- 1Institut Jean-Pierre Bourgin, INRA, AgroParisTech, CNRS, Université Paris-Saclay, Versailles, France
- 2UMR MIA-Paris, AgroParisTech, Institut National de la Recherche Agronomique, Université Paris-Saclay, Paris, France
- 3Institute of Plant Sciences Paris-Saclay, Centre National de la Recherche Scientifique, Institut National de la Recherche Agronomique, Université Paris-Sud, Université Evry, Université Paris-Saclay, Orsay, France
- 4Institute of Plant Sciences Paris-Saclay, Université Paris Diderot, Sorbonne Paris-Cité, Orsay, France
- 5Institut de Recherche en Horticulture et Semences, Université d’Angers, Institut National de la Recherche Agronomique, Agrocampus Ouest, UMR 1345, SFR 4207 QUASAV, Angers, France
- 6GQE – Le Moulon, Institut National de la Recherche Agronomique, Université Paris-Sud, Centre National de la Recherche Scientifique, AgroParisTech, Université Paris-Saclay, Gif-sur-Yvette, France
Dormancy and germination vigor are complex traits of primary importance for adaptation and agriculture. Intraspecific variation in cytoplasmic genomes and cytonuclear interactions were previously reported to affect germination in Arabidopsis using novel cytonuclear combinations that disrupt co-adaptation between natural variants of nuclear and cytoplasmic genomes. However, specific aspects of dormancy and germination vigor were not thoroughly explored, nor the parental contributions to the genetic effects. Here, we specifically assessed dormancy, germination performance and longevity of seeds from Arabidopsis plants with natural and new genomic compositions. All three traits were modified by cytonuclear reshuffling. Both depth and release rate of dormancy could be modified by a changing of cytoplasm. Significant changes on dormancy and germination performance due to specific cytonuclear interacting combinations mainly occurred in opposite directions, consistent with the idea that a single physiological consequence of the new genetic combination affected both traits oppositely. However, this was not always the case. Interestingly, the ability of parental accessions to contribute to significant cytonuclear interactions modifying the germination phenotype was different depending on whether they provided the nuclear or cytoplasmic genetic compartment. The observed deleterious effects of novel cytonuclear combinations (in comparison with the nuclear parent) were consistent with a contribution of cytonuclear interactions to germination adaptive phenotypes. More surprisingly, we also observed favorable effects of novel cytonuclear combinations, suggesting suboptimal genetic combinations exist in natural populations for these traits. Reduced sensitivity to exogenous ABA and faster endogenous ABA decay during germination were observed in a novel cytonuclear combination that also exhibited enhanced longevity and better germination performance, compared to its natural nuclear parent. Taken together, our results strongly support that cytoplasmic genomes represent an additional resource of natural variation for breeding seed vigor traits.
Introduction
The evolutionary success of angiosperms is largely due to the invention of seeds, which protect and scatter the next generation and provide it with resources upon germination. Germination is a complex process determined by the intrinsic properties of seeds and influenced by biotic and abiotic environment, aging-related damage, which can occur during seed storage, and dormancy, which prevents germination under favorable conditions. In nature, dormancy allows the seed to await for the favorable season for seedling success (Finch-Savage and Footitt, 2017). Once dormancy is released, the capacity of seeds to germinate in a wide range of environmental conditions contributes to adaptation, and germination speed has a fitness impact in competitive situations (Dubois and Cheptou, 2012). Germination is also a trait of primary interest for agriculture, where seed vigor, defined as the properties that ensure a fast and synchronized germination in field conditions (Finch-Savage and Bassel, 2016), is crucial for the quality of seed lots. In contrast to its adaptive role in natural conditions, dormancy is not a desirable trait in crops, as it leads to low and unsynchronized seedling emergence rates in agricultural production (Shu et al., 2015). In the laboratory, seed vigor is assessed through the speed and uniformity of germination in favorable conditions, germination performance under stress conditions, and the ability to survive storage (Finch-Savage et al., 2010; Rajjou et al., 2012). Seed longevity, i.e., ability to survive and maintain germination performance despite physiological damage occurring during storage, is an important component of seed vigor (Rajjou and Debeaujon, 2008; Sano et al., 2016).
Arabidopsis thaliana (hereafter Arabidopsis) has been widely used to decipher seed biology traits through mutant studies and genome-wide analyses (North et al., 2010). Arabidopsis natural accessions display genetic variation for seed dormancy, longevity and germination vigor (Alonso-Blanco et al., 2003; Clerkx et al., 2004; Bentsink et al., 2010; Vallejo et al., 2010; Yuan et al., 2016b). For example, the release of seed dormancy during post-harvest (PH) dry storage occurred at variable speeds in 112 Arabidopsis accessions, with the time of storage required to reach 50% of germination ranging from 3.5 to 264 days (Debieu et al., 2013). In addition, Arabidopsis natural variation in seed dormancy and longevity has been shown relevant for adaptation in ecological studies (Donohue et al., 2005b; Huang et al., 2010; Kronholm et al., 2012; Debieu et al., 2013; Postma et al., 2015). A number of quantitative trait loci (QTL) for dormancy, longevity, germination speed, and germination tolerance to stresses have been reported (van Der Schaar et al., 1997; Alonso-Blanco et al., 2003; Clerkx et al., 2004; Bentsink et al., 2010; Galpaz and Reymond, 2010; Huang et al., 2010; Joosen et al., 2012; Nguyen et al., 2012; Yuan et al., 2016a). Further major advances in our understanding of the genetic bases of natural variation in seed dormancy and germination are being provided from the functional study of the genes underlying these loci (Shu et al., 2015; Nonogaki, 2017). For example, DOG1, a major locus for adaptive dormancy (Bentsink et al., 2006; Kronholm et al., 2012) conserved among plants, can control seed dormancy in an ABA-independent manner (Née et al., 2017b). Functional studies suggested that DOG1 acts through its interaction with RDO5 and AHG1/3 (Née et al., 2017a) and contributes to the seasonal timing of germination through a temperature-dependent control of GA-regulated germination-promoting enzymes, at least in Brassicaceae (Graeber et al., 2014). ABA and GA are the two major hormones that govern the balance between dormancy and germination through their antagonist effects: ABA promotes the induction and maintenance of seed dormancy, whereas GAs promote germination by stimulating tegument and endosperm rupture and embryo cellular elongation (Finch-Savage and Leubner-Metzger, 2006; Shu et al., 2016).
Comparatively to nuclear genetic control on the establishment of seed traits important for adaptation or agriculture, little is known about the role of cytoplasmic variation in these traits, despite the crucial role of mitochondria and chloroplasts in seed quality and germination performance. Upon imbibition, mitochondrial respiration resumes almost immediately to fuel the very active metabolism which is required during imbibition and germination (Paszkiewicz et al., 2017). Plastids are the site of the early steps of ABA and GA syntheses. Both mitochondria and chloroplasts have endosymbiotic origins and their co-evolution with the nucleus of the host cell has shaped nuclear and organellar genomes, while organizing the compartmentalized metabolism of the plant cell (Dyall et al., 2004; Kutschera and Niklas, 2005). The tuning of mitochondrial and chloroplast functions is under a complex and still elusive network of genetic and metabolic regulations (Rurek, 2016; De Souza et al., 2017). This is mainly ensured and controlled by nuclear-encoded factors, some of which interact with organelle genes or their products. Numerous genes encoding organellar proteins are expressed during germination and their inactivation often affects the process (Demarsy et al., 2011; Yang et al., 2011; Savage et al., 2013; Sew et al., 2016). In addition, transcriptional regulation of specific mitochondrial protein-encoding genes during seed germination has been largely documented (Howell et al., 2008; Narsai et al., 2011; Law et al., 2012). Also, plastid gene expression is required for proper seed development in Arabidopsis (Bryant et al., 2011) and maize (Sosso et al., 2012).
Based on the severe consequences for seed development and germination arising from anomalies in the genetic and physiological activities of plastids and mitochondria, it could be assumed that natural cytoplasmic variation affecting these traits is strongly limited, if not suppressed, by natural selection. However, a few studies have reported results suggesting an impact of natural variation in cytoplasmic genomes on seed physiological traits. A seminal study on reciprocal Arabidopsis F1s and backcross generations showed that cytoplasm variation impacted germination efficiency (Corey et al., 1976). A recent report on seed germination performance of reciprocal hybrids between Arabidopsis lyrata populations suggested that a disruption of cytonuclear co-adaptation contributed to the lower germination of F1 and F2 hybrid generations (Hämälä et al., 2017). Furthermore, in a study using Arabidopsis cytolines, each combining the nuclear genome of a natural variant with the cytoplasmic genomes of a different variant, we previously demonstrated the impact of cytoplasmic natural variation and cytonuclear interactions on adaptive traits in the field, including germination (Roux et al., 2016). Here, using the same cytolines, we have investigated further the effect of natural genetic variation within organelles on specific physiological seed traits relevant to adaptation and agriculture, namely dormancy, germination performance, and longevity. We found that genetic variation in organelle genomes could impact these three traits, depending on the nuclear background. We also showed that parental natural accessions had contrasted contributions to the cytonuclear effect on germination phenotype depending whether they provided the nuclear or cytoplasmic genomes. Furthermore, we uncovered novel cytonuclear combinations that displayed higher performance than their natural nuclear parent, suggesting that cytoplasmic variation could potentially be used in breeding of seed traits. The improved germination performance and seed longevity of a novel cytonuclear combination was associated with more efficient endogenous ABA degradation and lower exogenous ABA sensitivity.
Materials and Methods
Plant Material
Cytolines are genotypes combining the nuclear genome of one parent with the organelle genomes of another conspecific parent. Hereafter, a cytoline possessing the cytoplasm of accession “A” and the nucleus of accession “B” is designated [A]B. We used series of cytolines derived from eight natural accessions of Arabidopsis, namely: Blh-1, Bur-0, Ct-1, Cvi-0, Ita-0, Jea, Oy-0, and Sha, which were selected for their wide genetic diversity (Mckhann et al., 2004; Moison et al., 2010). Production and genotyping of the cytolines were previously reported (Roux et al., 2016). Seed stocks of the 64 genotypes (56 cytolines and 8 parental accessions) are available at the Versailles Arabidopsis Stock Center (CRB1).
Unless specified, the seed stocks (called CRB seeds) used were obtained in a growth chamber and previously used in the study of Roux et al. (2016). They are conserved in controlled conditions (4°C, 15–30% relative humidity, RH) in the Versailles Arabidopsis Stock Center. These seed lots had been stored for at least 18 months when used for the experiments described here.
For dormancy studies, two additional seed productions (new-1 and new-2) of the 64 genotypes were realized in a growth chamber under the same conditions as in Roux et al. (2016) (light 16 h at 21°C, dark 8 h at 18°C), using two plants per genotype, each on either of the two available shelves.
An additional seed production of the Sha and [Blh-1]Sha lines was realized in the greenhouse, seeds from four individuals were pooled for each genotype.
Supplementary Table S1 summarizes the origin of seeds used in each experiment.
Seed Dormancy Assays
For the 64 genotypes, the depth of dormancy was measured on freshly harvested seeds then at 3, 6, and 9 months of after-ripening (designated 0PH, 3PH, 6PH, and 9PH). Seed imbibition was conducted in Petri dishes (Ø 55 mm) on filter papers with three sheets of absorbent paper (Roundfilter paper circles, Ø 45 mm; Schleicher and Schuell, Dassel, Germany) covered by a black membrane filter (ME 25/31, Ø 45 mm; Schleicher and Schuell, Dassel, Germany) wetted with 1.3 mL of ultrapure water. Seeds were then incubated in a controlled culture room under continuous light (Philips TRM HOW/33 RS tubes, 70 μmol.m-2.s-1) at 15°C or 25°C. Germination (protrusion of the radicle after rupture of the testa and endosperm) was assessed daily during 12 days by visual examination, although the maximum germination percentage was consistently reached after 96 h in any conditions. A scheme of this experiment is shown in Supplementary Figure S1A.
Results were analyzed independently for each PH time and considering both germination temperatures in order to better control the variability of germination success, except for 0PH whose only results at 15°C were considered because none of the genotypes germinated at 25°C upon harvest. The [Sha]Cvi-0 and [Ct-1]Jea genotypes were excluded from the analyzed dataset due to missing data for at least one biological replicate.
Germination Performance Tests in Challenging Conditions
The complete set of 64 genotypes was tested for germination performance on water and NaCl, after stratification. Seeds were placed on a blue blotter papers (Anchor Paper Company, SGB1924B) positioned on the germination medium (Phytoblend, 0.5% in water) with or without NaCl, in square Petri dishes (120 × 120 × 17 mm). Because seed germination tolerance to NaCl is variable from one accession to another (Galpaz and Reymond, 2010), each nuclear series was tested on the NaCl concentration (Supplementary Table S2) that resulted in approximately 50% germination of the parental accession in a preliminary experiment. Each dish contained four to eight genotypes and was duplicated or triplicated. Stratification treatment was applied at 4°C in the dark for 4 days. Then, dishes were transferred into a phytotron system providing continuous light from three sides at 25°C (Sanyo MLR-351H, Versatile environmental test chamber). Germination time was counted from the transfer into the phytotron. For the following of germination kinetics, images were taken at five to six time points between 24 h and 72 h of germination with a digital camera (Nikon Coolpix-P510, Nikon, France). Germination was visually assessed using ImageJ2. The maximum germination percentage (Gmax) was considered reached when no additional germination was observed after another 24 h. A scheme of this experiment is shown in Supplementary Figure S1B.
Following the same procedure, the Sha nuclear series was tested on mannitol (200 mM) or KCl (100 mM). ABA (50 μM) sensitivity tests were realized on Sha, [Blh-1]Sha and [Ita-0]Sha, except that germination was monitored visually daily during 4 days.
Controlled Deterioration Treatment (CDT)
The controlled deterioration treatment (CDT) was performed in order to mimic natural seed aging (Tesnier and Strookman-Donkers, 2002; Rajjou et al., 2008). These experiments were performed on the Sha and Ct-1 nuclear series, on CRB seeds.
Briefly, dry mature seeds were first equilibrated at 75% RH (20°C) during 3 days. After this step, day 0 controls were immediately dried back in a desiccator with silica gel for 3 days. Treatment was done by storing the seeds (at 75% RH, using sealed boxes with saturated NaCl solution) for 10, 20, 30, and 40 days at 35°C. After the treatment, seeds were dried back on silica gel as above. Germination percentage was measured after seed imbibition on water under continuous light at 23°C. A scheme of this experiment is shown in Supplementary Figure S1C.
Measurement of Na and K Ion Contents in Arabidopsis Seeds
A germination experiment was set as described above for germination performance, with or without NaCl (100 mM), except that seeds were collected either immediately after stratification or after 20 h of germination. Three replicates were realized. Seeds were rinsed three times with 50 mL of sterile water and dried at 100°C overnight. The dry weight of each sample was measured and samples were digested in 2 mL of 70% HNO3 for a total of 3 h with temperature ramping from 80 to 120°C. Potassium and sodium contents were determined with a Varian AA240FS atomic absorption spectrometer (Agilent Technologies, Santa Clara, CA, United States) and concentrations calculated by comparison with Na+ and K+ standards.
Measurement of ABA Content in Arabidopsis Seeds
ABA content was measured on dry seeds and on stratified seeds after 6 h of germination, using the same experiment setting as described above for germination performance, with or without NaCl (100 mM). Twenty mg of seeds were ground in a mortar with liquid nitrogen and lyophilized with a freeze-drier. For each sample, 10 mg of freeze-dried powder were extracted with 0.8 mL of acetone/water/acetic acid (80/19/1 v:v:v). ABA stable labeled isotope used as internal standard was prepared as described in Le Roux et al. (2014). Two nanograms of each standard were added to the sample. The extract was vigorously shaken for 1 min, sonicated for 1 min at 25 Hz, shaken for 10 min at 4°C in a Thermomixer (Eppendorf®), and then centrifuged (8,000 × g, 4°C, 10 min). The supernatants were collected, and the pellets were re-extracted twice with 0.4 mL of the same extraction solution, then vigorously shaken (1 min) and sonicated (1 min; 25 Hz). After the centrifugations, the three supernatants were pooled and dried (final volume of 1.6 mL). Each dry extract was dissolved in 140 μL acetone, filtered, and analyzed using a Waters Acquity ultra performance liquid chromatograph coupled to a Waters Xevo triple quadrupole mass spectrometer TQS (UPLC-ESI-MS/MS). The compounds were separated on a reverse-phase column (Uptisphere C18 UP3HDO, 100∗2.1 mm∗3 μm particle size; Interchim, France) using a flow rate of 0.4 mL.min-1 and a binary gradient: (A) acetic acid 0.1% in water (v/v) and (B) acetonitrile with 0.1% acetic acid. The following binary gradient was used (t, % A): (0 min, 98%), (3 min, 70%), (7.5 min, 50%), (8.5 min, 5%), (9.6 min, 0%), (13.2 min, 98%), (15.7 min, 98%). Mass spectrometry was conducted in electrospray and Multiple Reaction Monitoring scanning mode (MRM mode), in negative ion mode (see Le Roux et al., 2014 for monitored transitions). Relevant instrumental parameters were set as follows: capillary 1.5 kV (negative mode), source block and desolvation gas temperatures 130°C and 500°C, respectively. Nitrogen was used to assist the cone and desolvation (150 L.h-1 and 800 L.h-1, respectively), argon was used as the collision gas at a flow of 0.18 mL.min-1.
Statistical Analyses
All analyses were performed with the R software. For all experiments, the selected models and the contrast tests are detailed in Supplementary Methods S1.
For all experiments measuring seed germination, germination success was analyzed using a generalized linear model (McCullagh and Nelder, 1989) assuming a binomial distribution with the dispersion parameter fixed at unity and a logit link function.
For each experiment, the model was selected as follows: (i) factors included in the model were defined according to the experimental design; (ii) starting from the most complete model, nested models were sequentially fitted and the best one according to BIC was selected. Once the model selected, the contrasts relevant to the addressed question were tested, and p-values were adjusted using the Bonferroni procedure to control the family-wise error rate (FWER). A contrast was declared significant if its adjusted p-value was lower than 0.05.
We performed a contrast test procedure to detect significant interacting cytoplasm × nucleus combinations: each test concerned a pair of cytoplasms (C1 and C2) and a pair of nuclei (Na and Nb), designed in the text as ‘cytonuclear interacting combination’; a significant p-value indicated that the effect of changing cytoplasm C1 to C2 in the Na nuclear background differed from the effect of the same change in the Nb background, H0 {([C1]Na-[C2]Na) - ([C1]Nb-[C2]Nb) = 0}. The analysis of data from the complete set of 64 genotypes (8 parents and 56 cytolines) thus allows for the testing of 784 cytonuclear interacting combinations (28 pairs of nuclei × 28 pairs of cytoplasms).
ABA content and Na+/K+ ion ratio were analyzed using linear models. The selection of the model and tests for effects were conducted as described above.
Results
Effects of Cytoplasmic Variations and Cytonuclear Interactions on Seed Dormancy
Following on from the observation of differences in germination rate in field-grown Arabidopsis cytolines (Roux et al., 2016), we sought to evaluate whether cytoplasmic variation and cytonuclear interactions specifically impacted seed primary dormancy and the kinetics of its release during after ripening storage. Germination of seeds of the complete set of 64 genotypes (8 natural accessions and 56 cytolines) was tested at harvest and 3, 6, and 9 months post-harvest (0PH, 3PH, 6PH, or 9PH), without any stratification treatment, in two temperature conditions, 15°C and 25°C (Supplementary Table S3, Figure 1 and Supplementary Figure S2). As expected from the wide variation for dormancy among the panel of parental accessions, the nuclear background had a strong effect on germination. Notably, we had repeatedly observed that Ita-0 is a deeply dormant accession and needs stratification treatment even after several months of after-ripening to fully germinate. In this experiment, no or low germination was obtained with seeds of the Ita-0 nuclear series in any conditions. Hence, these genotypes were excluded from the subsequent analyses. Most seed lots germinated better at 15°C (Figure 1) than at 25°C (Supplementary Figure S2), as previously reported for Col-0 freshly harvested seeds (Leymarie et al., 2012), except those of the Bur-0 nuclear series, which reached almost 100% germination at 25°C at harvest, but displayed lower germination success at 15°C.
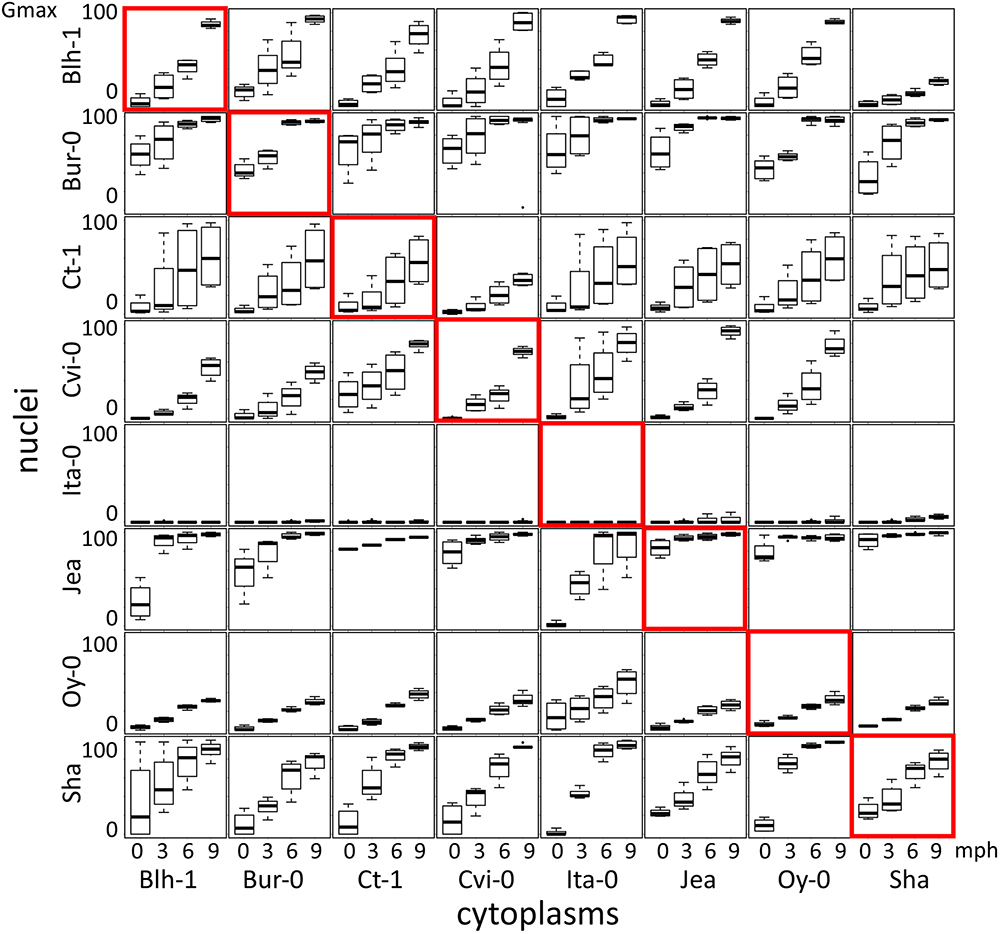
Figure 1. Dormancy release during after ripening in cytoline series. Each panel shows boxplots of the maximum germination percentage at 15°C for one cytonuclear combination 0, 3, 6, and 9 months after harvest (mph). Rows present genotypes sharing their nucleus and columns those sharing their cytoplasm. Natural accessions are framed in red. The [Sha]Cvi-0 cytoline could not be tested due to the small amount of seeds obtained by hand pollination on this male-sterile genotype.
We tested whether germination of cytolines was modified compared to their natural nuclear parent. Table 1 shows that changing organelle genomes had effects on dormancy, but not to the same extent nor in the same direction in all nuclear backgrounds. When effects were observed, foreign cytoplasms tended to enhance dormancy in Ct-1, Jea, Oy-0 and Sha nuclear backgrounds (lower germination in cytolines compared to nuclear natural parent), whereas they rather lowered dormancy in Blh-1, Bur-0, and Cvi-0 backgrounds (higher germination in cytolines). Interestingly, the impact of a given cytoplasm differed according to the nucleus it was associated with, suggesting that dormancy was influenced by cytonuclear interactions. We thus tested for significant cytonuclear interacting combinations impacting dormancy, i.e., for differences between two given nuclei in the effect of a given change of cytoplasm, for all possible pairs of cytoplasms and all possible pairs of nuclei (see the section “Materials and Methods” and Supplementary Methods S1 for details). As some genotypes were excluded from the analysis due to missing data or total absence of germination, 441 combinations were tested for 0PH data, and 505 for data at other PH times. After Bonferroni corrections for multiple tests, we found 177 significant cytonuclear interacting combinations at 0PH, and 232, 186, and 204 at 3PH, 6PH, and 9PH, respectively (Supplementary Table S4). This indicated that the depth of seed primary dormancy is modulated by genetic variation in interacting nuclear- and organelle-encoded partners.
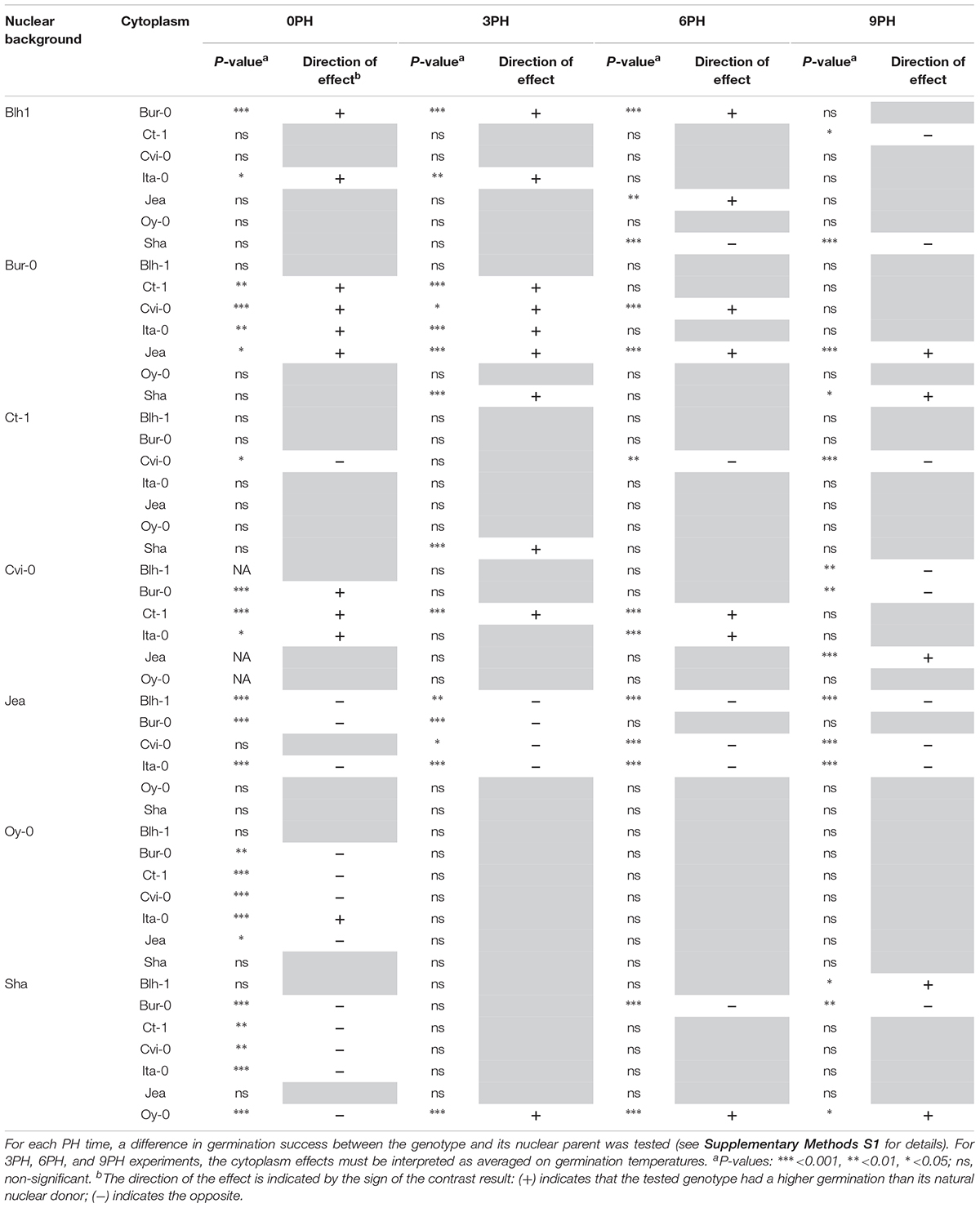
Table 1. Impact of foreign cytoplasms on germination after various post-harvest times in the different nuclear backgrounds.
As seen in Table 1, observed effects of foreign cytoplasms on dormancy often persisted in successive PH times, which was somewhat expected since an effect on the depth of dormancy at harvest would still be detectable after some after-ripening, according to the kinetics of dormancy release. Similarly, we observed persisting effects of cytonuclear interacting combinations over several PH times (Supplementary Table S4): among the 391 cytonuclear interacting combinations that influenced germination at any PH time, 237 (60%) were significant at two PH times or more and 47 (12%) at all four PH times and, in the vast majority (between 86% and 100%) of the cases, they modified germination in the same direction (Table 2), in accordance with a persistence of the observed effects during after-ripening.
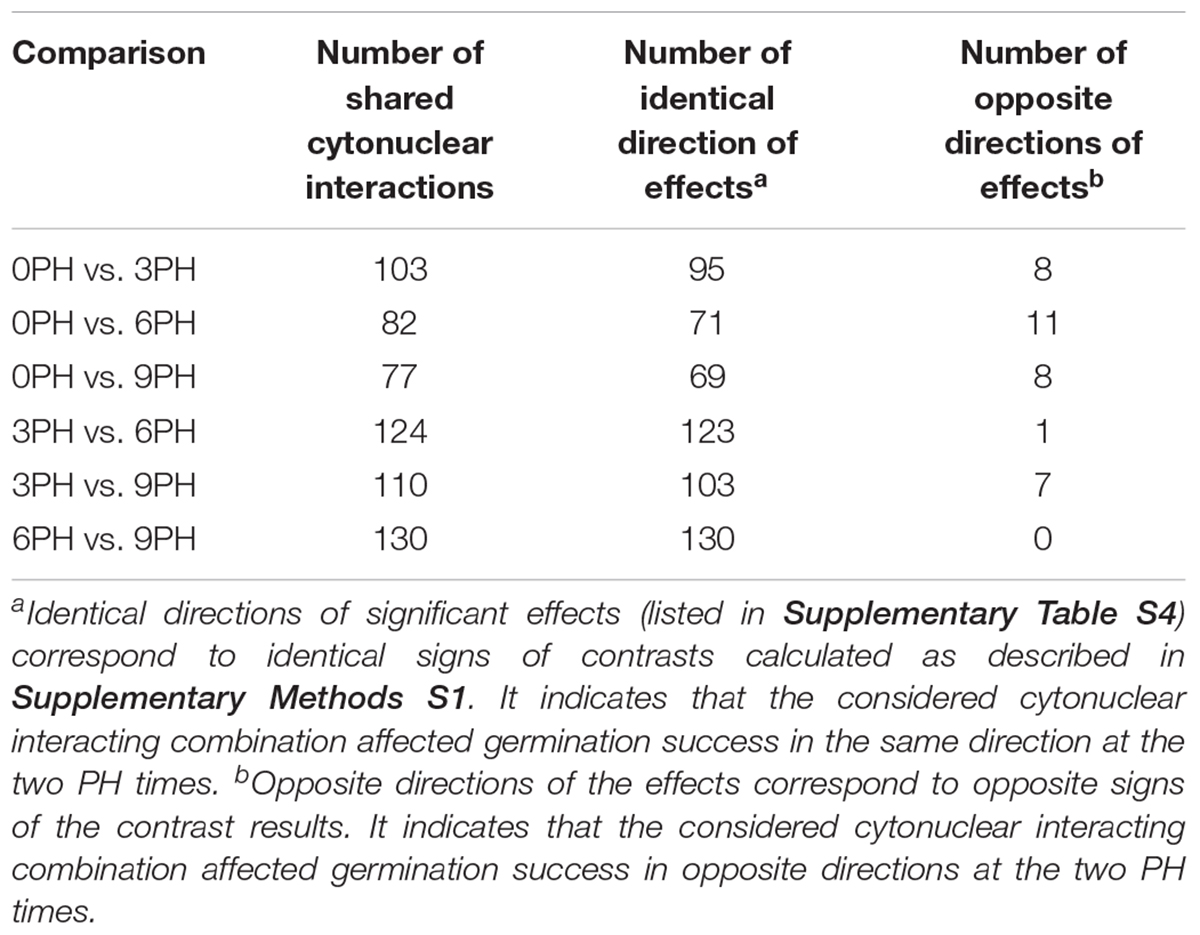
Table 2. Comparison of directions of the effects of significant cytonuclear interacting combinations shared by two PH times.
Interestingly, the rate of dormancy release during after-ripening also seemed to be modified by foreign cytoplasms in some cases: as seen in Table 1, no difference compared to the natural nuclear parent at 0PH could be followed by either better (e.g., [Jea]Cvi-0) or lower (e.g., [Sha]Blh-1) germination percentage in later PH times. In addition, a modification in the kinetics of dormancy release was indicated by a change in the contrast sign between PH times in two cases: [Oy-0]Sha germinated less than Sha at 0PH but better at following PH times; oppositely, [Bur-0]Cvi-0 germinated better than Cvi-0 at 0PH, but less at 9PH. Similarly, we interpreted opposite signs of contrast for the effects of a cytonuclear interacting combination significant at to PH times (Table 2) as indication that it altered the kinetics of dormancy release.
Effects of Cytoplasmic Variations and Cytonuclear Interactions on Germination Performance
In order to assess whether a disruption of cytonuclear co-adaptation also affected the germination performance of seeds after dormancy release, we measured the germination, after stratification, of the complete set of genotypes under permissive and challenging conditions, specifically in the presence of NaCl, at least 18 months after harvest. We used salt stress to enhance differences in germination performance that could be difficult to assess after the stratification treatment. Because seed germination tolerance to NaCl is variable from one accession to another (Galpaz and Reymond, 2010), each nuclear series was tested on the NaCl concentration that resulted in 50% germination of the parental accession in a preliminary experiment. Time plots are shown in Supplementary Figure S3. Despite the stratification treatment, 100% of germination was not reached on water in Ct-1 and Cvi-0 nuclear series, although germination had reached the plateau (Gmax). Nevertheless, we considered the Gmax as an indicator of germination performance and tested, in each nuclear series, the germination performance of cytolines compared to the natural accession, averaged on the two conditions (Supplementary Table S5, Figure 2 and see Supplementary Methods S1 for details). The results clearly showed that the sensitivity of germination to a cytoplasm change was very variable among the nuclear backgrounds, Blh-1 being very sensitive whereas Ct-1 and Oy-0 were not. In general, when affecting germination, foreign cytoplasms had a negative effect. However, three cytolines ([Ct-1]Ita-0, [Ct-1]Jea and [Blh-1]Sha) did germinate better than their respective nuclear parent. After Bonferroni correction for multiple tests, 202 out of 784 cytonuclear interacting combinations (26%, Supplementary Table S4) significantly impacted germination success in this experiment. Thus, as dormancy, germination performance was influenced by interactions between organelle- and nuclear-encoded factors that vary among the panel of parental accessions.
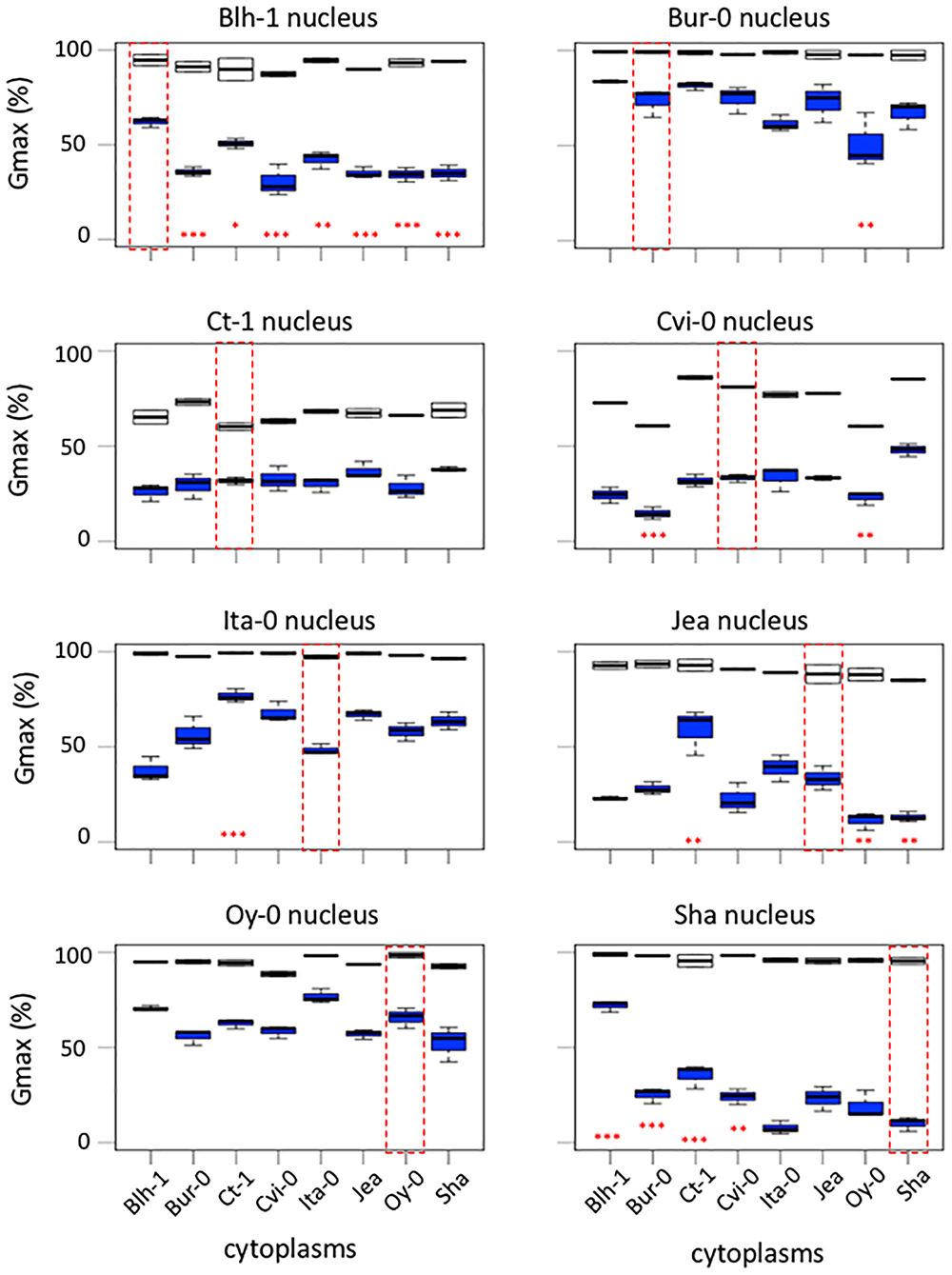
Figure 2. Germination performance of cytoline series. Each panel shows boxplots of the maximum germination percentage (Gmax) of a nuclear series of cytolines, highlighting the natural parental accession in a red dotted frame. White, germination on water; blue, germination on NaCl. Significant differences of germination performance, averaged on the presence of salt, of cytolines compared to their nuclear natural parent are indicated with red stars. P-value: ∗∗∗<0.001, ∗∗<0.01, ∗<0.05 (see Supplementary Methods S1 for details).
Among the 505 cytonuclear interacting combinations that were tested both for germination performance and for dormancy, 92 were significant for both traits. This represented 80% of the interacting combinations impacting germination performance (92/115), but only 23% of those impacting dormancy for at least one PH (92/392). In addition, the cytonuclear interacting combinations that influenced both dormancy and germination performance impacted germination success more often in the same direction in both experiments than would be randomly expected (Table 3, Chi2 test p-value < 0.001), indicating a negative correlation between dormancy and germination performance.
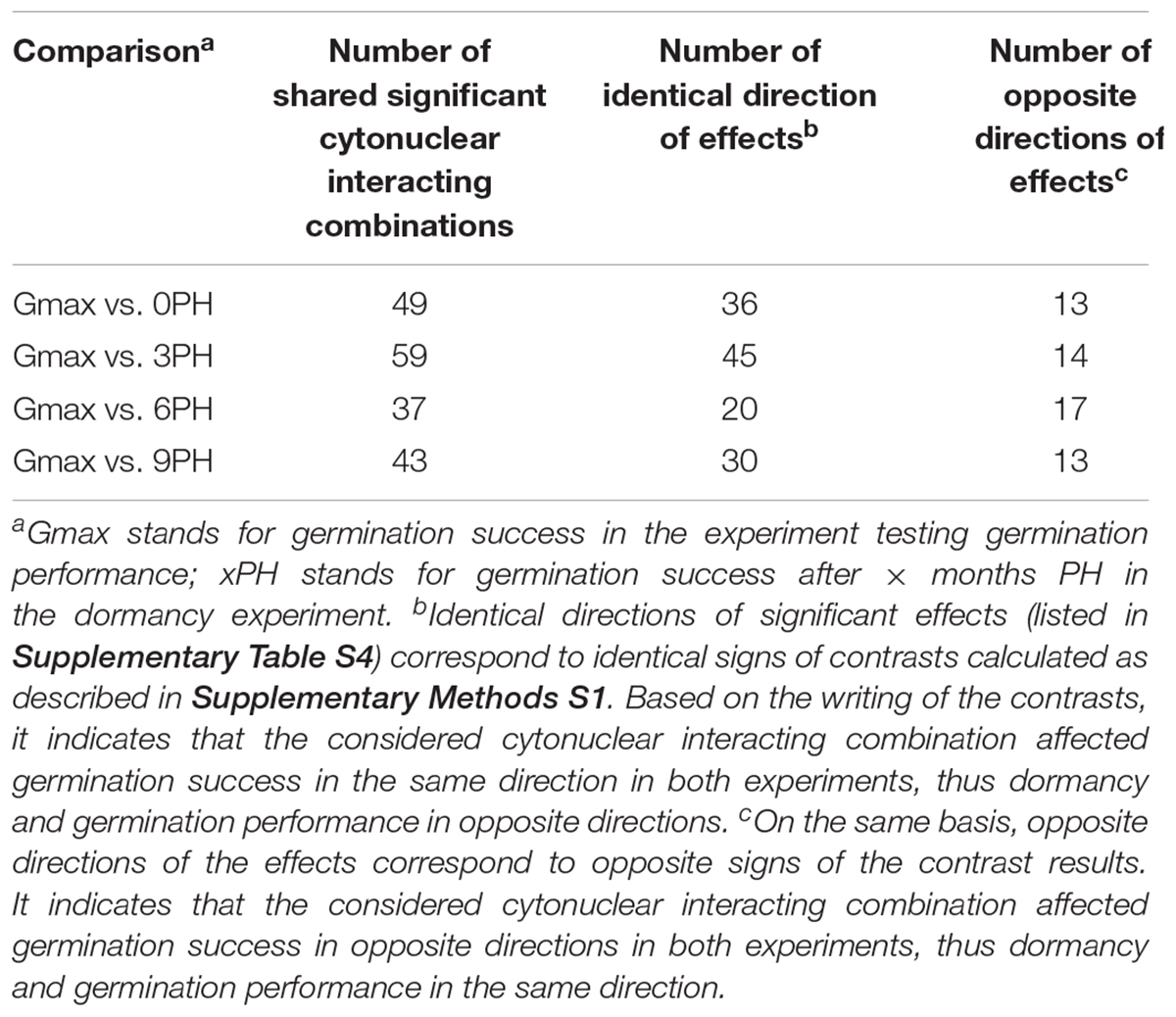
Table 3. Comparison of directions of effects of cytonuclear interacting combinations significant both in the dormancy and germination performance experiments.
Contribution of Individual Accessions to Cytonuclear Interactions That Impact Germination
In the above analyses, the impact on germination traits of a shuffling of genetic compartments depended on the origin of the genomes. To better assess this variation, we analyzed the contribution of individual accessions to cytonuclear interacting combinations affecting dormancy or germination performance. Figure 3 indicates, for each accession, the number of significant cytonuclear interacting combinations that involved its cytoplasm or its nucleus and that impacted dormancy depth, conservatively estimated as effects maintained through all PH times (Figure 3A) or germination performance after storage (Figure 3B). Among the 47 cytonuclear interacting combinations that impacted dormancy depth, 28 involved the Ita-0 cytoplasm and 33 involved the Jea nucleus. Among the 202 significant interacting combinations that affected germination performance after storage, 100 involved the Blh-1 cytoplasm and 76 the Sha nucleus. When considering the contribution of accessions to cytonuclear interacting combinations that affect both dormancy and germination performance, the Blh-1 and Ita-0 cytoplasms and the Sha nucleus clearly emerged (Supplementary Figure S3).
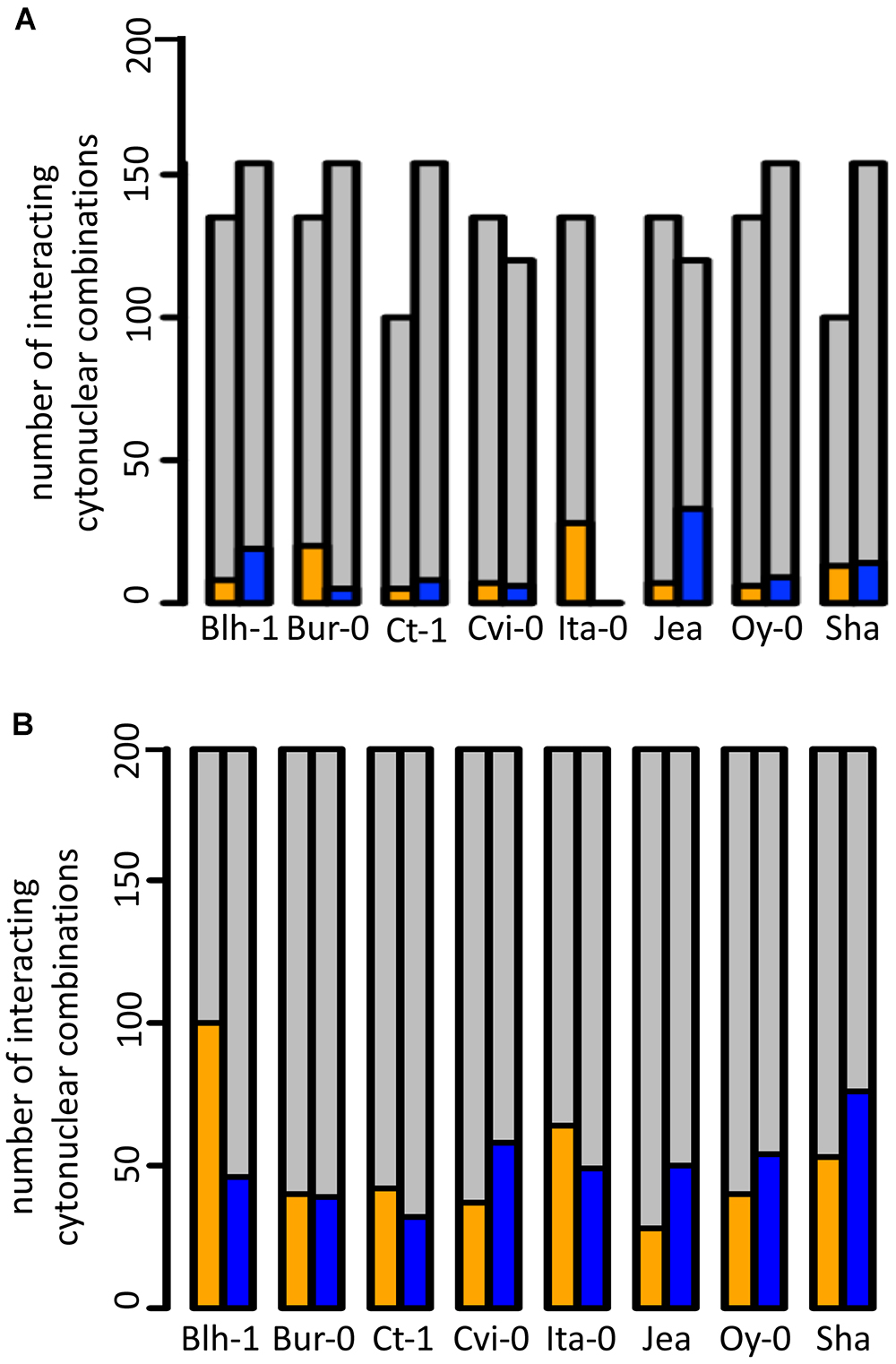
Figure 3. Contribution of individual accessions to cytonuclear interacting combinations impacting germination traits. Cytonuclear interacting combinations are plotted according to the contribution of individual accessions. For each accession, the number of combinations involving its cytoplasm (orange) or its nucleus (blue) that significantly impacted dormancy depth at the four PH times (A) or germination performance after storage (B) are indicated upon the total number of tested cytonuclear interacting combinations (gray).
Effects of Cytoplasm Variation on Seed Longevity in Sha and Ct-1 Nuclear Backgrounds
Along with dormancy and germination performance, longevity is an important component of seed vigor. Thus, we addressed whether this trait was also under the influence of cytoplasmic variation. We selected the Sha and Ct-1 series because these two nuclear backgrounds represented two extreme cases in their contribution to cytonuclear effects in the previous experiments (Figure 3 and Supplementary Figure S4). The longevity of seeds from these two cytoline series was estimated by measuring their viability (germination percentage in standard conditions) after 0, 10, 20, 30 or 40 days of CDT (Supplementary Table S6 and Figure 4). We used the same seed lots as in the assays for germination performance after storage. In this experiment, all genotypes of the Ct-1 series reached 100% germination. We concluded that the partial seed imbibition followed by desiccation included in the CDT treatment, even for the control, acted as a priming treatment releasing the residual dormancy observed in the experiment on germination performance described above. The kinetics of viability loss were different in the two nuclear backgrounds, revealing an expected nuclear effect on seed longevity (Figure 4). Interestingly, four cytolines appeared less sensitive to CDT than their natural nuclear parent, whereas none was more sensitive (Table 4 and see Supplementary Methods S1 for details). In addition, in both nuclear backgrounds, the selected statistical models included an interaction between the cytoplasm and the length of CDT treatment, which suggested that the loss of germination ability between two treatment durations was under the influence of the cytoplasmic genomes. We could not test directly for significant cytonuclear interacting combinations, because the two series were measured in different experiments and the results analyzed independently. Nevertheless, in Blh-1/Sha and Ita-0/Sha cytoplasm pairs, individual cytoplasms had opposite effects in the two nuclear backgrounds (Supplementary Table S7), indicating an influence of cytonuclear epistasis on germination after CDT. Strikingly, the Sha cytoplasm performed better than the Blh-1 and the Ita-0 cytoplasms in the Ct-1 nuclear background but worse when associated with its natural nuclear partner.
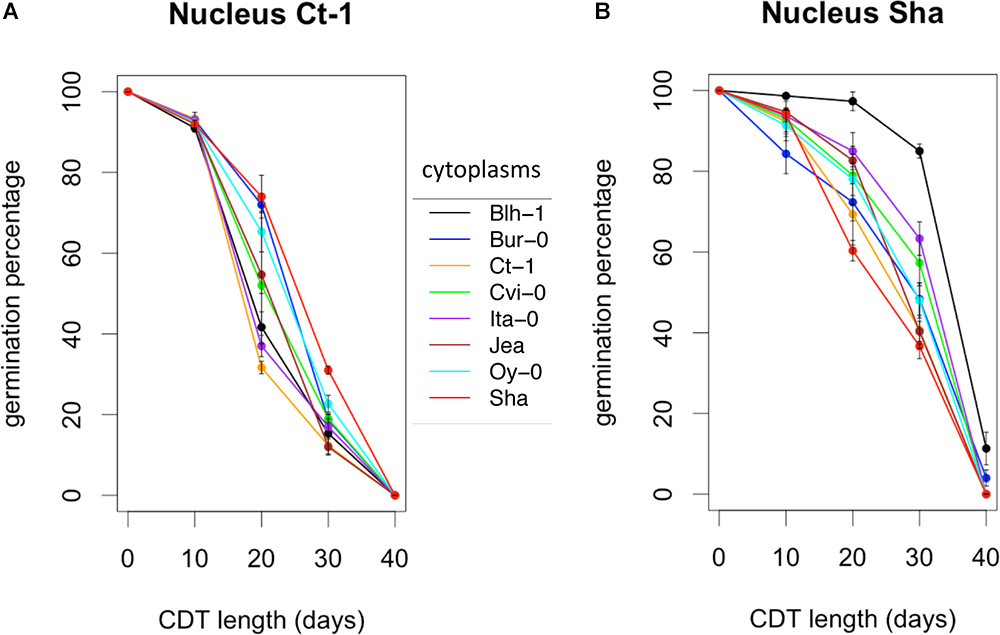
Figure 4. Kinetics of loss of germination efficiency during artificial aging. Controlled deterioration treatment (35°C, 75% RH) was applied for different durations to seeds from cytolines of the Ct-1 (A) and Sha (B) nuclear series. The means of maximum germination percentage from three replicates were plotted. Vertical bars indicate standard deviations from the mean. The colors of the graphs indicate the cytoplasms of the genotypes: black, Blh-1; blue, Bur-0; orange, Ct-1; green, Cvi-0; purple, Ita-0; brown, Jea; cyan, Oy-0; red, Sha.
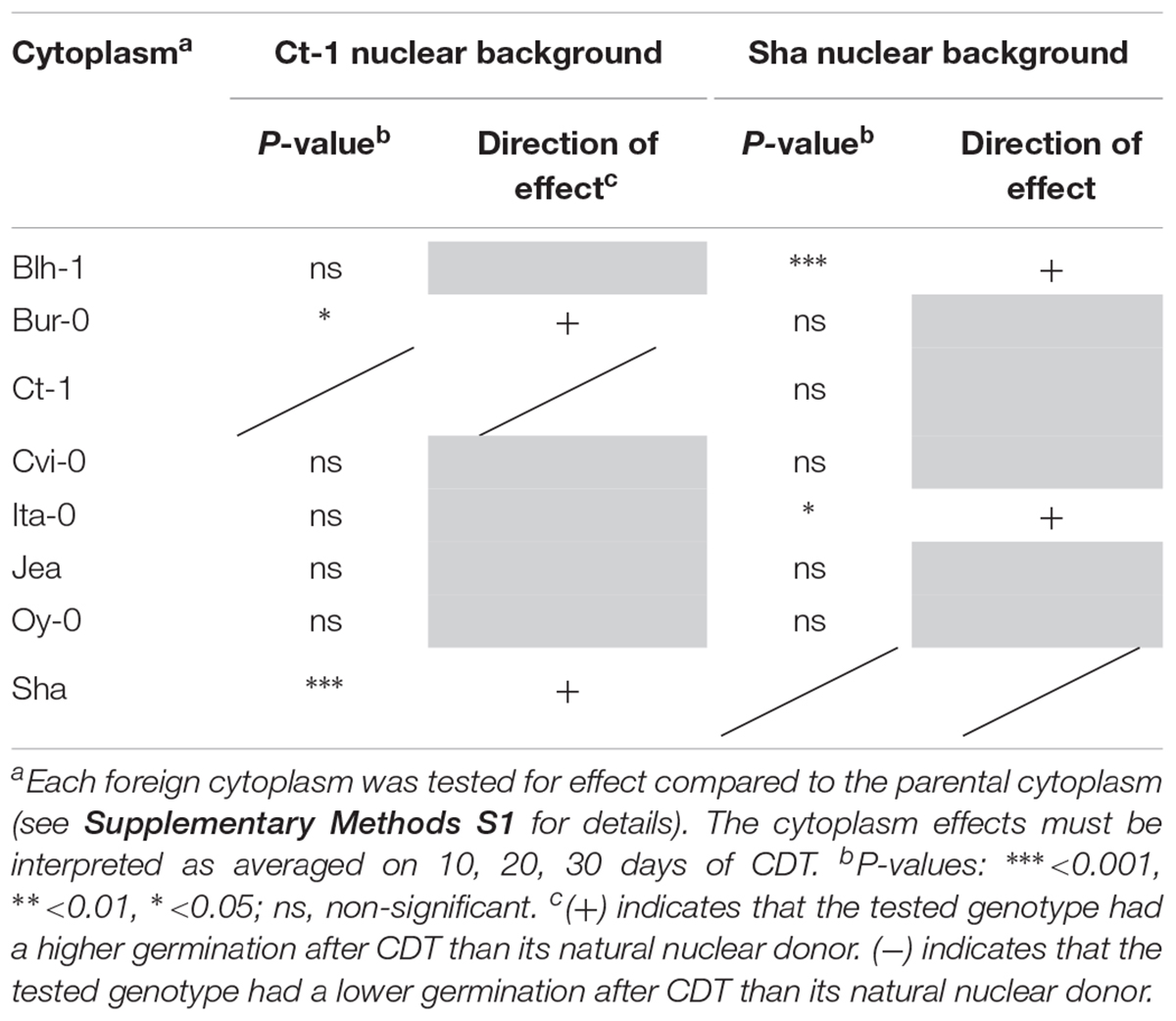
Table 4. Impact of foreign cytoplasms on germination after CDT in the Sha and Ct-1 nuclear backgrounds.
Physiological Clues for Germination Performance of the [Blh-1]Sha Line
A closer look at the 202 cytonuclear interacting combinations that influence germination performance revealed that the [Blh-1]Sha combination was involved in 46 (23%, Supplementary Table S4) of them, indicating that this cytonuclear combination had a major effect on germination. In addition, this genetic combination also had a strong impact on CDT tolerance. We thus decided to get further insight into the behavior of [Blh-1]Sha seeds.
In order to test whether the peculiar behavior of the [Blh-1]Sha seeds was due to the conditions of seed production, we tested germination performance of [Blh-1]Sha and Sha seeds from two productions in different environments, growth chamber and greenhouse. We observed higher germination performance of the cytoline compared to the natural accession for both seed productions (Supplementary Figure S5). An environmental cause of the higher germination performance of [Blh-1]Sha was therefore very unlikely. However, we also observed that this positive effect did not persist in seed samples that had been stored several months at room temperature in the laboratory.
We tested whether the germination performance of [Blh-1]Sha was possibly maintained in the presence of mannitol (200 mM) and KCl (100 mM), by analyzing the germination efficiency of the Sha cytoline series (Supplementary Figure S6). The concentrations applied were chosen to compare results to those of the NaCl treatment (100 mM) in the germination performance experiment. The germination rate of the [Blh-1]Sha cytoline was consistently higher than that of the other genotypes under both osmotic and ionic stresses, although these stresses had a milder effect than the NaCl treatment on all genotypes, as previously reported (Munns and Tester, 2008). The germination curve of [Blh-1]Sha on KCl grouped with those of the other lines in the absence of KCl (Supplementary Figure S6).
It was conceivable that the better germination performance of [Blh-1]Sha seeds would be amplified by an increased tolerance to salt in germination under NaCl stress. Since Na+/K+ ratio is a key indicator of salt tolerance (Shabala and Cuin, 2008), potassium and sodium contents were analyzed in seeds of [Blh-1]Sha, [Ita-0]Sha and Sha. The [Ita-0]Sha was used as a control cytoline with germination performance similar to Sha (Figure 2). Very little sodium accumulated in seeds in the absence of NaCl. When germinated on NaCl, both [Blh-1]Sha and [Ita-0]Sha seeds showed a lower Na+/K+ ratio than Sha seeds (Figure 5). As the two cytolines had different germination responses on NaCl compared to Sha, we considered it unlikely that the Na+/K+ balance is the primary cause of the increased germination success of the [Blh-1]Sha cytoline on NaCl. These results reinforced our hypothesis that the intrinsic germination capacity of [Blh-1]Sha seeds was enhanced compared to its natural parent.
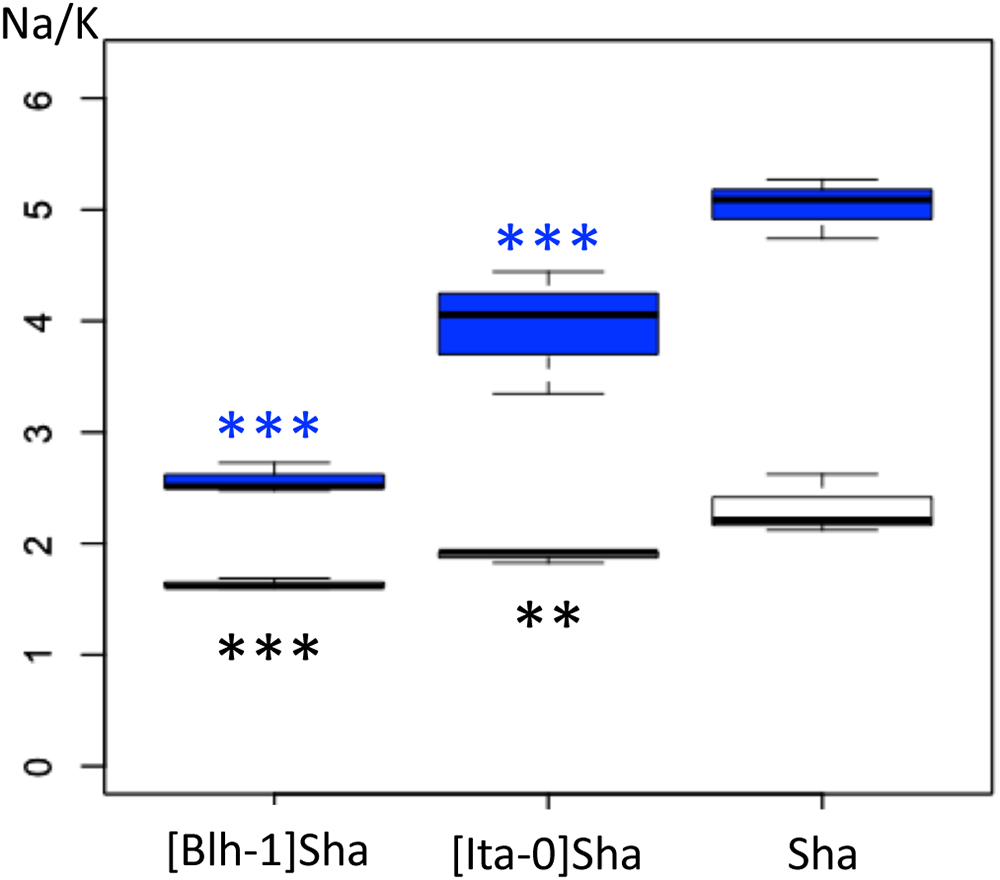
Figure 5. Ratios of Na+ and K+ ion contents in seeds from lines with different cytoplasms associated with the Sha nucleus. Ratios of Na+ and K+ ion contents just after stratification (white boxplots) or after 20 h of germination (blue boxplots) measured in seeds on 100 mM NaCl. The Na/K ratios were different between the three genotypes in pairwise contrast tests. On the figure, only p-values for comparisons of the cytolines to Sha are shown, ∗∗∗<0.001, ∗∗<0.01.
We then focused on ABA content and sensitivity to further explore physiological parameters that could contribute to the enhanced germination performance of [Blh-1]Sha seeds. We measured the endogenous ABA content of seeds from [Blh-1]Sha, [Ita-0]Sha, and Sha lines. The [Ita-0]Sha was used as a control cytoline with germination performance similar to Sha (Figure 2). ABA contents were measured in both dry and stratified seeds placed under germination conditions for 6 h, in the presence or absence of NaCl (Figure 6A). The experiment was repeated after 10 further months of storage in controlled conditions (Versailles Stock Centre), yielding similar results. The combined analysis of data from both experiments (see Supplementary Methods S1 for details) indicated that dry seeds of [Blh-1]Sha contained more ABA than those of [Ita-0]Sha and Sha (p-value < 0.001), whereas they contained less ABA after 6 h of germination, either on water or NaCl (p-value < 0.01). The sensitivity of germinating seeds to exogenous ABA (50 μM) was tested on the same seed stocks after the second experiment (Figure 6B). The [Blh-1]Sha seeds were less sensitive to ABA treatment than Sha seeds. Taken together, the results suggest that both endogenous ABA metabolism and ABA sensitivity are modified in [Blh-1]Sha seeds compared to Sha seeds.
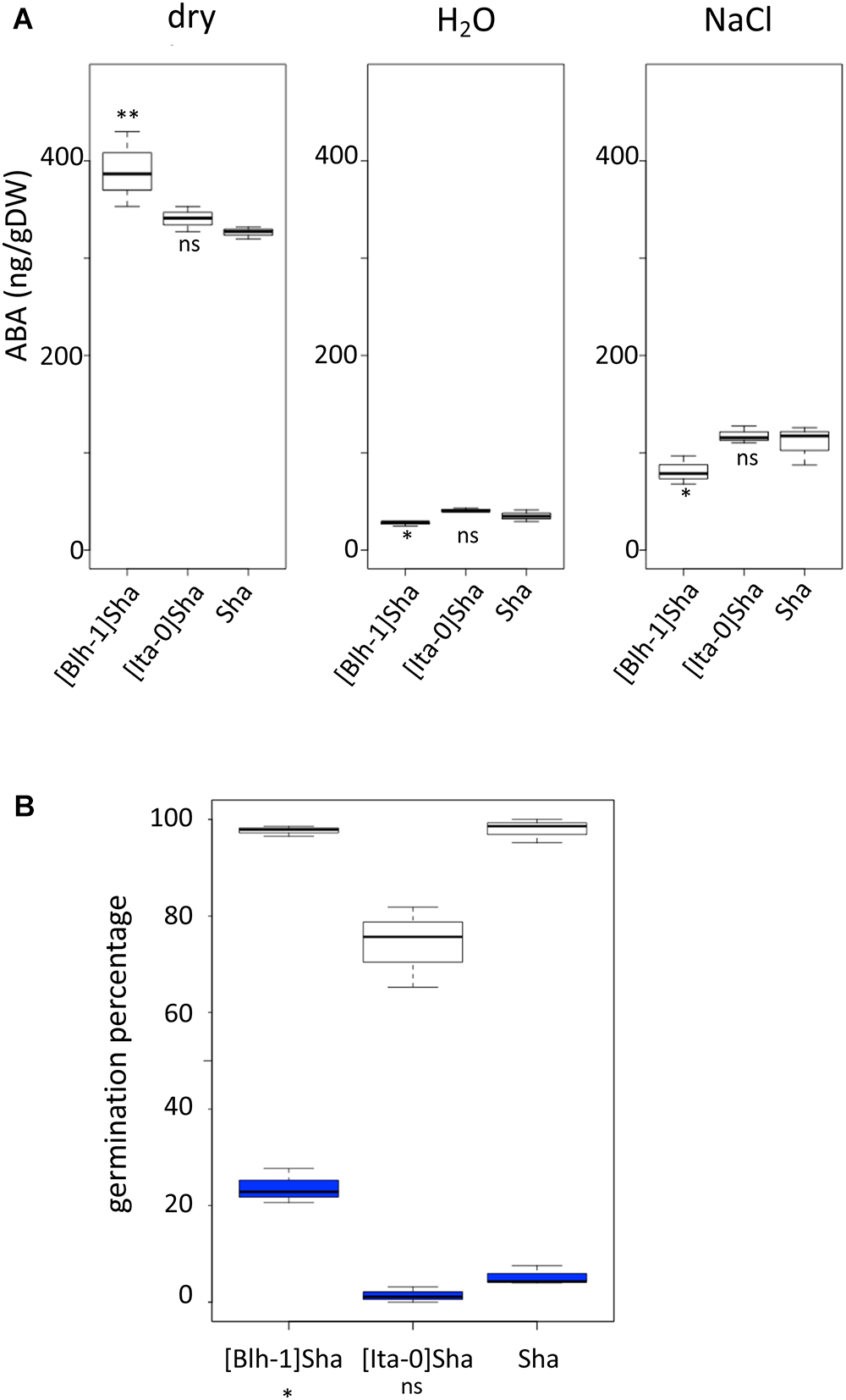
Figure 6. ABA content and sensitivity in seeds from lines of the Sha nucleus series. (A) ABA content of seeds from [Blh-1]Sha, [Ita-0]Sha and Sha genotypes, at the dry stage (left panel), or after stratification followed by 6 h of germination on water (middle panel) or on salt (right panel). Results of comparisons between the cytolines and Sha in the plotted experiment are shown, ∗∗<0.01, ∗<0.05; ns, not significant. (B) Germination of stratified seeds from [Blh-1]Sha, [Ita-0]Sha and Sha on water (white boxplots) or on 50 μM ABA (blue boxplots). Germination rate was monitored after 4 days. Results of the test for a different germination response to treatment of each cytoline compared to the parent (significant cytoplasm × treatment effect) are indicated; ∗p-value < 0.05; ns, not significant (see Supplementary Methods S1 for details).
Discussion
In this study, we examined seed physiological traits important for adaptation and of relevance to agriculture, in relation to cytoplasmic genetic variants and to the co-adaptation between genomic compartments. Cytolines are original and valuable genetic resources to enlighten cytoplasmic and cytonuclear effects (Roux et al., 2016). The analysis of a cytoline series from a given nuclear parent allows assessment of the consequences of a disruption of cytonuclear co-adaptation in the considered nuclear background. The di-allele cross design used to produce the cytolines is suited to the detection of cytonuclear genetic interactions. When examining seed traits, this approach does not distinguish effects acting at the level of the mother plant from those acting at the level of the zygote(s). Nevertheless, as both the mother plant and progeny have the same genotype, it does not preclude the detection of cytoplasmic or cytonuclear effects, keeping in mind that they could act in the mother plant and/or in the progeny.
Dormancy was assessed by monitoring germination of freshly harvested seeds, or seeds after 3, 6, or 9 months of after-ripening, without stratification. Disruption of cytonuclear co-adaptation had variable effects on dormancy depending on the nuclear background, both in the number of cytolines with modified dormancy and in the direction of the effect. When dormancy differed in a cytoline compared to its parent, it tended to be deeper when the parent was weakly dormant (e.g., Jea, Sha) and weaker in the deeply dormant Cvi-0 background. This suggests that coadapted variants of nuclear and organellar genes contributed to adaptive dormancy. However, this was not always verified: in the weakly dormant Bur-0, novel cytonuclear combinations enhanced germination. The Bur-0 nuclear series was remarkably different from the others regarding germination at the two tested temperature conditions: in contrast to the other nuclear series, the seeds of the Bur-0 nuclear series had no dormancy phenotype at 25°C (Supplementary Figure S2) but displayed dormancy at 15°C (Figure 1). A peculiar response to sowing temperature of Bur-0 seeds was also reported in a study examining a set of 73 accessions where they displayed almost 100% germination at 26°C and around 50% germination at 18°C (Schmuths et al., 2006). Genetic variation for the rate of dormancy loss after-ripening was previously reported for Arabidopsis (Barua et al., 2012). Here, by revealing that novel genetic combinations modify the kinetics of dormancy release, we provide evidence that this rate can be modulated by cytonuclear genetic interactions.
Germination performance was estimated through the germination percentage of seeds in normal and challenging conditions. The effects of foreign cytoplasms tended to lower germination performance, which is expected if optimal germinative success is assumed for natural coadapted genotypes. However, some novel cytonuclear combinations had better germination performance than their nuclear parent, suggesting that natural accessions do not always have optimal germination performance, at least in laboratory conditions. A good germination efficiency under challenging stress conditions was reported as a component of seed vigor and related to germination speed (Finch-Savage and Bassel, 2016; Yuan et al., 2016b). Although the limited number of time points precluded the precise fitting of germination curves and reliable comparison of germination speeds, examination of the germination time plots (Supplementary Figure S3) strongly suggested that cytolines with higher germination performance also germinated quicker than their natural parent, whereas those performing worse germinated slower. In addition, the germination percentage was influenced by cytonuclear interactions independently of the presence of salt (no third-order level of interaction), which was consistent with the idea that the detected cytonuclear effects affect germination performance per se rather than salt tolerance. This was further verified in [Blh-1]Sha and [Ita]Sha where a marker of salt tolerance (lower Na+/K+ ratio) was not linked to germination performance, whereas the specific effect of the Blh-1 cytoplasm on ABA decay rate was observed both on water and NaCl. We therefore conclude that our analysis identified effects of cytoplasm variation and cytonuclear interactions on a component of seed vigor. As longevity is also associated to seed vigor, we assessed germination after CDT in Ct-1 and Sha nuclear series, which presented contrasted sensitivities to cytoplasmic change for germination performance and dormancy. Strikingly, disruption of cytonuclear co-adaptation had no or positive effect on seed longevity, at least for the genetic variation tested. This was unexpected since lower seed longevity is generally indicative of low seed vigor and an unfit genotype. It is interesting to note that [Sha]Ct-1 and [Blh-1]Sha displayed both higher longevity and germination performance compared to their natural parents. It is conceivable that both phenotypes revealed the same underlying beneficial effect on seed vigor of the new cytonuclear combinations. However, the [Ita-0]Sha cytoline, which had a higher seed longevity than Sha, did not germinated better. A negative correlation between dormancy and seed longevity determined by co-located QTLs was observed in recombinant inbred-line populations of Arabidopsis (Nguyen et al., 2012). This negative correlation was not verified here: we observed deeper dormancy at 0PH and higher tolerance to CDT in the [Ita-0]Sha cytoline than in Sha. This suggests that the cytonuclear interaction effects on seed longevity and dormancy in this cytoline are not driven by the loci reported in Nguyen’s work or, not exclusively, that this cytoplasm change has different impacts on the Sha allelic effects on seed dormancy and longevity at these loci. It is also conceivable that the apparently contradiction between our result and that from Nguyen et al. (2012) come from differences in the conditions used to estimate seed longevity, i.e., survival after several years of dry storage versus CDT for 10–40 days.
Some accessions contributed more than others to the cytonuclear interacting combinations affecting germination, and individual accessions contributed differently to interactions that affect dormancy depth and germination performance after storage. For example, the Jea nucleus had the highest contribution to significant cytonuclear interacting combinations for dormancy. This suggests that, when changing the organellar partners of cytonuclear epistasis involved in dormancy tuning, Jea alleles lead to different consequences on the phenotype than alleles from other parents.
We found that the majority of cytonuclear interacting combinations that modulated germination performance also altered dormancy, with a negative correlation between dormancy and germination performance in most cases (Table 3). This is consistent with the idea that dormancy and germination speed are parts of a continuum that control germination (Finch-Savage and Leubner-Metzger, 2006). We exclude that residual dormancy of Ct-1 and Cvi-0 series induced a bias toward this conclusion because the contributions of these nuclei to significant cytonuclear interacting combinations shared by germination performance and dormancy experiments are among the fewest observed (Supplementary Figure S4). The continuity of the biological process underlying dormancy and seed vigor was recently brought back to light by a report that linked genetic variation in ABA sensitivity with seed germination performance in Brassica oleracea (Awan et al., 2018). ABA content was also reported to be related to germination speed in B. oleracea (Morris et al., 2016). In this context, our results on the [Blh-1]Sha cytoline seem particularly relevant. At the moment, a formal causal link cannot be made between the enhanced germination vigor of the cytoline on one hand, and faster ABA decay or reduced ABA sensitivity on the other hand. Nevertheless, these observations provide an interesting path for further research. In parallel to deciphering the physiological consequences of novel genetic combinations that modulate seed performance, genetic studies should also lead to the identification of gene variants that underlie the effects reported here. They will necessitate the identification of QTLs for germination and/or dormancy whose allelic effects depend on the cytoplasmic background (Budar and Roux, 2011), as well as the exploration of relevant genetic variants in cytoplasmic genomes.
One remarkable and unexpected result of the present study is the enhanced seed behavior of some cytolines compared to natural genotypes that were shaped by coevolution. Interestingly, the cytoplasm of the drought adapted Kas-1 accession was reported to have a strong and negative effect on water use efficiency in a QTL analysis (Mckay et al., 2008), also suggesting that the natural genotype was not optimal for this trait. It is conceivable that trade-offs between adaptive characters have led to the maintenance, in natural variants of Arabidopsis, of suboptimal genomic combinations for adaptive traits. Indeed, trade-offs between traits under selection, including germination, have been previously shown to underlie natural genetic variation in Arabidopsis (Donohue et al., 2005a; Todesco et al., 2010; Debieu et al., 2013). In light of these results, we suggest that more attention is given to cytoplasmic variation and its effect on seed vigor in the genetic resources of cultivated species.
Data Availability
All datasets generated for this study are included in supplementary files.
Author Contributions
FB conceived the study. CB, LR, M-LM-M, DM, and FB designed the experiments. CB, BG, AB, and SC realized the experiments and collected the data. M-LM-M, BV, and TM-H modeled the data. CB, LR, M-LM-M, DM, and FB analyzed the results and wrote the manuscript with input from all authors.
Funding
This work was funded by the Plant Biology and the Genetics and Plant Breeding INRA Departments (“Cytolignées pilotes”-2010 and “Cytoressources”-2011) and by the ANR BIOADAPT program (ANR-12_ADAP-0004). Institute Jean-Pierre Bourgin (IJPB) and Institute of Plant Sciences Paris-Saclay (IPS2) benefit from support from the LABEX Saclay Plant Sciences-SPS (ANR-10-LABX-0040-SPS). Ion dosages were performed on a device of the LABEX Saclay Plant Sciences-SPS.
Conflict of Interest Statement
The authors declare that the research was conducted in the absence of any commercial or financial relationships that could be construed as a potential conflict of interest.
Acknowledgments
We thank Simon Law, Annie Marion-Poll, and Helen North for helpful comments on the manuscript. We are grateful to Sébastien Thomine for his help in ion dosages. A pre-print of this manuscript has been deposited on the BioRxiv repository (Boussardon et al., 2018).
Supplementary Material
The Supplementary Material for this article can be found online at: https://www.frontiersin.org/articles/10.3389/fpls.2019.00032/full#supplementary-material
Footnotes
References
Alonso-Blanco, C., Bentsink, L., Hanhart, C. J., Blankestijn-de Vries, H., and Koornneef, M. (2003). Analysis of natural allelic variation at seed dormancy loci of Arabidopsis thaliana. Genetics 164, 711–729.
Awan, S., Footitt, S., and Finch-Savage, W. E. (2018). Interaction of maternal environment and allelic differences in seed vigour genes determines seed performance in Brassica oleracea. Plant J. 94, 1098–1108. doi: 10.1111/tpj.13922
Barua, D., Butler, C., Tisdale, T. E., and Donohue, K. (2012). Natural variation in germination responses of Arabidopsis to seasonal cues and their associated physiological mechanisms. Ann. Bot. 109, 209–226. doi: 10.1093/aob/mcr264
Bentsink, L., Hanson, J., Hanhart, C. J., Blankestijn-de Vries, H., Coltrane, C., Keizer, P., et al. (2010). Natural variation for seed dormancy in Arabidopsis is regulated by additive genetic and molecular pathways. Proc. Natl. Acad. Sci. U.S.A. 107, 4264–4269. doi: 10.1073/pnas.1000410107
Bentsink, L., Jowett, J., Hanhart, C. J., and Koornneef, M. (2006). Cloning of DOG1, a quantitative trait locus controlling seed dormancy in Arabidopsis. Proc. Natl. Acad. Sci. U.S.A. 103, 17042–17047. doi: 10.1073/pnas.0607877103
Boussardon, C., Martin-Magniette, M.-L., Godin, B., Benamar, A., Vittrant, B., Citerne, S., et al. (2018). Novel cytonuclear combinations modify Arabidopsis seed physiology and vigor. bioRxiv [Preprint]. doi: 10.1101/370098
Bryant, N., Lloyd, J., Sweeney, C., Myouga, F., and Meinke, D. (2011). Identification of nuclear genes encoding chloroplast-localized proteins required for embryo development in Arabidopsis. Plant Physiol. 155, 1678–1689. doi: 10.1104/pp.110.168120
Budar, F., and Roux, F. (2011). The role of organelle genomes in plant adaptation: time to get to work! Plant Signal. Behav. 6, 635–639.
Clerkx, E. J. M., El-Lithy, M. E., Vierling, E., Ruys, G. J., Blankestijn-de Vries, H., Groot, S. P. C., et al. (2004). Analysis of natural allelic variation of Arabidopsis seed germination and seed longevity traits between the accessions Landsberg erecta and Shakdara, using a new recombinant inbred line population. Plant Physiol. 135, 432–443. doi: 10.1104/pp.103.036814
Corey, L. A., Matzinger, D. F., and Cockerham, C. C. (1976). Maternal and reciprocal effects on seedling characters in Arabidopsis thaliana (L.) Heynh. Genetics 82, 677–683.
Debieu, M., Tang, C., Stich, B., Sikosek, T., Effgen, S., Josephs, E., et al. (2013). Co-Variation between seed dormancy, growth rate and flowering time changes with latitude in Arabidopsis thaliana. PLoS One 8:e61075. doi: 10.1371/journal.pone.0061075.s002
Demarsy, E., Buhr, F., Lambert, E., and Lerbs-Mache, S. (2011). Characterization of the plastid-specific germination and seedling establishment transcriptional programme. J. Exp. Bot. 63, 925–939. doi: 10.1093/jxb/err322
De Souza, A., Wang, J.-Z., and Dehesh, K. (2017). Retrograde signals: integrators of interorganellar communication and orchestrators of plant development. Annu. Rev. Plant Biol. 68, 85–108. doi: 10.1146/annurev-arplant-042916-041007
Donohue, K., Dorn, L., Griffith, C., Kim, E., Aguilera, A., Polisetty, C. R., et al. (2005a). Environmental and genetic influences on the germination of Arabidopsis thaliana in the field. Evolution 59, 740–757.
Donohue, K., Dorn, L., Griffith, C., Kim, E., Aguilera, A., Polisetty, C. R., et al. (2005b). The evolutionary ecology of seed germination of Arabidopsis thaliana: variable natural selection on germination timing. Evolution 59, 758–770.
Dubois, J., and Cheptou, P.-O. (2012). Competition/colonization syndrome mediated by early germination in non-dispersing achenes in the heteromorphic species Crepis sancta. Ann. Bot. 110, 1245–1251. doi: 10.1093/aob/mcs203
Dyall, S. D., Brown, M. T., and Johnson, P. J. (2004). Ancient invasions: from endosymbionts to organelles. Science 304, 253–257. doi: 10.1126/science.1094884
Finch-Savage, W. E., and Bassel, G. W. (2016). Seed vigour and crop establishment: extending performance beyond adaptation. J. Exp. Bot. 67, 567–591. doi: 10.1093/jxb/erv490
Finch-Savage, W. E., Clay, H. A., Lynn, J. R., and Morris, K. (2010). Towards a genetic understanding of seed vigour in small-seeded crops using natural variation in Brassica oleracea. Plant Sci. 179, 582–589. doi: 10.1016/j.plantsci.2010.06.005
Finch-Savage, W. E., and Footitt, S. (2017). Seed dormancy cycling and the regulation of dormancy mechanisms to time germination in variable field environments. J. Exp. Bot. 68, 843–856. doi: 10.1093/jxb/erw477
Finch-Savage, W. E., and Leubner-Metzger, G. (2006). Seed dormancy and the control of germination. New Phytol. 171, 501–523. doi: 10.1111/j.1469-8137.2006.01787.x
Galpaz, N., and Reymond, M. (2010). Natural variation in Arabidopsis thaliana revealed a genetic network controlling germination under salt stress. PLoS One 5:e15198. doi: 10.1371/journal.pone.0015198
Graeber, K., Linkies, A., Steinbrecher, T., Mummenhoff, K., Tarkowská, D., Tureèková, V., et al. (2014). Delay of germination 1 mediates a conserved coat-dormancy mechanism for the temperature- and gibberellin-dependent control of seed germination. Proc. Natl. Acad. Sci. U.S.A. 111, E3571–E3580. doi: 10.1073/pnas.1403851111
Hämälä, T., Mattila, T. M., Leinonen, P. H., Kuittinen, H., and Savolainen, O. (2017). Role of seed germination in adaptation and reproductive isolation in Arabidopsis lyrata. Mol. Ecol. 26, 3484–3496. doi: 10.1111/mec.14135
Howell, K. A., Narsai, R., Carroll, A., Ivanova, A., Lohse, M., Usadel, B., et al. (2008). Mapping metabolic and transcript temporal switches during germination in rice highlights specific transcription factors and the role of rna instability in the germination process. Plant Physiol. 149, 961–980. doi: 10.1104/pp.108.129874
Huang, X., Schmitt, J., Dorn, L., Griffith, C., Effgen, S., Takao, S., et al. (2010). The earliest stages of adaptation in an experimental plant population: strong selection on QTLS for seed dormancy. Mol. Ecol. 19, 1335–1351. doi: 10.1111/j.1365-294X.2010.04557.x
Joosen, R. V. L., Arends, D., Willems, L. A. J., Ligterink, W., Jansen, R. C., and Hilhorst, H. W. M. (2012). Visualizing the genetic landscape of Arabidopsis seed performance. Plant Physiol. 158, 570–589. doi: 10.1104/pp.111.186676
Kronholm, I., Picó, F. X., Alonso-Blanco, C., Goudet, J., Meaux, J., and de. (2012). Genetic basis of adaptation in Arabidopsis thaliana: local adaptation at the seed dormancy QTL DOG1. Evolution 66, 2287–2302. doi: 10.5061/dryad.4rp76r87
Kutschera, U., and Niklas, K. J. (2005). Endosymbiosis, cell evolution, and speciation. Theory Biosci. 124, 1–24. doi: 10.1016/j.thbio.2005.04.001
Law, S. R., Narsai, R., Taylor, N. L., Delannoy, E., Carrie, C., Giraud, E., et al. (2012). Nucleotide and RNA metabolism prime translational initiation in the earliest events of mitochondrial biogenesis during Arabidopsis germination. Plant Physiol. 158, 1610–1627. doi: 10.1104/pp.111.192351
Le Roux, C., Del Prete, S., Boutet-Mercey, S., Perreau, F., Balagué, C., Roby, D., et al. (2014). The hnRNP-Q protein LIF2 participates in the plant immune response. PLoS One 9:e99343. doi: 10.1371/journal.pone.0099343.s007
Leymarie, J., Vitkauskaité, G., Hoang, H. H., Gendreau, E., Chazoule, V., Meimoun, P., et al. (2012). Role of reactive oxygen species in the regulation of Arabidopsis seed dormancy. Plant Cell Physiol. 53, 96–106. doi: 10.1093/pcp/pcr129
McCullagh, P., and Nelder, J. A. (1989). Generalized Linear Models, 2nd Edn. London: Chapman & Hall.
Mckay, J. K., Richards, J. H., Nemali, K. S., Sen, S., Mitchell-Olds, T., Boles, S., et al. (2008). Genetics of drought adaptation in Arabidopsis thaliana II. QTL analysis of a new mapping population, KAS-1 x TSU-1. Evolution 62, 3014–3026. doi: 10.1111/j.1558-5646.2008.00474.x
Mckhann, H. I., Camilleri, C., Berard, A., Bataillon, T., David, J. L., Reboud, X., et al. (2004). Nested core collections maximizing genetic diversity in Arabidopsis thaliana. Plant J. 38, 193–202. doi: 10.1111/j.1365-313X.2004.02034.x
Moison, M., Roux, F., Quadrado, M., Duval, R., Ekovich, M., Lê, D.-H., et al. (2010). Cytoplasmic phylogeny and evidence of cyto-nuclear co-adaptation in Arabidopsis thaliana. Plant J. 63, 728–738. doi: 10.1111/j.1365-313X.2010.04275.x
Morris, K., Barker, G. C., Walley, P. G., Lynn, J. R., and Finch-Savage, W. E. (2016). Trait to gene analysis reveals that allelic variation in three genes determines seed vigour. New Phytol. 212, 964–976. doi: 10.1111/nph.14102
Munns, R., and Tester, M. (2008). Mechanisms of salinity tolerance. Annu. Rev. Plant Biol. 59, 651–681. doi: 10.1146/annurev.arplant.59.032607.092911
Narsai, R., Law, S. R., Carrie, C., Xu, L., and Whelan, J. (2011). In-depth temporal transcriptome profiling reveals a crucial developmental switch with roles for RNA processing and organelle metabolism that are essential for germination in Arabidopsis. Plant Physiol. 157, 1342–1362. doi: 10.1104/pp.111.183129
Née, G., Kramer, K., Nakabayashi, K., Yuan, B., Xiang, Y., Miatton, E., et al. (2017a). Delay of germination1 requires PP2Cphosphatases of the ABA signalling pathway tocontrol seed dormancy. Nat. Commun. 8:72. doi: 10.1038/s41467-017-00113-6
Née, G., Xiang, Y., and Soppe, W. J. (2017b). The release of dormancy, a wake-up call for seeds to germinate. Curr. Opin. Plant Biol. 35, 8–14. doi: 10.1016/j.pbi.2016.09.002
Nguyen, T. P., Keizer, P., van Eeuwijk, F., Smeekens, S., and Bentsink, L. (2012). Natural variation for seed longevity and seed dormancy are negatively correlated in Arabidopsis. Plant Physiol. 160, 2083–2092. doi: 10.1104/pp.112.206649
Nonogaki, H. (2017). Seed biology updates – Highlights and new discoveries in seed dormancy and germination research. Front. Plant Sci. 8:524. doi: 10.3389/fpls.2017.00524
North, H., Baud, S., Debeaujon, I., Dubos, C., Dubreucq, B., Grappin, P., et al. (2010). Arabidopsis seed secrets unravelled after a decade of genetic and omics-driven research. Plant J. 61, 971–981. doi: 10.1111/j.1365-313X.2009.04095.x
Paszkiewicz, G., Gualberto, J. M., Benamar, A., Macherel, D., and Logan, D. C. (2017). Arabidopsis seed mitochondria are bioenergetically active immediately upon imbibition and specialize via biogenesis in preparation for autotrophic growth. Plant Cell 29, 109–128. doi: 10.1105/tpc.16.00700
Postma, F. M., Lundemo, S., and Agren, J. (2015). Seed dormancy cycling and mortality differ between two locally adapted populations of Arabidopsis thaliana. Ann. Bot. 117, 249–256. doi: 10.1093/aob/mcv171
Rajjou, L., and Debeaujon, I. (2008). Seed longevity: survival and maintenance of high germination ability of dry seeds. CR Biol. 331, 796–805. doi: 10.1016/j.crvi.2008.07.021
Rajjou, L., Duval, M., Gallardo, K., Catusse, J., Bally, J., Job, C., et al. (2012). Seed Germination and Vigor. Annu. Rev. Plant Biol. 63, 507–533.
Rajjou, L., Lovigny, Y., Groot, S. P. C., Belghazi, M., Job, C., and Job, D. (2008). Proteome-wide characterization of seed aging in Arabidopsis: a comparison between artificial and natural aging protocols. Plant Physiol. 148, 620–641. doi: 10.1104/pp.108.123141
Roux, F., Mary-Huard, T., Barillot, E., Wenes, E., Botran, L., Durand, S., et al. (2016). Cytonuclear interactions affect adaptive traits of the annual plant Arabidopsis thaliana in the field. Proc. Natl. Acad. Sci. U.S.A. 113, 3687–3692. doi: 10.1073/pnas.1520687113
Rurek, M. (2016). Participation of non-coding RNAs in plant organelle biogenesis. Acta Biochim. Pol. 63, 653–663. doi: 10.18388/abp.2016_1346
Sano, N., Rajjou, L., North, H. M., Debeaujon, I., Marion-Poll, A., and Seo, M. (2016). Staying alive: molecular aspects of seed longevity. Plant Cell Physiol. 57, 660–674. doi: 10.1093/pcp/pcv186
Savage, L. J., Imre, K. M., Hall, D. A., and Last, R. L. (2013). Analysis of essential Arabidopsis nuclear genes encoding plastid-targeted proteins. PLoS One 8:e73291. doi: 10.1371/journal.pone.0073291.s012
Schmuths, H., Bachmann, K., Weber, W. E., Horres, R., and Hoffmann, M. H. (2006). Effects of preconditioning and temperature during germination of 73 natural accessions of Arabidopsis thaliana. Ann. Bot. 97, 623–634. doi: 10.1093/aob/mcl012
Sew, Y. S., Ströher, E., Fenske, R., and Millar, A. H. (2016). Loss of mitochondrial malate dehydrogenase activity alters seed metabolism impairing seed maturation and post-germination growth in Arabidopsis. Plant Physiol. 171, 849–863. doi: 10.1104/pp.16.01654
Shabala, S., and Cuin, T. A. (2008). Potassium transport and plant salt tolerance. Physiol. Plant 133, 651–669. doi: 10.1111/j.1399-3054.2007.01008.x
Shu, K., Liu, X.-D., Xie, Q., and He, Z.-H. (2016). Two faces of one seed: hormonal regulation of dormancy and germination. Mol. Plant 9, 34–45. doi: 10.1016/j.molp.2015.08.010
Shu, K., Meng, Y. J., Shuai, H. W., Liu, W. G., Du, J. B., Liu, J., et al. (2015). Dormancy and germination: how does the crop seed decide? Plant Biol. 17, 1104–1112. doi: 10.1111/plb.12356
Sosso, D., Canut, M., Gendrot, G., Dedieu, A., Chambrier, P., Barkan, A., et al. (2012). PPR8522 encodes a chloroplast-targeted pentatricopeptide repeat protein necessary for maize embryogenesis and vegetative development. J. Exp. Bot. 63, 5843–5857. doi: 10.1093/jxb/ers232
Tesnier, K., and Strookman-Donkers, H. M. (2002). A controlled deterioration test for Arabidopsis thaliana reveals genetic variation in seed quality. Seed Sci. Technol. 30, 149–165.
Todesco, M., Balasubramanian, S., Hu, T. T., Traw, M. B., Horton, M., Epple, P., et al. (2010). Natural allelic variation underlying a major fitness trade-off in Arabidopsis thaliana. Nature 465, 632–636. doi: 10.1038/nature09083
Vallejo, A. J., Yanovsky, M. J., and Botto, J. F. (2010). Germination variation in Arabidopsis thaliana accessions under moderate osmotic and salt stresses. Ann. Bot. 106, 833–842. doi: 10.1093/aob/mcq179
van Der Schaar, W., Alonso-Blanco, C., Léon-Kloosterziel, K. M., Jansen, R. C., van Ooijen, J. W., and Koornneef, M. (1997). QTL analysis of seed dormancy in Arabidopsis using recombinant inbred lines and MQM mapping. Heredity 79(Pt 2), 190–200.
Yang, L., Peng, X., and Sun, M.-X. (2011). AtNG1 encodes a protein that is required for seed germination. Plant Sci. 181, 457–464. doi: 10.1016/j.plantsci.2011.07.011
Yuan, W., Flowers, J. M., Sahraie, D. J., Ehrenreich, I. M., and Purugganan, M. D. (2016a). Extreme QTL mapping of germination speed in Arabidopsis thaliana. Mol. Ecol. 25, 4177–4196. doi: 10.1111/mec.13768
Keywords: cytolines, cytonuclear co-adaptation, cytonuclear interaction, dormancy, seed longevity, germination, seed vigor
Citation: Boussardon C, Martin-Magniette M-L, Godin B, Benamar A, Vittrant B, Citerne S, Mary-Huard T, Macherel D, Rajjou L and Budar F (2019) Novel Cytonuclear Combinations Modify Arabidopsis thaliana Seed Physiology and Vigor. Front. Plant Sci. 10:32. doi: 10.3389/fpls.2019.00032
Received: 05 October 2018; Accepted: 10 January 2019;
Published: 05 February 2019.
Edited by:
Philippe Giegé, Center for the National Scientific Research (CNRS), FranceReviewed by:
Elina Welchen, National University of the Littoral, ArgentinaVéronique Larosa, University of Liège, Belgium
Copyright © 2019 Boussardon, Martin-Magniette, Godin, Benamar, Vittrant, Citerne, Mary-Huard, Macherel, Rajjou and Budar. This is an open-access article distributed under the terms of the Creative Commons Attribution License (CC BY). The use, distribution or reproduction in other forums is permitted, provided the original author(s) and the copyright owner(s) are credited and that the original publication in this journal is cited, in accordance with accepted academic practice. No use, distribution or reproduction is permitted which does not comply with these terms.
*Correspondence: Françoise Budar, ZnJhbmNvaXNlLmJ1ZGFyQGlucmEuZnI=
†Present address: Clément Boussardon, Department of Plant Physiology, Umeå Plant Science Centre, Umeå University, Umeå, Sweden