.1016/0304-3770(96)01025-X
Cronin, J. T., Bhattarai, G. P., Allen, W. J., and Meyerson, L. A. (2015). Biogeography of a plant invasion: plant-herbivore interactions. Ecology 96, 1115–1127. doi: 10.1890/14-1091.1
Crutsinger, G. M., Souza, L., and Sanders, N. J. (2008). Intraspecific diversity and dominant genotypes resist plant invasions. Ecol. Lett. 11, 16–23.
Cui, B., Hua, Y., Wang, C., Liao, X., Tan, X., and Tao, W. (2010). Estimation of ecological water requirements based on habitat response to water level in Huanghe River Delta, China. Chin. Geogr. Sci. 20, 318–329. doi: 10.1007/s11769-010-0404-6
Den Hartog, C., Květ, J., and Sukopp, H. (1989). Reed. A common species in decline. Aquat. Bot. 35, 1–4. doi: 10.1016/0304-3770(89)90062-4
Douhovnikoff, V., and Hazelton, E. L. (2014). Clonal growth: Invasion or stability? A comparative study of clonal architecture and diversity in native and introduced lineages of Phragmites australis (Poaceae). Am. J. Bot. 10, 1577–1584. doi: 10.3732/ajb.1400177
Drenovsky, R. E., Grewell, B. J., D’Antonio, C. M., Funk, J. L., James, J. J., Molinari, N., et al. (2012). A functional trait perspective on plant invasion. Ann. Bot. 110, 141–153. doi: 10.1093/aob/mcs100
Dukes, J. S., and Mooney, H. A. (1999). Does global change increase the success of biological invaders? Trends Ecol. Evol. 14, 135–139.
Dykyjová, D., Ondok, J. P., and Priban, K. (1970). Seasonal changes in productivity and vertical structure of reed-stands (Phragmites communis Trin.). Photosynthetica 4, 280–287.
Eid, I. M., Shaltout, K. H., Al-Sodany, Y. M., and Kai Jensen, K. (2010). Effects of abiotic conditions on Phragmites australis along geographic gradients in Lake Burullus. Egypt. Aquat. Bot. 92, 86–92. doi: 10.1016/j.aquabot.2009.10.010
Elhaak, M. A., Eldin, A. S., and Sammour, R. H. (1993). Response of Phragmites australis to water-stress from flooding to drought. Pak. J. Bot. 25, 41–46.
Eller, F., and Brix, H. (2012). Different genotypes of Phragmites australis show distinct phenotypic plasticity in response to nutrient availability and temperature. Aquat. Bot. 103, 89–97. doi: 10.1016/j.aquabot.2012.07.001
Eller, F., Lambertini, C., Nguyen, L. X., Achenbach, L., and Brix, H. (2013). Interactive effects of elevated temperature and CO2 on two phylogeographically distinct clones of common reed (Phragmites australis). AoB PLANTS 5, pls051. doi: 10.1093/aobpla/pls051
Eller, F., Lambertini, C., Nguyen, L. X., and Brix, H. (2014a). Increased invasive potential of non-native Phragmites australis: elevated CO2 and temperature alleviate salinity effects on photosynthesis and growth. Glob. Change Biol. 20, 531–543.
Eller, F., Lambertini, C., Nielsen, M. W., Radutoiu, S., and Brix, H. (2014b). Expression of major photosynthetic and salt-resistance genes in invasive reed lineages grown under elevated CO2 and temperature. Ecol. Evol. 4, 4161–4172. doi: 10.1002/ece3.1282
Engels, J. G., and Jensen, K. (2010). Role of biotic interactions and physical factors in determining the distribution of marsh species along an estuarine salinity gradient. Oikos 119, 679–685. doi: 10.1111/j.1600-0706.2009.17940.x
Engloner, A. I. (2004). Annual growth dynamics and morphological differences of reed (Phragmites australis [Cav.] Trin. ex Steudel) in relation to water supply. Flora 199, 256–262. doi: 10.1078/0367-2530-00153
Engloner, A. I. (2009). Structure, growth dynamics and biomass of reed (Phragmites australis) - A review. Flora 204, 331–346. doi: 10.1016/j.flora.2008.05.001
Engloner, A. I., and Major, A. (2011). Clonal diversity of Phragmites australis propagating along water depth gradient. Aquat. Bot. 94, 172–176. doi: 10.1016/j.aquabot.2011.02.007
Engloner, A. I., and Szego, D. (2016). Genetic diversity of riverine reed stands indicating the water regime of the habitat. Ecol. Indic. 61, 846–849. doi: 10.1016/j.ecolind.2015.10.037
Erdei, L., Horváth, F., Tari, I., Pécsváradi, A., Szegletes, Z., and Dulai, S. (2001). Differences in photorespiration, glutamine synthetase and polyamines between fragmented and closed stands of Phragmites australis. Aquat. Bot. 69, 165–176. doi: 10.1016/S0304-3770(01)00136-X
Franks, S. J., Weber, J. J., and Aitken, S. N. (2014). Evolutionary and plastic responses to climate change in terrestrial plant populations. Evol. Appl. 7, 123–139. doi: 10.1111/eva.12112
Galloway, J. N., Dentener, F. J., Capone, D. G., Boyer, E. W., Howarth, R. W., Seitzinger, S. P., et al. (2004). Nitrogen cycles: past, present, and future. Biogeochemistry 70, 153–226. doi: 10.1007/s10533-004-0370-0
Gao, L., Tang, S., Zhuge, L., Nie, M., Zhu, Z., Li, B., et al. (2012). Spatial genetic structure in natural populations of Phragmites australis in a mosaic of saline habitats in the Yellow River Delta, China. PLOS ONE 7:e43334. doi: 10.1371/journal.pone.0043334
Ge, Z. M., Zhang, L. Q., Yuan, L., and Zhang, C. (2014). Effects of salinity on temperature-dependent photosynthetic parameters of a native C3 and a non-native C4 marsh grass in the Yangtze estuary, China. Photosynthetica 52, 484–492. doi: 10.1007/s11099-014-0055-4
Gigante, D., Angiolini, C., Landucci, F., Maneli, F., Nisi, B., Vaselli, O., et al. (2014). New occurrence of reed bed decline in southern Europe: do permanent flooding and chemical parameters play a role? C. R. Biol. 337, 487–498. doi: 10.1016/j.crvi.2014.05.005
Gioria, M., and Osborne, B. A. (2014). Resource competition in plant invasions: emerging patterns and research needs. Front. Plant Sci. 5:501. doi: 10.3389/fpls.2014.00501
Gong, C. M., Bai, J., Deng, J. M., Wang, G. X., and Liu, X. P. (2011). Leaf anatomy and photosynthetic carbon metabolic characteristics in Phragmites communis in different soil water availability. Plant Ecol. 212, 675–687. doi: 10.1007/s11258-010-9854-2
Gorai, M., Vadel, A. M., and Neffati, M. (2006). Seed germination characteristics of Phragmites communis: effects of temperature and salinity. Belg. J. Bot. 139, 78–86.
Gorenflot, R., Hubac, J. M., Jay, M., and Lalande, P. (1983). “Geographic distribution, polyploidy and pattern of flavonoids in Phragmites australis (Cav.) Trin. ex Steud,” in Numerical Taxonomy, ed. J. Felsenstein (Berlin: Springer), 474–478.
Gries, C., Kappen, L., and Losch, R. (1990). Mechanism of flood tolerance in reed, Phragmites australis (Cav) Trin Ex Steudel. New Phytol. 114, 589–593. doi: 10.1111/j.1469-8137.1990.tb00429.x
Guo, W.-Y., Lambertini, C., Li, X.-Z., Meyerson, L. M., and Brix, H. (2013). Invasion of Old World Phragmites australis in the New World: precipitation and temperature patterns combined with human influences redesign the invasive nice. Glob. Change Biol. 19, 3406–3422.
Guo, W.-Y., Lambertini, C., Nguyen, L. X., Li, X.-Z., and Brix, H. (2014). Preadaptation and post-introduction evolution facilitate the invasion of Phragmites australis in North America. Ecol. Evol. 4, 4567–4577. doi: 10.1002/ece3.1286
Han, Z., and Cui, B. (2016). Performance of macrophyte indicators to eutrophication pressure in ponds. Ecol. Eng. 96, 8–19. doi: 10.1016/j.ecoleng.2015.10.019
Hanganu, J., Mihail, G., and Coops, H. (1999). Responses of ecotypes of Phragmites australis to increased seawater influence: a field study in the Danube Delta, Romania. Aquat. Bot. 64, 351–358. doi: 10.1016/S0304-3770(99)00062-5
Hansen, D. L., Lambertini, C., Jampeetong, A., and Brix, H. (2007). Clone-specific differences in Phragmites australis: effects of ploidy level and geographic origin. Aquat. Bot. 86, 269–279. doi: 10.1016/j.aquabot.2006.11.005
Haslam, S. M. (1970). The performance of Phragmites communis Trin. in relation to water-supply. Ann. Bot. 34, 867–877. doi: 10.1093/oxfordjournals.aob.a084418
Haslam, S. M. (1972). Phragmites communis Trin. (Arundo phragmites L., ? Phragmites australis (Cav.) Trin. ex Steudel). J. Ecol. 60, 585–610. doi: 10.2307/2258363
Haslam, S. M. (1973). Some aspects of the life history and autecology of Phragmites communis Trin. A review. Pol. Arch. Hydrobiol. 20, 79–100.
Haslam, S. M. (1975). The performance of Phragmites communis Trin. in relation to temperature. Ann. Bot. 39, 883–888. doi: 10.1093/oxfordjournals.aob.a085006
Hauber, D. P., Saltonstall, K., White, D. A., and Hood, C. S. (2011). Genetic variation in the common reed, Phragmites australis, in the Mississippi River delta marshes: evidence for multiple introductions. Estuar. Coast 34, 851–862. doi: 10.1007/s12237-011-9391-9
Hauber, D. P., White, D. A., Powers, S. P., and Defrancesch, F. R. (1991). Isozyme variation and correspondence with unusual infrared reflectance patterns in Phragmites australis (Poaceae). Plant Syst. Evol. 178, 1–8. doi: 10.1007/BF00937978
Hazelton, E. L., Mozdzer, T. J., Burdick, D. M., Kettenring, K. M., and Whigham, D. F. (2014). Phragmites australis management in the United States: 40 years of methods and outcomes. AoB PLANTS 6, plu001. doi: 10.1093/aobpla/plu001
Hazelton, E. L. G., Knight, T. J., and Theodose, T. A. (2010). Glutamine synthetase partitioning in native and introduced salt marsh grasses. Mar. Ecol. Prog. Ser. 414, 57–64. doi: 10.3354/meps08704
Hellings, S. E., and Gallagher, J. L. (1992). The effects of salinity and flooding on Phragmites australis. J. Appl. Ecol. 29, 41–49. doi: 10.3732/ajb.1600062
Henriques, F. S., and Webb, M. E. (1989). Comparative study of two grasses from different habitats by scanning electron microscopy. Cytologia 54, 299–305. doi: 10.1508/cytologia.54.299
Hernández-Crespo, C., Oliver, N., Bixquert, J., Gargallo, S., and Martín, M. (2016). Comparison of three plants in a surface flow constructed wetland treating eutrophic water in a Mediterranean climate. Hydrobiologia 774, 183–192. doi: 10.1007/s10750-015-2493-9
Hiesey, W. M., Clausen, J., and Keck, D. D. (1942). Relations between climate and intraspecific variation in plants. Am. Nat. 76, 5–22. doi: 10.1086/281009
Holdredge, C., Bertness, M. D., von Wettberg, E., and Silliman, B. R. (2010). Nutrient enrichment enhances hidden differences in phenotype to drive a cryptic plant invasion. Oikos 119, 1776–1784. doi: 10.1111/j.1600-0706.2010.18647.x
Holmes, G. D., Hall, N. E., Gendall, A. R., Boon, P. I., and James, E. A. (2016). Using transcriptomics to identify differential gene expression in response to salinity among Australian Phragmites australis clones. Front. Plant Sci. 7:432. doi: 10.3389/fpls.2016.00432
Hughes, A. R., Schenck, F. R., Bloomberg, J., Hanley, T. C., Feng, D., Gouhier, T. C., et al. (2016). Biogeographic gradients in ecosystem processes of the invasive ecosystem engineer Phragmites australis. Biol. Invasions 18, 2577–2595. doi: 10.1007/s10530-016-1143-0
Ikegami, M., van Hal, S., van Rheenen, J. W. A., Whigham, D. F., and Werger, M. J. A. (2008). Spatial division of labor of Schoenoplectus americanus. Plant Ecol. 199, 55–64. doi: 10.1007/s11258-008-9411-4
IPCC (2007). Summary for Policymakers. Climate Change 2007: The Physical Science Basis. Contribution of Working Group I to the Fourth Assessment Report of the Intergovernmental Panel on Climate Change. Cambridge: Cambridge University Press.
IPCC (2014). Climate Change 2014: Synthesis Report. Contribution of Working Groups I, II and III to the Fifth Assessment Report of the Intergovernmental Panel on Climate Change, eds Core Writing Team, R. K. Pachauri, and L. A. Meyer. Geneva: IPCC, 151.
Irmak, S., Kabenge, I., Rudnick, D., Knezevic, S., Woodward, D., and Moravek, M. (2013). Evapotranspiration crop coefficients for mixed riparian plant community and transpiration crop coefficients for Common reed, Cottonwood and Peach-leaf willow in the Platte River Basin, Nebraska-United States. J. Hydrol. 481, 177–190. doi: 10.1016/j.jhydrol.2012.12.032
Jackson, M. B., and Armstrong, W. (1999). Formation of aerenchyma and the processes of plant ventilation in relation to soil flooding and submergence. Plant Biol. 1, 274–287. doi: 10.1111/j.1438-8677.1999.tb00253.x
Jump, A. S., and Peñuelas, J. (2005). Running to stand still: adaptation and the response of plants to rapid climate change. Ecol. Lett. 8, 1010–1020. doi: 10.1111/j.1461-0248.2005.00796.x
Karunaratne, S., Asaeda, T., and Yutani, K. (2003). Growth performance of Phragmites australis in Japan: influence of geographic gradient. Environ. Exp. Bot. 50, 51–66. doi: 10.1016/S0098-8472(02)00114-4
Kettenring, K. M., de Blois, S., and Hauber, D. P. (2012). Moving from a regional to a continental perspective of Phragmites australis invasion in North America. AoB PLANTS 2012:pls040. doi: 10.1093/aobpla/pls040
Kettenring, K. M., McCormick, M. K., Baron, H. M., and Whigham, D. F. (2010). Phragmites australis (common reed) invasion in the Rhode river subestuary of the Chesapeake bay: disentangling the effects of foliar nutrients, genetic diversity, patch size, and seed viability. Estuar. Coasts 33, 118–126. doi: 10.1007/s12237-009-9241-1
Kettenring, K. M., McCormick, M. K., Baron, H. M., and Whigham, D. F. (2011). Mechanisms of Phragmites australis invasion: feedbacks among genetic diversity, nutrients, and sexual reproduction. J. Appl. Ecol. 48, 1305–1313. doi: 10.1111/j.1365-2664.2011.02024.x
Kettenring, K. M., and Mock, K. E. (2012). Genetic diversity, reproductive mode, and dispersal differ between the cryptic invader, Phragmites australis, and its native conspecific. Biol. Invasions 14, 2489–2504. doi: 10.1007/s10530-012-0246-5
Kettenring, K. M., Mock, K. E., Zaman, B., and McKee, M. (2016). Life on the edge: reproductive mode and rate of invasive Phragmites australis patch expansion. Biol. Invasions 18, 2475–2495. doi: 10.1007/s10530-016-1125-2
Kettenring, K. M., and Whigham, D. F. (2009). Seed viability and seed dormancy of non-native Phragmites australis in suburbanized and forested watersheds of the Chesapeake Bay, United States. Aquat. Bot. 91, 199–204. doi: 10.1016/j.aquabot.2009.06.002
Kettenring, K. M., Whigham, D. F., Hazelton, E. L. G., Gallagher, S. K., and Weiner, H. M. (2015). Biotic resistance, disturbance, and mode of colonization impact the invasion of a widespread, introduced wetland grass. Ecol. Appl. 25, 466–480. doi: 10.1890/14-0434.1
Kim, J., Verma, S. B., and Billesbach, D. P. (1998). Seasonal variation in methane emission from a temperate Phragmites-dominated marsh: effect of growth stage and plant-mediated transport. Glob. Change Biol. 5, 433–440. doi: 10.1046/j.1365-2486.1999.00237.x
Kim, S. Y., and Kang, H. (2008). Effects of elevated CO2 on below-ground processes in temperate marsh microcosms. Hydrobiologia 605, 123–130. doi: 10.1007/s10750-008-9325-0
Knutson, T. R., McBride, J. L., Chan, J., Emanuel, K., Holland, G., Landsea, C., et al. (2010). Tropical cyclones and climate change. Nat. Geosci. 3, 157–163. doi: 10.1038/ngeo779
Kolada, A. (2016). The use of helophytes in assessing eutrophication of temperate lowland lakes: Added value? Aquat. Bot. 129, 44–54. doi: 10.1016/j.aquabot.2015.12.002
Koppitz, H. (1999). Analysis of genetic diversity among selected populations of Phragmites australis world-wide. Aquat. Bot. 64, 209–221. doi: 10.1016/S0304-3770(99)00051-0
Koppitz, H. (2004). Effects of flooding on the amino acid and carbohydrate patterns of Phragmites australis. Limnologica 34, 37–47. doi: 10.1016/S0075-9511(04)80020-3
Koppitz, H., Dewender, M., Ostendorp, W., and Schmieder, K. (2004). Amino acids as indicators of physiological stress in common reed Phragmites australis affected by an extreme flood. Aquat. Bot. 7, 277–294. doi: 10.1016/j.aquabot.2004.05.002
Kriticos, D. J., Webber, B. L., Leriche, A., Ota, N., Bathols, J., Macadam, I., et al. (2012). CliMond: global high resolution historical and future scenario climate surfaces for bioclimatic modelling. Methods Ecol. Evol. 3, 53–64. doi: 10.1111/j.2041-210X.2011.00134.x
Kühl, H., Woitke, P., and Kohl, J. G. (1997). Strategies of nitrogen cycling of Phragmites australis at two sites differing in nutrient availability. Int. Rev. Gesamten Hydrobiol. 82, 57–66. doi: 10.1002/iroh.19970820108
Lambertini, C. (2016). Heteroplasmy due to chloroplast paternal leakage: another insight into Phragmites haplotypic diversity in North America. Biol. Invasions 18, 2443–2455. doi: 10.1007/s10530-016-1193-3
Lambertini, C., Eller, F. P., Achenbach, L., Nguyen, L. X., Guo, W.-Y., and Brix, H. (2012a). “Revisiting Phragmites australis variation in the Danube Delta with DNA molecular techniques,” in International Conference Proceedings: Water Resources and Wetlands, 14–16 September 2012, Tulcea, 142–150.
Lambertini, C., Gustafsson, M. H. G., Frydenberg, J., Lissner, J., Speranza, M., and Brix, H. (2006). A phylogeographic study of the cosmopolitan genus Phragmites (Poaceae) based on AFLPs. Plant Syst. Evol. 258, 161–182. doi: 10.1007/s00606-006-0412-2
Lambertini, C., Mendelsshon, I. A., Gustafsson, M. G. H., Olesen, B., Riis, T., Sorrell, B. K., et al. (2012b). Tracing the origin of Gulf Coast Phragmites (Poaceae) – a story of long distance dispersal and hybridization. Am. J. Bot. 99, 538–551. doi: 10.3732/ajb.1100396
Lambertini, C., Sorrell, B. K., Riis, T., Olesen, B., and Brix, H. (2012c). Exploring the borders of European Phragmites within a cosmopolitan genus. AoB PLANTS 2012:pls020. doi: 10.1093/aobpla/pls020
Latzel, V., and Klimesova, J. (2010). Transgenerational plasticity in clonal plants. Evol. Ecol. 24, 1537–1543. doi: 10.1007/s10682-010-9385-2
Lavergne, S., and Molofsky, J. (2004). Reed canary grass (Phalaris arundinacea) as a biological model in the study of plant invasions. Crit. Rev. Plant Sci. 23, 415–429. doi: 10.1080/07352680490505934
Lavergne, S., and Molofsky, J. (2007). Increased genetic variation and evolutionary potential drive the success of an invasive grass. Proc. Natl. Acad. Sci. U.S.A. 104, 3883–3888. doi: 10.1073/pnas.0607324104
Lee, J., and An, S. (2015). Effect of dikes on the distribution and characteristics of Phragmites australis in temperate intertidal wetlands located in the south sea of Korea. Ocean Sci. J. 50, 49–59. doi: 10.1007/s12601-015-0004-6
Lessmann, J. M., Brix, H., Bauer, V., Clevering, O. A., and Comín, F. A. (2001). Effect of climatic gradients on the photosynthetic responses of four Phragmites australis populations. Aquat. Bot. 69, 109–126. doi: 10.1016/S0304-3770(01)00133-4
Li, F., Xie, Y., Chen, X., Hou, Z., Li, X., Deng, Z., et al. (2013). Succession of aquatic macrophytes in the modern Yellow River Delta after 150 years of alluviation. Wetlands Ecol. Manage. 21, 219–228. doi: 10.1007/s11273-013-9297-3
Lissner, J., and Schierup, H. H. (1997). Effects of salinity on the growth of Phragmites australis. Aquat. Bot. 55, 247–260. doi: 10.1016/S0304-3770(96)01085-6
Lissner, J., Schierup, H. H., Comín, F. A., and Astorga, V. (1999a). Effect of climate on the salt tolerance of two Phragmites australis populations.: I. Growth, inorganic solutes, nitrogen relations and osmoregulation. Aquat. Bot. 64, 317–333.
Lissner, J., Schierup, H. H., Comín, F. A., and Astorga, V. (1999b). Effect of climate on the salt tolerance of two Phragmites australis populations.: II. Diurnal CO2 exchange and transpiration. Aquat. Bot. 64, 335–350. doi: 10.1016/S0304-3770(99)00061-3
Ma, B., Lv, X., Warren, A., and Gong, J. (2013). Shifts in diversity and community structure of endophytic bacteria and archaea across root, stem and leaf tissues in the common reed, Phragmites australis, along a salinity gradient in a marine tidal wetland of northern China. Antonie Van Leeuwenhoek 104, 759–768. doi: 10.1007/s10482-013-9984-3
Mathiasen, P., and Premoli, A. C. (2016). Living on the edge: adaptive and plastic responses of the tree Nothofagus pumilio to a long-term transplant experiment predict rear-edge upward expansion. Oecologia 181, 607–619. doi: 10.1007/s00442-016-3568-7
Matoh, T., Matsushita, N., and Takahashi, E. (1988). Salt tolerance of the reed plant Phragmites communis. Physiol. Plant. 72, 8–14. doi: 10.1111/j.1399-3054.1988.tb06615.x
Mauchamp, A., and Methy, M. (2004). Submergence-induced damage of photosynthetic apparatus in Phragmites australis. Environ. Exp. Bot. 51, 227–235. doi: 10.1016/j.envexpbot.2003.11.002
McCormick, M. K., Kettenring, K. M., Baron, H. M., and Whigham, D. F. (2010a). Extent and mechanisms of Phragmites australis spread in the Rhode River subestuary of the Chesapeake Bay, Maryland (USA). Wetlands 30, 67–74. doi: 10.1007/s13157-009-0007-0
McCormick, M. K., Kettenring, K. M., Baron, H. M., and Whigham, D. F. (2010b). Spread of invasive Phragmites australis in estuaries with differing degrees of development: genetic patterns, Allee effects and interpretation. J. Ecol. 98, 1369–1378. doi: 10.1111/j.1365-2745.2010.01712.x
Meadows, R. E. (2006). Aboveground Competition between Native and Introduced Phragmites in Two Tidal Marsh Basins in Delaware. MS thesis, Delaware State University, Dover, DE.
Meriste, M., Kirsimäe, K., and Freiberg, L. (2012). Relative sea-level changes at shallow coasts inferred from reed bed distribution over the last 50 years in Matsalu bay, the Baltic Sea. J. Coast. Res. 28, 1–10. doi: 10.2112/JCOASTRES-D-10-00049.1
Meyerson, L. A., Cronin, J. T., Bhattarai, G. P., Brix, H., Lambertini, C., Lucanova, M., et al. (2016a). Do ploidy level and nuclear genome size and latitude of origin modify the expression of Phragmites australis traits and interactions with herbivores? Biol. Invasions 18, 2531–2549. doi: 10.1007/s10530-016-1200-8
Meyerson, L. A., Cronin, J. T., and Pyšek, P. (2016b). Phragmites australis as a model organism for studying plant invasions. Biol. Invasions 18, 2421–2431. doi: 10.1007/s10530-016-1132-3
Meyerson, L. A., Lambert, A. M., and Saltonstall, K. (2010a). A tale of three lineages: expansion of common reed (Phragmites australis) in the US Southwest and Gulf Coast. Invasive Plant Sci. Manage. 3, 515–520. doi: 10.1614/IPSM-D-09-00052.1
Meyerson, L. A., Lambertini, C., McCormick, M. K., and Whigham, D. F. (2012). Hybridization of common reed in North America? The answer is blowing in the wind. AoB PLANTS 2012:pls022. doi: 10.1093/aobpla/pls022
Meyerson, L. A., Saltonstall, K., Windham, L., Kiviat, E., and Findlay, S. (2000). A comparison of Phragmites australis in freshwater and brackish marsh environments in North America. Wetl. Ecol. Manage. 8, 89–103. doi: 10.1023/A:1008432200133
Meyerson, L. A., Viola, D. V., and Brown, R. N. (2010b). Hybridization of invasive Phragmites australis with a native subspecies in North America. Biol. Invasions 12, 103–111. doi: 10.1007/s10530-009-9434-3
Milla, R., Cornelissen, J. H. C., van Logtestijn, R. S. P., Toet, S., and Aerts, R. (2006). Vascular plant responses to elevated CO2 in a temperate lowland Sphagnum peatland. Plant Ecol. 182, 13–24. doi: 10.1007/s11258-005-9028-9
Minchinton, T. E. (2002). Precipitation during El Niño correlates with increasing spread of Phragmites australis in New England, United States, coastal marshes. Mar. Ecol. Prog. Ser. 242, 305–309. doi: 10.3354/meps242305
Mitsch, W. J., Bernal, B., Nahlik, A. M., Mander,Ü., Zhang, L., Anderson, C. J., et al. (2013). Wetlands, carbon, and climate change. Landsc. Ecol. 28, 583–597. doi: 10.1007/s10980-012-9758-8
Molina-Montenegro, M. A., Galleguillos, C., Oses, R., Acuña-Rodríguez, I. S., Lavín, P., Gallardo-Cerda, J., et al. (2016). Adaptive phenotypic plasticity and competitive ability deployed under a climate change scenario may promote the invasion of Poa annua in Antarctica. Biol. Invasions 18, 603–618. doi: 10.1007/s10530-015-1033-x
Molina-Montenegro, M. A., and Naya, D. E. (2012). Latitudinal patterns in phenotypic plasticity and fitness-related traits: assessing the climatic variability hypothesis (CVH) with an invasive plant species. PLOS ONE 7:e47620. doi: 10.1371/journal.pone.0047620
Moore, G. E., Burdick, D. M., Peter, C. R., and Keirstead, D. R. (2012). Belowground biomass of Phragmites australis in coastal marshes. Northeast. Nat. 19, 611–626. doi: 10.1656/045.019.0406
Morgan, J. M. (1984). Osmoregulation and water stress in higher plants. Annu. Rev. Plant Physiol. 35, 299–319. doi: 10.1146/annurev.pp.35.060184.001503
Mozdzer, T. J., Brisson, J., and Hazelton, E. L. G. (2013). Physiological ecology and functional traits of North American native and Eurasian introduced Phragmites australis lineages. AoB PLANTS 5:plt048. doi: 10.1093/aobpla/plt048
Mozdzer, T. J., Caplan, J. S., Hager, R. N., Proffitt, C. E., and Meyerson, L. A. (2016a). Contrasting trait responses to latitudinal climate variation in two lineages of an invasive grass. Biol. Invasions 18, 2649–2660. doi: 10.1007/s10530-016-1218-y
Mozdzer, T. J., Langley, J. A., Mueller, P., and Megonigal, J. P. (2016b). Erratum to: deep rooting and global change facilitate spread of invasive grass. Biol. Invasions 18, 2619–2631. doi: 10.1007/s10530-016-1242-y
Mozdzer, T. J., and Megonigal, J. P. (2012). Jack-and-Master trait responses to elevated CO2 and N: a comparison of native and introduced Phragmites australis. PLOS ONE 7:e42794. doi: 10.1371/journal.pone.0042794
Mozdzer, T. J., and Megonigal, J. P. (2013). Increased methane emissions by an introduced Phragmites australis lineage under global change. Wetlands 33, 609–615. doi: 10.1007/s13157-013-0417-x
Mozdzer, T. J., and Zieman, J. C. (2010). Ecophysiological differences between genetic lineages facilitate the invasion of non-native Phragmites australis in North American Atlantic coast wetlands. J. Ecol. 98, 451–458. doi: 10.1111/j.1365-2745.2009.01625.x
Mozdzer, T. J., Zieman, J. C., and McGlathery, K. J. (2010). Nitrogen uptake by native and invasive temperate coastal macrophytes: importance of dissolved organic nitrogen. Estuar. Coasts 33, 784–797. doi: 10.1007/s12237-009-9254-9
Munguia-Rosas, M. A., Ollerton, J., Parra-Tabla, V., and De-Nova, J. A. (2011). Meta-analysis of phenotypic selection on flowering phenology suggests that early flowering plants are favoured. Ecol. Lett. 14, 511–521. doi: 10.1111/j.1461-0248.2011.01601.x
Münzbergová, Z., and Hadincová, V. (2017). Transgenerational plasticity as an important mechanism affecting response of clonal species to changing climate. Ecol. Evol. 7, 5236–5247. doi: 10.1002/ece3.3105
Münzbergová, Z., Hadincová, V., Skálová, H., and Vandvik, V. (2017). Genetic differentiation and plasticity interact along temperature and precipitation gradients to determine plant performance under climate change. J. Ecol. 105, 1358–1373. doi: 10.1111/1365-2745.12762
Nada, R. M., Khedr, A. H. A., Serag, M. S., and El-Nagar, N. A. (2015). Growth, photosynthesis and stress-inducible genes of Phragmites australis (Cav.) Trin. Ex Steudel from different habitats. Aquat. Bot. 124, 54–62. doi: 10.1016/j.aquabot.2015.03.007
Nakamura, M., Nakamurac, T., Tsuchiyaa, T., and Noguchi, K. (2013). Functional linkage between N acquisition strategies and aeration capacities of hydrophytes for efficient oxygen consumption in roots. Physiol. Plant. 147, 135–146. doi: 10.1111/j.1399-3054.2012.01643.x
Naumann, J. C., Young, D. R., and Anderson, J. E. (2007). Linking leaf chlorophyll fluorescence properties to physiological responses for detection of salt and drought stress in coastal plant species. Physiol. Plant. 131, 422–433. doi: 10.1111/j.1399-3054.2007.00973.x
Nechwatal, J., Wielgoss, A., and Mendgen, K. (2008). Flooding events and rising water temperatures increase the significance of the reed pathogen Pythium phragmitis as a contributing factor in the decline of Phragmites australis. Hydrobiologia 613, 109–115. doi: 10.1007/s10750-008-9476-z
Nguyen, L. X., Lambertini, C., Sorrell, B. K., Eller, F., Achenbach, L., and Brix, H. (2013). Photosynthesis of co-existing Phragmites haplotypes in their non-native range: Are characteristics determined by adaptations derived from their native origin? AoB PLANTS 5:plt016. doi: 10.1093/aobpla/plt016
Niu, S., Luo, Y., Li, D., Cao, S., Xia, J., Li, J., et al. (2014). Plant growth and mortality under climatic extremes: an overview. Environ. Exp. Bot. 98, 13–19. doi: 10.1016/j.envexpbot.2013.10.004
Oney, B., Reineking, B., O’Neill, G., and Kreyling, J. (2013). Intraspecific variation buffers projected climate change impacts on Pinus contorta. Ecol. Evol. 3, 437–449. doi: 10.1002/ece3.426
Ostendorp, W. (1989). ‘Die-back’ of reeds in Europe - a critical review of Literature. Aquatic Botany 35, 5–26. doi: 10.1016/0304-3770(89)90063-6
Ostendorp, W. (1999). Susceptibility of lakeside Phragmites reeds to environmental stresses: examples from lake Constance-Untersee (SW-Germany). Limnologica 29, 21–27. doi: 10.1016/S0075-9511(99)80035-8
Ostendorp, W., and Dienst, M. (2012). Geschichte der seeuferröhrichte in der grenzzone des Bodensee-Untersees. Mitt. Thurgauischen Naturforschungs Ges. 66, 155–197.
Ostendorp, W., Dienst, M., and Schmieder, K. (2003). Disturbance and rehabilitation of lakeside Phragmites reeds following an extreme flood in Lake Constance (Germany). Hydrobiologia 506, 687–695. doi: 10.1023/B:HYDR.0000008622.60094.6d
Packer, J. G., Meyerson, L. A., Richardson, D. M., Brundu, G., Allen, W. J., Bhattarai, G. P., et al. (2017a). Global networks for invasion science: benefits, challenges and guidelines. Biol. Invasions 19, 1081–1096. doi: 10.1007/s10530-016-1302-3
Packer, J. G., Meyerson, L. A., Skalova, H., Pysek, P., and Kueffer, C. (2017b). Biological flora of the British isles: Phragmites australis. J. Ecol. 105, 1123–1162. doi: 10.1111/1365-2745.12797
Packett, C. R., and Chambers, R. M. (2006). Distribution and nutrient status of haplotypes of the marsh grass Phragmites australis along the Rappahannock River in Virginia. Estuar. Coasts 29, 1222–1225. doi: 10.1007/BF02781822
Pagter, M., Bragato, C., and Brix, H. (2005). Tolerance and physiological responses of Phragmites australis to water deficit. Aquat. Bot. 81, 285–299. doi: 10.1016/j.aquabot.2005.01.002
Pagter, M., Bragato, C., Malagoli, M., and Brix, H. (2009). Osmotic and ionic effects of NaCl and Na2SO4 salinity on Phragmites australis. Aquat. Bot. 90, 43–51. doi: 10.1016/j.aquabot.2008.05.005
Pauca-Comanescu, M., Clevering, O. A., Hanganu, J., and Gridin, M. (1999). Phenotypic differences among ploidy levels of Phragmites australis growing in Romania. Aquat. Bot. 64, 223–234. doi: 10.1016/S0304-3770(99)00052-2
Paul, J., Kirk, H., and Freeland, J. (2011). Genetic diversity and differentiation of fragmented reedbeds (Phragmites australis) in the United Kingdom. Hydrobiologia 665, 107–115. doi: 10.1007/s10750-011-0608-5
Pauls, S. U., Nowak, C., Balint, M., and Pfenninger, M. (2013). The impact of global climate change on genetic diversity within populations and species. Mol. Ecol. 22, 925–946. doi: 10.1111/mec.12152
Poorter, H., Niinemets, U., Poorter, L., Wright, I. J., and Villar, R. (2009). Causes and consequences of variation in leaf mass per area (LMA): a meta-analysis. New Phytol. 182, 565–588. doi: 10.1111/j.1469-8137.2009.02830.x
Post, E. (2013). Ecology of Climate Change: The Importance of Biotic Interactions. Princeton, NJ: Princeton University Press.
Price, A. L., Fant, J. B., and Larkin, D. J. (2014). Ecology of native vs. introduced Phragmites australis (common reed) in Chicago-area wetlands. Wetlands 34, 369–377. doi: 10.1007/s13157-013-0504-z
Pyšek, P., Jarošík, V., Hulme, P. E., Pergl, J., Hejda, M., Schaffner, U., et al. (2012). A global assessment of invasive plant impacts on resident species, communities and ecosystems: the interaction of impact measures, invading species’ traits and environment. Glob. Change Biol. 18, 1725–1737. doi: 10.1111/j.1365-2486.2011.02636.x
Pyšek, P., Pergl, J., Essl, F., Lenzner, B., Dawson, W., Kreft, H., et al. (2017). Naturalized alien flora of the world: species diversity, taxonomic and phylogenetic patterns, geographic distribution and global hotspots of plant invasion. Preslia 89, 203–274. doi: 10.23855/preslia.2017.203
Raunkiaer, C. (1893). En ny form af tagrør: Phragmites communis Trin. F. coarctata. Bot. Tidsskr. 18, 274–278.
Rechav, Y. (1967). Ecotypic Differentiation in Phragmites communis Trin. M.Sc. thesis, Tel-Aviv University, Tel-Aviv.
Reich, P. B., and Oleksyn, J. (2008). Climate warming will reduce growth and survival of Scots pine except in the far north. Ecol. Lett. 11, 588–597. doi: 10.1111/j.1461-0248.2008.01172.x
Rintamaki, E., and Aro, E. M. (1985). Photosynthetic and photorespiratory enzymes in widely divergent plant-species with special reference to the moss Ceratodon purpureus - properties of ribulose bisphosphate carboxylase/oxygenase, phosphoenolpyruvate carboxylase and glycolate oxidase. J. Exp. Bot. 36, 1677–1684. doi: 10.1093/jxb/36.11.1677
Rodewald-Rudescu, L. (1974). Das Schilfrohr, Phragmites communis Trinius Binnengewässer 27. Stuttgart: E. Schweizerbart, 1–302.
Rodriguez, M., and Brisson, J. (2016). Does the combination of two plant species improve removal efficiency in treatment wetlands? Ecol. Eng. 91, 302–309. doi: 10.1016/j.ecoleng.2016.02.047
Rolletschek, H., Hartzendorf, T., Rolletschek, A., and Kohl, J. G. (1999). Biometric variation in Phragmites australis affecting convective ventilation and amino acid metabolism. Aquat. Bot. 64, 291–302. doi: 10.1016/S0304-3770(99)00057-1
Romero, J. A., Brix, H., and Comin, F. A. (1999). Interactive effects of N and P on growth, nutrient allocation and NH4 uptake kinetics by Phragmites australis. Aquat. Bot. 64, 369–380. doi: 10.1016/S0304-3770(99)00064-9
Rooth, J. E., Stevenson, J. C., and Cornwall, J. C. (2003). Increased sediment accretion rates following invasion by Phragmites australis: the role of litter. Estuaries 26, 475–483. doi: 10.1007/BF02823724
Sage, R. F. (2016). Tracking the evolutionary rise of C4 metabolism. J. Exp. Bot. 67, 2919–2922. doi: 10.1093/jxb/erw137
Saltmarsh, A., Mauchamp, A., and Rambal, S. (2006). Contrasted effects of water limitation on leaf functions and growth of two emergent co-occurring plant species, Cladium mariscus and Phragmites australis. Aquat. Bot. 84, 191–198. doi: 10.1016/j.aquabot.2005.09.010
Saltonstall, K. (2002). Cryptic invasion by a non-native genotype of the common reed, Phragmites australis, into North America. Proc. Natl. Acad. Sci. U.S.A. 99, 2445–2449. doi: 10.1073/pnas.032477999
Saltonstall, K. (2003). Microsatellite variation within and among North American lineages of Phragmites australis. Mol. Ecol. 12, 1689–1702. doi: 10.1046/j.1365-294X.2003.01849.x
Saltonstall, K., Castillo, H. E., and Blossey, B. (2014). Confirmed field hybridization of native and introduced Phragmites australis (Poaceae) in North America. J. Am. Bot. 101, 211–215. doi: 10.3732/ajb.1300298
Saltonstall, K., and Lambert, A. (2015). What happens in Vegas, better stay in Vegas: Phragmites australis hybrids in the Las Vegas wash. Biol. Invasions 18, 2463–2474. doi: 10.1007/s10530-016-1167-5
Saltonstall, K., and Stevenson, J. C. (2007). The effect of nutrients on seedling growth of native and introduced Phragmites australis. Aquat. Bot. 86, 331–336. doi: 10.1016/j.aquabot.2006.12.003
Schmid, S. F., Stocklin, J., Hamann, E., and Kesselring, H. (2017). High-elevation plants have reduced plasticity in flowering time in response to warming compared to low-elevation congeners. Basic Appl. Ecol. 21, 1–12. doi: 10.1016/j.baae.2017.05.003
Schöb, C., Armas, C., Guler, M., Prieto, I., and Pugnaire, F. I. (2013). Variability in functional traits mediates plant interactions along stress gradients. J. Ecol. 101, 753–762. doi: 10.1111/1365-2745.12062
Scholefield, P. A., Doick, K. J., Herbert, B. M. J., Hewitt, C. N. S., Schnitzler, J. P., Pinelli, P., et al. (2004). Impact of rising CO2 on emissions of volatile organic compounds: isoprene emission from Phragmites australis growing at elevated CO2 in a natural carbon dioxide spring Plant. Cell Environ. 27, 393–401. doi: 10.1111/j.1365-3040.2003.01155.x
Sciance, M. B., Patrick, C. J., Weller, D. E., Williams, M. N., McCormick, M. K., and Hazelton, E. L. G. (2016). Local and regional disturbances associated with the invasion of Chesapeake Bay marshes by the common reed Phragmites australis. Biol. Invasions 18, 2661–2677. doi: 10.1007/s10530-016-1136-z
Sorrell, B. K., Brix, H., Schierup, H. H., and Lorenzen, B. (1997). Die-back of Phragmites australis: influence on the distribution and rate of sediment methanogenesis. Biogeochemistry 36, 173–188. doi: 10.1023/A:1005761609386
Soukup, A., Votrubova, O., and Cizkova, H. (2000). Internal segmentation of rhizomes of Phragmites australis: protection of the internal aeration system against being flooded. New Phytol. 145, 71–75. doi: 10.1046/j.1469-8137.2000.00555.x
Springate, D. A., and Kover, P. X. (2014). Plant responses to elevated temperatures: a field study on phenological sensitivity and fitness responses to simulated climate warming. Glob. Change Biol. 20, 456–465. doi: 10.1111/gcb.12430
Stebbins, G. L. (1971). Adaptive radiation of reproductive characteristics in angiosperms, II: seeds and seedlings. Annu. Rev. Ecol. Syst. 2, 237–260. doi: 10.1146/annurev.es.02.110171.001321
Suda, J., Meyerson, L. A., Leitch, I. J., and Pyšek, P. (2015). The hidden side of plant invasions: the role of genome size. New Phytol. 205, 994–1007. doi: 10.1111/nph.13107
Szczepanska, W., and Szczepanski, A. (1976). Growth of Phragmites communis Trin, Typha latifolia L, and Typha angustifolia L in relation to the fertility of soils. Pol. Arch. Hydrobiol. 23, 233–248.
Takahashi, R., Nishio, T., Ichizen, N., and Takano, T. (2007). High-affinity K+ transporter PhaHAK5 is expressed only in salt-sensitive reed plants and shows Na+ permeability under NaCl stress. Plant Cell Rep. 26, 1673–1679. doi: 10.1007/s00299-007-0364-1
Tang, L., Gao, Y., Wang, C. H., Li, B., Chen, J. K., and Zhao, B. (2013). Habitat heterogeneity influences restoration efficacy: implications of a habitat-specific management regime for an invaded marsh. Estuar. Coast. Shelf Sci. 125, 20–26. doi: 10.1016/j.ecss.2013.03.013
Tho, B. T., Sorrell, B. K., Lambertini, C., Eller, F., and Brix, H. (2016). Phragmites australis: how do genotypes of different phylogeographic origins differ from their invasive genotypes in growth, nitrogen allocation and gas exchange? Biol. Invasions 18, 2563–2576. doi: 10.1007/s10530-016-1158-6
Thuiller, W., Lavorel, S., Araujo, M. B., Sykes, M. T., Prentice, I. C., and Mooney, H. A. (2005). Climate change threats to plant diversity in Europe. Proc. Natl. Acad. Sci. U.S.A. 102, 8245–8250. doi: 10.1073/pnas.0409902102
Touchette, B. W., Iannacone, L. R., Turner, G. E., and Frank, A. R. (2007). Drought tolerance versus drought avoidance: a comparison of plant-water relations in herbaceous wetland plants subjected to water withdrawal and repletion. Wetlands 27, 656–667. doi: 10.1672/0277-5212(2007)27[656:DTVDAA]2.0.CO;2
Trenberth, K. E., Dai, A., van der Schrier, G., Jones, P. D., Barichivich, J., Briffa, K. R., et al. (2014). Global warming and changes in drought. Nat. Clim. Change 4, 17–22. doi: 10.1038/nclimate2067
Tripathee, R., and Schäfer, K. V. R. (2014). Above- and belowground biomass allocation in four dominant salt marsh species of the eastern United States. Wetlands 35, 21–30. doi: 10.1007/s13157-014-0589-z
Tulbure, M. G., Ghioca-Robrecht, D. M., Johnston, C. A., and Whigham, D. F. (2012). Inventory and ventilation efficiency of nonnative and native Phragmites australis (common reed) in tidal wetlands of the Chesapeake Bay. Estuar. Coasts 35, 1353–1359. doi: 10.1007/s12237-012-9529-4
Tulbure, M. G., and Johnston, C. A. (2010). Environmental conditions promoting non-native Phragmites australis expansion in Great Lakes coastal wetlands. Wetlands 30, 577–587. doi: 10.1007/s13157-010-0054-6
Tulbure, M. G., Johnston, C. A., and Auger, D. L. (2007). Rapid invasion of a Great Lakes coastal wetland by non-native Phragmites australis and Typha. J. Great Lakes Res. 33, 269–279. doi: 10.3394/0380-1330(2007)33[269:RIOAGL]2.0.CO;2
Tylová, E., Steinbachová, L., Soukup, A., Gloser, V., and Votrubová, O. (2013). Pore water N:P and NH4+:NO3- alter the response of Phragmites australis and Glyceria maxima to extreme nutrient regimes. Hydrobiologia 700, 141–155. doi: 10.1007/s10750-012-1225-7
Tylova-Munzarova, E., Bent Lorenzen, B., Hans Brix, H., and Olga Votrubova, O. (2005). The effects of NH4+ and NO3- on growth, resource allocation and nitrogen uptake kinetics of Phragmites australis and Glyceria maxima. Aquat. Bot. 81, 326–342. doi: 10.1016/j.aquabot.2005.01.006
Ulrich, K. E., and Burton, T. M. (1985). The effects of nitrate, phosphate and potassium fertilization on growth and nutrient uptake patterns of Phragmites australis (Cav) Trin ex Steudel. Aquat. Bot. 21, 53–62. doi: 10.1016/0304-3770(85)90095-6
Valladares, F., Matesanz, S., Guilhaumon, F., Araujo, M. B., Balaguer, L., Benito-Garzon, M., et al. (2014). The effects of phenotypic plasticity and local adaptation on forecasts of species range shifts under climate change. Ecol. Lett. 17, 1351–1364. doi: 10.1111/ele.12348
van der Putten, W. H. (1997). Die-back of Phragmites australis in European wetlands: an overview of the European research programme on reed die-back and progression (1993–1994). Aquat. Bot. 59, 263–275. doi: 10.1016/S0304-3770(97)00060-0
Van der Toorn, J. (1972). Variability of Phragmites australis (Cav) Trin ex Steudel in relation to the environment. Van Zee Land 48, 1–122.
van Kleunen, M., Dawson, W., Essl, F., Pergl, J., Winter, M., Weber, E., et al. (2015). Global exchange and accumulation of non-native plants. Nature 525, 100–103. doi: 10.1038/nature14910
Vartapetian, B. B., and Jackson, M. B. (1997). Plant adaptations to anaerobic stress. Ann. Bot. 79, 3–20. doi: 10.1093/oxfordjournals.aob.a010303
Vasquez, E. A., Glenn, E. P., Brown, J. J., Guntenspergen, G. R., and Nelson, S. G. (2005). Salt tolerance underlies the cryptic invasion of North American salt marshes by an introduced haplotype of the common reed Phragmites australis (Poaceae). Mar. Ecol. Prog. Ser. 298, 1–8. doi: 10.3354/meps298001
Vasquez, E. A., Glenn, E. P., Guntenspergen, G. R., Brown, J. J., and Nelson, S. G. (2006). Salt tolerance and osmotic adjustment of Spartina alterniflora (Poaceae) and the invasive M haplotype of Phragmites australis (Poaceae) along a salinity gradient. Am. J. Bot. 93, 1784–1790. doi: 10.3732/ajb.93.12.1784
Vermaat, J. E., Bos, B., and van der Burg, P. (2016). Why do reed beds decline and fail to re-establish? A case study of Dutch peat lakes. Freshw. Biol. 61, 1580–1589. doi: 10.1111/fwb.12801
Vilà, M., Espinar, J. L., Hejda, M., Hulme, P. E., Jarošík, V., Maron, J. L., et al. (2011). Ecological impacts of invasive alien plants: a meta-analysis of their effects on species, communities and ecosystems. Ecol. Lett. 14, 702–708. doi: 10.1111/j.1461-0248.2011.01628.x
Violle, C., Enquist, B. J., McGill, B. J., Jiang, L. I. N., Albert, C. H., Hulshof, C., et al. (2012). The return of the variance: intraspecific variability in community ecology. Trends Ecol. Evol. 27, 244–252. doi: 10.1016/j.tree.2011.11.014
Visser, M. E. (2016). Phenology: interactions of climate change and species. Nature 535, 236–237. doi: 10.1038/nature18905
Vretare, V., Weisner, S. E. B., Strand, J. A., and Graneli, W. (2001). Phenotypic plasticity in Phragmites australis as a functional response to water depth. Aquat. Bot. 69, 127–145. doi: 10.1016/S0304-3770(01)00134-6
Vymazal, J. (2013). Emergent plants used in free water surface constructed wetlands: a review. Ecol. Eng. 61, 582–592. doi: 10.1016/j.ecoleng.2013.06.023
Wang, H., Hao, L., Wen, J., Zhang, C., and Liang, H. (1998). Differential expression of photosynthesis-related genes of reed ecotypes in response to drought and saline habitats. Photosynthetica 35, 61–69. doi: 10.1023/A:1006817714739
Wang, W., Wang, C., Sardans, J., Tong, C., Jia, R., Zeng, C., et al. (2015). Flood regime affects soil stoichiometry and the distribution of the invasive plants in subtropical estuarine wetlands in China. Catena 128, 144–154. doi: 10.1016/j.catena.2015.01.017
Weisner, S. E. B., and Strand, J. A. (1996). Rhizome architecture in Phragmites australis in relation to water depth: implications for within-plant oxygen transport distances. Folia Geobot. 31, 91–97. doi: 10.1007/BF02803998
White, S. D., Deegan, B. M., and Ganf, G. G. (2007). The influence of water level fluctuations on the potential for convective flow in the emergent macrophytes Typha domingensis and Phragmites australis. Aquat. Bot. 86, 369–376. doi: 10.1016/j.aquabot.2007.01.006
White, S. D., and Ganf, G. G. (2002). A comparison of the morphology, gas space anatomy and potential for internal aeration in Phragmites australis under variable and static water regimes. Aquat. Bot. 73, 115–127. doi: 10.1016/S0304-3770(02)00010-4
Whyte, R. S., Trexel-Kroll, D., Klarer, D. M., Shields, R., and Francko, D. A. (2008). The invasion and spread of Phragmites australis during a period of low water in a Lake Erie coastal wetland. J. Coast. Res. 55, 111–120. doi: 10.2112/SI55-19.1
Wilson, E. O. (eds). (1988). “The current state of biological diversity,” in Biodiversity (Washington, DC: National Academy Press), 3–18.
Windham, L., and Meyerson, L. A. (2003). Effects of common reed (Phragmites australis) expansions on nitrogen dynamics of tidal marshes of the northeastern U.S. Estuaries 26, 452-464. doi: 10.1007/BF02823722
Winkel, A., Pedersen, O., Ella, E., Ismail, A. M., and Colmer, T. D. (2014). Gas film retention and underwater photosynthesis during field submergence of four contrasting rice genotypes. J. Exp. Bot. 65, 3225–3233. doi: 10.1093/jxb/eru166
Wright, J. P., and Jones, C. G. (2004). Predicting effects of ecosystem engineers on patch-scale species richness from primary productivity. Ecology 85, 2071–2081. doi: 10.1890/02-8018
Wu, C. A., Murray, L. A., and Heffernan, K. E. (2015). Evidence for natural hybridization between native and introduced lineages of Phragmites australis in the Chesapeake Bay watershed. Am. J. Bot. 102, 805–812. doi: 10.3732/ajb.1500018
Xiang, J., Jiang, A. N., Fang, Y. P., Huang, L. B., and Zhang, H. (2012). Effects of soil water gradient on stress-resistant enzyme activities in Phragmites australis from Yellow River Delta. Proc. Environ. Sci. 13, 2464–2468. doi: 10.1016/j.proenv.2012.01.236
Yamasaki, S., and Tange, I. (1981). Growth responses of Zizania latifolia, Phragmites australis and Miscanthus sacchariflorus to varying inundation. Aquat. Bot. 10, 229–239. doi: 10.1016/0304-3770(81)90025-5
Yarwood, S. A., Baldwin, A. H., Mateu, M. G., and Buyer, J. S. (2016). Archeal rhizosphere communities differ between the native and invasive lineages of the wetland plant Phragmites australis (common reed) in a Chesapeake Bay subestuary. Biol. Invasions 18, 2717–2728. doi: 10.1007/s10530-016-1144-z
Yu, J., Wang, X., Ning, K., Li, Y., Wu, H., Fu, Y., et al. (2012). Effects of salinity and water depth on germination of Phragmites australis in coastal wetland of the Yellow River Delta. Clean Soil Air Water 40, 1154–1158. doi: 10.1002/clen.201100743
Zemlin, R., Kühl, H., and Kohl, J. G. (2000). Effects of seasonal temperature on shoot growth dynamics and shoot morphology of common reed (Phragmites australis). Wetl. Ecol. Manag. 8, 447–457. doi: 10.1023/A:1026566103296
Zhai, X. (2013). Direct Optimization for Classification with Boosting. Ph.D. dissertation, Wright State University, Fairborn, OH.
Zhang, G., and Deng, C. (2012). Gas exchange and chlorophyll fluorescence of salinity-alkalinity stressed Phragmites australis seedlings. J. Food Agric. Environ. 10, 880–884.
Zhao, Y. J., Qing, H., Zhao, C. J., Zhou, C. F., Zhang, W. G., Xiao, Y., et al. (2010). Phenotypic plasticity of Spartina alterniflora and Phragmites australis in response to nitrogen addition and intraspecific competition. Hydrobiologia 637, 143–155. doi: 10.1007/s10750-009-9992-5
Zheng, W. J., Zheng, X. P., and Zhang, C. L. (2000). A survey of photosynthetic carbon metabolism in 4 ecotypes of Phragmites australis in northwest China: leaf anatomy, ultrastructure, and activities of ribulose 1, 5-bisphosphate carboxylase, phosphoenolpyruvate carboxylase and glycollate oxidase. Physiol. Plant. 110, 201–208. doi: 10.1034/j.1399-3054.2000.110209.x
Zhu, X., Chen, G., and Zhang, C. (2001). Photosynthetic electron transport, photophosphorylation, and antioxidants in two ecotypes of reed (Phragmites communis Trin) from different habitats. Photosynthetica 39, 183–189. doi: 10.1023/A:1013766722604
Zhu, X. Y., Jing, Y., Chen, G. C., Wang, S. M., and Zhang, C. L. (2003a). Solute levels and osmoregulatory enzyme activities in reed plants adapted to drought and saline habitats. Plant Growth Regul. 41, 165–172. doi: 10.1023/A:1027381006811
Zhu, X. Y., Wang, S. M., and Zhang, C. L. (2003b). Composition and characteristic differences in photosynthetic membranes of two ecotypes of reed (Phragmites communis L) from different habitats. Photosynthetica 41, 97–104. doi: 10.1023/A:1025820731410
Keywords: atmospheric CO2, climate change, eutrophication, global distribution, intraspecific variation, invasive species, salinity, temperature
Citation: Eller F, Skálová H, Caplan JS, Bhattarai GP, Burger MK, Cronin JT, Guo W-Y, Guo X, Hazelton ELG, Kettenring KM, Lambertini C, McCormick MK, Meyerson LA, Mozdzer TJ, Pyšek P, Sorrell BK, Whigham DF and Brix H (2017) Cosmopolitan Species As Models for Ecophysiological Responses to Global Change: The Common Reed Phragmites australis. Front. Plant Sci. 8:1833. doi: 10.3389/fpls.2017.01833
Received: 20 June 2017; Accepted: 10 October 2017;
Published: 16 November 2017.
Edited by:
Sebastian Leuzinger, Auckland University of Technology, New ZealandReviewed by:
Michael J. O’Brien, Estacion Experimental de Zonas Aridas (CSIC), SpainBartosz Adamczyk, University of Helsinki, Finland
Copyright © 2017 Eller, Skálová, Caplan, Bhattarai, Burger, Cronin, Guo, Guo, Hazelton, Kettenring, Lambertini, McCormick, Meyerson, Mozdzer, Pyšek, Sorrell, Whigham and Brix. This is an open-access article distributed under the terms of the Creative Commons Attribution License (CC BY). The use, distribution or reproduction in other forums is permitted, provided the original author(s) or licensor are credited and that the original publication in this journal is cited, in accordance with accepted academic practice. No use, distribution or reproduction is permitted which does not comply with these terms.
*Correspondence: Franziska Eller, franziska.eller@bios.au.dk
†Present address: Eric L.G. Hazelton, Cold Regions Research and Engineering Laboratory, US Army Corps of Engineers, Hanover, NH, United States