- 1Department of Agriculture, Food and Environment, University of Pisa, Pisa, Italy
- 2Center for Climate Change Impacts, University of Pisa, Pisa, Italy
Chlorophyll fluorescence analysis is one of the most powerful and widely used techniques to study the effect of stresses on the photosynthetic process. From the first utilization, the Fv/Fm ratio has been largely used as a sensitive indicator of plant photosynthetic performance. Decreases of this index are indicative of the reduction of photosystem II (PSII) efficiency, namely photoinhibition. In the last 20 years, application of chlorophyll fluorescence has been largely improved, and many other informative parameters have been established to detect PSII photochemical efficiency and the partitioning of light energy to alternative dissipative mechanisms (qE, energy-dependent quenching; qZ, zeaxanthin-dependent quenching and qI, photoinhibitory quenching; qH, sustained photoprotective antenna quenching; qM, quenching dependent to chloroplast movement; qT, light harvesting complexes II–I state-transition) such as the recently developed “photoprotective power” of non-photochemical quenching (pNPQ). This review reports a brief description of the main chlorophyll fluorescence parameters and a wide analysis of the current bibliography on the use of different parameters which are useful to detect events of PSII photoinhibition. In addition, in view of the inherent differences in morpho-anatomical, physiological and biochemical features between C3 and C4 metabolism, possible differences in terms of photoinhibition between C3 and C4 plant species under stress conditions are proposed. The attempt is to highlight the limits of their comparison in terms of susceptibility to photoinhibition and to propose direction of future research which, assisted by chlorophyll fluorescence, should improve the knowledge of the different sensitivity of C3 and C4 to abiotic stressors.
Photoinhibition and Stress
Photoinhibition is a phenomenon leading to a reduction of photosynthetic activity principally due to light-induced decreases in CO2 assimilation (Baker, 1996). Even though the reduction in photoassimilation may be dependent to damages to many components of the photosynthetic machinery, frequently the term photoinhibition is used to define light-induced inhibition of photosystem II (PSII) activity (Powles, 1984; Aro et al., 1993; Murata et al., 2007). As light is the energy needed to drive the photosynthetic process, photoinhibition is unavoidable when light exceeds the photosynthetic rate. However, the extent of photoinhibition depends on the balance between photodamage and repair mechanisms of PSII core (Demmig-Adams et al., 2012). The classical molecular scheme of photoinhibition was interpreted as the generation of reactive oxygen species (ROS) induced by excessive reduction of the primary acceptor of PSII, plastoquinone QA, or by charge recombination between acceptor and donor side of PSII (Aro et al., 1993). The generated ROS are responsible for the damage to PSII reaction centers. However, many studies conducted on this topic give another interpretation of the photoinhibition mechanism (Murata et al., 2012): ROS do not damage PSII reaction centers directly but inhibit the repair of PSII (by inhibiting protein synthesis), with resultant stimulation of the photoinhibition of PSII. In the new scheme photodamage to PSII occurs by two consecutive steps: (i) the light-dependent destruction of the Mn cluster of the oxygen-evolving complex and (ii) the inactivation of the PSII reaction centers by light that has been absorbed by chlorophyll (Ohnishi et al., 2005).
Plants are sessile organisms subjected to daily abiotic stresses that determine detrimental effects on photosynthetic apparatus. Indeed, in addition to high sunlight, stresses such as water or mineral shortage, high and low temperature, heavy metal toxicity and air pollution, can determine at what point the light absorbed by chlorophyll pigments becomes excessive for the requirement of photosynthetic machinery (Murata et al., 2007). The first hypothesis on the effects of environmental stresses on PSII activity suggested that stressors accelerate the photoinhibition to PSII (Björkman and Powles, 1984; Melis, 1999; Adir et al., 2003). Recently, this hypothesis has changed and many researchers have demonstrated that the repair mechanism of PSII is more sensitive to environmental stresses than the process of photodamage itself (Nishiyama et al., 2001; Allakhverdiev and Murata, 2004; Takahashi and Murata, 2008; Kangasjärvi et al., 2012; Nishiyama and Murata, 2014). Photosystem II is the most susceptible component to be damaged in the thylakoid membranes. Therefore the principal result of abiotic stress is to make PSII prone to photoinhibition (Nishiyama et al., 2006). On the contrary, PSI is less frequently damaged due to a very efficient photoprotection mechanism which can prevent photoinhibition (Gururani et al., 2015). Photoinhibition to PSI occurs when the supply of electrons from PSII exceeds its capacity to accept electrons (Tikkanen and Grebe, 2018). The recovery process in PSI, when photodamaged, is prolonged (Tikkanen et al., 2014) and in some cases not wholly reversible (Kudoh and Sonoike, 2002). However, the protective mechanisms involved in PSI photoinhibition are not well-understood, because studied PSI photoinhibition occurred in isolated thylakoids under non-stressed conditions, or in vivo only in specific environmental conditions and species-specific manner (Teicher et al., 2000; Zhang and Scheller, 2004; Sonoike, 2011; Zivcak et al., 2015). Nonetheless, some studies revealed that some factors regulate PSI photoprotection, i.e., the proton gradient dependent or cyt b6f-mediated slow-down of electron transport rate (Joliot and Johnson, 2011; Suorsa et al., 2012) and the light-harvesting of PSII (LHCII)-mediated excitation of PSII and PSI via non-photochemical quenching and the phosphorylation of LCHII (Grieco et al., 2012). In addition to these factors, Tikkanen et al. (2014) reported that PSII photoinhibition slows down the electron transport rate and prevents ROS generation and photodamage to PSI. Moreover, Ballottari et al. (2014) reported a zeaxanthin-dependent regulation of PSI functional antenna size in Arabidopsis thaliana. The authors showed that similar to its action in PSII, zeaxanthin binding to components of PSI leads to the formation of carotenoid radical cations, quenching a portion of the excitation energy absorbed.
The phenomenon of photoinhibition has been studied for well over a century by using multiple biochemical, biophysical and genetic approaches. Probably, chlorophyll fluorescence is one of the most utilized ecophysiological techniques to study the photosynthetic process in plants (Murchie and Lawson, 2013) as evidenced by the high number of user-friendly, non-invasive and portable chlorophyll fluorometers that are available today. However, despite the simplicity of the utilization of chlorophyll fluorometers, the theory and interpretation of data arising from chlorophyll fluorescence measurements is still a complex matter. Many reviews report the theoretical background of chlorophyll fluorescence analysis and a huge body of research reports the utilization of this methodology to provide information related to the photosynthetic process (Maxwell and Johnson, 2000; Baker, 2008; Guidi and Calatayud, 2014; Kalaji et al., 2014, 2017; Guo and Tan, 2015; Ruban, 2016; Stirbet et al., 2018). The first important parameter derived from the Kautsky curve was the Fv/Fm ratio (Krause, 1988) and subsequently, it became a key parameter to detect the PSII photoinhibition induced by a stress factor (Krause and Weis, 1991). To determine this ratio, a weak modulated measuring beam is applied to determine minimal fluorescence yield in a dark-adapted leaf (F0), and a saturating flash is then superimposed to induce the maximal yield of chlorophyll fluorescence (Fm). The ratio Fv/Fm [(Fm -F0)/Fm] represents an estimator of the maximal photochemical efficiency of PSII and is utilized to detect the loss of function of PSII reaction centers (Öquist et al., 1992). Values of Fv/Fm ranges typically between 0.75 and 0.85, and this ratio is proportional to the quantum yield of photochemistry (Kitajima and Butler, 1975). A decline of this ratio is considered to be a good indicator of photoinhibition that may result from two different processes (Öquist et al., 1992): a decrease in the rate constant of PSII photochemistry caused by damages to the PSII reaction centers and/or an increase in the rate constant of non-radiative dissipation of excitation energy. The decrease in the rate constant for PSII photochemistry leads to a rise in initial fluorescence at open PSII traps (F0) whereas an increase in the rate constant of non-radiative energy dissipation leads to a decrease in both initial fluorescence (F0), and maximum fluorescence at closed PSII traps (Fm) (Kitajima and Butler, 1975). However, sometimes the decrease of Fv/Fm ratio is not linearly related to the amount of inactivated PSII reaction centers (Park et al., 1996). Indeed, it is worthwhile to underline that the Fv/Fm ratio is sometimes considered (erroneously) as an indicator of PSII photoinactivation, but this ratio decreases not only due to the closure of PSII reaction centers but also when other processes compete with charge separation such as the thermal dissipation of absorbed light (Malnoë, 2018), as detailed below.
Although the parameters reported above can provide useful information, the introduction of chlorophyll fluorescence quenching analysis has given a further advance in the detection of PSII photoinhibition (van Kooten and Snel, 1990; Bolhàr-Nordenkampf and Öquist, 1993). Quenching analysis permits the separation of the contributions of photochemical and non-photochemical processes in the quenching of variable fluorescence, by inducing a temporary closure of all PSII reaction centers by a strong saturating light pulse (Schreiber et al., 1995; Baker, 2008). The decrease in fluorescence due to photochemistry, i.e., the charge separation, is named photochemical quenching. Regarding photochemistry, the most useful parameter derived from quenching analysis is the measure of the efficiency of PSII (ΦPSII; Genty et al., 1989). Superficially similar to ΦPSII, another parameter which may be derived from quenching analysis is the coefficient of photochemical quenching, qP, that indicates the proportion of open PSII reaction centers (Maxwell and Johnson, 2000). The parameter ΦPSII provides information on the electron transport rate and, differently to the Fv/Fm ratio (determined in dark-adapted conditions) on the nature of photoinhibition. Indeed, the decline in ΦPSII is due to the inactivation of PSII reaction centers aimed at photoprotection (Krause et al., 1990) or may be a mechanism which adjusts the efficiency of PSII to photosynthetic photon flux density (Critchley, 1994). Besides, under light ΦPSII depends on the activity of energy-consuming biochemical reactions of CO2 assimilation (Genty et al., 1989). Besides the proportion of light energy tunneled to photochemistry, quenching analysis can be used to determine the amount of light energy dissipated by alternative mechanisms, namely non-photochemical quenching (Logan et al., 2014). With quenching analysis, it is indeed possible to identify a key parameter, the non-photochemical quenching (NPQ), that represents the fastest process of rapid and reversible thermal dissipation of absorbed light energy in the PSII antenna (Niyogi, 2000; Müller et al., 2001; Horton and Ruban, 2005; Ruban et al., 2012).
Even though NPQ is viewed as a dissipation mechanism into heat, several components are involved: the energy-dependent (qE), zeaxanthin-dependent (qZ) and photoinhibitory quenching (qI) (Derks et al., 2015). Among these mechanisms, qE and qZ are essential for photoprotection while qI could represent the photoinhibitory damage to PSII reaction centers (Ruban et al., 2012). qE is the fastest and most effective component at photoprotecting PSII reaction centers from damage (Nilkens et al., 2010; Goss and Lepetit, 2014). In excess light conditions, a transthylakoidal proton gradient (ΔpH) is generated, activating qE (Noctor et al., 1993). Acidification of thylakoid lumen induces the protonation of the PSII subunit S (PSbS) protein, which then activates violaxanthin epo-oxidase, which in turn converts violaxanthin (Vio) into zeaxanthin (Zea) (Demmig-Adams, 1990). Both PsbS and Zea act as allosteric modulators that increase the sensitivity of LHCII to the lumen protons inducing qE (Horton et al., 2000; Ruban et al., 2012). The coefficient qZ was characterized by Dall’Osto et al. (2005) and Nilkens et al. (2010); it is formed within 10–30 min, and it is independent to PSbS and ΔpH even though strictly dependent to Zea epoxidation and induces conformational changes of the minor antenna protein CP26. qE and qZ, determine changes of the LHCII, while the last quenching coefficient qI is relatively slower to relax (hours or more), and involves a loss in the number of active PSII reaction centers from photoinhibition (Derks et al., 2015). The qI coefficient is the result of photoinhibition (Baker, 1996) and is due predominantly to inactivation and/or degradation of D1 protein. Nevertheless, qI depends only partially to D1 degradation (Demmig and Björkman, 1987; Chow et al., 1989) and this independent portion has been recently named qH (Malnoë et al., 2017) that encompasses different processes. Some of which aimed at photoprotecting, occur in the antenna possibly with similar mechanisms involved in qE and qZ. However, these components act in a different way to dissipate the excess of excitation energy and are involved in the adaptive response to environmental constraints (Malnoë et al., 2017). The qH coefficient gives information on the decrease of the Fv/Fm ratio. Indeed, a decrease in the ratio can be attributable to a high F0 (due to the inactivation of PSI reaction centers or to the antenna detachment), but also to both decrease in F0 and Fm attributable to the presence of qH.
In theory, the components of NPQ can be separated, but practically this not always a simple matter due to the heterogeneity of their kinetics of induction and relaxation that can provide sometimes misleading results, i.e., the overlapping of the recovery time of qZ and qI (Nilkens et al., 2010). Therefore, as both photoinhibition/photodamage and NPQ compromise the measure of ΦPSII (Ruban and Murchie, 2012), a new fluorescence methodology has been developed to assay in vivo the protective potential of NPQ (Ruban and Murchie, 2012; Ruban and Belgio, 2014). The method consists in monitoring photoinhibition that results from the decline in qP in the dark, measured immediately after illumination to determine the parameter qPd. Both qPd and NPQ parameters are utilized to fit ΦPSII. The methodology allows the separation of the effectiveness of photoprotective NPQ from the photoinhibitory effect occurring in leaves (Ruban and Belgio, 2014; Giovagnetti and Ruban, 2015; Ware et al., 2015; Lo Piccolo et al., 2018).
Usually, the term NPQ is related to the dissipation of excess light energy absorbed as heat, even though some mechanisms quench the excess of excitation energy without heat dissipation. Among processes that do not dissipate excess energy as heat (Malnoë, 2018), fluorescence decline can be dependent to chloroplast movement induced by white or blue actinic light (the component qM; Cazzaniga et al., 2013; Dall’Osto et al., 2014). Even the re-distribution of energy between the two photosystems determines a decrease in chlorophyll fluorescence at room temperature: the process results in the II–I state transition and is due to the movement of phosphorylated LHCII away from PSII (Quick and Stitt, 1989). This component of NPQ is termed qT even though it does not contribute significantly to NPQ at saturating light intensities (Nilkens et al., 2010) and it can also be depressed under high light conditions (Mekala et al., 2015). Ferroni et al. (2014) reported in lycophytes an extra-qT mechanism that could reflect the use of PSI as an energy quencher for PSII through an energy spillover mechanism. Interestingly, energy spillover to PSI was also suggested to be functionally relevant in angiosperms, in particular when the light intensity becomes so high that the plant’s capacity for qE is saturated, and is therefore insufficient for effective photoprotection (Ferroni et al., 2016; Tiwari et al., 2016).
In conclusion, PSII photoinhibition represents a mechanism by which plants limit the photodamage to PSII but also preserves PSI which is not equipped with its own repair mechanisms (Sonoike, 2011; Tikkanen et al., 2014; Järvi et al., 2015). In conclusion, photoinhibition of PSII was previously considered as a solely negative mechanism to limit photodamage to PSII, effectively limiting the photosynthetic process. The new perspective of PSII photoinhibition also understands it as a means to protect PSI, which is not equipped with its own repair mechanisms.
Photoinhibition in C3 and C4 Species
It is generally accepted that C4 plants such as maize, sorghum, and sugarcane have impressively higher levels of photosynthetic efficiency than most C3 species, such as wheat and rice (Kajala et al., 2011). This is attributable to the different mechanism of carbon fixation connected to both morpho-anatomical and biochemical differences existing between C3 and C4 species. C3 photosynthesis only uses the Calvin cycle to fix CO2, an event which is catalyzed by ribulose-1,5-bisphosphate carboxylase (Rubisco) and takes place inside of the chloroplasts of mesophyll cells (MC). Conversely, in C4 species the photosynthetic activities are partitioned between mesophyll and bundle sheath cells (BSC) that are anatomically and biochemically distinct. In C4 photosynthesis, the first step of carbon fixation is catalyzed by phosphoenolpyruvate (PEP) carboxylase which conjugates CO2 to PEP forming oxaloacetate (OAA). Then, OAA is reduced to malate, which then diffuses into the BSC where it is decarboxylated, and CO2 enters the Calvin cycle. In this way, the C4 photosynthetic metabolism operates a CO2-concentrating mechanism around Rubisco, which in turn significantly reduces the Rubisco oxygenase activity. In addition, BSC chloroplasts contain low level of PSII (Romanowska et al., 2017) and, although in BSC chloroplasts PSII contains all polypeptides involved in electron transport and oxygen evolution, it is largely inactive (Romanowska et al., 2008). In contrast, MC chloroplasts have higher PSII activity but practically absent Rubisco activity (Furbank and Foyer, 1988).
The metabolic mechanism in C4 species increases the energy utilization efficiency by reducing the subsequent energy loss due to photorespiration, as occurs in C3 species, which results in higher photosynthetic performances and water use efficiency of C4 when compared to C3 metabolism (Majeran et al., 2010). This CO2 concentrating mechanism in C4 metabolism is thought to have evolved in response to declining atmospheric CO2 concentrations over geological time scales (Ehleringer et al., 1991). A higher water use efficiency is attributable to the fact that the CO2 concentrating mechanism allows C4 species to maintain a large diffusion gradient for CO2 and operate at lower leaf conductance than C3 species, thereby reducing water loss by transpiration (Long, 1999). Besides these general features, C4 species are further classified into subtypes according to decarboxylation reaction that they utilized, a feature which makes an additional difference in their energy use efficiency and, in turn, their photosynthetic yield: NADP-malic enzyme (NADP-ME), NAD-malic enzyme (NAD-ME) and PEP carboxykinase (PEPCK) (Hatch, 1987). However, no “pure” PEPCK-type C4 species have been actually described, and currently NAD-ME (e.g., Panicum virgatum, Pennisetum glaucum, Amaranthus spp.) and NADP-ME subtypes (e.g., Zea mays, Saccharum spp., and Sorghum bicolor) are suggested as distinct C4 biochemical pathways, both with or without the additional service of the PEPCK pathway (Rao and Dixon, 2016). All these features make C4 species better adapted to high light intensities and high temperatures, and therefore C4 species are mainly present in warmer areas, such as the tropical/subtropical regions (Edwards et al., 2010). Given that different abiotic stressors (e.g., drought, salinity, and cold) lead in most cases to a surplus of energy absorbed by the leaves in relation to a stress-altered CO2 assimilation, we might expect C4 species to be less prone to photoinhibition and photodamage than C3 under abiotic stress, especially under limited water availability (i.e., drought and salinity). But is this the truth? And connected to this, why do C4 species curiously only represent less than 4% of the total world’s flora (Ghannoum, 2009)? And “why are there no C4 forests?” (Sage and Sultmanis, 2016).
Unfortunately, there is little information concerning the comparison between C3 and C4 photosynthetic performances under the same stressful conditions, and even less information is available on the susceptibility of photoinhibition in C4 versus C3 species subjected to abiotic stressors. Besides the difficulty of drawing a clear picture between C4 and C3 photosynthesis under stress which arises from differences of experimental conditions, the situation is further complicated by the different C4 metabolisms (NADP-ME, NAD-ME, and PEPCK). Below, on the basis of the inherent differences between C3 and C4 photosynthesis, and revising the few reports on the subject, we attempt to solve the question as to whether C4 species are or are not less prone to photoinhibition when exposed to abiotic stresses. We are aware that (i) different results about the inherently lower or similar values of Fv/Fm ratio in C4 than C3 species (Table 1) and (ii) different experimental conditions, sometimes more favorable to C3 or C4 species, further complicate the comparison and (iii) MC present greater photodamage than BSC (Pokorska et al., 2009).
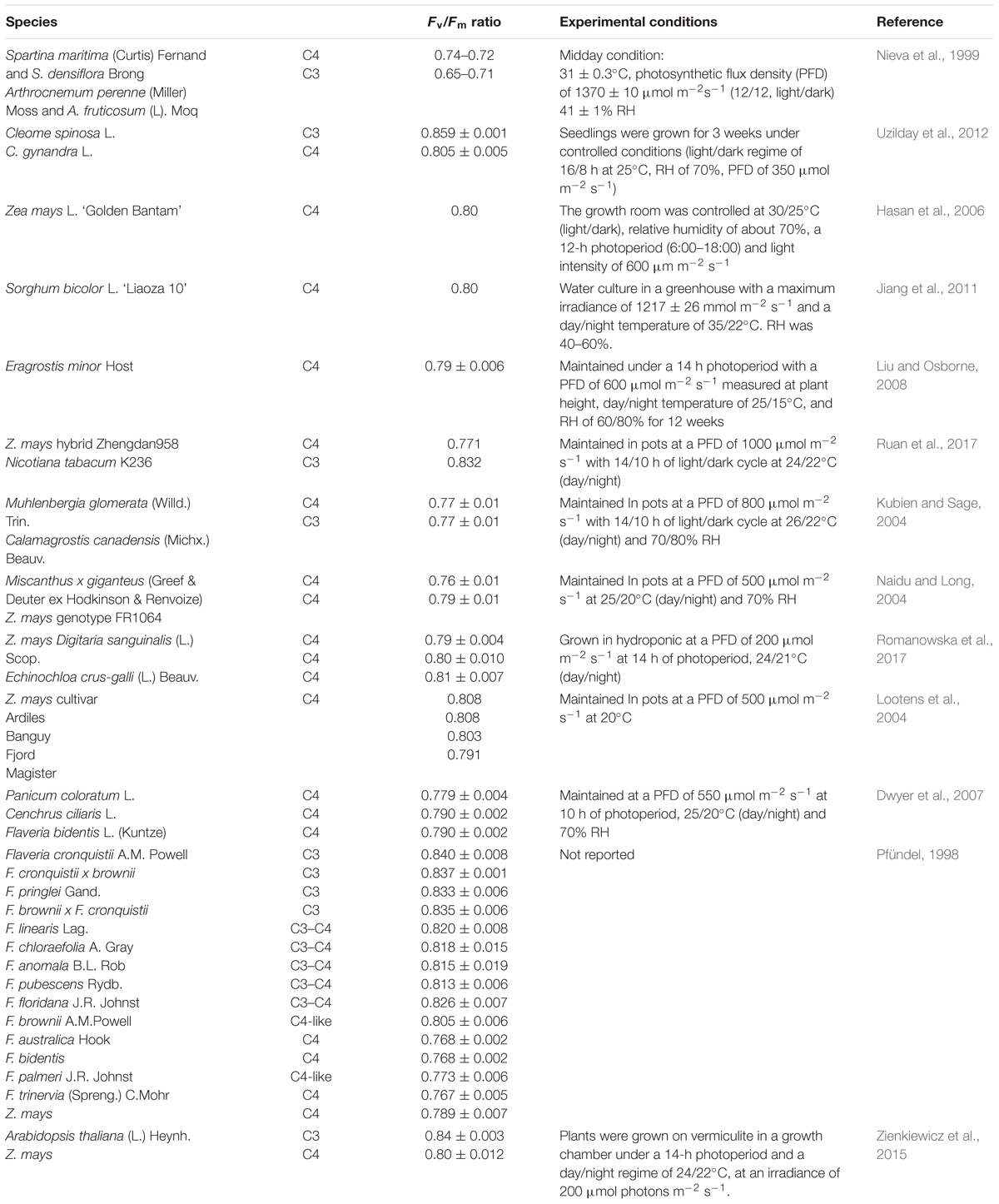
Table 1. Values of the Fv/Fm ratio in C3, C3-C4, and C4 species determined in different experimental conditions.
As explained above, water use efficiency is typically higher in C4 than C3 species, and it is linked to the CO2 concentrating mechanisms in C4 photosynthetic metabolism (Downes, 1969). However, stomatal limitation posed by a stressor (e.g., drought) potentially induces a reduction in photosynthetic CO2 uptake at a large extent in C4 species than in C3 species, because C4 photosynthesis operates at (or close to) the inflection point of the photosynthetic CO2 response (Wand et al., 2001). Under drought-controlled conditions, Ripley et al. (2007) demonstrated that, besides stomatal limitations, a lower CO2 assimilation rate in C4 versus C3 subspecies of Alloteropsis semialata was caused by a lower linear electron flux and a reduction of PSII photochemical efficiency under water limitation, rather than in differences between increased electron flux toward alternative sinks. Similarly, Killi et al. (2017) found that different maize genotypes had a higher decline of Fv/Fm and ΦPSII than sunflower genotypes under water stress conditions. The authors also observed that the combination of drought and high temperature (35°C) resulted again in higher photoinhibition and a lower PSII efficiency in maize than sunflower, thereby suggesting that C4 species are poorer performers than C3 species under stressful conditions, even at a temperature for which the C3 metabolism should be disadvantaged. In other cases, it has been observed that the limited capacity for photorespiration or the Mehler reaction (which act as significant alternative electron sinks in C3 species) was deleterious under water stress, a condition in which the absorbed light largely exceeded the carboxylation energy requirement (Ghannoum, 2009). Practically, this may explain why C4 is equally or in most cases even more sensitive to water stress than C3 photosynthesis (despite the greater water use efficiency of the C4 species), and clarify the paradox of why C4 species abundance declines in parallel with decreasing in annual rainfall (Paruelo and Lauenroth, 1996; Tieszen et al., 1997).
Another well-known factor that increases the probability of photoinhibition in plants is the exposure to low temperatures, especially when occurring in conjunction with high light (Pietrini and Massacci, 1998), but possible differences in C3 versus C4 species are less obvious. C4 species typically have a reduced amount of Rubisco in BSC chloroplasts as compared to C3 MC, and therefore BSC reactions can represent a key limiting factor at low temperature, a condition in which the CO2 solubility, and consequently, its diffusion is reduced (Kubien et al., 2003). Furthermore, C3 plants have a higher maximum quantum yield of CO2 than C4 plants below 30°C (Ehleringer and Pearcy, 1983), which may permit C3 plants to maintain higher rates of CO2 fixation at low temperatures (Ehleringer, 1978). Under both high light and low temperature, the cold-adapted species Muhlenbergia glomerata (C4) and Calamagrostis canadensis (C3) showed a similar susceptibility to photoinhibition (Kubien and Sage, 2004) and this seems attributable to the ability of cold-adapted C4 species to activate the xanthophyll cycle (Kubien and Sage, 2004). Of note, although it is a reversible effect, such dynamic photoinhibition may reduce the photosynthetic performances of C4 in cold climates, thus reducing their competitiveness with C3 cold-adapted species and explaining their reduced distribution at high latitude and elevations.
What about the low relative abundance of C4 species and near-absence of C4 trees (Sage and Sultmanis, 2016)? Among other explanations (phenotypical, ecological, and evolutionary), the physiological hypothesis proposed C4 species to have a lower photosynthetic ability than C3 species in shade and/or fluctuating light conditions, such as in forest understoreys. In particular, it is conceivable that the main limiting factors consist of a lower quantum yield for CO2 (Ehleringer, 1978) and a reduced ability to use the fluctuating light efficiently (Kubásek et al., 2013). In fact, Kubásek et al. (2013) demonstrated that C3 and C4 species did not display photoinhibition when subjected to 950 μmol m-2s-1 light; however, only C4 species displayed photoinhibition (evidenced by the slight but significant reduction in Fv/Fm ratio) when the same photon flux density was provided by simulated light fleck conditions over the whole experimental period. This was attributable to the photoinactivation of PSII and, in parallel, the activation of photoprotective mechanisms in C4 species. The photosynthetic metabolism in C4 species also responds less promptly to fluctuating light conditions because it requires more enzymatic steps than C3 to be light activated and to promote the metabolite gradient between MC and BSC (Sage and Pearcy, 2000). In addition, deactivation of Calvin cycle enzymes during low-light periods is faster in C4 than C3 species, causing faster reduction of CO2 quantum yield in C4 species in dynamic light conditions (Horton and Neufeld, 1998). Noteworthy, in C4 species under fluctuating light the CO2 quantum yield is additionally reduced by the increment of CO2 ‘leakiness’ in BSC, i.e., the proportion of CO2 fixed by PEPC in the mesophyll that leaks from BSC to the mesophyll without being re-fixed by Rubisco (Tazoe et al., 2008). These factors result in C4 species being inferior competitors to C3 species in conditions of shade or low-light conditions (flecked and dappled), and it can explain why C4 species are excluded from the ecological niche of the understory and why there are no C4 forests, an aspect which, besides biochemical limitations, seems principally attributable to a low phenotypic plasticity of C4 than C3 (Sage and McKown, 2006) species correlated with their shorter evolutionary history (Sage and Sultmanis, 2016).
Actual Limits and Future Perspectives
As clearly evident by the two sections of this review, a gap exists between advances in chlorophyll fluorescence and the application of chlorophyll fluorescence parameters to improve knowledge on the mechanisms involved in photoprotection in C3 against C4 species. Surely, different morpho-anatomical and biochemical features in C4 compared with C3 species (and even different C4 metabolisms) further complicate this type of comparison. For this reason, we summarize below the main differences which actually limit the comparison between C3 and C4 metabolisms, propose the direction for future research toward this goal, and propose how chlorophyll fluorescence may assist in this research.
Firstly, while the ‘lack’ of photorespiration in C4 leaves increases the efficiency of Rubisco activity strongly, photorespiration is also essential for the protection of the photosynthetic apparatus of plants against photoinactivation under high irradiance (Heber et al., 1996). While photorespiration is assumed to be almost absent in C4 species under optimal conditions, it does appear to increase under conditions of limited CO2 availability, which can result from stresses which limit stomatal conductance (e.g., drought and salinity). For example, when wheat and maize plants were compared under 340 μbar CO2, photorespiration is fivefold higher in wheat as compared to maize. However, when the CO2 concentration was severely reduced (50 μbar intercellular CO2 concentration) photorespiration was substantially increased in both species (Dai et al., 1993). In contrast, studies have also reported that photorespiration did not contribute to reduced carbon gain in C4 species (Carmo-Silva et al., 2008; Lopes et al., 2011). In view of the higher involvement of the Mehler reaction in C4 species (compared to photorespiration) even at low O2 concentration (Laisk and Edwards, 1998), possible differences in the role of alternative sinks in C3 and C4 species should be considered. These factors show that there is a need to clarify the contribution of photorespiration as a possible alternative sink of electron transport rate when attempting to describe the intricate differences between C3 and C4 metabolisms under stomata-constraining stressors.
Secondly, gradients of PSI/PSII ratios differ substantially between C3 MC, C4 MC, and BSC, and even within C4 NAD-ME/NADP-ME metabolisms (Pfundel and Neubohn, 1999). This has serious consequences when attempting to describe the minute differences between the photochemical and non-photochemical processes in the thylakoid membranes of C3, C3-C4, and C4 species. Indeed, one should consider that values of Fv/Fm are usually under-estimated because of the contribution of PSI to the overall signal of chlorophyll fluorescence (Pfündel, 1998), even at room temperature where PSI fluorescence was thought to be negligible (Krause and Weis, 1991). However, at room temperature it has been reported that the contribution of PSI fluorescence influenced both F0 and Fm in C3 and C4 species (Pfündel, 1998). This effect was consistent in C3, C3-C4, and C4 species, but in view of the relatively higher abundance of PSI in C4 species, F0 fluorescence accounted for 30% in C3 and 50% in C4 species (Pfündel, 1998). The abovementioned was consistent with the observation of a low chlorophyll fluorescence emission by PSII in NADP-ME species versus NAD-ME species (Takabayashi et al., 2005; Kirchhoff et al., 2013), the former having much lower levels of PSII in BSC. Therefore, we believe that for future research it is of crucial importance to deepen our understanding of influences to the photochemistry of PSI, for example by NIR-absorbance changes of PSI measured by the pulse amplitude modulation technique. In addition, it has been recently demonstrated that it is possible to differentiate between changes occurring to PSI, plastocyanin (PC) and ferredoxin (Fd) by a newly developed measuring system which combines the measure of chlorophyll fluorescence with the assessment of changes in NIR spectral regions (Klughammer and Schreiber, 2016). This allows the direct measurement of P700/PC, which has been demonstrated to strongly influence the rate of photosynthesis (Schöttler and Tóth, 2014), and provides for the first time direct measurement of Fd-dependent cyclic electron flow in vivo (Klughammer and Schreiber, 2016). The latter aspect is particularly relevant not only when comparing C3 versus C4 species, but also when comparing NAD-ME versus NADP-ME metabolisms, which are characterized by a different contribution between the two known pathways of cyclic electron flows around PSI: chloroplastic NAD(P)H dehydrogenase (NDH)-dependent flow and Fd-dependent flow (Takabayashi et al., 2005). In the past, the NDH-dependent pathway was measured by transient increase in chlorophyll fluorescence of PSII after turning off actinic illumination. However, due to the reduction of the plastoquinone pool by the reducing equivalents accumulated during actinic illumination (Takabayashi et al., 2005), it was impossible to assess the contribution of the Fd-dependent cyclic flow by chlorophyll fluorescence measurements.
Thirdly, when considering the different proportion between PSI/PSII ratios in C3 and C4 cells, it should be taken into account that the preferential distribution of PSII in stacked thylakoid regions (Dekker and Boekema, 2005) can significantly influence the LHCII mobility and the rate of repair of PSII when photodamaged, given that protein complexes in stacked regions (included LHCII and PSII components) have about a twofold lower mobility than unstacked regions of thylakoid membranes (Kirchhoff et al., 2013). In C3 species, photodamage to PSII occurs in stacked regions whereas the repairing mechanisms occur in unstacked regions, therefore requiring the mobility of PSII components (Tikkanen and Aro, 2012). This type of spatial separation between photodamage and repairing of PSII is not present in agranal BSC of C4 species, and it can explain the faster repairing of PSII in agranal BSC than MC in maize (Pokorska and Romanowska, 2007). As a consequence, despite NADP-ME metabolism is usually devoted to the production of ATP by cyclic electron transport and BSC are enriched in PSI and depleted of PSII, the faster capacity of the PSII repair process, connected to the PSII–PSI electron flux, should be considered when comparing C3 versus C4 species.
Conclusion
The superiority of C4 over C3 photosynthesis under high light and elevated temperature is doubtless. Conversely, C4 species can be inferior competitors when subjected to other abiotic stressors, such as drought or cold, due to a higher susceptibility for photoinhibition. Assisted by chlorophyll fluorescence, future investigations should improve the knowledge on the different sensitivity of C3 and C4 to abiotic stressors; clarify as to whether photoinhibition occurs more frequently as a chronic or reversible process in C4; and deepen the understanding of light-induced reactions of C3 and C4 metabolisms with particular emphasis to the PSI photochemistry. This would give new perspectives and insights on the knowledge of C4 metabolism(s) under stress.
Author Contributions
All authors listed have made a substantial, direct and intellectual contribution to the work, and approved it for publication.
Funding
cpp18re fee total waiver provided by frontiers editorial office (Ines Pires).
Conflict of Interest Statement
The authors declare that the research was conducted in the absence of any commercial or financial relationships that could be construed as a potential conflict of interest.
Acknowledgments
We are grateful to Mr. Kramer-Walter for the critical revision of the manuscript.
References
Adir, N., Zer, H., Shochat, S., and Ohad, I. (2003). Photoinhibition: a historical perspective. Photosynth. Res. 76, 343–370. doi: 10.1023/A:1024969518145
Allakhverdiev, S. I., and Murata, N. (2004). Environmental stress inhibits the synthesis de novo of proteins involved in the photodamage-repair cycle of photosystem II in Synechocystis sp. PCC 6803. Biochim. Biophys. Acta 1657, 23–32.
Aro, E. M., Virgin, I., and Andersson, B. (1993). Photoinhibition of Photosystem II. Inactivation, protein damage and turnover. Biochim. Biophys. Acta 1143, 113–134. doi: 10.1016/0005-2728(93)90134-2
Baker, N. R. (1996). “Photoinhibition of Photosynthesis,” in Light as an Energy Source and Information Carrier in Plant Physiology, eds R. C. Jennings, G. Zucchelli, F. Ghetti, and G. Colombetti (New York, NY: Plenum Press), 89–97. doi: 10.1007/978-1-4613-0409-8_7
Baker, N. R. (2008). Chlorophyll fluorescence: a probe of photosynthesis in vivo. Annu. Rev. Plant Biol. 59, 89–113. doi: 10.1146/annurev.arplant.59.032607.092759
Ballottari, M., Alcocer, M. J., D’Andrea, C., Viola, D., Ahn, T. K., Petrozza, A., et al. (2014). Regulation of PSI light harvesting by zeaxanthin. Proc. Natl. Acad. Sci. U.S.A. 111, E2431–E2438. doi: 10.1073/pnas.1404377111
Björkman, O., and Powles, S. B. (1984). Inhibition of photosynthetic reactions under water stress: interaction with light level. Planta 161, 490–504. doi: 10.1007/BF00407081
Bolhàr-Nordenkampf, H. R., Öquist, G., and Long, S. P. (1993). “Chlorophyll fluorescence as a tool in photosynthesis research,” in Photosynthesis and Production in a Changing Environment, eds D. O. Hall, J. M. O. Scurlock, H. R. Bolhàr-Nordenkampf, and R. C. Leegood (Dordrecht: Springer), 193–206.
Carmo-Silva, A. E., Powers, S. J., Keys, A. J., Arrabaça, M. C., and Parry, M. A. J. (2008). Photorespiration in C4 grasses remains slow under drought conditions. Plant Cell Environ. 31, 925–940. doi: 10.1111/j.1365-3040.2008.01805.x
Cazzaniga, S., Dall’Osto, L., Kong, S. G., Wada, M., and Bassi, R. (2013). Interaction between avoidance of photon absorption, excess energy dissipation and zeaxanthin synthesis against photooxidative stress in Arabidopsis. Plant J. 76, 568–579. doi: 10.1111/tpj.12314
Chow, W. S., Osmond, C. B., and Huang, L. K. (1989). Photosystem II function and herbicide binding sites during photoinhibition of spinach chloroplasts in-vivo and in-vitro. Photosynth. Res. 21, 17–26. doi: 10.1007/BF00047171
Critchley, C. (1994). “D1 protein turnover: response to photodamage or regulatory mechanism?,” in Photoinhibition of Photosynthesis, eds N. R. Baker and J. R. Bowyer Jr. (Oxford: Bios Scientific), 195–203.
Dai, Z., Ku, M. S. B., and Edwards, G. E. (1993). C4 photosynthesis. The CO2-concentrating mechanism and photorespiration. Plant Physiol. 103, 83–90. doi: 10.1104/pp.103.1.83
Dall’Osto, L., Caffarri, S., and Bassi, R. (2005). A mechanism of nonphotochemical energy dissipation, independent from PsbS, revealed by a conformational change in the antenna protein CP26. Plant Cell 17, 1217–1232. doi: 10.1105/tpc.104.030601
Dall’Osto, L., Cazzaniga, S., Wada, M., and Bassi, R. (2014). On the origin of a slowly reversible fluorescence decay component in the Arabidopsis npq4 mutant. Philos. Trans. R. Soc. Lond. B Biol. Sci. 369:20130221. doi: 10.1098/rstb.2013.0221
Dekker, J. P., and Boekema, E. J. (2005). Supramolecular organization of thylakoid membrane proteins in green plants. Biochim. Biophys. Acta 1706, 12–39. doi: 10.1016/j.bbabio.2004.09.009
Demmig, B., and Björkman, O. (1987). Comparison of the effect of excessive light on chlorophyll fluorescence (77 K) and photon yield of O2 evolution in leaves of higher plants. Planta 171, 171–184. doi: 10.1007/BF00391092
Demmig-Adams, B. (1990). Carotenoids and photoprotection: a role for the xanthophyll zeaxanthin. Biochim. Biophys. Acta 1020, 1–24. doi: 10.1016/0005-2728(90)90088-L
Demmig-Adams, B., Cohu, C. M., Muller, O., and Adams, W. W. III. (2012). Modulation of photosynthetic energy conversion efficiency in nature: from seconds to seasons. Photosynth. Res. 113, 75–88. doi: 10.1007/s11120-012-9761-6
Derks, A., Schaven, K., and Bruce, D. (2015). Diverse mechanisms for photoprotection in photosynthesis. Dynamic regulation of photosystem II excitation in response to rapid environmental change. Biochim. Biophys. Acta 1847, 468–485. doi: 10.1016/j.bbabio.2015.02.008
Downes, R. W. (1969). Differences in transpiration rates between tropical and temperate grasses under controlled conditions. Planta 88, 261–273. doi: 10.1007/BF00385069
Dwyer, S. A., Ghannoum, O., Nicotra, A., and Von Caemmerer, S. (2007). High temperature acclimation of C4 photosynthesis is linked to changes in photosynthetic biochemistry. Plant Cell Environ. 30, 53–66. doi: 10.1111/j.1365-3040.2006.01605.x
Edwards, E. J., Osborne, C. P., Strömberg, C. A., Smith, S. A., and C4 Grasses Consortium. (2010). The origins of C4 grasslands: integrating evolutionary and ecosystem science. Science 328, 587–591. doi: 10.1126/science.1177216
Ehleringer, J., and Pearcy, R. W. (1983). Variation in quantum yield for CO2 uptake among C3 and C4 plants. Plant Physiol. 73, 555–559. doi: 10.1104/pp.73.3.555
Ehleringer, J. R. (1978). Implications of quantum yield differences on the distributions of C3 and C4 grasses. Oecologia 31, 255–267. doi: 10.1007/BF00346246
Ehleringer, J. R., Sage, R. F., Flanagan, L. B., and Pearcy, R. W. (1991). Climate change and the evolution of C4 photosynthesis. Trends Ecol. Evol. 6, 95–99. doi: 10.1016/0169-5347(91)90183-X
Ferroni, L., Angeleri, M., Pantaleoni, L., Pagliano, C., Longoni, P., Marsano, F., et al. (2014). Light-dependent reversible phosphorylation of the minor photosystem II antenna Lhcb6 (CP24) occurs in lycophytes. Plant J. 77, 893–905. doi: 10.1111/tpj.12437
Ferroni, L., Suorsa, M., Aro, E.-M., Baldisserotto, C., and Pancaldi, S. (2016). Light acclimation in the lycophyte Selaginella martensii depends on changes in the amount of photosystems and on the flexibility of the light-harvesting complex II antenna association with both photosystems. New Phytol. 211, 554–568. doi: 10.1111/nph.13939
Furbank, R. T., and Foyer, C. H. (1988). C4 plants as valuable model experimental systems for the study of photosynthesis. New Phytol. 109, 265–277. doi: 10.1042/BST20150148
Genty, B., Briantais, J.-M., and Baker, N. R. (1989). The relationship between quantum yield of photosynthetic electron transport and quenching of chlorophyll fluorescence. Biochim. Biophys. Acta 990, 87–92. doi: 10.1016/S0304-4165(89)80016-9
Ghannoum, O. (2009). C4 photosynthesis and water stress. Ann. Bot. 103, 635–644. doi: 10.1093/aob/mcn093
Giovagnetti, V., and Ruban, A. V. (2015). Discerning the effects of photoinhibition and photoprotection on the rate of oxygen evolution in Arabidopsis leaves. J. Photochem. Photobiol. 152, 272–278. doi: 10.1016/j.jphotobiol.2015.09.010
Goss, R., and Lepetit, B. (2014). Biodiversity of NPQ. J. Plant Physiol. 172, 13–32. doi: 10.1016/j.jplph.2014.03.004
Grieco, M., Tikkanen, M., Paakkarinen, V., Kangasjärvi, S., and Aro, E. M. (2012). Steady-state phosphorylation of light-harvesting complex ii proteins preserves Photosystem I under fluctuating white light. Plant Physiol. 160, 1896–1910. doi: 10.1104/pp.112.206466
Guidi, L., and Calatayud, A. (2014). Non-invasive tools to estimate stress-induced changes in photosynthetic performance in plants inhabiting Mediterranean areas. Environ. Exp. Bot. 103, 42–52. doi: 10.1016/j.envexpbot.2013.12.007
Guo, Y., and Tan, J. (2015). Recent advances in the application of chlorophyll a fluorescence from Photosystem II. Photochem. Photobiol. 91, 1–14. doi: 10.1111/php.12362
Gururani, M. A., Venkatesh, J., and Tran, L. S. P. (2015). Regulation of photosynthesis during abiotic stress-induced photoinhibition. Mol. Plant 8, 1304–1320. doi: 10.1016/j.molp.2015.05.005
Hasan, R., Kawasaki, M., Taniguchi, M., and Miyake, H. (2006). Salinity stress induces granal development in bundle sheath chloroplasts of maize, an NADP-malic enzyme-type C4 plant. Plant Prod. Sci. 9, 256–265. doi: 10.1626/pps.9.256
Hatch, M. D. (1987). C4 photosynthesis: a unique elend of modified biochemistry, anatomy and ultrastructure. Biochim. Biophys. Acta 895, 81–106. doi: 10.1016/S0304-4173(87)80009-5
Heber, U., Bligny, R., Streb, P., and Douce, R. (1996). Photorespiration is essential for the protection of the photosynthetic apparatus of C3 plants against photoinactivation under sunlight. Plant Biol. 109, 307–315. doi: 10.1111/j.1438-8677.1996.tb00578.x
Horton, J. L., and Neufeld, H. S. (1998). Photosynthetic responses of Microstegium vimineum (Trin.) A. Camus, a shade-tolerant, C4 grass, to variable light environments. Oecologia 114, 11–19. doi: 10.1007/s004420050414
Horton, P., and Ruban, A. V. (2005). Molecular design of the photosystem II light-harvesting antenna: photosynthesis and photoprotection. J. Exp. Bot. 56, 365–373. doi: 10.1093/jxb/eri023
Horton, P., Ruban, A. V., and Wentworth, M. (2000). Allosteric regulation of the light harvesting system of photosystem II. Philos. Trans. R. Soc. B 355, 1361–1370. doi: 10.1098/rstb.2000.0698
Järvi, S., Suorsa, M., and Aro, E. M. (2015). Photosystem II repair in plant chloroplasts. Regulation, assisting proteins and shared components with photosystem II biogenesis. Biochim. Biophys. Acta 1847, 900–909. doi: 10.1016/j.bbabio.2015.01.006
Jiang, C. D., Wang, X., Gao, H.-Y., Shi, L., and Chow, W. S. (2011). Systemic regulation of leaf anatomical structure, photosynthetic performance, and high-light tolerance in sorghum. Plant Physiol. 155, 1416–1424. doi: 10.1104/pp.111.172213
Joliot, P., and Johnson, G. N. (2011). Regulation of cyclic and linear electron flow in higher plants. Proc. Natl. Acad. Sci. U.S.A. 108, 13317–13322. doi: 10.1073/pnas.1110189108
Kajala, K., Covshoff, S., Karki, S., Woodfield, H., Tolley, B. J., Dionora, M. J., et al. (2011). Strategies for engineering a two-celled C4 photosynthetic pathway into rice. J. Exp. Bot. 62, 3001–3010. doi: 10.1093/jxb/err022
Kalaji, H. M., Schansker, G., Brestic, N., Bussotti, F., Calatayud, A., Ferroni, L., et al. (2017). Frequently asked questions about chlorophyll fluorescence, the sequel. Photosynth. Res. 132, 13–66. doi: 10.1007/s11120-016-0318-y
Kalaji, H. M., Schansker, G., Ladle, R. J., Goltsev, V., Bosa, K., Allakhverdiev, S. I., et al. (2014). Frequently asked questions about in vivo chlorophyll fluorescence: practical issues. Photosynth. Res. 122, 121–158. doi: 10.1007/s11120-014-0024-6
Kangasjärvi, S., Neukermans, J., Li, S., Aro, E.-M., and Noctor, G. (2012). Photosynthesis, photorespiration, and light signalling in defence responses. J. Exp. Bot. 63, 1619–1636. doi: 10.1093/jxb/err402
Killi, D., Bussotti, F., Raschi, A., and Haworth, M. (2017). Adaptation to high temperature mitigates the impact of water deficit during combined heat and drought stress in C3 sunflower and C4 maize varieties with contrasting drought tolerance. Physiol. Plant 159, 130–147. doi: 10.1111/ppl.12490
Kirchhoff, H., Sharpe, R. M., Herbstova, M., Yarbrough, R., and Edwards, G. E. (2013). Differential mobility of pigment-protein complexes in granal thylakoid membranes in C3 and C4 plants. Plant Physiol. 161, 497–507. doi: 10.1104/pp.112.207548
Kitajima, M., and Butler, W. L. (1975). Quenching of chlorophyll fluorescence and primary photochemistry in chloroplasts by dibromothymoquinone. Biochim. Biophys. Acta 376, 105–115. doi: 10.1016/0005-2728(75)90209-1
Klughammer, C., and Schreiber, U. (2016). Deconvolution of ferredoxin, plastocyanin, and P700 transmittance changes in intact leaves with a new type of kinetic LED array spectrophotometer. Photosynth. Res. 128, 195–214. doi: 10.1007/s11120-016-0219-0
Krause, G. H. (1988). Photoinhibition of photosynthesis. An evaluation of damaging and protective mechanisms. Physiol. Plantarum 74, 566–574. doi: 10.1111/j.1399-3054.1988.tb02020.x
Krause, G. H., Somersalo, S., Zumbusch, E., Weyers, B., and Laasch, H. (1990). On the mechanism of photoinhibition in chloroplasts. Relationship between changes in fluorescence and activity of Photosystem II. J. Plant Physiol. 136, 472–479. doi: 10.1016/S0176-1617(11)80038-6
Krause, G. H., and Weis, E. (1991). Chlorophyll fluorescence and photosynthesis: the basics. Annu. Rev. Plant Physiol. Plant Mol. Biol. 42, 313–349. doi: 10.1146/annurev.pp.42.060191.001525
Kubásek, J., Urban, O., and Šantrůček, J. (2013). C4 plants use fluctuating light less efficiently than do C3 plants: a study of growth, photosynthesis and carbon isotope discrimination. Physiol. Plant 149, 528–539. doi: 10.1111/ppl.12057
Kubien, D. S., and Sage, R. F. (2004). Dynamic photo-inhibition and carbon gain in a C4 and a C3 grass native to high latitudes. Plant Cell Environ. 27, 1424–1435. doi: 10.1111/j.1365-3040.2004.01246.x
Kubien, D. S., von Caemmerer, S., Furbank, R. T., and Sage, R. F. (2003). C4 photosynthesis at low temperature: a study using transgenic plants with reduced amounts of Rubisco. Plant Physiol. 132, 1577–1585. doi: 10.1104/pp.103.021246
Kudoh, H., and Sonoike, K. (2002). Irreversible damage to photosystem I by chilling in the light: cause of the degradation of chlorophyll after returning to normal growth temperature. Planta 215, 541–548. doi: 10.1007/s00425-002-0790-9
Laisk, A., and Edwards, G. E. (1998). Oxygen and electron flow in C4 photosynthesis: mehler reaction, photorespiration and CO2 concentration in the bundle sheath. Planta 205, 632–645. doi: 10.1007/s004250050366
Liu, M.-Z., and Osborne, C. P. (2008). Leaf cold acclimation and freezing injury in C3 and C4 grasses of the mongolian plateau. J. Exp. Bot. 59, 4161–4170. doi: 10.1093/jxb/ern257
Lo Piccolo, E., Landi, M., Pellegrini, E., Agati, G., Giordano, C., Giordani, T., et al. (2018). Multiple consequences induced by epidermally-located anthocyanins in young, mature and senescent leaves of Prunus. Front. Plant Sci. 9:917. doi: 10.3389/fpls.2018.00917
Logan, B. A., Demmig-Adams, B., Adams, W. W., and Bilger, W. (2014). “Context, quantification, and measurement guide for non-photochemical quenching of chlorophyll fluorescence,” in Non-Photochemical Quenching and Energy Dissipation in Plants, Algae and Cyanobacteria, eds B. Demmig-Adams, G. Garab, W. W. Adams, and Govindjee (Dordrecht: Springer), 187–201. doi: 10.1007/978-94-017-9032-1_7
Long, S. P. (1999). “Environmental responses,” in C4 Plant Biology, eds R. F. Sage and R. K. Monson (Toronto: Academic Press), 215–249. doi: 10.1016/B978-012614440-6/50008-2
Lootens, P., Van Waes, J., and Carlier, L. (2004). Effect of a short photoinhibition stress on photosynthesis, chlorophyll a fluorescence, and pigment contents of different maize cultivars. can a rapid and objective stress indicator be found? Photosynthetica 42, 187–192. doi: 10.1023/B:PHOT.0000040589.09614.a0
Lopes, M. S., Araus, J. L., van Heerden, P. D. R., and Foyer, C. H. (2011). Enhancing drought tolerance in C4 crops. J. Exp. Bot. 62, 3135–3153. doi: 10.1093/jxb/err105
Majeran, W., Friso, G., Ponnala, L., Connolly, B., Huang, M., Reidel, E., et al. (2010). Structural and metabolic transitions of C4 leaf development and differentiation defined by microscopy and quantitative proteomics in maize. Plant Cell 22, 3509–3542. doi: 10.1105/tpc.110.079764
Malnoë, A., Schultink, A., Shahrasbi, S., Rumeau, D., Havaux, M., and Niyogi, K. K. (2017). The plastid lipocalin LCNP is required for sustained photoprotective energy dissipation in arabidopsis. Plant Cell 30, 196–208. doi: 10.1105/tpc.17.00536
Malnoë, M. (2018). Photoinhibition or photoprotection of photosynthesis? Update on the (newly termed) sustained quenching component qH. Environ. Exp. Bot. 154, 123–133. doi: 10.1016/j.envexpbot.2018.05.005
Maxwell, K., and Johnson, G. N. (2000). Chlorophyll fluorescence - a practical guide. J. Exp. Bot. 51, 659–668. doi: 10.1093/jexbot/51.345.659
Mekala, N. R., Suorsa, M., Rantala, M., Aro, E. M., and Tikkanen, M. (2015). Plants actively avoid state transitions upon changes in light intensity: role of light-harvesting complex II protein dephosphorylation in high light. Plant Physiol. 168, 721–734. doi: 10.1104/pp.15.00488
Melis, A. (1999). Photosystem II damage and repair cycle in chloroplasts: what modulates the rate of photodamage in vivo? Trends Plant Sci. 4, 130–135.
Müller, P., Li, X.-P., and Niyogi, K. K. (2001). Non-photochemical quenching. A response to excess light energy. Plant Physiol. 125, 1558–1566. doi: 10.1104/pp.125.4.1558
Murata, N., Allakhverdiev, S. I., and Nishiyama, Y. (2012). The mechanism of photoinhibition in vivo: re-evaluation of the roles of catalase, α-tocopherol, non-photochemical quenching, and electron transport. Biochim. Biophys. Acta 1817, 1127–1133. doi: 10.1016/j.bbabio.2012.02.020
Murata, N., Takahashi, S., Nishiyama, S., and Allakhverdiev, S. I. (2007). Photoinhibition of photosystem II under environmental stress. Biochim. Biophys. Acta 1767, 414–421. doi: 10.1016/j.bbabio.2006.11.019
Murchie, E. H., and Lawson, T. (2013). Chlorophyll fluorescence analysis: a guide to good practice and understanding some new applications. J. Exp. Bot. 64, 3983–3998. doi: 10.1093/jxb/ert208
Naidu, S. L., and Long, S. P. (2004). Potential mechanisms of low-temperature tolerance of C4 photosynthesis in Miscanthus×giganteus: an in vivo analysis. Planta 220, 145–155. doi: 10.1007/s00425-004-1322-6
Nieva, F. J. J., Castellanos, E. M., Figueroa, M. E., and Gil, F. (1999). Gas exchange and chlorophyll fluorescence of C3 and C4 saltmarsh species. Photosynthetica 36, 397–406. doi: 10.1023/A:1007024019133
Nilkens, M., Kress, E., Lambrev, P., Miloslavina, Y., Muller, M., Holzwarth, A. R., et al. (2010). Identification of a slowly inducible zeaxanthin-dependent component of non-photochemical quenching of chlorophyll fluorescence generated under steady-state conditions in Arabidopsis. Biochim. Biophys. Acta 1797, 466–475. doi: 10.1016/j.bbabio.2010.01.001
Nishiyama, Y., Allakhverdiev, S. I., and Murata, N. (2006). A new paradigm for the action of reactive oxygen species in the photoinhibition of photosystem II. Biochim. Biophys. Acta 1757, 742–749. doi: 10.1016/j.bbabio.2006.05.013
Nishiyama, Y., and Murata, N. (2014). Revised scheme for the mechanism of photoinhibition and its application to enhance the abiotic stress tolerance of the photosynthetic machinery. Appl. Microbiol. Biotechnol. 98, 8777–8796. doi: 10.1007/s00253-014-6020-0
Nishiyama, Y., Yamamoto, H., Allakhverdiev, S. I., Inaba, M., Yokota, A., and Murata, N. (2001). Oxidative stress inhibits the repair of photodamage to the photosynthetic machinery. EMBO J. 20, 5587–5594. doi: 10.1093/emboj/20.20.5587
Niyogi, K. K. (2000). Safety valves for photosynthesis. Curr. Opin. Plant Biol. 3, 455–460. doi: 10.1016/S1369-5266(00)00113-8
Noctor, G., Ruban, A. V., and Horton, P. (1993). Modulation of ΔpH-dependent nonphotochemical quenching of chlorophyll fluorescence in spinach chloroplasts. Biochim. Biophys. Acta 1183, 339–344. doi: 10.1016/0005-2728(93)90237-A
Ohnishi, N., Allakhverdiev, S. I., Takahashi, S., Higashi, S., Watanabe, M., Nishiyama, Y., et al. (2005). Two-step mechanism of photodamage to photosystem ii: step 1 occurs at the oxygen-evolving complex and step 2 occurs at the photochemical reaction center. Biochem 44, 8494–8499. doi: 10.1021/bi047518q
Öquist, G., Chow, W. S., and Anderson, J. M. (1992). Photoinhibition of photosynthesis represents a mechanism for the long-term regulation of photosystem II. Planta 186, 450–460. doi: 10.1007/BF00195327
Park, Y., Chow, W. S., Osmond, C. B., and Anderson, J. M. (1996). Electron transport to oxygen mitigates against the photoinactivation of Photosystem II in vivo. Photosynth. Res. 50, 23–32. doi: 10.1007/BF00018218
Paruelo, J. M., and Lauenroth, K. W. (1996). Relative abundance of plant functional types in grasslands and shrublands of North America. Ecol. Appl. 6, 1212–1224. doi: 10.2307/2269602
Pfündel, E. (1998). Estimating the contribution of photosystem I to total leaf chlorophyll fluorescence. Photosynth. Res. 56, 185–195. doi: 10.1023/A:1006032804606
Pfundel, E., and Neubohn, B. (1999). Assessing photosystem I and II distribution in leaves from C4 plants using confocal laser scanning microscopy. Plant Cell Environ. 22, 1569–1577. doi: 10.1046/j.1365-3040.1999.00521.x
Pietrini, F., and Massacci, A. (1998). Leaf anthocyanin content changes in Zea mays L. grown at low temperature: significance for the relationship between the quantum yield of PS II and the apparent quantum yield of CO2 assimilation. Photosynth. Res. 58, 213–219. doi: 10.1023/A:1006152610137
Pokorska, B., and Romanowska, E. (2007). Photoinhibition and D1 protein degradation in mesophyll and agranal bundle sheath thylakoids of maize. Funct. Plant Biol. 34, 844–852. doi: 10.1071/FP07067
Pokorska, B., Zienkiewicz, M., Powikrowska, M., Drozak, A., and Romanowska, E. (2009). Differential turnover of the photosystem II reaction centre D1 protein in mesophyll and bundle sheath chloroplasts of maize. Biochim. Biophys. Acta 1787, 1161–1169. doi: 10.1016/j.bbabio.2009.05.002
Powles, S. B. (1984). Photoinhibition of photosynthesis induced by visible light. Ann. Rev. Plant Physiol. 35, 15–44. doi: 10.1146/annurev.pp.35.060184.000311
Quick, W. P., and Stitt, M. (1989). An examination of factors contributing to non-photochemical quenching of chlorophyll fluorescence in barley leaves. Biochim. Biophys. Acta 977, 287–296. doi: 10.1016/S0005-2728(89)80082-9
Rao, X., and Dixon, R. A. (2016). The differences between NAD-ME and NADP-ME subtypes of C4 photosynthesis: more than decarboxylating enzymes. Front. Plant Sci. 7:1525. doi: 10.3389/fpls.2016.01525
Ripley, B. S., Gilbert, M. E., Ibrahim, D. G., and Osborne, C. P. (2007). Drought constraints on C4 photosynthesis: stomatal and metabolic limitations in C3 and C4 subspecies of Alloteropsis semialata. J. Exp. Bot. 58, 1351–1363. doi: 10.1093/jxb/erl302
Romanowska, E., Buczyńska, A., Wasilewska, W., Krupnik, T., Drożak, A., Rogowski, P., et al. (2017). Differences in photosynthetic responses of NADP-ME type C4 species to high light. Planta 245, 641–657. doi: 10.1007/s00425-016-2632-1
Romanowska, E., Kargul, J., Powikrowska, M., Finazzi, G., Nield, J., Drozak, A., et al. (2008). Structural organization of photosynthetic apparatus in agranal chloroplasts of maize. J. Biol. Chem. 283, 26037–26046. doi: 10.1074/jbc.M803711200
Ruan, Y., Li, X., Wang, Y., Jiang, S., Song, B., Guo, Z., et al. (2017). Photoinhibition of leaves with different photosynthetic carbon assimilation characteristics in maize (Zea mays). Am. J. Plant Sci. 8, 328–339. doi: 10.4236/ajps.2017.83023
Ruban, A. V. (2016). Nonphotochemical chlorophyll fluorescence quenching: mechanism and effectiveness in protecting plants from photodamage. Plant Physiol. 170, 1903–1916. doi: 10.1104/pp.15.01935
Ruban, A. V., and Belgio, E. (2014). The relationship between maximum tolerated light intensity and photoprotective energy dissipation in the photosynthetic antenna: chloroplast gains and losses. Philos. Trans. R. Soc. B 369:20130222. doi: 10.1098/rstb.2013.0222
Ruban, A. V., Johnson, M. P., and Duffy, C. D. P. (2012). The photoprotective molecular switch in the photosystem II antenna. Biochim. Biophys. Acta 1817, 167–181. doi: 10.1016/j.bbabio.2011.04.007
Ruban, A. V., and Murchie, E. H. (2012). Assessing the photoprotective effectiveness of non-photochemical chlorophyll fluorescence quenching: a new approach. Biochim. Biophys. Acta 1817, 977–982. doi: 10.1016/j.bbabio.2012.03.026
Sage, R. F., and McKown, A. D. (2006). Is C4 photosynthesis less phenotypically plastic than C3photosynthesis? J. Exp. Bot. 57, 303–317. doi: 10.1093/jxb/erj040
Sage, R. F., and Pearcy, R. W. (2000). “The physiological ecology of C4 photosynthesis,” in Photosynthesis: Physiology and Metabolism, eds R. C. Leegood, T. D. Sharkey, and S. von Caemmerer (Dordrecht: Kluwer Academic), 497–532.
Sage, R. F., and Sultmanis, S. (2016). Why are there no C4 forests? J. Plant Physiol. 203, 55–68. doi: 10.1016/j.jplph.2016.06.009
Schöttler, M. A., and Tóth, S. Z. (2014). Photosynthetic complex stoichiometry dynamics in higher plants: environmental acclimation and photosynthetic flux control. Front. Plant Sci. 5:188. doi: 10.3389/fpls.2014.00188
Schreiber, U., Hormann, H., Neubauer, C., and Klughammer, C. (1995). Assessment of photosystem II photochemical quantum yield by chlorophyll fluorescence quenching analysis. Funct. Plant Biol. 22, 209–220. doi: 10.1016/j.bbabio.2010.01.016
Sonoike, K. (2011). Photoinhibition of photosystem I. Physiol. Plant 142, 56–64. doi: 10.1111/j.1399-3054.2010.01437.x
Stirbet, A., Lazár, D., Kromdijk, J., and Govindjee, G. (2018). Chlorophyll a fluorescence induction: can just a one-second measurement be used to quantify abiotic stress responses? Photosynthetica 56, 86–104. doi: 10.1007/s11099-018-0770-3
Suorsa, M., Järvi, S., Grieco, M., Nurmi, M., Pietrzykowska, M., Rantala, M., et al. (2012). PROTON GRADIENT REGULATION5 is essential for proper acclimation of Arabidopsis photosystem I to naturally and artificially fluctuating light conditions. Plant Cell 24, 2934–2948. doi: 10.1105/tpc.112.097162
Takabayashi, A., Kishine, M., Asada, K., Endo, T., and Sato, F. (2005). Differential use of two cyclic electron flows around photosystem I for driving CO2-concentration mechanism in C4 photosynthesis. Proc. Natl. Acad. Sci. U.S.A. 102, 16898–16903. doi: 10.1073/pnas.0507095102
Takahashi, S., and Murata, N. (2008). How do environmental stresses accelerate photoinhibition? Trends Plant Sci. 13, 178–182. doi: 10.1016/j.tplants.2008.01.005
Tazoe, Y., Hanba, Y. T., Furumoto, T., Noguchi, K., and Terashima, I. (2008). Relationships between quantum yield for CO2 assimilation, activity of key enzymes and CO2 leakiness in Amaranthus cruentus, a C4 dicot, grown in high or low light. Plant Cell Physiol. 49, 19–29. doi: 10.1093/pcp/pcm160
Teicher, H. B., Møller, B. L., and Scheller, H. V. (2000). Photoinhibition of photosystem I in field-grown barley (Hordeum vulgare L.): induction, recovery and acclimation. Photosynth. Res. 64, 53–61. doi: 10.1023/A:1026524302191
Tieszen, L. L., Reed, B. C., Bliss, N. B., Wylie, B. K., and DeJong, D. D. (1997). NDVI, C3 and C4 production, and distributions in great plains grassland land cover classes. Ecol. Appl. 7, 59–78.
Tikkanen, M., and Aro, M. (2012). Thylakoid protein phosphorylation in dynamic regulation of photosystem II in higher plants. Biochim. Biophys. Acta 1817, 232–238. doi: 10.1016/j.bbabio.2011.05.005
Tikkanen, M., and Grebe, S. (2018). Switching off photoprotection of photosystem I – a novel tool for gradual PSI photoinhibition. Physiol. Plant 162, 156–161. doi: 10.1111/ppl.12618
Tikkanen, M., Mekala, N. R., and Aro, E. M. (2014). Photosystem II photoinhibition-repair cycle protects photosystem I from irreversible damage. Biochim. Biophys. Acta 1837, 210–215. doi: 10.1016/j.bbabio.2013.10.001
Tiwari, A., Mamedov, F., Grieco, M., Suorsa, M., Jajoo, A., Styring, S., et al. (2016). Photodamage of iron-sulphur clusters in photosystem I induces non-photochemical energy dissipation. Nat. Plants 2:16035. doi: 10.1038/nplants.2016.35
Uzilday, B., Turkan, I., Sekmen, A. H., Ozgur, R., and Karakaya, H. C. (2012). Comparison of ROS formation and antioxidant enzymes in Cleome gynandra (C4) and Cleome spinosa (C3) under drought stress. Plant Sci. 182, 59–70. doi: 10.1016/j.plantsci.2011.03.015
van Kooten, O., and Snel, J. F. H. (1990). The use of chlorophyll fluorescence nomenclature in plant stress physiology. Photosynth. Res. 25, 147–150. doi: 10.1007/BF00033156
Wand, S. J. E., Midgley, G. F., and Stock, W. D. (2001). Growth responses to elevated CO2 in NADP-ME, NAD-ME and PCK C4 grasses and a C3 grass from South Africa. Aust. J. Plant Physiol. 28, 13–25. doi: 10.1071/PP99104
Ware, M. A., Belgio, E., and Ruban, A. V. (2015). Photoprotective capacity of non-photochemical quenching in plants acclimated to different light intensities. Photosynth. Res. 126, 261–274. doi: 10.1007/s11120-015-0102-4
Zhang, S., and Scheller, H. V. (2004). Photoinhibition of photosystem I at chilling temperature and subsequent recovery in Arabidopsis thaliana. Plant Cell Physiol. 45, 1595–1602. doi: 10.1093/pcp/pch180
Zienkiewicz, M., Drożak, A., Wasilewska, V., Bacławska, I., Przedpełska-Wąsowicz, E., and Romanowska, E. (2015). The short-term response of Arabidopsis thaliana (C3) and Zea mays (C4) chloroplasts to red and far red light. Planta 242, 1479–1493. doi: 10.1007/s00425-015-2392-3
Keywords: environmental stress, photochemistry, photoinhibition, photosynthesis, photosystem II efficiency
Citation: Guidi L, Lo Piccolo E and Landi M (2019) Chlorophyll Fluorescence, Photoinhibition and Abiotic Stress: Does it Make Any Difference the Fact to be a C3 or C4 Species? Front. Plant Sci. 10:174. doi: 10.3389/fpls.2019.00174
Received: 23 November 2018; Accepted: 01 February 2019;
Published: 14 February 2019.
Edited by:
Marian Brestic, Slovak University of Agriculture, SlovakiaReviewed by:
Ilektra Sperdouli, Institute of Plant Breeding and Genetic Resources, Hellenic Agricultural Organisation (HAO), GreeceRaquel Esteban, University of the Basque Country, Spain
Lorenzo Ferroni, University of Ferrara, Italy
Copyright © 2019 Guidi, Lo Piccolo and Landi. This is an open-access article distributed under the terms of the Creative Commons Attribution License (CC BY). The use, distribution or reproduction in other forums is permitted, provided the original author(s) and the copyright owner(s) are credited and that the original publication in this journal is cited, in accordance with accepted academic practice. No use, distribution or reproduction is permitted which does not comply with these terms.
*Correspondence: Lucia Guidi, bHVjaWEuZ3VpZGlAdW5pcGkuaXQ=