- 1Rubber Research Institute, Chinese Academy of Tropical Agricultural Sciences, Danzhou, China
- 2College of Tropical Agriculture and Forestry, Hainan University, Haikou, China
- 3Institute of Tropical Bioscience and Biotechnology, Chinese Academy of Tropical Agricultural Sciences, Haikou, China
Sucrose-metabolizing enzymes in plant leaves have hitherto been investigated mainly in temperate plants, and rarely conducted in tandem with gene expression and sugar analysis. Here, we investigated the sugar content, gene expression, and the activity of sucrose-metabolizing enzymes in the leaves of Hevea brasiliensis, a tropical tree widely cultivated for natural rubber. Sucrose, fructose and glucose were the major sugars detected in Hevea leaves at four developmental stages (I to IV), with starch and quebrachitol as minor saccharides. Fructose and glucose contents increased until stage III, but decreased strongly at stage IV (mature leaves). On the other hand, sucrose increased continuously throughout leaf development. Activities of all sucrose-cleaving enzymes decreased markedly at maturation, consistent with transcript decline for most of their encoding genes. Activity of sucrose phosphate synthase (SPS) was low in spite of its high transcript levels at maturation. Hence, the high sucrose content in mature leaves was not due to increased sucrose-synthesizing activity, but more to the decline in sucrose cleavage. Gene expression and activities of sucrose-metabolizing enzymes in Hevea leaves showed striking differences compared with other plants. Unlike in most other species where vacuolar invertase predominates in sucrose cleavage in developing leaves, cytoplasmic invertase and sucrose synthase (cleavage direction) also featured prominently in Hevea. Whereas SPS is normally responsible for sucrose synthesis in plant leaves, sucrose synthase (synthesis direction) was comparable or higher than that of SPS in Hevea leaves. Mature Hevea leaves had an unusually high sucrose:starch ratio of about 11, the highest reported to date in plants.
Introduction
Mature leaves are the main photosynthetic organs that fix atmospheric carbon to produce sucrose to feed non-photosynthetic tissues via phloem-mediated long distance transport in most plants (Lambers et al., 1998). Newly emerging leaves, being sink tissues, are net importers of assimilates. They pass through developmental stages of varying durations according to the species until they assume the role of net exporters of assimilates. Such development involves structural and physiological changes, in which the participation of enzymes in carbohydrate metabolism is noteworthy (Foyer and Paul, 2001). Normal leaf formation and maturation is critical not only to growth, development and defense responses of plants themselves but to productivity in agriculture.
Sucrose metabolism plays fundamental roles in plant leaf development. In the past three decades, rapid progress has been achieved in understanding the dynamics of sucrose-metabolizing enzymes during leaf development (Turgeon, 1989; Foyer and Paul, 2001; Ruan, 2014). Sucrose-metabolizing enzymes are functionally divided into cleavage and synthesis groups. The cleavage group includes invertase and sucrose synthase (Sus). The former hydrolyzes sucrose irreversibly into two reducing hexose sugars (glucose and fructose), whereas the latter cleaves sucrose reversibly in the presence of UDP into UDP-glucose (UDPG) and fructose. According to subcellular locations and optimum pH, invertase is classified into cell-wall, vacuolar, and cytoplasmic isoforms, designated CWI, VIN and NIN, respectively (Tymowska-Lalanne and Kreis, 1998). CWI and VIN are characterized by an acidic pH optimum (3.5–5.5), whereas NIN by an alkaline or neutral pH optimum (6.8–8.0) (Roitsch and González, 2004). In nearly all plant species explored to date (Schaffer et al., 1987; Singh and Luthra, 1988; Huber, 1989; Turgeon, 1989; Ricaud et al., 1994; Alaoui-Sossé et al., 1996; Ruan, 2014), VIN is the major sucrose-cleaving enzyme during leaf development whereas NIN and Sus appear much less important. Sucrose synthesis is catalyzed by two distinct enzymes in higher plants: sucrose phosphate synthase (SPS) and Sus. Using UDPG and Fructose-6-P as substrates, SPS synthesizes sucrose-6-P that is then dephosphorylated into sucrose by sucrose phosphate phosphatase (SPP) (Huber and Huber, 1996). Sus synthesizes sucrose exactly in the reverse reaction as it cleaves sucrose. Generally, SPS rather than Sus is regarded as the main enzyme responsible for sucrose synthesis in leaves (Winter and Huber, 2000; Ren and Zhang, 2013; Wang et al., 2013). In developing leaves, sucrose-cleaving enzymes, especially VIN, are very active; they contribute to rapid leaf growth by providing high amounts of reducing sugars as substance to form cell carbon skeletons and fuel various energy-consuming mechanisms (Pantin et al., 2012). In mature leaves, however, sucrose-cleaving enzymes usually maintain much lower activities compared to young leaves, whereas sucrose-synthesizing enzymes, especially SPS, exhibit high activities. In recent years, transgenic studies have been conducted to explore the effects of sucrose metabolizing enzymes on leaf development. In transgenic cotton overexpressing a potato Sus gene, expansion of young leaves is accelerated with significantly increased fructose concentrations and a slight decline for sucrose (Xu et al., 2012). Similar results are observed in transgenic tobacco overexpressing a loquat VIN (Wang et al., 2015). Existing knowledge on sucrose-metabolizing enzymes during leaf development has mainly been drawn from studies on temperate species (Huber, 1989; Foyer and Paul, 2001; Roitsch and González, 2004; Ruan, 2014). It is hence worthwhile considering whether the general understanding thus acquired could be extended to the entire plant kingdom.
The rubber tree (Hevea brasiliensis; Hevea hereafter) is an important tropical species, being the sole commercial source of natural rubber (cis-1,4-polyisoprene) (van Beilen and Poirier, 2007). Hevea uses sucrose as the precursor molecule for rubber biosynthesis and latex regeneration in rubber-producing laticifers (Tupy, 1989). Physiological and molecular regulation of sucrose metabolism has been intensively studied in relation to rubber production in the laticifers of the Hevea trunk, which is the site tapped for latex (Tupy, 1989; Dusotoit-Coucaud et al., 2010; Tang et al., 2010; Liu et al., 2015, 2016). By comparison, very few studies are available with regard to sucrose metabolism in Hevea leaves, although the co-existence of VIN and NIN isoforms has been shown in both growing and developed Hevea leaves (de Oliveira et al., 2006). Hevea leaf development has been investigated mainly with respect to leaf diseases (Lieberei et al., 1989; Voss, 2001; Kursar and Coley, 2003; Lieberei, 2007), especially South American leaf blight (SALB), a fungal disease that has hampered Hevea planting in South and Central America. Cyanogenic metabolism, lignin and anthocyanin biosynthesis have been shown to correlate closely with the susceptibility of Hevea leaves during development (Lieberei, 2007; Fang et al., 2016).
This study aimed to look at the correlation between sucrose metabolizing enzymes and transcript levels during four different developmental stages in Hevea leaves in an attempt to fill the gap outside temperate species.
Materials and Methods
Plant Materials
Hevea leaves at four progressive developmental stages (Fang et al., 2016), i.e., bronze (I), color-change (II), pale green (III), and bright green (mature; IV) were sampled at 10 a.m. from untapped 4-year-old tissue-cultured plants of the cultivar Reyan7-33-97 that were cultivated in the experimental plantation of the Rubber Research Institute, Chinese Academy of Tropical Agricultural Sciences (Danzhou, Hainan, China). Three individual trees with similar girth and growth vigor were chosen, each of which was used as a separate biological repeat.
Enzyme Extraction
Leaf soluble proteins were extracted according to the method of Walker and Huber (1989) with slight modifications. Fresh leaves (0.2 g) were ground using a mortar and pestle in 2 mL ice cold extraction buffer containing 50 mM HEPES-NaOH (pH 7.5), 1 mM EDTA, 5 mM Mg2+, 10 mM β-mercaptoethanol, 0.05% (v/v) Triton X-100 and 1% PVP40 (w/v). The homogenate was centrifuged at 20,000 g for 10 min. The supernatant was desalted and made free of soluble sugars on a Sephadex G-25 column equilibrated with extraction buffer minus the Triton X-100 and PVP40 prior to four enzyme assays, viz. VIN, NIN, Sus, and SPS.
To extract CWI, the centrifugation pellet was suspended in 100 μL of 1 M NaCl and 400 μL of extraction buffer, and kept at 4°C overnight. The suspension was centrifuged at 20,000 g for 10 min with the supernatant used for the CWI assay.
Protein concentrations of enzyme extract were determined according to the method of Bradford (1976).
Enzyme Assays
Invertase activities were determined as described previously with modifications (Tupý, 1969; Miron and Schaffer, 1991). Assays were tested in a reaction mixture of 100 μL containing 30 μL enzyme extract and 100 mM sucrose in the reaction buffer (50 mM HEPES, 5 mM Mg2+ and 1 mM EDTA). The pHs of the reaction buffers were adjusted to 7.5, 4.5, and 3.5, respectively, for the three invertases: NIN, VIN and CWI. The mixture was chilled on ice immediately after incubation at 37°C for 1 h and then adjusted to 1mL by adding 900 μL of 3, 5-dinitrosalicylic acid reagent (DNS), followed by 5 min of incubation in a boiling water bath. The blank control contained 30 μL of boiled enzyme extract. Contents of reducing sugars produced in the reaction mixture were measured using dinitrosalicylic acid reagent in conformity with Miller (1959). Enzyme activity was expressed in units per mg of protein, where one unit (U) was defined as 1 μmol of sucrose cleaved per hour under the experiment conditions.
The activity of SPS was determined by measuring the sucrose (plus sucrose-6-P) formed in the assay mixture (100 μL) that contained 30 μL enzyme extract, 5 mM UDPG, and 40 mM fructose-6-P in the reaction buffer (50 mM HEPES, 5 mM Mg2+ and 1 mM EDTA, pH 6.5) (Huber, 1981). After incubation at 37°C for 1 h, 100 μL of 1 M NaOH was added to terminate the reaction and destroy the unreacted hexose simultaneously by placing the tubes in boiling water for 10 min. The blank differed from the assay mixture by lacking UDPG, and it was kept on ice prior to the addition of NaOH. After chilling on ice, 250 μL of 0.1% (w/v) resorcinol in 95% ethanol and 750 μL of 30% HCl were added to each tube, followed by incubation at 80°C for 10 min. The tubes were then centrifuged at 20,000 g for 5 min, and the OD520 was measured by a Spectramax 250 microplate reader (Molecular Devices, Sunnyvale, CA, United States). Enzyme activity was expressed in units per mg of protein, where one unit (U) was defined as 1 μmol of sucrose produced per hour.
Sus catalyzes a reversible reaction that both hydrolyzes and synthesizes sucrose. Sus activity in the synthesis direction was assayed as for SPS except that the pH of reaction buffer was changed to 8.5, and fructose-6-P substituted with fructose. Sus activity in the cleavage reaction was assayed as for invertase except that the pH of reaction buffer was changed to 6.5, and 5 mM UDP was supplemented. The blank control contained no UDP. Enzyme activities were expressed in units per mg of protein, where one unit (U) was defined as 1 μmol of sucrose produced or cleaved per hour.
In line with standard practices in enzymology, blanks are expected to contain substrates as well. However, leaving out the substrates UDPG (SPS, SSS) or UDP (SSC) from the blanks did not alter the OD520 readouts (Supplementary Table S1). Accordingly, HPLC-ELSD analysis of the UDPG and UDP substrates showed complete absence of sucrose and fructose (Supplementary Figure S1).
Soluble Sugar Extraction
Leaves were desiccated at 105°C for 30 min and then at 80°C to constant weight. The dried leaves were ground into powder using a mortar and pestle in room temperature, and kept in a dryer prior to use. Soluble sugars were extracted according to Ma et al. (2014) with modifications. Approximately 100 mg was sonicated in 800 μL double-distilled water (ddH2O) for 30 min and then incubated at 80°C for 30 min with intermittent shaking for every 5 min. The mixture was centrifuged at 4°C at 20,000 g for 10 min. The supernatant was kept at -20°C, whereas the pellet was subjected to a second round of extraction with 200 μL of ddH2O. The supernatant pooled from the two rounds of extraction was dried under vacuum at 60°C, then dissolved in 80% ethanol (v/v) and kept at -20°C overnight. The suspension was centrifuged at 4°C in 20,000 g for 10 min. The supernatant was dried again, dissolved in 50% acetonitrile (v/v), and stored at -20°C before use.
To prepare phloem exudate, 1-year-old Hevea branches bearing mature leaves were excised with a sharp knife, and the cuts were cleaned with sterile water, and then subjected to the extraction of phloem exudate following the protocol of King and Zeevaart (1974). The exudates obtained were lyophilized, and dissolved in 50% acetonitrile (2 mL per branch), and stored at -20°C before use.
Soluble Sugar Analysis by HPLC-ELSD
The high-performance liquid chromatography (HPLC)-evaporative light scattering detection (ELSD) method was used to determine soluble sugars in Hevea leaves and phloem exudate. HPLC analysis was performed on a Waters e2695 separations module (Waters, Milford, MA, United States) equipped with an Alltech 3300 ESLD detector (Alltech, Deerfield, IL, United States). Separation was achieved on a XBridgeTM Amide column [4.6 mm × 250 mm i.d., 3.5 μm particle size (Waters, Milford, MA, United States)]. All samples and standards were filtered through 0.45 μm Millipore filters before loading samples of 10 μL onto the machine. The HPLC-ELSD conditions were optimized following Ma et al. (2014) with a solvent ratio of 85 acetonitrile:15 water (v/v), a flow rate of 1 mL/min, the column and drift tube temperatures set at 45 and 82°C, respectively, and the nebulizer gas flow rate set at 2 L/min. Peaks were quantified using calibration standards of HPLC grade sugars, viz. glucose, fructose, sucrose, raffinose, and stachyose (Sigma–Aldrich, Shanghai, China), and sorbitol and quebrachitol (Shanghai Yuan ye Bio-Technology Co., Ltd., Shanghai, China).
Analysis of Starch Content
Starch extraction and content assay in Hevea leaves were performed following the procedures of the Total Starch Assay Kit (Megazyme, Bray, Ireland).
RNA Isolation and cDNA Synthesis
Total RNA of Hevea leaves was extracted using the Plant Total RNA Extraction Kit (BioTeke Corporation, Beijing, China) following the manufacturer’s instructions. RNA integrity was checked by agarose gel electrophoresis while RNA concentration and purity were examined using a NanoDrop 2000 spectrophotometer (Thermo Fisher Scientific, Wilmington, DE, United States). RNA samples were prepared DNA-free, and reverse transcribed to cDNA using the PrimeScriptTM First Strand cDNA Synthesis Kit (TaKaRa, Dalian, China) following the manufacturer’s protocol.
Gene Expression Profile Based on RNA-Seq Analysis
The RNA-Seq data of Hevea leaves at four developmental stages (I to IV) in our previous study (Fang et al., 2016) were divided into two sets: developing (stage I to III) and mature (stage IV). The two data sets were exploited to determine the expression in FPKM (Fragments Per Kilobase of transcript per Million fragments mapped) of sucrose-metabolizing genes in developing and mature leaves by using the RSEM software (Li and Dewey, 2011).
Quantitative Real-Time PCR Analysis
Specific PCR primers for the main sucrose-metabolizing genes (Supplementary Table S2) were designed by Primer Premier 5, and their specificity was confirmed by agarose electrophoresis and sequencing of the PCR products. Quantitative real-time PCR (qPCR) was carried out using the SYBR Premix Ex Taq II (2×; Tli RNaseH Plus; TaKaRa, Dalian, China) on a CFX96 TouchTM Real-Time PCR detection system (Bio-Rad, Hercules, CA, United States). Quantification cycle values, PCR efficiency, correlation coefficients and the relative fold change of expression were determined as previously described (Long et al., 2015, 2016). The Hevea YLS8 gene was selected as an internal control based on the results of our previous work (Li et al., 2011).
Statistical Analysis
All statistical analyses were conducted using SPSS Statistics 22. Duncan’s test was used to compare sugar concentrations, enzyme activity, and gene expression in Hevea leaves across the four developmental stages. Differences were accepted as significant at P < 0.05. Correlation coefficients were determined between activity of sucrose-metabolizing enzymes and soluble sugar contents or gene expression in the course of Hevea leaf development. Correlation was accepted as significant at P < 0.05 or 0.01.
Results
Changes in Specific Leaf Weight during Leaf Development
In the process of Hevea leaf development, leaf colors changed from bronze (stage I) to color change (stage II), pale-green (stage III), and then to bright green (stage IV), identical to our previous report (Fang et al., 2016). Leaf size (cm2) increased rapidly from stages I (9.6 ± 0.6) to III (83.0 ± 10.6), and remained unchanged thereafter (Table 1). On the contrary, specific leaf weight (mg fresh weight per cm2) decreased progressively from stages I (12.5 ± 1.0) to III (9.8 ± 1.7), but resumed to a high value of 12.9 ± 1.5 in mature leaves (stage IV) (Table 1).
Changes in Sugar Composition during Leaf Development
To determine soluble sugar composition in Hevea leaves and branch phloem exudates, the HPLC-ELSD system was used. Six sugars, namely glucose, fructose, sorbitol, sucrose, raffinose, and stachyose that are reported to be abundant or translocated in most plant species (Zamski and Schaffer, 1996) served as reference standards, together with quebrachitol which is the predominant non-rubber carbon compound in Hevea latex (Bealing, 1981). Raffinose and stachyose, easily separated from the five other sugars by the HPLC-ELSD method (Supplementary Figure S2A), were not detected in Hevea leaves and phloem exudate. Glucose, fructose, sucrose, sorbitol, and quebrachitol were well separated in 28 min by the optimized HPLC-ELSD method (Supplementary Figure S2B). Fructose, glucose, and sucrose turned out to be the main soluble sugars in Hevea leaves although their relative abundance varied with leaf development (Supplementary Figures S3A–D). Interestingly, Hevea leaves contained also small amounts of quebrachitol, but no sorbitol. Hevea phloem exudate from branches exhibited a profile of sugar composition similar to that of the leaf (Supplementary Figure S3E), except that sucrose was in far greater abundance than the other sugars, consistent with a previous assumption of sucrose being the major transported carbohydrate in Hevea (Tupy, 1989).
Changes in leaf contents of glucose, fructose, sucrose, and starch during leaf development are depicted in Figure 1. Starch accumulated in the course of leaf development, with a 2.5-fold increase from stage I to stage IV. At all leaf stages, however, starch was much lower than the other three sugars. In mature leaves (stage IV), starch comprised only 5.7% of total sugars, whereas sucrose accounted for 63.5%. Glucose and fructose had comparable concentrations except for stage III where the content of fructose was almost double that of glucose. Concentrations of fructose and glucose increased progressively with leaf development, and their combined content was more than 1.5-fold that of sucrose, but then decreased abruptly at maturation to less than 0.5-fold that of sucrose at the same stage (Figure 1). The content of sucrose remained almost unchanged from stage I to II, and then increased noticeably at stage III, before peaking at maturation (stage IV).
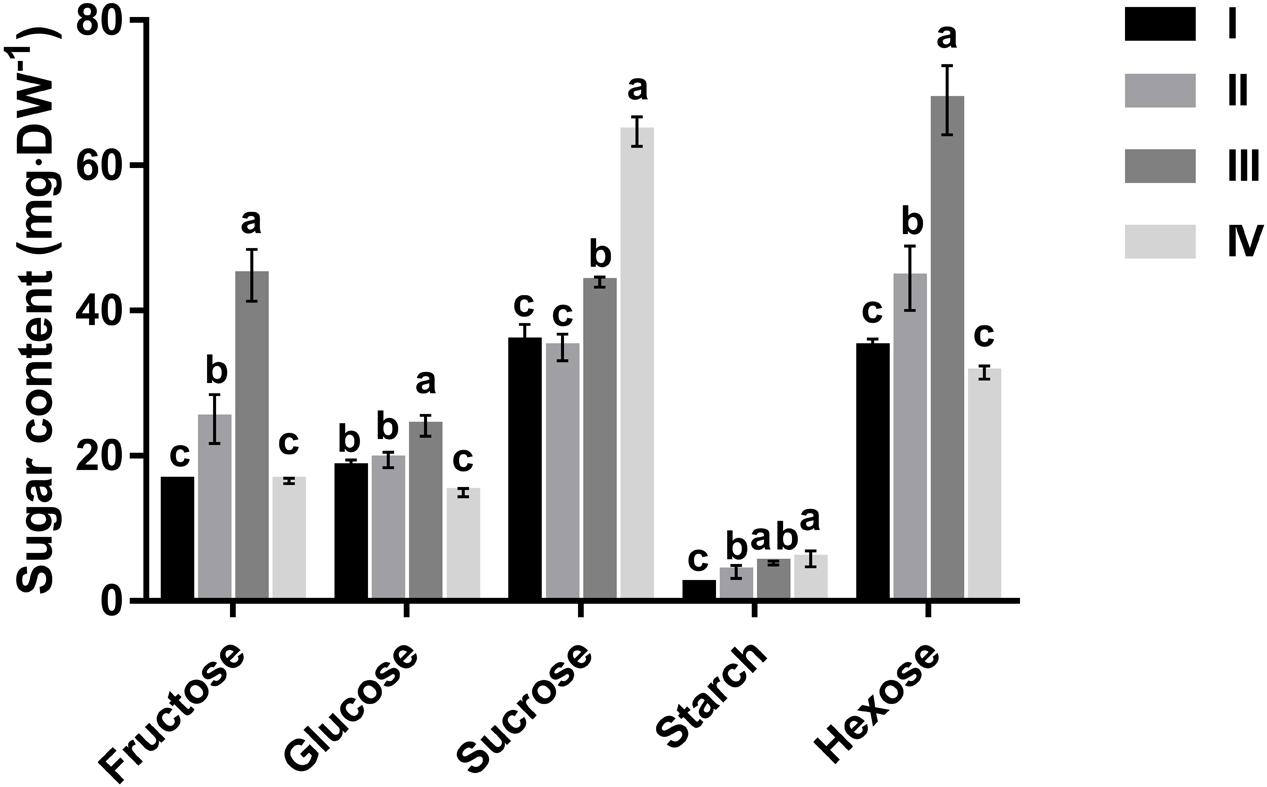
FIGURE 1. Changes in fructose, glucose, sucrose, starch and hexose concentrations of Hevea leaves at four developmental stages (I to IV). Values are the means ± SE of three replicates. Different letters above the bars of respective sugars indicate a significant difference (P < 0.05) across different developmental leaf stages.
Activity of Sucrose-Metabolizing Enzymes during Leaf Development
All sucrose-cleaving enzymes, i.e., VIN, NIN, CWI, and Sus in the cleavage direction (SSC) showed comparable activities in developing leaves (stages I to III) that were significantly higher than in mature leaves (stage IV) (Figure 2). Higher activity of sucrose-cleaving enzymes corresponded well to a higher hexose content in developing leaves (Figure 1). Examined in detail, the four sucrose-cleaving enzymes exhibited obvious variation in activity. VIN activity increased almost two-fold between stages I and II, and then decreased progressively to stage IV, reaching a level of only one fourth of its highest at stage II (Figure 2A). NIN activity remained constantly high throughout the three developing stages (I to III), but decreased significantly by 40% at maturation (stage IV) (Figure 2B). CWI and SSC activities shared a similar pattern of variation, both of which increased progressively with leaf development until stage III, and then declined abruptly at stage IV by 50% and 80%, respectively (Figures 2C,D). In mature leaves, NIN and CWI activities were significantly higher than those of VIN and SSC. Interestingly, sucrose-synthesizing enzymes, i.e., SPS and Sus in the synthesis direction (SSS) behaved similarly to sucrose-cleaving enzymes by displaying higher activity in developing leaves than in mature ones (Figures 2E,F). Correlations between activities of sucrose-metabolizing enzymes and the soluble sugar content were investigated in Hevea leaves during their development (Table 2). CWI activity correlated positively with fructose content at a significant level, whereas SSC and SSS activities showed significant positive correlation with glucose content. Interestingly, NIN activity exhibited a significant negative correlation with sucrose content, suggesting that the extent of its cleavage by NIN influenced its presence in the leaf.
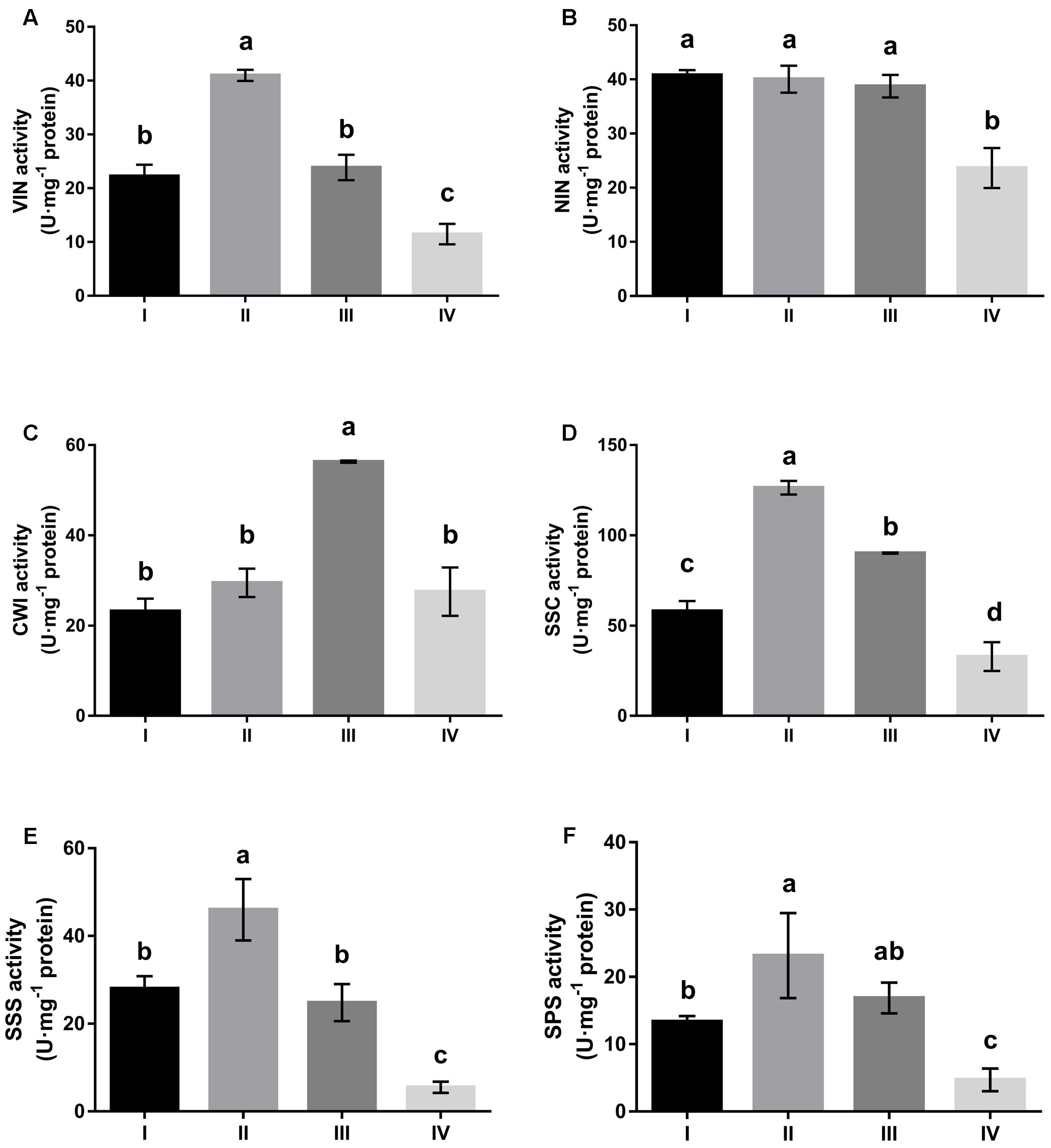
FIGURE 2. Changes in activities of sucrose-metabolizing enzymes at four stages of Hevea leaf development. (A) Vacuolar invertase (VIN); (B) alkaline/neutral invertase (NIN); (C) cell wall invertase (CWI); (D) sucrose synthase in the cleavage direction (SSC); (E) sucrose synthase in the synthesis direction (SSS); (F) sucrose phosphate synthase (SPS). Values are the means ± SE of three replicates. Different letters above the bars indicate a significant difference with P < 0.05 across different developmental leaf stages.

TABLE 2. Correlation coefficients between activity of sucrose-metabolizing enzymes and soluble sugar content in the course of Hevea leaf development.
Expression of Sucrose-Metabolizing Enzymes during Leaf Development
To investigate the transcriptional regulation of sucrose-metabolizing enzymes and their roles in Hevea leaf development, the principal encoding genes expressed in developing and mature leaves were first identified among respective gene families of sucrose-metabolizing enzymes (VIN, NIN, CWI, SPS and Sus) using the RNA-Seq data (Figure 3). The main members thus identified from different gene families, viz. HbVIN1 to 3, HbNIN1, 2, 6 and 8, HbCWI1 to 3, HbSus2 to 5, and HbSPS2 and 3 were explored for their expression dynamics with leaf development by qPCR analysis (Figure 4).
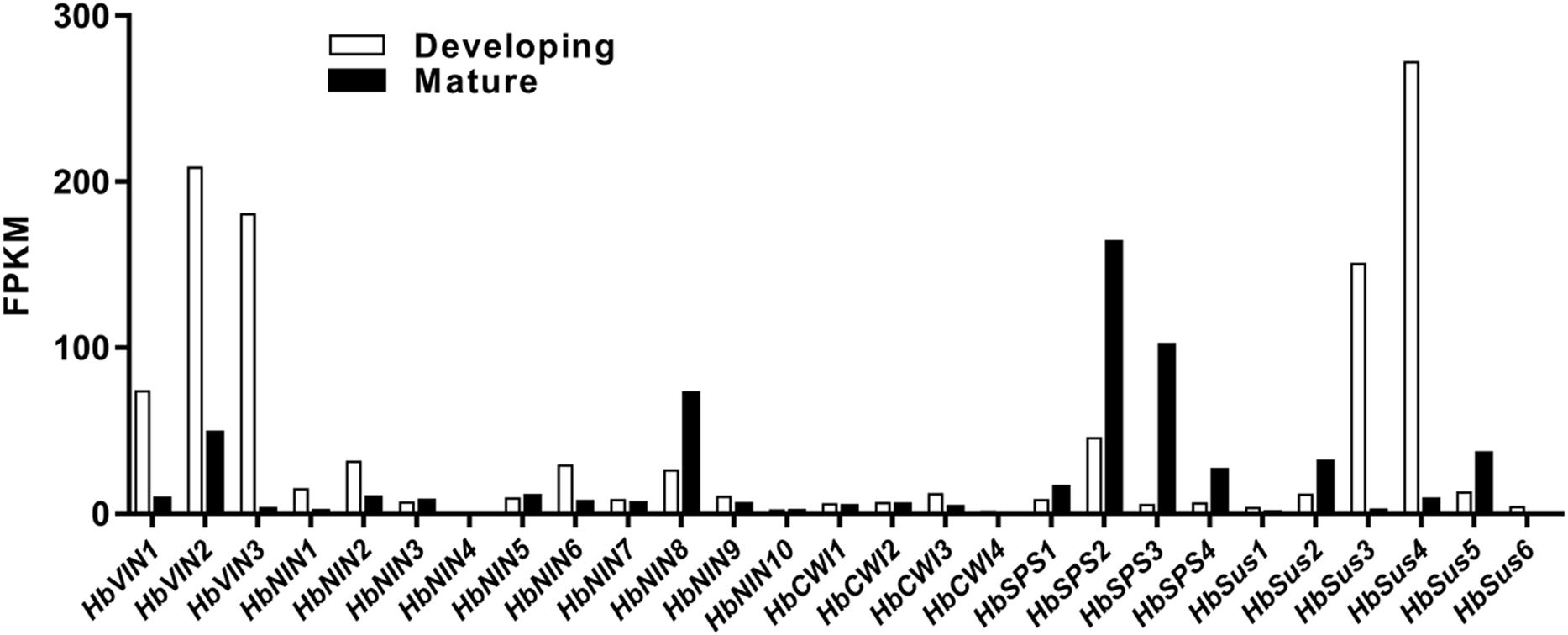
FIGURE 3. Expression of sucrose-metabolizing genes in developing and mature Hevea leaves determined by RNA-Seq analysis. Expression of the respective genes is represented in Fragments Per Kilobase of transcript per Million mapped reads (FPKM). Values for developing leaves are means of stages I to III.
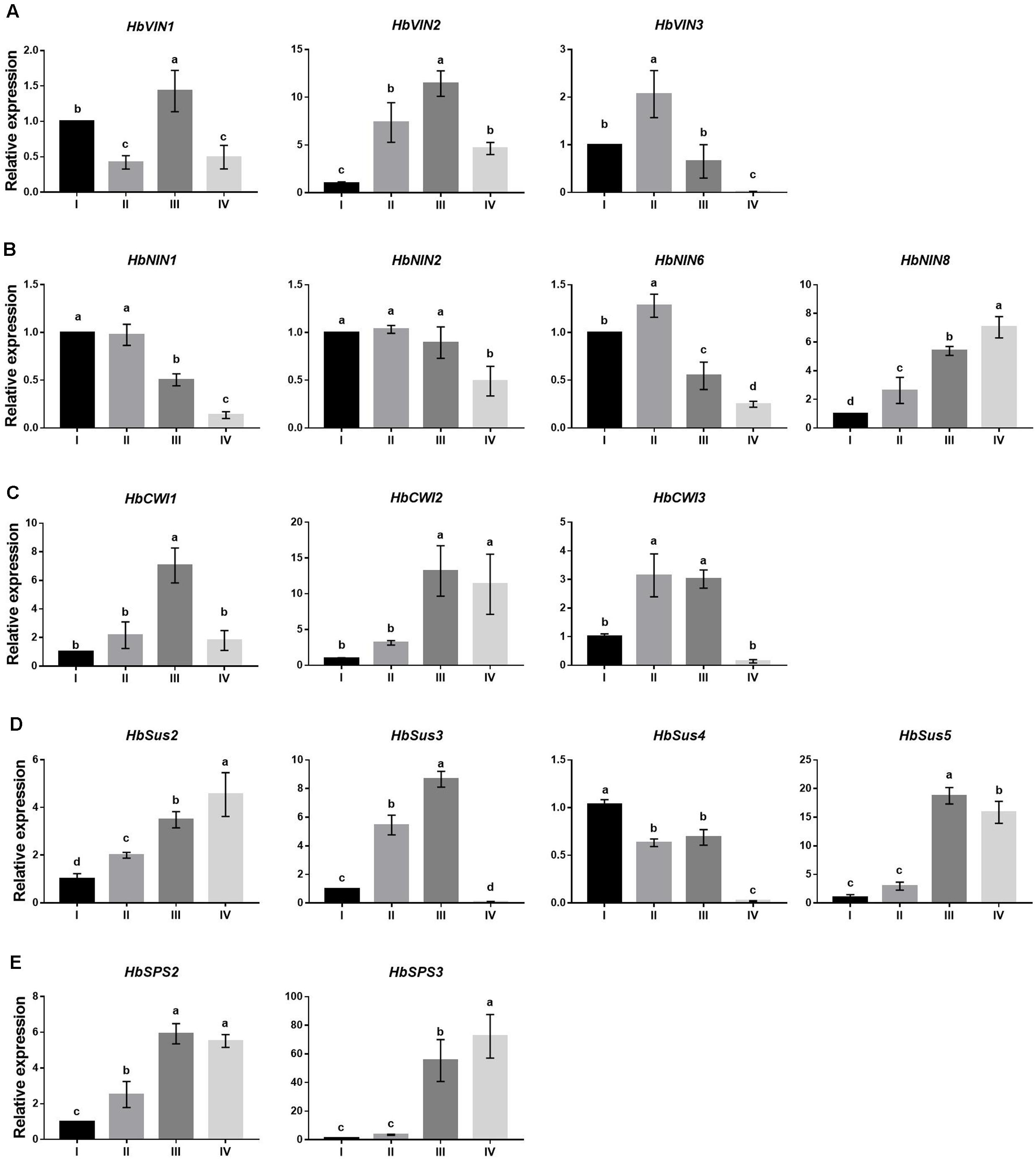
FIGURE 4. Changes in expression by qPCR of sucrose-metabolizing genes at four stages (I to IV) of Hevea leaf development. (A) Three vacuolar invertase genes, HbVIN1, 2 and 3; (B) four alkaline/neutral invertase genes, HbNIN1, 2, 6 and 8; (C) three cell-wall invertase genes, HbCWI1, 2 and 3; (D) four sucrose synthase genes, HbSus2 to 5; (E) two sucrose phosphate synthase genes, HbSPS2 and 3. Expression of respective genes was normalized against the level at leaf stage I. Values are means ± SE of three replicates. Different letters above the bars indicate a significant difference with P < 0.05 across different developmental leaf stages.
Expressions of all three HbVIN genes in Hevea leaves decreased significantly between stages III and IV (Figure 4A), corresponding well to an abrupt depression in VIN activity at stage IV (Figure 2A). However, the three HbVIN genes exhibited variation during the three developing leaf stages (I to III), with a constant rise for HbVIN2 but different fluctuations for HbVIN1 and 3. Except for HbNIN8 that accumulated transcripts throughout the leaf development, the other three HbNIN genes analyzed, namely HbNIN1, 2 and 6 showed consistently higher expressions in developing leaves (stages I to III) than in mature leaves (stage IV) (Figure 4B), consistent with the higher NIN activity observed in developing leaves (Figure 2B). All three HbCWI genes displayed high expressions at stage III, but expression decreased significantly at stage IV in HbCWI1 and 3 (Figure 4C); this trend was consistent with the changes in CWI activity (Figure 2C). The four HbSus genes displayed different patterns of expression during the four developmental leaf stages (Figure 4D). Expressions of both HbSus2 and 3 increased constantly from stages I to III. At stage IV, HbSus3 decreased abruptly to a barely detectable level whereas HbSus2 increased further. Expression of HbSus4 was the highest at stage I, and maintained high levels through stages II and III, but decreased significantly to a very low level at stage IV. HbSus5 expression was much higher at late stages (III and IV) than the early stages (I and II). Both HbSPS2 and 3 exhibited high gene activity in mature leaves at stage IV (Figure 4E), despite the lowest SPS enzyme activity observed at this stage (Figure 2F). Correlation analyses revealed that among the 16 sucrose-metabolizing related genes explored, expressions of only three genes, viz. HbNIN2, HbCWI1 and HbSus3, correlated significantly with the activities of their respective enzymes (Table 3).
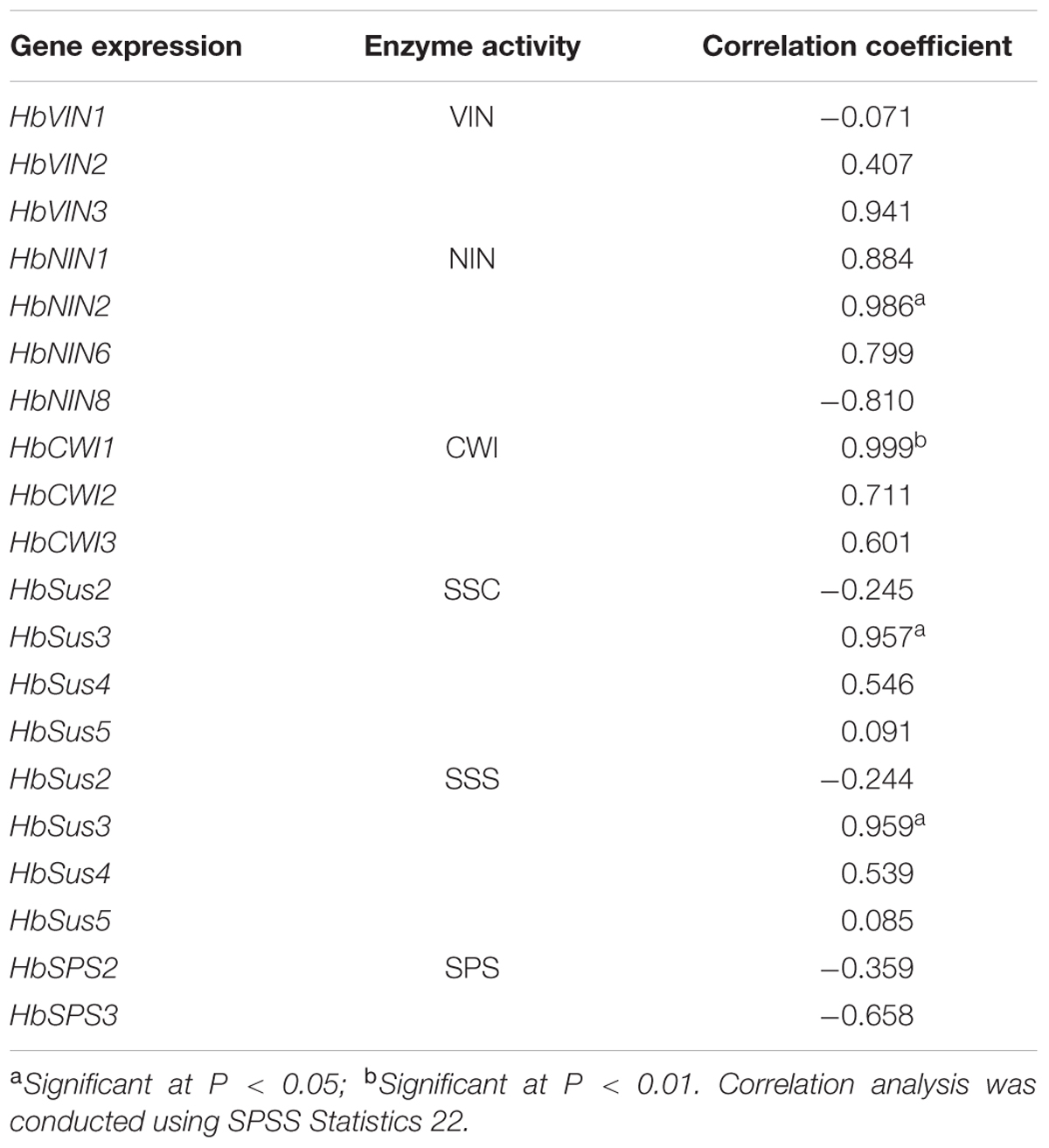
TABLE 3. Correlation coefficients between enzyme activity and gene expression in the course of Hevea leaf development.
Discussion
Variation in the contents of different sugars during Hevea leaf development is consistent with the strong requirement of reducing sugars for energy and carbon skeletons in developing leaves (representing a sink), and the functions of mature leaves (source) that export excess photoassimilates mainly in the form of sucrose in higher plants (Turgeon, 1989). Hevea leaf area increased almost exponentially in the three developing stages, and peaked at stage III (pale-green) (Table 1). Similarly, hexose content of Hevea leaves increased with the leaf development, and also reached the maximum at stage III, being 1.57 times that of sucrose content (Figure 1). Such a leaf development requires a massive influx of water into the vacuoles (Pantin et al., 2012), and the high hexose contents observed in expanding Hevea leaves would facilitate the entry of water to establish high cell turgor pressure for rapid leaf expansion. A similar observation has been reported in immature grapevine leaves where the hexose content that represented more than 75% of the total carbohydrates decreased almost three fold in mature leaves (Patakas, 2000). High hexose contents are beneficial to the developing leaf as they are essential for fast cell division and expansion (da Silva Bonome et al., 2011). Moreover, the high hexose concentrations meet the requirement of a controlled cell-wall polymer synthesis that contributes to the extension of the pre-existing cell wall to accommodate cell growth (Cosgrove, 2005). High levels of hexose in developing leaves are due to the high activities of four types of sucrose-cleaving enzymes, i.e., VIN, NIN, CWI and Sus in the cleavage direction (SSC) (Figure 2). Similar results are obtained in peach, a sorbitol synthesizing species, where activities of VIN, NIN and SSC and the hexose content were the highest in the first rapidly expanding leaf (Merlo and Passera, 1991).
A common feature among sucrose-metabolizing enzymes in the course of Hevea leaf development is that these enzymes tended to be highly expressed in young leaves, with expression declining significantly at maturation, regardless of whether the enzymes were functioning in sucrose synthesis or cleavage (Figure 2). A similar trend has also been observed in eggplants, cassava, grapevine, sugar cane and maize (Claussen et al., 1985), lemongrass (Singh and Luthra, 1988), chicory (Gupta et al., 1991), common oak (Alaoui-Sossé et al., 1996), and sugar beet (Pavlinova et al., 2002). The synchronized change of sucrose cleavage and synthesis activity facilitates the keeping of sucrose levels within an appropriate range in Hevea leaves, an essential function critical for normal plant development (Yadav et al., 2014). In plant leaves, SPS activity is usually the rate-limiting enzyme for sucrose synthesis, whereas Sus activity is mainly involved in sucrose catabolism (Winter and Huber, 2000). At different developmental stages of Hevea leaves, however, Sus activity in the synthesis direction (SSS) was comparable or even higher than SPS (Figure 2). Of the four HbSus genes examined, expressions of HbSus3 exhibited a significant positive correlation with both SSS and SSC enzyme activities (Table 3). On the other hand, expressions of neither HbSPS gene explored were consistent with the observed enzyme activity (Figures 2F, 4E and Table 3). This phenomenon where gene activity is not reflected in enzyme activity has also been observed in other plant species. For example, in ‘La France’ pear leaves (Suzue et al., 2006), SPS activity remained almost constant throughout the developmental stages although SPS mRNA levels are much higher in mature leaves than in young ones. Post-translational modification of the enzyme may play an essential role in controlling leaf SPS activity (Doehlert and Huber, 1983; Walker and Huber, 1989; Zhou et al., 2002).
One apparent discrepancy between Hevea and other plant species lies in the activities of NIN. In other plants reported so far, VIN is always the primary enzyme responsible for sucrose catabolism in developing or mature leaves, whereas NIN activities are much lower or even undetectable in leaves (Singh and Luthra, 1988; Huber, 1989; Alaoui-Sossé et al., 1996; Winter and Huber, 2000). For example, VIN activity was reported to be much higher in young leaves than in mature ones in many other plant species, including lemongrass (Singh and Luthra, 1988), common oak (Alaoui-Sossé et al., 1996), sugar beet (Pavlinova et al., 2002), and pear (Suzue et al., 2006). In this study, however, NIN exhibited activities comparable to the other three sucrose-cleaving enzymes (VIN, CWI and SSC) throughout all leaf stages (Figure 2), suggesting an active participation of NIN in Hevea leaf development. Of the four sucrose-cleaving enzymes, only NIN exhibited a negative correlation at a significant level for its expression with sucrose concentration in developing Hevea leaves, further corroborating its roles in this process (Table 2). Of the ten NIN genes identified in Hevea (Liu et al., 2015), eight displayed substantial expression in developing and/or mature leaves (Figure 3), providing additional evidence for the active roles of NIN in leaf development in the rubber tree.
Starch and sucrose are the principal photoassimilates accumulated in leaves of higher plants. However, the relative abundance of starch versus sucrose varies greatly among species. Among ten temperate grass species explored by Huber (1989), most had higher leaf starch content, and the ratios of starch to sucrose varied from about 22.0 in a soybean cultivar to about 0.5 in a pea cultivar. Similarly, of thirteen C4 species investigated (Lunn and Hatch, 1995), only two accumulated sucrose comparable to starch in their leaves during the day, whereas the others accumulated much more starch than sucrose. In mature leaves of young common oak, starch content was also much higher than sucrose (Alaoui-Sossé et al., 1996). Results from the present study showed that mature Hevea leaves had a sucrose:starch ratio of ∼11.0 (Figure 1), the highest value as compared with other plants reported to date. The leaves of cassava, another economically important tropical species within the same Euphorbiaceae family as Hevea, had a sucrose:starch ratio of ∼5.0 (Zhang et al., 2015). Of the grass species, timothy, an important cool-season herbage also showed a high sucrose:starch ratio of ∼6.0 in its leaves at stages of both heading and anthesis (Ould-Ahmed et al., 2017). However, this grass species is known to preserve fructans as the major non-structural carbohydrates in its leaves (Bélanger and McQueen, 1998). It is worth noting that leaf contents of both sucrose and starch exhibited a diurnal change in Arabidopsis (Smith et al., 2004), and the time of harvesting, therefore, might influence the sucrose:starch ratio in Hevea leaves, which were harvested this study at 10:00 am. Hevea leaves contained also small amounts of quebrachitol (Supplementary Figure S3), a cyclic polyol with medicinal uses reported in a small number of plant species (Díaz et al., 2008). Considering the high abundance [(about 1.2% (w/v)] of quebarchitol in Hevea latex (Bealing, 1969) and the presence of laticifers in the leaves, latex might be the source of the quebrachitol detected in Hevea leaves. The physiological functions of quebrachitol are still unclear in Hevea although a quebrachitol transporter (HbPLT2) has been identified in this species, and its expression was induced by ethylene treatment or wounding of the bark in a study (Dusotoit-Coucaud et al., 2010).
To conclude, this study provides the first atlas of developmental dynamics in activity and gene expression of sucrose-metabolizing enzymes as well as contents of various sugars in the leaves of Hevea, a tropical tree species. Although the behavior of certain genes and enzymes connected with sucrose metabolism mirrors that of other plants species, several novel characters show up. Unlike in most other species where VIN is the major sucrose-cleaving enzyme in developing leaves, NIN and Sus in the cleavage direction are no less prominent in Hevea. Whereas SPS is mainly responsible for sucrose synthesis in the leaves of most plant species, Sus in the synthesis direction is comparable or even higher than that of SPS in the different developmental stages of Hevea leaves. The findings obtained here would help in the understanding of the regulation of sucrose metabolism during leaf development in the plant kingdom and in the rubber tree in particular.
Author Contributions
JZ conducted most of the experiments and data analysis, and drafted the primary manuscript. JQ coordinated the project. JHL helped develop the HPLC-ESLD method. XX and YF participated in gene expression analysis. JXL contributed in pilot studies. CT conceived the study, designed the experiments, and modified the manuscript. All the authors read and approved the manuscript.
Funding
This work was supported partially by the Natural Science Foundation of China (31570672 and 31400565), and the Fundamental Research Funds for Rubber Research Institute, CATAS (1630022015003).
Conflict of Interest Statement
The authors declare that the research was conducted in the absence of any commercial or financial relationships that could be construed as a potential conflict of interest.
The reviewer HCJvR and handling Editor declared their shared affiliation.
Acknowledgments
The authors thank Prof. Dr. Hoong-Yeet Yeang for correcting the manuscript and valuable comments.
Supplementary Material
The Supplementary Material for this article can be found online at: https://www.frontiersin.org/articles/10.3389/fpls.2018.00058/full#supplementary-material
References
Alaoui-Sossé, B., Ricaud, S., Barnola, P., and Dizengremel, P. (1996). Rhythmic growth and carbon allocation in Quercus robur. Sucrose metabolizing enzymes in leaves. Physiol. Plant 96, 667–673. doi: 10.1111/j.1399-3054.1996.tb00241.x
Bealing, F. (1969). Carbohydrate metabolism in Hevea latex—availability and utilisation of substrates. J. Rubb. Res. Inst. Malasiya 21, 445–455.
Bealing, F. (1981). Quebrachitol synthesis in Hevea brasiliensis. J. Rubb. Res. Inst. Malasiya 29, 111–122.
Bélanger, G., and McQueen, R. E. (1998). Analysis of the nutritive value of timothy grown with varying N nutrition. Grass Forage Sci. 53, 109–119. doi: 10.1046/j.1365-2494.1998.5320109.x
Bradford, M. M. (1976). A rapid and sensitive method for the quantitation of microgram quantities of protein utilizing the principle of protein-dye binding. Anal. Biochem. 72, 248–254. doi: 10.1016/0003-2697(76)90527-3
Claussen, W., Loveys, B., and Hawker, J. (1985). Comparative investigations on the distribution of sucrose synthase activity and invertase activity within growing, mature and old leaves of some C3 and C4 plant species. Physiol. Plant 65, 275–280. doi: 10.1111/j.1399-3054.1985.tb02395.x
Cosgrove, D. J. (2005). Growth of the plant cell wall. Nat. Rev. Mol. Cell Biol. 6, 850–861. doi: 10.1038/nrm1746
da Silva Bonome, L., Moreira, S., de Oliveira, L., and de Jesus Sotero, A. (2011). Metabolism of carbohydrates during the development of seeds of the brazilian rubber tree [Hevea brasiliensis (Willd. Ex Adr. de Juss) Muell.-Arg.]. Acta Physiol. Plant 33, 211–219. doi: 10.1007/s11738-010-0540-8
de Oliveira, P. D., de Oliveira, L. E. M., and Delú Filho, N. (2006). Optimization of invertase assay conditions in rubber tree plants (Hevea brasiliensis Muell. Arg. Rev. Árvore 30, 687–692. doi: 10.1590/S0100-67622006000500001
Díaz, M., González, A., Castro-Gamboa, I., Gonzalez, D., and Rossini, C. (2008). First record of l-quebrachitol in Allophylus edulis (Sapindaceae). Carbohydr. Res. 343, 2699–2700. doi: 10.1016/j.carres.2008.07.014
Doehlert, D. C., and Huber, S. C. (1983). Regulation of spinach leaf sucrose phosphate synthase by glucose-6-phosphate, inorganic phosphate, and pH. Plant Physiol. 73, 989–994. doi: 10.1104/pp.73.4.989
Dusotoit-Coucaud, A., Porcheron, B., Brunel, N., Kongsawadworakul, P., Franchel, J., Viboonjun, U., et al. (2010). Cloning and characterization of a new polyol transporter (HbPLT2) in Hevea brasiliensis. Plant Cell Physiol. 51, 1878–1888. doi: 10.1093/pcp/pcq151
Fang, Y., Mei, H., Zhou, B., Xiao, X., Yang, M., Huang, Y., et al. (2016). De novo transcriptome analysis reveals distinct defense mechanisms by young and mature leaves of Hevea brasiliensis (Para Rubber Tree). Sci. Rep. 6:33151. doi: 10.1038/srep33151
Foyer, C. H., and Paul, M. J. (2001). Source–sink relationships. Plant Psychol. 78, 519–524. doi: 10.1038/npg.els.0001304
Gupta, A. K., Mann, P., Kaur, N., and Singh, R. (1991). Profiles of enzymes of sucrose metabolism in the leaves of chicory (Cichorium intybus) during development. Plant Sci. 77, 191–196. doi: 10.1016/0168-9452(91)90088-P
Huber, S. C. (1981). Interspecific variation in activity and regulation of leaf sucrose phosphate synthetase. Z. Pflanzenphysiol. 102, 443–450. doi: 10.1016/S0044-328X(81)80179-1
Huber, S. C. (1989). Biochemical mechanism for regulation of sucrose accumulation in leaves during photosynthesis. Plant Physiol. 91, 656–662. doi: 10.1104/pp.91.2.656
Huber, S. C., and Huber, J. L. (1996). Role and regulation of sucrose-phosphate synthase in higher plants. Annu. Rev. Plant Physiol. Plant Mol. Biol. 47, 431–444. doi: 10.1146/annurev.arplant.47.1.431
King, R. W., and Zeevaart, J. A. D. (1974). Enhancement of phloem exudation from cut petioles by chelating agents. Plant Physiol. 53, 96–103. doi: 10.1104/pp.53.1.96
Kursar, T., and Coley, P. (2003). Convergence in defense syndromes of young leaves in tropical rainforests. Biochem. Syst. Ecol. 31, 929–949. doi: 10.1016/S0305-1978(03)00087-5
Lambers, H., Chapin, F. S. III, and Pons, T. L. (ed.). (1998). “Photosynthesis, respiration, and long-distance transport”, in Plant Psychology Ecology (New York, NY: Springer), 10–153. doi: 10.1007/978-1-4757-2855-2_2
Li, B., and Dewey, C. N. (2011). RSEM: accurate transcript quantification from RNA-Seq data with or without a reference genome. BMC Bioinformatics 12:323. doi: 10.1186/1471-2105-12-323
Li, H., Qin, Y., Xiao, X., and Tang, C. (2011). Screening of valid reference genes for real-time RT-PCR data normalization in Hevea brasiliensis and expression validation of a sucrose transporter gene HbSUT3. Plant Sci. 181, 132–139. doi: 10.1016/j.plantsci.2011.04.014
Lieberei, R. (2007). South American leaf blight of the rubber tree (Hevea spp.): new steps in plant domestication using physiological features and molecular markers. Ann. Bot. 100, 1125–1142. doi: 10.1093/aob/mcm133
Lieberei, R., Biehl, B., Giesemann, A., and Junqueira, N. T. (1989). Cyanogenesis inhibits active defense reactions in plants. Plant Physiol. 90, 33–36. doi: 10.1104/pp.90.1.33
Liu, J. P., Zhuang, Y. F., Guo, X. L., and Li, Y. J. (2016). Molecular mechanism underlying ethylene stimulation of latex production in rubber tree (Hevea brasiliensis). Trees 30, 1913–1921. doi: 10.1007/s00468-016-1455-9
Liu, S., Lan, J., Zhou, B., Qin, Y., Zhou, Y., Xiao, X. H., et al. (2015). HbNIN2, a cytosolic alkaline/neutral-invertase, is responsible for sucrose catabolism in rubber-producing laticifers of Hevea brasiliensis (para rubber tree). New Phytol. 206, 709–725. doi: 10.1111/nph.13257
Long, X., He, B., Fang, Y., and Tang, C. (2016). Identification and characterization of the glucose-6-phosphate dehydrogenase gene family in the rubber tree, Hevea brasiliensis: role in latex regeneration and stress response. Front. Plant Sci. 7:215. doi: 10.3389/fpls.2016.00215
Long, X., He, B., Wang, C., Fang, Y., Qi, J., and Tang, C. (2015). Molecular identification and characterization of the pyruvate decarboxylase gene family associated with latex regeneration and stress response in rubber tree. Plant Physiol. Biochem. 87, 35–44. doi: 10.1016/j.plaphy
Lunn, J. E., and Hatch, M. D. (1995). Primary partitioning and storage of photosynthateDS in sucrose and starch in leaves of C 4 plants. Planta 197, 385–391. doi: 10.1007/BF00202661
Ma, C. M., Sun, Z., Chen, C. B., Zhang, L. L., and Zhu, S. H. (2014). Simultaneous separation and determination of fructose, sorbitol, glucose and sucrose in fruits by HPLC-ELSD. Food Chem. 145, 784–788. doi: 10.1016/j.foodchem
Merlo, L., and Passera, C. (1991). Changes in carbohydrate and enzyme levels during development of leaves of Prunus persica, a sorbitol synthesizing species. Physiol. Plant 83, 621–626. doi: 10.1111/j.1399-3054.1991.tb02478.x
Miller, G. L. (1959). Use of dinitrosalicylic acid reagent for determination of reducing sugar. Anal. Chem. 31, 426–428. doi: 10.1021/ac60147a030
Miron, D., and Schaffer, A. A. (1991). Sucrose phosphate synthase, sucrose synthase, and invertase activities in developing fruit of Lycopersicon esculentum mill. and the sucrose accumulating Lycopersicon hirsutum humb. and bonpl. Plant Physiol. 95, 623–627. doi: 10.1104/pp.95.2.623
Ould-Ahmed, M., Decau, M. L., Morvan-Bertrand, A., Prud’homme, M. P., Lafrenière, C., and Drouin, P. (2017). Fructan, sucrose and related enzyme activities are preserved in timothy (Phleum pratense L.) during wilting. Grass Forage Sci. 72, 64–79. doi: 10.1111/gfs.12209
Pantin, F., Simonneau, T., and Muller, B. (2012). Coming of leaf age: control of growth by hydraulics and metabolics during leaf ontogeny. New Phytol. 196, 349–366. doi: 10.1111/j.1469-8137
Patakas, A. (2000). Changes in the solutes contributing to osmotic potential during leaf ontogeny in grapevine leaves. Am. J. Enol. Vitic. 51, 223–226.
Pavlinova, O., Balakhontsev, E., Prasolova, M., and Turkina, M. (2002). Sucrose-phosphate synthase, sucrose synthase, and invertase in sugar beet leaves. Russ. J. Plant Physiol. 49, 68–73. doi: 10.1023/A:1013712311720
Ren, X. D., and Zhang, J. J. (2013). Research progresses on the key enzymes involved in sucrose metabolism in maize. Carbohydr. Res. 368, 29–34. doi: 10.1016/j.carres
Ricaud, S., Alaoui-Sossé, B., Dizengremel, P., and Barnola, P. (1994). Carbohydrate status and invertase activities in relation to survival and weak dormancy of the latent buds in Platanus acerifolia Willd. Plant Physiol. Biochem. 32, 277–283.
Roitsch, T., and González, M. C. (2004). Function and regulation of plant invertases: sweet sensations. Trends Plant Sci. 9, 606–613. doi: 10.1016/j.tplants.2004.10.009
Ruan, Y. L. (2014). Sucrose metabolism: gateway to diverse carbon use and sugar signaling. Annu. Rev. Plant Biol. 65, 33–67. doi: 10.1146/annurev-arplant-050213-040251
Schaffer, A. A., Sagee, O., Goldschmidt, E. E., and Goren, R. (1987). Invertase and sucrose synthase activity, carbohydrate status and endogenous IAA levels during Citrus leaf development. Physiol. Plant 69, 151–155. doi: 10.1111/j.1399-3054.1987.tb01959.x
Singh, N., and Luthra, R. (1988). Sucrose metabolism and essential oil accumulation during lemongrass (Cymbopogon flexuosus Stapf.) leaf development. Plant Sci. 57, 127–133. doi: 10.1016/0168-9452(88)90078-7
Smith, S. M., Fulton, D. C., Chia, T., Thorneycroft, D., Chapple, A., Dunstan, H., et al. (2004). Diurnal changes in the transcriptome encoding enzymes of starch metabolism provide evidence for both transcriptional and posttranscriptional regulation of starch metabolism in Arabidopsis leaves. Plant Physiol. 136, 2687–2699. doi: 10.1104/pp.104.044347
Suzue, Y., Tsukuda, M., Hatano, S., Kanayama, Y., Yamada, K., Shiratake, K., et al. (2006). Changes in the activity and gene expression of sorbitol- and sucrose-related enzymes with leaf development of ‘La France’ pear. J. Japan Soc. Hortic. Sci. 75, 45–50. doi: 10.2503/jjshs.75.45
Tang, C. R., Huang, D. B., Yang, J. H., Liu, S. J., Sakr, S., Li, H. P., et al. (2010). The sucrose transporter HbSUT3 plays an active role in sucrose loading to laticifer and rubber productivity in exploited trees of Hevea brasiliensis (para rubber tree). Plant Cell Environ. 33, 1708–1720. doi: 10.1111/j.1365-3040.2010.02175.x
Tupý, J. (1969). Stimulatory effects of 2, 4-dichlorophenoxyacetic acid and of 1-naphthylacetic acid on sucrose level, invertase activity and sucrose utilization in the latex of Hevea brasiliensis. Planta 88, 144–153. doi: 10.1007/BF01391120
Tupy, J. (1989). “Sucrose supply and utilization for latex production,” in Physiology of Rubber Tree Latex (Boca Raton), eds J. d’Auzac, J. L. Jacob, H. Chrestin (Florida: CRC Press), 179–218.
Turgeon, R. (1989). The sink-source transition in leaves. Annu. Rev. Plant Biol. 40, 119–138. doi: 10.1146/annurev.pp.40.060189.001003
Tymowska-Lalanne, Z., and Kreis, M. (1998). The plant invertases: physiology, biochemistry and molecular biology. Adv. Bot. Res. 28, 71–117. doi: 10.1016/S0065-2296(08)60294-3
van Beilen, J. B., and Poirier, Y. (2007). Establishment of new crops for the production of natural rubber. Trends Biotechnol. 25, 522–529. doi: 10.1016/j.tibtech
Voss, K. (2001). Biologische Bedeutung und Aktivierbarkeit der [Beta]-D-Glykosidase in BläTtern Von Hevea Brasiliensis (Willd.) Muell. Arg. (1865). Ph.D. thesis, University of Hamburg, Germany.
Walker, J. L., and Huber, S. C. (1989). Regulation of sucrose-phosphate-synthase activity in spinach leaves by protein level and covalent modification. Planta 177, 116–120. doi: 10.1007/BF00392161
Wang, J., Nayak, S., Koch, K., and Ming, R. (2013). Carbon partitioning in sugarcane (Saccharum species). Front. Plant Sci. 4:201. doi: 10.3389/fpls.2013.00201
Wang, Y., Chen, J., Feng, J., Qin, Q., and Huang, J. (2015). Overexpression of a loquat (Eriobotrya japonica Lindl.) vacuolar invertase affects sucrose levels and growth. Plant Cell Tissue Organ Cult. 123, 99–108. doi: 10.1007/s11240-015-0817-0
Winter, H., and Huber, S. C. (2000). Regulation of sucrose metabolism in higher plants: localization and regulation of activity of key enzymes. Crit. Rev. Biochem. Mol. Biol. 35, 253–289. doi: 10.1080/10409230008984165
Xu, S. M., Brill, E., Llewellyn, D. J., Furbank, R. T., and Ruan, Y. L. (2012). Overexpression of a potato sucrose synthase gene in cotton accelerates leaf expansion, reduces seed abortion, and enhances fiber production. Mol. Plant. 5, 430–441. doi: 10.1093/mp/ssr090
Yadav, U. P., Ivakov, A., Feil, R., Duan, G. Y., Walther, D., Giavalisco, P., et al. (2014). The sucrose–trehalose 6-phosphate (Tre6P) nexus: specificity and mechanisms of sucrose signalling by Tre6P. J. Exp. Bot. 65, 1051–1068. doi: 10.1093/jxb/ert457
Zamski, E., and Schaffer, A. A. (eds). (1996). “Photoassimilate distribution in plants and crops: source–sink relationships,” in Books in Soils, Plants, and the Environment, (New York, NY: Marcel Dekker).
Zhang, Y., Ding, Z., Ma, F., Chauhan, R. D., Allen, D. K., Brutnell, T. P., et al. (2015). Transcriptional response to petiole heat girdling in cassava. Sci. Rep. 5:8414. doi: 10.1038/srep08414
Keywords: rubber tree, leaf development, sucrose metabolism, sugar content, enzyme activity, gene expression
Citation: Zhu J, Qi J, Fang Y, Xiao X, Li J, Lan J and Tang C (2018) Characterization of Sugar Contents and Sucrose Metabolizing Enzymes in Developing Leaves of Hevea brasiliensis. Front. Plant Sci. 9:58. doi: 10.3389/fpls.2018.00058
Received: 29 September 2017; Accepted: 11 January 2018;
Published: 01 February 2018.
Edited by:
Wim Van den Ende, KU Leuven, BelgiumReviewed by:
Henry Christopher Janse van Rensburg, KU Leuven, BelgiumJérémy Lothier, University of Angers, France
Copyright © 2018 Zhu, Qi, Fang, Xiao, Li, Lan and Tang. This is an open-access article distributed under the terms of the Creative Commons Attribution License (CC BY). The use, distribution or reproduction in other forums is permitted, provided the original author(s) and the copyright owner are credited and that the original publication in this journal is cited, in accordance with accepted academic practice. No use, distribution or reproduction is permitted which does not comply with these terms.
*Correspondence: Chaorong Tang, Y2hhb3Jvbmd0YW5nQDEyNi5jb20=
†Co-first authors