- Department of Plant Agriculture, University of Guelph, Guelph, ON, Canada
During vegetative growth maize can accumulate luxury nitrogen (N) in excess of what is required for biomass accumulation. When post-silking N uptake is restricted, this luxury N may mitigate N stress by acting as an N reserve that buffers grain yield and maintains plant function. The objective of this study was to determine if and how luxury accumulation of N prior to silking can buffer yield against post-silking N and/or water stress in maize. In a greenhouse experiment, maize was grown in high (Nveg) and low (nveg) N conditions during vegetative growth. The nveg treatment did not affect biomass accumulation or leaf area by silking but did accumulate less total N compared to the Nveg treatment. The Nveg treatment generated a reserve of 1.1 g N plant-1. Plants in both treatments were then subjected to water and/or N stress after silking. 15N isotope tracers were delivered during either vegetative or reproductive growth to measure N remobilization and the partitioning of post-silking N uptake with and without a luxury N reserve. Under post-silking N and/or water stress, yield was consistently greater in Nveg compared to nveg due to a reduction in kernel abortion. The Nveg treatment resulted in greater kernel numbers and increased N remobilization to meet grain N demand under post-silking N stress. Luxury N uptake at silking also improved leaf area longevity in Nveg plants compared to nveg under post-silking N stress, leading to greater biomass production. While post-silking N uptake was similar across Nveg and nveg, Nveg plants partitioned a greater proportion of post-silking N to vegetative organs, which may have assisted with the maintenance of leaf function and root N uptake capacity. These results indicate that N uptake at silking in excess of vegetative growth requirements can minimize the effect of N and/or water stress during grain-fill.
Introduction
In maize (Zea mays L.) as in other globally important cereals, nitrogen (N) taken up during vegetative growth before silking is later remobilized to the ear to support kernel formation. Roughly 45–65% of maize grain N at maturity is derived from N remobilization, while N taken up during reproductive growth supplies the rest (Gallais et al., 2007; Ciampitti and Vyn, 2012; Ning et al., 2017). But under abiotic stresses the typical patterns of N remobilization and partitioning are disrupted (Ciampitti and Vyn, 2013; de Oliveira Silva et al., 2017). Notably, when whole-season or post-silking N availability is low, grain N will rely increasingly on remobilized N (Friedrich and Schrader, 1979; Ta and Weiland, 1992), possibly acting as a compensatory mechanism to support kernel N demand when post-silking N uptake is low (Ciampitti and Vyn, 2012; Mueller et al., 2017). This phenomenon is also observed in wheat (Palta et al., 1994). However, an increase in N remobilization under post-silking N stress can lower yield by reducing leaf and root function, thereby decreasing assimilate production and nutrient capture earlier than normal (Triboi and Triboi-Blondel, 2002). While maize can utilize previously acquired N for grain development, it relies heavily on concurrent assimilate supply to meet grain assimilate demand. This leads to the “yield dilemma” of N remobilization (Masclaux-Daubresse et al., 2010), whereby high rates of N remobilization are tied to a decrease in yield due to leaf senescence reducing assimilate production during grain-fill. As N remobilization increases in maize, post-silking N uptake is normally reduced as well (Gallais and Coque, 2005; Ciampitti and Vyn, 2013). Presumably this is because N remobilization also reduces assimilate supply to roots (Muchow, 1988; Ta and Weiland, 1992; Pan et al., 1995; Liedgens et al., 2000), but also perhaps because N remobilization reduces the demand for post-silking N (Mueller and Vyn, 2018).
In well-fertilized farm fields, the risk of post-silking N stress is largely due to excessive rainfall events that lead to large field-scale N losses via leaching or denitrification (Eagle et al., 2017), or water deficits, which can limit N availability (Gonzalez-Dugo et al., 2010). Under droughty conditions maize roots can access water in deeper soil layers, but N is concentrated in the topsoil and arrives to the root surface via mass flow or diffusion, assisted by transpiration (Kim et al., 2008; McMurtrie and Näsholm, 2018). Water stress reduced biomass accumulation rates and yield, particularly when it is experienced around silking (Cakir, 2004), and because water stress reduces maize growth, it also lowers the N uptake needs of the plant (Gonzalez-Dugo et al., 2010). However, the reduction in maize N uptake that is commonly observed under water limitation (e.g., Teixeira et al., 2014) is not solely attributable to a reduction in maize growth, but also to a direct reduction in the accessibility of soil N for crop uptake (Lemaire et al., 1996; Buljovcic and Engels, 2001).
Plénet and Lemaire (2000), as well as Ciampitti and Vyn (2012), hypothesized that the accumulation of luxury N – N taken up during vegetative growth that is not used for biomass production but instead incorporated into storage pools – may be able to buffer against N stresses experienced during grain-fill. In the former study, irrigated maize was found to benefit from luxury pre-silking N uptake beyond that required for maximum biomass accumulation, concluding that a luxury N pool of ∼30 kg N ha-1 by silking may be beneficial (Plénet and Lemaire, 2000). The authors concluded that this reserve of vegetative N buffers grain yield since soil N availability during grain-fill is normally insufficient to support N demand (Plénet and Lemaire, 2000). But the possible mechanism(s) underlying this response to luxury N uptake, such as the quantification of grain N sources or changes in canopy longevity, were not explored. In the latter study, a synthesis-analysis, the authors observed that grain yield was strongly correlated to N uptake at silking (Ciampitti and Vyn, 2012). Based on this relationship the authors suggested that, as a pathway to yield improvement, maize breeding efforts should focus on increasing pre-silking N uptake and forming an N reserve. However, no assessment was made as to the importance of a pre-silking N reserve under post-silking N or water stress (Ciampitti and Vyn, 2012). Both studies reason that, by minimizing dependence on post-silking N uptake, a reserve of luxury N accumulated by silking can allow the plant to meet grain N demand when post-silking N uptake is low.
Several researchers have used 15N isotope tracing to quantify maize response to post-silking N stress. In a greenhouse experiment, Friedrich and Schrader (1979) labelled maize with 15N during vegetative growth and then imposed N stress after silking. N remobilization from leaves, stems and roots was enhanced under post-silking N stress relative to control. In a field study using 15N multi-stage pulse labelling, de Oliveira Silva et al. (2017) quantified differences in post-silking N allocation to plant organs at discreet time periods during grain-fill. When no N was applied, a much greater proportion of post-silking N was allocated to developing ears, at the expense of leaves, relative to a moderately fertilized control. In a greenhouse experiment, Paponov and Engels (2005) applied 15N at the start of grain-fill to measure the partitioning of post-silking N under high and low N supply. A greater proportion of post-silking N uptake was allocated to the grain under low N and they noted that under N stress, N remobilization from leaves was enhanced relative to high N conditions (Paponov and Engels, 2005). Together these results demonstrate that under post-silking N stress, maize N metabolism changes to ensure that ear or kernel N supply is conserved at the expense of the N status of vegetative organs. As such they are in concordance with the idea that a reserve of luxury pre-silking N can buffer yield under post-silking N stress by allowing greater N remobilization to the grain without impinging on leaf or root function.
The objectives of this study were to determine: (i) if maize has the capacity to accumulate N in excess of that required for biomass production (luxury N uptake), (ii) quantify the contribution of pre-silking N remobilization and post-silking N uptake as sources of grain N, and (iii) measure biomass accumulation, leaf area, leaf carbon exchange rates (CER), grain yield and yield components to determine the potential value of luxury N to buffer against post-silking N and water stress.
Materials and Methods
Greenhouse Experiment Setup and Design
The experiment was performed in the Crop Science Greenhouse at the University of Guelph, ON, Canada. Seeds of the maize hybrid DKC39-97 (Dekalb (Monsanto); Winnipeg, MB, Canada) were sown individually in 19-L plastic pots, filled with Turface MVP® (Profile Products LLC; Buffalo Grove, IL, United States), an inert calcined clay widely used as growing medium for maize (Goron et al., 2015). All pots were free-draining due to holes on the bottom, with a mesh screen ensuring roots did not exit the pots. A constant temperature regime of 27°C day/21°C night was used throughout the experiment, while high pressure sodium and metal halide lamps provided supplemental lighting on a 16-h photoperiod starting at dawn.
A nested factor design was used in the experiment with two N supply treatments (high and low N), imposed during vegetative growth under well-watered conditions. Five days after R1 (silking) (Abendroth et al., 2011) plants were placed into one of four treatments consisting of two N supply levels (high and low N) and two levels of water supply (well-watered and water stress) applied in combination. The experiment was setup as a completely randomized block design, with six blocks (i.e., replicates) each containing all eight treatments. Treatments were applied to individual plants, and plant pots were rotated within the blocks every 7 to 10 days to avoid border effects.15N isotope tracers were delivered to plants in four of the six blocks, with plants labelled once during either vegetative or reproductive growth. The other two blocks were treated identically except no 15N tracers were added. Blocks with 15N contained 24 plants while blocks not receiving 15N contained 16 plants. Total time from emergence to maturity was 90 days. The experiment was conducted in two separate greenhouses, each greenhouse containing three blocks, two blocks with 15N labelling and one block without 15N labelling.
Nitrogen and Water Supply Treatments
Final concentration of N was 20 mM in the high N solution and 5 mM in the low N solution. The high N and low N treatments were based on pre-experiments done in preparation for the present study. The low N treatment (5 mM) was selected because it could achieve similar biomass and leaf area at silking compared to 20 mM, but 20 mM generated much greater N uptake. Pre-experiments found that the N limitation at 5 mM was relatively mild, but sufficient to reduce grain yield relative to 20 mM. While several researchers have imposed N stress by withholding fertilizer N completely (e.g., Subedi and Ma, 2005), the growing medium those experiments used contained a proportion of field soil that could provide N for plant uptake via mineralization. Turface does not provide any N for plant uptake (Goron et al., 2015).
Each fertilizer treatment was delivered on a separate, parallel, solenoid system with automatic fertilizer injectors (Superdos; Dosmatic U.S.A., Inc.) and fertilizer solution was delivered to the pot surface with a spray stick (Acu-Spray Stick; Jain Irrigation). The nutrient mixture, based on the recipe of Echarte et al. (2008), was made by dissolving into 80 L of distilled water: 0.329 L H3PO4 (750 g kg-1), 0.600 kg KHCO3, 0.320 kg Ca(NO3)2, 0.64 kg MgSO4, and 0.048 kg of chelated micronutrient mix (Plant Products Co., Brampton, ON, Canada). In the high N nutrient solution 1.125 kg NH4–NO3 was added, and in the low N nutrient solution, 0.165 kg NH4–NO3. The mixture was diluted (20 times) during irrigation with distilled water. In addition to the nutrient mixture, distilled water irrigation was provided individually to each pot using an irrigation system that was parallel to the fertigation system described above.
Volumetric water content was monitored via 30 soil moisture sensors (EC-5; Decagon Devices, Pullman, WA, United States), with a minimum of 1 sensor in each treatment per block. All sensors were fitted with a custom calibration for use in Turface and outputs were coupled with a moisture characteristic curve for Turface calculated with the pressure plate technique (Premalal, 1997). Well-watered plants were irrigated to keep volumetric soil moisture between 35 and 40% (targeting –35 millibar), corresponding to a plant available water content between 80 and 90% of maximum. Pots in the water stress treatment imposed after silking were irrigated to keep volumetric soil moisture between 25 and 30% (targeting –500 millibar), equivalent to 57–68% of maximum plant available water content. These levels of water supply were selected based on a previous experiment that measured maize response to water supply in Turface. The level of water stress was chosen to be severe enough to reduce yield relative to well-watered plants without provoking terminal drought. Throughout the experiment, fertigation and irrigation water combined never exceeded the water holding capacity of the pots except at silking when pots were being transitioned from vegetative to reproductive treatments.
Treatment Imposition and 15N Labelling During Vegetative or Reproductive Growth
Nineteen days after plant emergence, when four fully emerged collared leaves were present (V4 growth stage; Abendroth et al., 2011) both high N (Nveg) and low N (nveg) were imposed under well-watered conditions. Each plant was monitored for the first appearance of silks. Five days after silk appearance the remaining plants were irrigated with distilled water to remove residual N in the growing medium and placed into one of the four post-silking treatments that lasted until maturity. These treatments were: (1) high N, well-watered (NWrep), (2) low N, well-watered (nWrep), (3) high N, water stress (Nwrep), or (4) low N, water stress (nwrep).
In the four blocks receiving 15N, plants were labelled once, either during vegetative growth or after silking during reproductive growth. During vegetative growth, a total application of 40 mg of 15N was applied to label plants in both Nveg and nveg. Four times over a labelling period of 15 days, 10 mg of 15N was delivered in a syringe by dissolving 680 mg of K15NO3 at 10% 15N abundance (purchased as 10% K15NO3 from Sigma-Aldrich; St. Louis, MO, United States) in 100 mL of water. The daily irrigation regime ensured that the applied N infiltrated into the root zone. At each 15N application date, all plants not receiving 15N during vegetative growth were given the same amount of unlabelled KNO3 in 100 mL of water to ensure equivalent amounts of water and KNO3 were provided to all plants. Plants labelled during reproductive growth received 90 mg of 15N. Nine times over a period of 24 days, beginning 8 days after silking, 10 mg of 15N was delivered to the base of each plant in the same process used during vegetative labelling. Plants that were not being labelled during reproductive growth were provided with equivalent amounts of water and KNO3 at each 15N application date to avoid confounding effects. In all eight treatment combinations, when plants were harvested at maturity, one plant was labelled during vegetative growth and one plant was labelled during reproductive growth per block.
Irrigating Pots at Silking to Remove 15N Applied During Vegetative Growth to Improve Estimates of N Remobilization to the Grain
To improve estimates of pre-silking N remobilization to the grain, 15N was removed from each pot at silking by irrigating pots for 30 min at a flow rate of 18 L min-1 and allowing excess water to continually drain from the bottom holes of the pot. Two studies were conducted to test the efficacy of this procedure in leaching residual 15N and preventing post-silking 15N uptake. To test the leachability of Turface MVP®, 2.25 g of N (in the form of dissolved KNO3) was added to 19-L pots filled with Turface MVP® without exceeding the water holding capacity of the pots. After resting for 1 day, pots were irrigated for 30 min or not at all (n = 4) and residual N in the Turface growing medium was measured via colorimetric analysis analogous to soil sampling for N content. Compared to the non-irrigated pots, Turface N content of the irrigated pots was 99% lower, indicating that most of the 2.25 g N added to each pot was leached away following the 30 min irrigation (Supplementary Table S1). As a second experiment, 40 mg of 15N was delivered via syringe to four 19-L pots filled with Turface MVP® by dissolving 2.25 g of K15NO3- at 10% 15N abundance in 400 mL of water. After 3 days, pots were irrigated for 30 min each (flow rate = 18 L min-1). Each pot was immediately sown with a hybrid maize seed DKC39-97 (Dekalb (Monsanto); Winnipeg, MB, Canada) and grown until V13 (Abendroth et al., 2011) in non-stress (high N and well-watered) conditions. At V13 the whole plant was harvested, ground and the Delta-15N (δ15N ‰) was determined to see if enrichment of plant tissue above natural abundance occurred. The δ15N ‰ is a measure of the level of 15N enrichment or depletion in a sample relative to natural abundance, with δ15N equal to zero at the international isotope standard of atmospheric N2 (0.3663% 15N) (Mariotti et al., 1981). The δ15N (‰) of these plants varied from –1.59 to –3.88 (0.3649 to 0.3657% 15N), indicating that the isotopic ratio was slightly below natural abundance and no excess 15N uptake occurred after the irrigation procedure (Supplementary Table S2).
Measurements
At silking four plants in Nveg and four plants in nveg were harvested in each block. Plant roots were first rinsed in distilled water and plants were dissected into 4 organ groups: leaves, stalk, roots and reproductive organs (cob, silks, tassel and husk). All plant parts were dried at 80°C in an oven for 48 h and weighed. On half of the harvested plants, the organs (leaves, stalk, roots, reproductive organs) were ground into a homogenous powder, and a 10-g subsample was used to determine total N content using dry combustion (Dumas method). Leaf area was measured for each harvested plant by passing leaves through a leaf area meter (LI-3100c, LI-COR, Lincoln, NE, United States). On a subset of leaves, leaf length and width at the widest point were also measured with a ruler prior to the leaf area meter measurement, and then later dried in an oven at 80°C for 48 h and weighed individually. These measurements were used to parameterize two regressions, one regressing leaf length and width (as measured by a ruler) to leaf area (cm2), and another regressing leaf weight to leaf area (cm2) (described in the Supplementary Material). These regressions were used to calculate leaf area during grain-fill. Potential kernel number was measured following Echarte and Tollenaar (2006), with spikelets at the tip of the cob, where kernel rows became non-uniform, not counted.
Leaf area was measured non-destructively at tasseling on all plants, by which time leaf emergence was complete. Length and width (at the widest point) of each individual leaf was measured with a ruler. Results from the destructive harvest at silking enabled ruler-based measurements of leaf length and width to be converted to leaf area. Leaf senescence during reproductive growth was measured one, two and three weeks after silking. Fully senesced leaves or parts of leaves which were senesced, identified visually as lacking green colour, and by touch as being brittle (Wolfe et al., 1988) were cut off from green areas using scissors. Senesced leaves or leaf fragments were dried for 48 h at 80°C and then weighed. Leaf area (cm2) was estimated based on dried leaf weight (g) using a regression parameterized using leaf data collected at silking (described in the Supplementary Material).
Leaf CER was measured with a LI-6400XT (LI-COR, Lincoln, NE, United States) at 2000 μmol m-2 s-1 photosynthetic photon flux density at the leaf surface, maintaining a CO2 concentration of 350 ppm and a leaf temperature of 30°C within the sample chamber. Measurements were taken on three leaves per plant: (i) the second leaf below the ear leaf, (ii) the ear leaf, and (iii) the second leaf above the ear leaf. Leaf CER was measured at mid-day, in a 6 cm2 area near the middle of the leaf that did not include the midrib. Measurements were made three times during the experiment: (i) at the beginning of silking, before the imposition of the reproductive treatments, (ii) 21 days after silking, and (iii) 34 days after silking.
At physiological maturity 80 plants were harvested, 16 from each of the four blocks receiving 15N and 8 from each of the two blocks not receiving 15N. Roots were washed immediately in distilled water, and all plants were dissected and organs placed into five groups: green leaves (or parts of green leaves), senesced leaves (or parts of senesced leaves), stalk, roots, grain, and non-grain reproductive organs (cob, husk and tassel). Plant parts were then dried at 80°C in an oven for 48 h and weighed. Kernel number was determined in a computerized seed counter (ESC-1; Agriculex, Guelph, ON) which allowed for average kernel weight to be calculated. Plant samples were analyzed for N concentration and 15N/14N ratio by dry combustion in an elemental analyzer (CHNS-O 1108; Carlo Erba, Italy) coupled to a continuous-flow stable isotope ratio mass spectrometer (DeltaplusXL; Thermo Finnigan, Germany) at the University of Waterloo Environmental Isotopes Laboratory in Waterloo, Canada.
15N Calculations
Calculations of N uptake, remobilization and partitioning were based on Paponov and Engels (2005), Gallais et al. (2006, 2007) and Ning et al. (2017). They were made assuming: (1) no isotopic discrimination between 14N and 15N within the maize plant, (2) no isotopic discrimination in reactions involved in N metabolism, (3) no N losses from the plant and (4) that 15N was uniformly distributed in the growing medium surrounding the roots (Gallais et al., 2006, 2007). 15N occurs naturally in maize tissue at approximately natural abundance (0.3663% 15N), and 15N accumulating in excess of this amount in a plant organ is assumed to come from 15N fertilizer. Total plant 15N derived from fertilizer could be calculated and the sum of 15N in each plant organ in excess of natural abundance. Because all plants labelled with 15N during vegetative growth were irrigated for 30 min at silking, it was assumed that that no residual 15N applied during vegetative growth was taken up after silking. The equations used in 15N calculations of N remobilization normally use a correction factor to account for this residual post-silking 15N uptake (e.g., Ning et al., 2017), but no correction factors are used here. In this study, the natural abundance of unlabelled maize grown in the greenhouse was determined empirically (n = 5) and a value close to the international standard of atmospheric N2 was used (range: 0.3733 ± 0.0031 %15N).
Calculation of Vegetative N Partitioning and Remobilization at Maturity
For every plant part sampled (e.g., roots, stalk, leaves), the amount of 15N derived from labelled fertilizer (i.e., the amount of 15N in excess over natural abundance), was calculated as:
where, q(x) equals the amount of 15N derived from labelled fertilizer (i.e., 15N% excess for part x), A(x) equals 15N abundance for organ x, AO equals 0.3733% 15N (i.e., natural abundance of 15N in maize for this experiment) and Qx is the total N content of part x.
For plants labelled prior to silking, the amount of 15N excess found in the grain q(grain) was assumed to come from 15N excess originating from pre-silking N uptake of labelled fertilizer that was then remobilized. The percent of pre-silking N remobilized (rem%) is thus equal to:
where, qgrain is the amount of excess 15N in the grain, and q(wpm) is the amount of excess 15N in the whole plant at maturity. Consequently, the amount of pre-silking N remobilized to the grain at maturity (Qrem(grain)) is equal to:
where, Q(wps) is equal to the total N content of the whole plant at silking. The percentage of grain N derived from remobilization (Grainrem) is equal to:
where, Q(grain) is the N content of the grain.
Calculations of Post-silking N Uptake and Partitioning
For plants labelled during reproductive growth the percentage of post-silking N uptake partitioned to the grain (Post%(grain)) is equal to:
Note that Eq. 5 is analogous to Eq. 2, used when plants were labelled during vegetative growth. It follows that the total amount of grain N originating from post-silking N uptake (Qpost(grain)) is equal too:
Note that Eqs 5 and 6 are generalizable to any plant organ. Q(wps) was the average N uptake for either vegetative treatments (Nveg and nveg) (n = 12). Q(wpm) was measured individually for each plant. For plants labelled during reproductive growth, the percent of grain N derived from remobilization (Grainrem):
Statistical Analysis
All statistical analyses were performed in R v.3.5.0 (R Core Development Team; Vienna, Austria) using a mixed effects model (lme function; nlme R package) with vegetative and reproductive treatments as fixed factors; random factors were replicate and greenhouse. The experiment was set up as a nested factor design (Eq. 8), with eight treatments in total (4 reproductive treatments and 2 vegetative treatments), and all statistical analyses were computed with vegetative treatments nested within reproductive treatments via the lsmeans function (lsmeans R package). Proportional data were analyzed using a beta regression (glmmTMB function; R package glmmTMB) and count data using a Poisson regression (glmer function; R package lme4). Green leaf area per plant during grain-fill was analyzed with a repeated measures ANOVA (lmer function; lme4 R package). Multiple means comparisons were performed with a Tukey’s test. For all statistical tests conducted, the type I error rate was set at 0.05. After fitting, all models were assessed for normally distributed residuals and homogenous error variance across factor levels; when residual non-normality or heterogeneity among factor levels was apparent the model covariance structure was adjusted to a heterogenous error model.
where, μ is the overall mean, βi is the fixed effect of the reproductive treatment i, βj(i) is the fixed effect of the vegetative treatment j nested within reproductive treatment i,αk is the random effect of greenhouse k, αl(k) is the random effect of block l within greenhouse k and εijkl is the random error.
Results
Luxury N Uptake Was Observed in the Nveg Treatment Relative to nveg at Silking
At silking total biomass and leaf area were statistically similar across nveg and Nveg, although total N content was greater in Nveg (Table 1). Leaf CER was the same across Nveg and nveg treatments (mean = 24.2 μmol m2 s-1) at all three sampled leaf positions (Supplementary Figure S1). This indicates that luxury N was accumulated during vegetative growth in the Nveg treatment. This luxury N accumulated primarily in the stalk and leaves (Figure 1). There was no difference in silking date (data not shown). Nveg had a greater spikelet count compared to nveg (805 vs. 656 spikelets plant-1) indicating that the increased N uptake in Nveg stimulated greater spikelet formation (Table 1).

Table 1. Plant parameters (mean ± S.E.) at silking across the high N (Nveg) and low N (nveg) treatments.
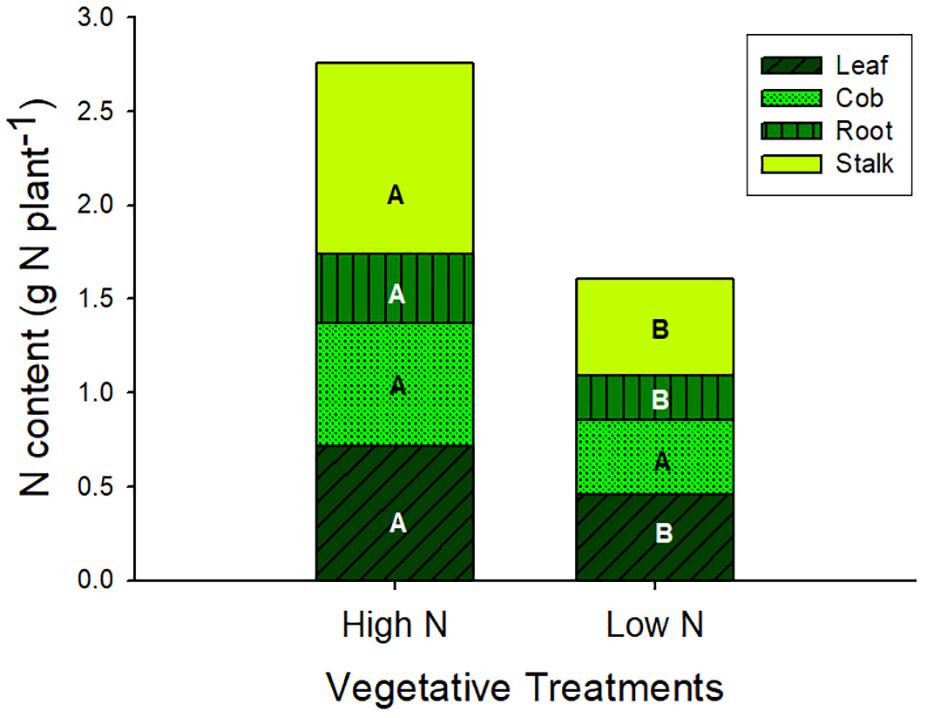
Figure 1. Plant N content at silking partitioned across vegetative organ in the vegetative treatments (mean ± S.E.). Different letters indicate significant differences in organ N content across the high N (Nveg) and low N (nveg) vegetative treatments at p < 0.05 (n = 6).
Grain N Sources and Post-silking N Partitioning in Vegetative and Reproductive Treatments
Based on 15N measurements post-silking water and/or N stress increased the percent of grain N derived from remobilization in both the Nveg and nveg treatment (Table 2). In Nveg, the proportion of grain N derived from remobilized increased from 61.9% under NWrep to an average of 85.9% in the reproductive stress treatments (nWrep, Nwrep and nwrep) (Table 2). Under nveg these values were 45.4 and 65.8% for NWrep and the stress treatments, respectively. Without a reserve of luxury N, the amount of N remobilized to the grain in nveg under post-silking stress was 0.77 g N plant-1, lower than the amount of N remobilized in Nveg (1.57 g N plant-1) but as a proportion of total pre-silking N, slightly greater (62.5% vs. 55.6%) (Supplementary Figure S2) according to 15N calculations. Overall, under post-silking water and/or N stress, a greater amount of pre-silking N was remobilized in Nveg compared to nveg, and this remobilized N comprised a larger proportion of grain N (Table 2).
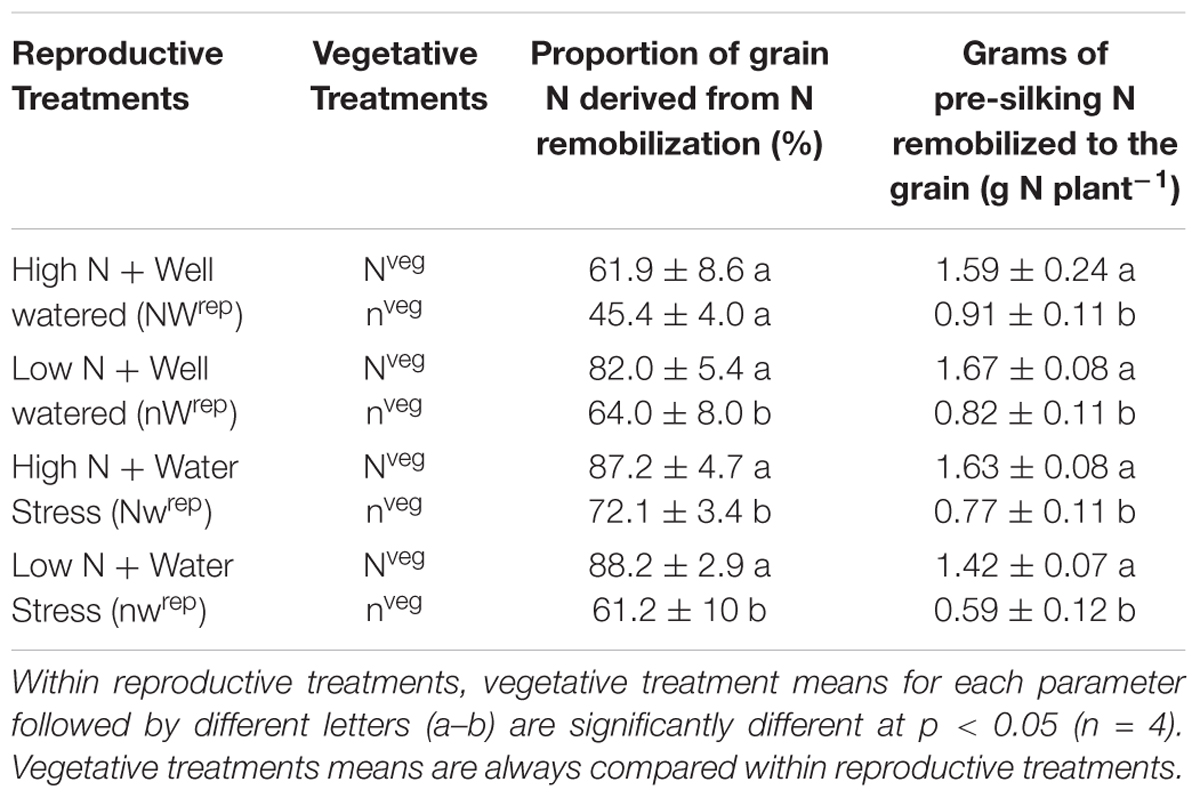
Table 2. Effect of the vegetative treatments (Nveg and nveg) on the proportion of grain N derived from remobilization and amount of pre-silking N remobilized to the grain as measured by the 15N method (mean ± S.E) across all four reproductive treatments (NWrep, nWrep, Nwrep, nwrep) at maturity.
Post-silking N and/or water stress treatments reduced post-silking N uptake relative to NWrep in both vegetative treatments (Table 3). Post-silking N uptake was similar for Nveg and nveg in all four reproductive treatments (Table 3), indicating that differences in N remobilization and pre-silking N uptake did not affect post-silking N accumulation. However, the allocation of post-silking N across plant organs differed between Nveg and nveg according to 15N measurements. Notably, under low N supply (nWrep and nwrep) nveg plants partitioned more post-silking N to the grain relative to Nveg (79.4% vs. 31.8%) (Table 3). Aside from the grain, by maturity the stalk was a major sink for post-silking N in Nveg, accounting for 23.8% of post-silking N uptake (Table 3). Under nveg, the stalk was a much smaller sink for post-silking N except when adequate N and water supply was supplied (NWrep). The roots were also a major sink of post-silking N, especially under water stress. Under water stress (Nwrep and nwrep) plants in Nveg partitioned more post-silking N to the roots relative to nveg (46.3% vs. 26.7%), and overall results indicate that under post-silking water stress the roots are a major sink for post-silking N (Table 3). The cob and leaves (both senesced leaves and green leaves) each accounted for less than 5% of post-silking N in all treatments, except for nveg-NWrep, where post-silking N accumulated in the cob and leaves to a much greater extent (Table 3).
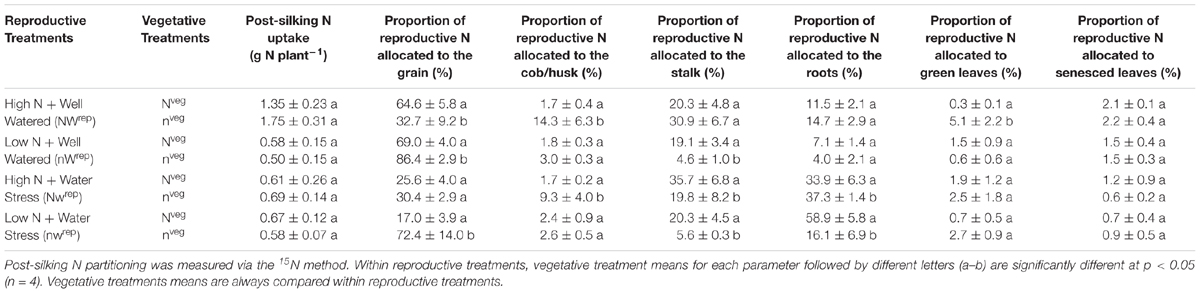
Table 3. Effect of the vegetative treatments (Nveg and nveg) on post-silking N uptake and partitioning of post-silking N uptake (mean ± S.E) across all four reproductive treatments (NWrep, nWrep, Nwrep, nwrep) at maturity.
Yield, Yield Components and Leaf Performance During Grain-Fill
Under post-silking N and water stress, yield was consistently greater in Nveg relative to nveg (Table 4), indicating that the pre-silking luxury N reserve buffered yield against N and/or water stress. However, under adequate post-silking N and water supply (NWrep), the luxury N reserve did not offer any benefit in terms of yield (Table 4). The yield components driving the differences in grain yield between Nveg and nveg under post-silking stress was kernel number, not kernel weight (Table 4).
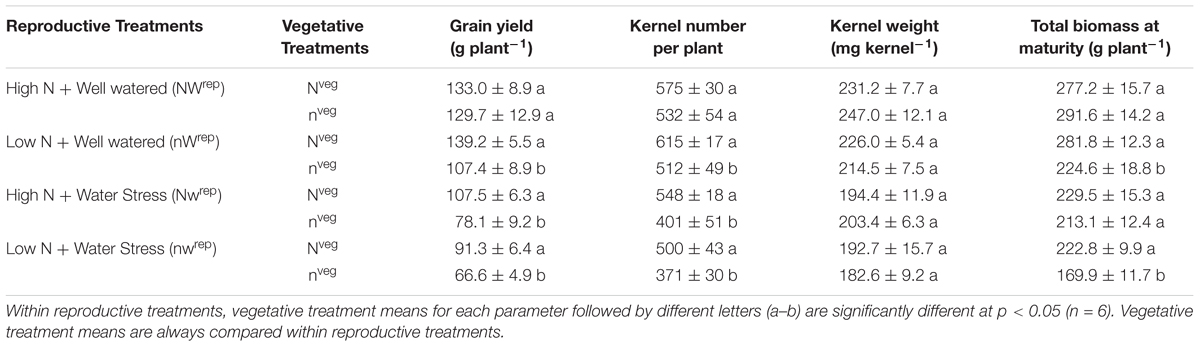
Table 4. Effect of the vegetative treatments (Nveg and nveg) on yield, yield components and total biomass (mean ± S.E.) across all four reproductive treatments (NWrep, nWrep, Nwrep, nwrep) at maturity.
During early and mid grain-fill, from 0 to 23 days after silking (DAS)1, leaf area was maintained longer in Nveg compared to nveg. This difference was statistically significant under post-silking N stress (nWrep and nwrep), indicating that the reserve of luxury pre-silking N could buffer leaf function under N stress, though this effect was not significant under post-silking water stress (Figure 2). Leaf CER was statistically similar across Nveg and nveg at mid grain-fill (21 DAS) and late grain-fill (35 DAS) on all three leaf positions (Figure 3). Thus, despite plants in the Nveg treatment remobilizing more than twice as much pre-silking N during grain-fill (Table 2), neither leaf area nor leaf CER was negatively affected compared to nveg during grain-fill. By maturity total biomass was greater in Nveg when post-silking N stress occurred (Table 4). This suggests that the improvements in post-silking leaf function observed in Nveg under post-silking N stress translated into greater post-silking assimilate production and biomass accumulation.
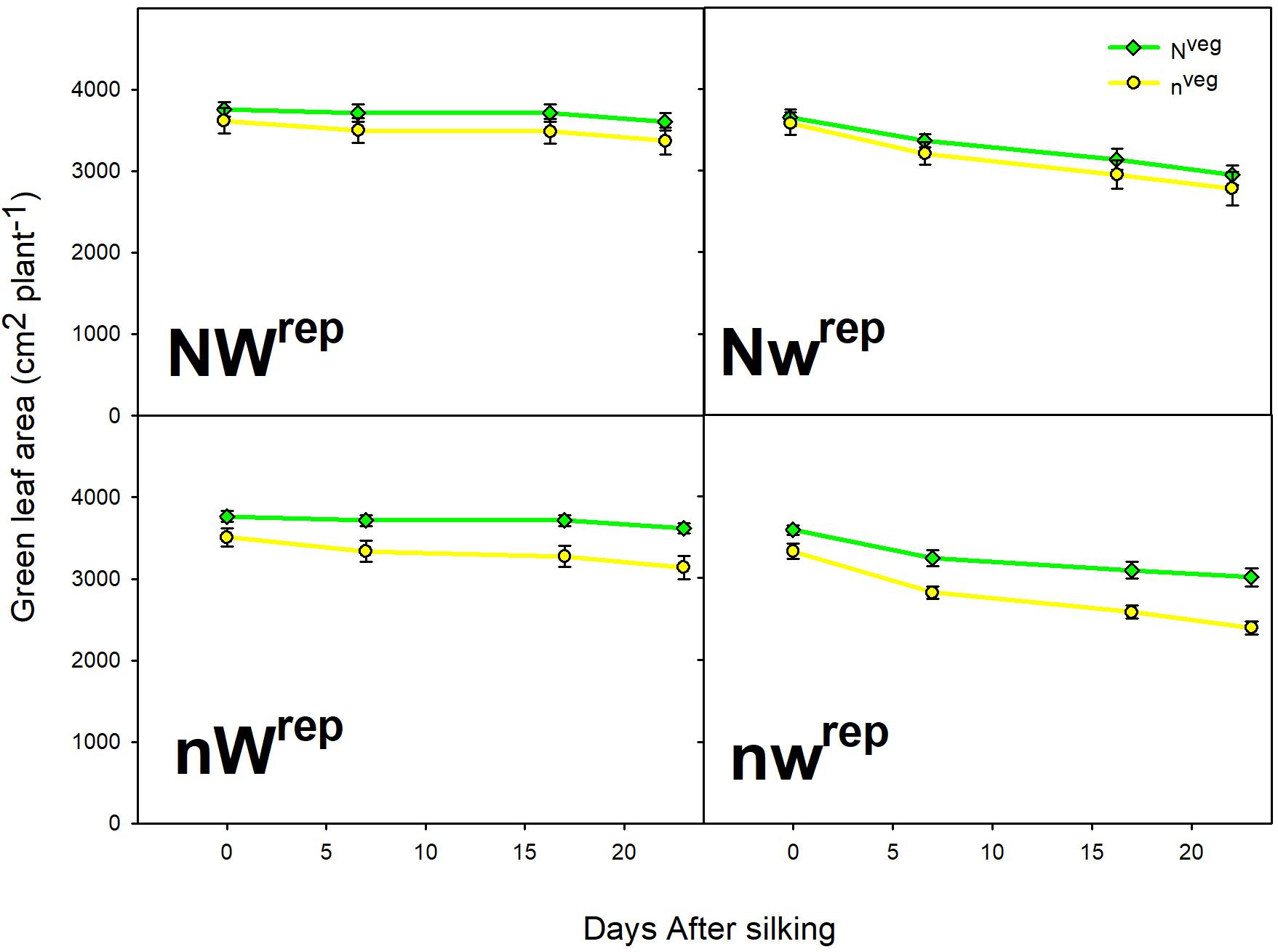
Figure 2. Green leaf area from silking to mid grain-filling in both vegetative treatments within all four reproductive treatments (mean ± S.E.). Repeated measures analysis indicates that the rate of green leaf area decline was significantly lower in Nveg compared to nveg in the nWrep and nwrep treatments at p < 0.05 (n = 6). No significant differences were found in the rate of leaf area decline between Nveg and nveg in the NWrep and Nwrep treatments at p < 0.05 (n = 6).
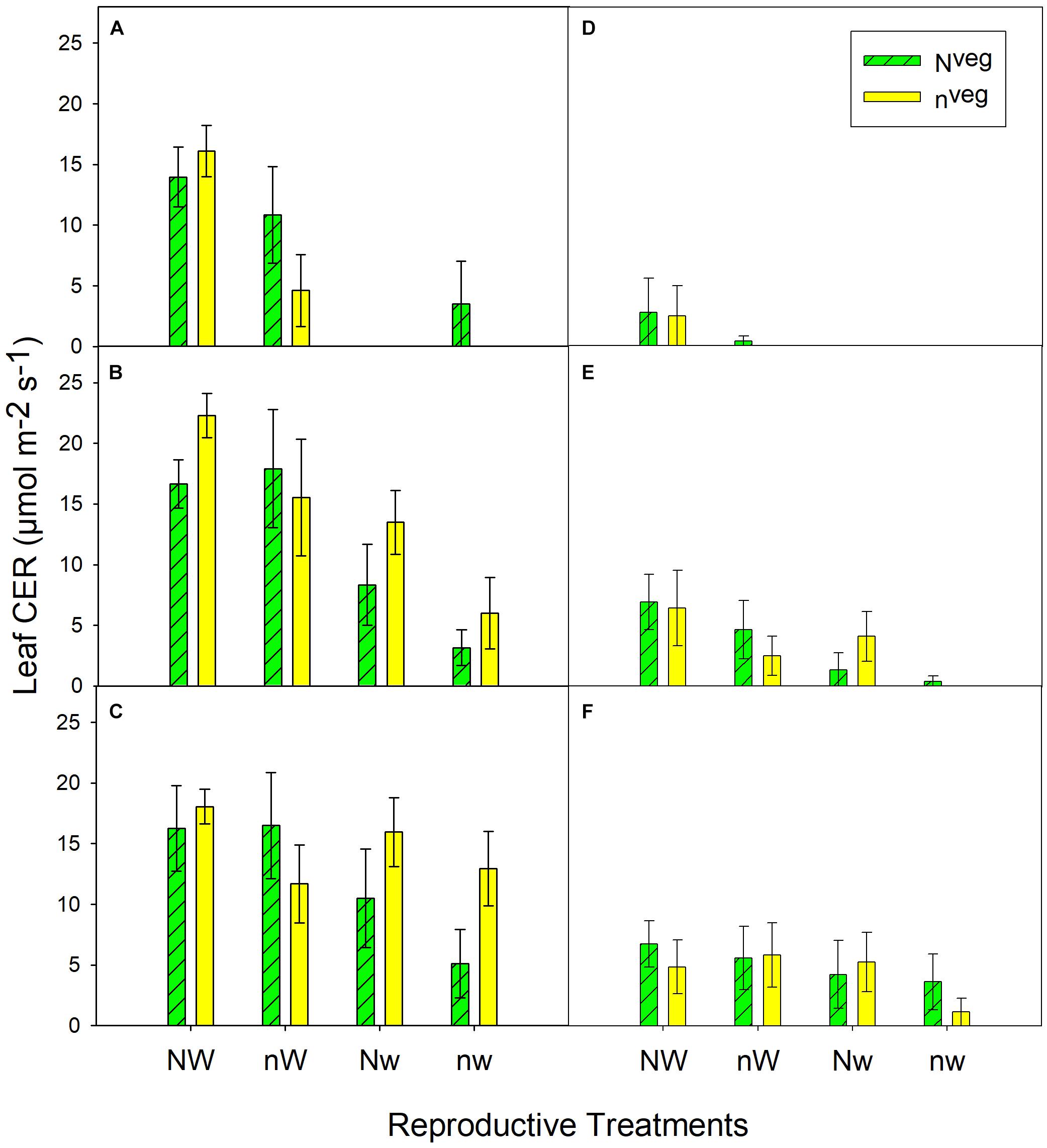
Figure 3. Leaf CER measured at mid grain-fill (21 days after silking; A–C) and late grain-fill (34 days after silking; D–F) on the 2nd leaf below the ear (A,D), the ear leaf (B,E) and the 2nd leaf above the ear (C,F) (mean ± S.E.). Vegetative treatment means nested within reproductive treatments were statistically similar at both dates and at all 3 leaf positions at p < 0.05 (n = 6). Missing bars indicate that leaves were fully senesced at that leaf position.
Discussion
This study reveals the mechanisms by which luxury N uptake accumulated before silking can buffer yield against N and/or water stresses experienced during reproductive growth. With adequate N and water supply imposed five days after silking (NWrep), kernel number and yield were similar between Nveg and nveg, indicating that luxury N did not benefit yield in non-stress conditions. When post-silking N and water were limiting growth factors, yield and kernel abortion were buffered by the presence of luxury N accumulated in the Nveg treatment (Table 4). At the same time, 15N tracers revealed that plants in Nveg remobilized more pre-silking N to the grain under post-silking N and/or water stress and remobilized N comprised an increasingly larger fraction of grain N compared to nveg (Table 2). Notably, pre-silking luxury N uptake was associated with greater maintenance of green leaf area during early and mid grain-fill under post-silking N stress (nWrep and nwrep) (Figure 2), and no reduction in leaf CER (Figure 3) or post-silking N uptake (Table 3) was observed in any reproductive treatment. These data suggest that vegetative N reserves accumulated in the Nveg plants buffered yield by preventing kernel abortion due to: (i) greater N remobilization, (ii) enhanced assimilate production during grain-fill.
Kernel number is particularly sensitive to N stress during the first ∼20 days after silking (Jacobs and Pearson, 1991; Below, 1997; Monneveux et al., 2005), when about 25% of total grain N is accumulated (Ning et al., 2017). The stalk is relied on heavily as a source of remobilized N (Cliquet et al., 1990; Chen et al., 2015; Yang et al., 2016), particularly during the first week or two after silking (Crawford et al., 1982; Ta and Weiland, 1992; Ning et al., 2017). Because the N and/or water stress treatments were imposed five days after silking, it is reasonable to believe that in the Nveg treatment, the reserve of stalk N was remobilized to the grain, preventing abortion of pollinated kernels after silking and improving kernel utilization of assimilates. At silking, stalk N content of Nveg plants was much greater than nveg (1.0 vs. 0.5 g N plant-1; Figure 1) while stalk biomass was similar (88 g plant-1 vs. 84 g plant-1). It appears that the stalk N reservoir can be remobilized under stress to prevent shortfalls in grain N supply. In NWrep, when post-silking N uptake was high relative to the three other post-silking treatments, final kernel number was similar in Nveg and nveg, indicating that this reserve was not required when post-silking N supply was adequate. Because leaf area, leaf CER and total biomass at silking were equivalent between Nveg and nveg, it appears unlikely that shortfalls in assimilate production around silking reduced kernel number in nveg. Rather, the shortfall in grain N supply may have reduced the ability of pollinated kernels to utilize assimilates. Investigating the reasons for decreased yield in N deficient plants, Ning et al. (2018) found that from silking to 20 days after silking, N deficiency reduced the ability of developing kernels to utilize assimilates, leading to feedback inhibition of photosynthesis (i.e., starch accumulation in the ear leaf). The luxury N accumulated in Nveg may have enabled better utilization of assimilates by the developing kernels. A more rigorous challenge to this theory using time-course experiments, with frequent sampling in the 2 weeks following silking, is needed. Yet luxury N reserve may not be as effective in terms of buffering yield if water stress disrupts kernel set at silking. For example, Schussler and Westgate (1994) found that plants with greater N reserves had similar levels of kernel abortion during a 2-day water stress imposed at pollination. In a companion experiment (unpublished), we found that when kernel set was reduced due to vegetative water stress, luxury N uptake did not protect against yield loss.
Importantly, we find that the much larger amount of pre-silking N remobilized in the Nveg treatment did not impinge on physiological processes related to yield. Green leaf area in Nveg from early to mid grain-fill was equal to or greater compared to nveg, which led to greater biomass accumulation in the Nveg treatments under post-silking N stress. Within each post-silking treatment, both leaf CER and post-silking N uptake were similar across Nveg and nveg treatments, indicating that much greater rates of N remobilization were maintained in Nveg without the typically observed reduction in leaf function or post-silking N uptake that typically occurs when N remobilization is enhanced by N stress. It is also notable that despite differences in grain yield (Table 4), post-silking N uptake was similar between Nveg and nveg under reproductive stress (Table 3). Several studies have found that grain yield is a major driver of post-silking N uptake (Akintoye et al., 1999; Worku et al., 2007; Liu et al., 2014), yet data from this experiment suggests that other factors, such as post-silking N availability or pre-silking N uptake, are important regulators of post-silking N uptake. We found that at all three nodal positions on both post-silking sampling dates, leaf CER was consistently greater in NWrep and Nwrep compared to nWrep and nwrep (Figure 3), indicating that post-silking N supply may increase assimilate supply, although this difference in leaf CER was numeric only. Leaf senescence was enhanced by post-silking water stress, irrespective of N supply, similar to the findings of Wolfe et al. (1988). But in conditions where water stress occurs concomitantly with N stress, it appears that accumulating a reserve of vegetative N can reduce the magnitude of the negative effect of water stress on leaf senescence. Mean kernel weight was reduced under combined water/N stress (nwrep) relative to NWrep (231 mg kernel-1 vs. 182 mg kernel-1; Table 4). Because kernel weight reductions occur when post-silking source-sink ratio declines (Borrás and Gambín, 2010; Hisse et al., 2019), it appears that Nveg was unable to completely prevent deterioration in the source-sink ratio during grain-fill under combined post-silking N and water stress.
Surprisingly, spikelet count at silking was affected by the vegetative N treatments (Table 1). Research from field settings research suggests that only severe N stress can reduce spikelet establishment at silking (Lemcoff and Loomis, 1986, 1994; DeBruin et al., 2018). A potential explanation for these results is that in the nveg treatment spikelets were either smaller, or kernel rows were less uniform toward the tip of the ear. Small spikelets and uneven spikelet rows would have reduced the number of spikelets counted at silking. In this experiment, final kernel number was always much less than spikelet number at silking, particularly under N and/or water stress when the proportion of aborted spikelets was 31 and 35% for Nveg and nveg, respectively. This is similar to the findings of Yan et al. (2018), who found that at high planting densities, up to 26% of fertilized kernels can be aborted after fertilization. But even in the NWrep treatment, the proportion of aborted spikelets reached 29% in Nveg and 19% in nveg. The magnitude of kernel number reductions under adequate N and water supply (NWrep) suggests that spikelet number at silking was not a major determinant of final kernel number, since yield was the same in the NWrep treatment. Most studies conclude that spikelet number is not generally a major determinant of final kernel number given that more spikelets are produced than survive to maturity even in non-stress conditions (Tollenaar, 1977; Vega et al., 2001; DeBruin et al., 2018). However, it is possible that by increasing spikelet production, luxury pre-silking N uptake allows for greater plasticity in the response of final kernel number to stress. A larger amount of spikelets at silking would presumably buffer final kernel number if abiotic stress would cause a fixed percentage of kernels to abort.
Several authors have noted the antagonistic relationship between the remobilization of N accumulated during vegetative growth and post-silking N uptake (Gallais et al., 2007; Ciampitti and Vyn, 2013). Mechanistically, this trade-off is thought to be a function of competition for N and assimilates between the grain and the roots (Triboi and Triboi-Blondel, 2002). Root system function declines after silking as deposition of N and carbohydrates in kernels reduces assimilate supply to the roots and enhances N remobilization from the roots (Osaki, 1995; Liedgens et al., 2000; Yu et al., 2014). This intra-plant competition for assimilates is enhanced by N remobilization from leaves, which reduces both overall assimilate production and assimilate allocation to roots (Pan et al., 1995; Rajcan and Tollenaar, 1999). In this experiment, while N remobilization was twice as high in Nveg compared to nveg, there was no difference in post-silking N uptake. This may be because, in Nveg, green leaf area was preserved despite much greater N remobilization. In a recent meta-analysis conducted by Mueller and Vyn (2016), there was surprisingly no evidence of a trade-off between remobilized and post-silking N. The authors speculated that this finding was due to the prevalence of studies in their dataset which used stay-green hybrids, known to maintain green leaf area by reducing leaf N remobilization in the face of strong grain N demand (Antonietta et al., 2014). Our findings provide support for the assertion that if a sufficiently sized reserve of pre-silking N is available, N remobilization that would otherwise reduce source strength is avoided. Kosgey et al. (2013) similarly speculated that utilization of stalk N in maize can delay leaf senescence during reproductive growth. We also found that under post-silking N stress, Nveg plants partitioned a greater fraction of N uptake to vegetative organs, which may have helped preserve organ function despite large amounts of N remobilization. Luxury N reserves accumulated pre-silking appear to resolve the “yield dilemma” in maize by allowing N remobilization to proceed without undue reductions in source strength, uncoupling N remobilization from reductions in post-silking N uptake.
Field studies find that in the absence of severe stress, N remobilization comprises between 35 and 65% of grain N (Ta and Weiland, 1992; Gallais et al., 2007; Ciampitti and Vyn, 2012; de Oliveira Silva et al., 2017). Under severe stress, however, this proportion shifts considerably. In NWrep, N remobilization comprised 53% of grain N, a value comparable to other studies. But under any post-silking N and/or water stress, N remobilization comprised a much greater proportion of grain N, particularly in Nveg plants with a reserve of luxury pre-silking N. This latter finding is comparable to those of other studies which imposed severe post-silking N stress. In a greenhouse study, when N was completely withheld after silking, 100% of grain N was derived from remobilized N (Friedrich and Schrader, 1979). In the field under less contrived conditions, Ta and Weiland (1992) also found that N stress increases the proportion of grain N derived from N remobilization; in one hybrid remobilized N comprised 70% of grain N under N stress. The amount of pre-silking N remobilized to the grain is largely a function of sink strength (i.e., grain yield) (Ciampitti and Vyn, 2013), so while N remobilization is enhanced under N stress, pre-silking N stress severe enough to decreases sink strength would presumably counteract this effect. In the present study, N stress was imposed five days after silking, and the high sink strength in Nveg may have stimulated greater N remobilization. Another explanation for the greater N remobilization observed in this study is the amount of pre-silking N uptake in the Nveg treatment. In the greenhouse, maize can take up substantial amounts of N if large quantities are provided (e.g., Subedi and Ma, 2005); pre-silking N uptake in greenhouse conditions may be greater than what is typically achieved in field conditions, permitting larger amounts of N to be remobilized.
We overcame a major complication associated with using 15N isotope tracers to quantify N remobilization. By growing plants in calcined clay, it was possible to leach 15N–KNO3 using irrigation, allowing for 15N applied during vegetative growth to be removed quickly and prior to reproductive growth. Our findings show that this method eliminates 15N–KNO3 in the pots and hence the chance that 15N will be taken up outside of the intended labelling period. This method can be useful in other 15N approaches and pulse-chase experiments, particularly in those circumstances when hydroponic experiments are not suitable, such as when imposing drought stress or otherwise trying to better replicate field conditions.
Unlike field-based 15N studies which quantify pathways of 15N fluxes in the field (e.g., Portela et al., 2006; O’Brien et al., 2012; de Oliveira Silva et al., 2017), this study was focused on the partitioning of 15N across maize organs (i.e., once assimilated into the plant). Both newly acquired N and previously acquired N are constantly cycling within the maize plant as proteins turn over (Gallais et al., 2006). Yet in this experiment, partitioning of post-silking N was measured only at maturity, reflecting the final destination of N at the end of the plant life cycle. The present study found that at maturity the leaves were very weak sinks for post-silking N, while stalks and roots were strong post-silking N sinks. However, 15N partitioning measured at maturity does not necessarily reflect the organs into which post-silking N was previously incorporated. de Oliveira Silva et al. (2017) measured post-silking N uptake in-season from silking to maturity. They identified much greater allocation of post-silking N to leaves (25–42%) during early and mid grain-fill, and that post-silking N allocation to leaves declined under N stress. In another 15N time-course study, Ta and Weiland (1992) found that while stalk N decreases from V14 to ∼30 days after silking as stalk N is remobilized to the ear, during the latter half of grain-fill stalk N content increases. By maturity, about 10–15% of 15N was found in the leaves depending on hybrid and N supply (Ta and Weiland, 1992). In a separate field study, Yang et al. (2017) found that leaf N content declined from silking to maturity while stalk N content increased. The low values of post-silking N allocated to the leaves in this experiment (less than 5%), and the large values of post-silking N allocated to the stalk and roots, are likely an artifact of the single sampling time at maturity, by which time large amounts of leaf N were already remobilized to other organs, and thus does not reflect in-season N allocation. Time-course measurements of post-silking N uptake are required to assess which organs post-silking N is assimilated into during grain-fill. It is, however, striking that vegetative organs such as the stalk and roots can be major sinks of post-silking N at maturity, and that under certain conditions, only a relatively small fraction of post-silking N uptake is partitioned to the grain.
While extending results from a greenhouse study to the field can only be done with caution, the results of this experiment have important implications for N fertilizer use in maize. Contemporary approaches to N fertilizer management are based on increasing the synchrony between crop N demand and N supply (Cassman et al., 2002; Chen et al., 2011). Maize N demand is typically conceptualized as a function of biomass accumulation rates, and so once crop growth rates are known, one can apply precisely the amount of N required to maintain that crop growth (Cassman et al., 2002; Sadras and Lemaire, 2014). The results of this study point to limitations in N fertilizer management strategies that either conceptualize maize N demand as a function of crop growth rates or take point estimates of crop N sufficiency/deficiency at vegetative stages of development (e.g., remotely sensed canopy reflectance; Pfeffer et al., 2010), since the potential importance of excessive or luxury N uptake in the face of future N stress cannot be accounted for. In years where high N losses are suspected due to weather, or where drought conditions limit post-silking N accessibility, proactive N management that provides for a degree of luxury pre-silking N uptake may be beneficial. Measures of crop N uptake at silking based on a sufficiency index or canopy LAI and leaf N content (Sadras and Lemaire, 2014), may be helpful in assessing luxury N uptake in maize. We also found that maize yield responded positively to additional post-silking N (Nveg to NWrep), suggesting that reactive N management up to R1 may be beneficial. Validation of these conclusions requires further exploration under field conditions, since it is unclear how results from this greenhouse experiment translate to production settings where maize is grown. However, at the level of the individual maize plant, this study demonstrates that luxury N accumulated prior to silking buffers yield under post-silking N and/or water stress.
Data Availability
The datasets generated for this study are available on request to the corresponding author.
Author Contributions
JN conceived the project, led the design of the experiment, data analysis and manuscript writing. HE and BD assisted with project development, experimental design and manuscript writing.
Funding
This research was supported by the Canada First Research Excellence Fund – Grant #499005, Grain Farmers of Ontario – Grant #53198, and an NSERC Industrial Post-Graduate Scholarship – Grant # 52484.
Conflict of Interest Statement
The authors declare that the research was conducted in the absence of any commercial or financial relationships that could be construed as a potential conflict of interest.
Acknowledgments
We would like to thank Donna Hancock, Ken Janovicek, Godfrey Chu, and Javier Di Matteo for their technical expertise. Thank you to Manish Raizada and Tom Bruulsema for their advice in the experimental design. Also a warm thank you to the summer students who assisted on the project: Tianxue (Sunny) He, Dustin Blackall, Yixuan (Mona) Wan, Michael March, Hannah Toews, and David Westerveld for their excellent work in the greenhouse and lab.
Supplementary Material
The Supplementary Material for this article can be found online at: https://www.frontiersin.org/articles/10.3389/fpls.2019.00318/full#supplementary-material
Footnotes
- ^Harvest occurred 39 DAS (Oct 1).
References
Abendroth, L. J., Elmore, R. W., Boyer, M. J., and Marlay, S. K. (2011). Corn Growth and Development. PMR 1009. Ames, IA: Iowa State Univ.
Akintoye, H. A., Kling, J. G., and Lucas, E. O. (1999). N-use efficiency of single, double and synthetic maize lines grown at four N levels in three ecological zones of West Africa. Field Crops Res. 60, 189–199. doi: 10.1016/S0378-4290(98)00122-1
Antonietta, M., Fanello, D. D., Acciaresi, H. A., and Guiamet, J. J. (2014). Senescence and yield responses to plant density in stay green and earlier-senescing maize hybrids from argentina. Field Crops Res. 155, 111–119. doi: 10.1016/j.fcr.2013.09.016
Below, F. E. (1997). “Growth and productivity of maize under nitrogen stress,” in Developing Drought and Low-N Tolerant Maize: Proceedings of a Symposium, eds G. O. Edmeades, M. Bänziger, H. R. Mickelson, and C. B. Peña-Valdivia (El Batan: CIMMYT), 235–240.
Borrás, L., and Gambín, B. L. (2010). Trait dissection of maize kernel weight: towards integrating hierarchical scales using a plant growth approach. Field Crops Res. 118, 1–12. doi: 10.1016/j.fcr.2010.04.010
Borras, L., and Otegui, M. E. (2001). Maize kernel weight response to postflowering source–sink ratio. Crop Sci. 41, 1816–1822. doi: 10.2135/cropsci2001.1816
Buljovcic, Z., and Engels, C. (2001). Nitrate uptake ability by maize roots during and after drought stress. Plant Soil 229, 125–135. doi: 10.1023/A:1004879201623
Cakir, R. (2004). Effect of water stress at different development stages on vegetative and reproductive growth of corn. Field Crops Res. 89, 1–16. doi: 10.1016/j.fcr.2004.01.005
Cassman, K. G., Dobermann, A., and Walters, D. T. (2002). Agroecosystems, nitrogen-use efficiency, and nitrogen management. AMBIO 31, 132–140. doi: 10.1579/0044-7447-31.2.132
Chen, X. P., Cui, Z. L., Vitousek, P. M., Cassman, K. G., Matson, P. A., Bai, J. S., et al. (2011). Integrated soil–crop system management for food security. Proc. Natl. Acad. Sci. U.S.A. 108, 6399–6404. doi: 10.1073/pnas.1101419108
Chen, Y., Xiao, C., Wu, D., Xia, T., Chen, Q., Chen, F., et al. (2015). Effects of nitrogen application rate on grain yield and grain nitrogen concentration in two maize hybrids with contrasting nitrogen remobilization efficiency. Eur. J. Agron. 62, 79–89. doi: 10.1016/j.eja.2014.09.008
Ciampitti, I. A., and Vyn, T. J. (2012). Physiological perspectives of changes over time in maize yield dependency on nitrogen uptake and associated nitrogen efficiencies: a review. Field Crops Res. 133, 48–67. doi: 10.1016/j.fcr.2012.03.008
Ciampitti, I. A., and Vyn, T. J. (2013). Grain nitrogen source changes over time in maize: a review. Crop Sci. 53, 366–377. doi: 10.2135/cropsci2012.07.0439
Cliquet, J. B., Deléens, E., and Mariotti, A. (1990). C and N mobilization from stalk and leaves during kernel filling by 13C and 15N tracing in Zea mays L. Plant Physiol. 94, 1547–1553. doi: 10.1104/pp.94.4.1547
Crawford, T. W., Rendig, V. V., and Broadbent, F. E. (1982). Sources, fluxes, and sinks of nitrogen during early reproductive growth of maize (Zea mays L.). Plant Physiol. 70, 1654–1660. doi: 10.1104/pp.70.6.1654
de Oliveira Silva, A., Camberato, J. J., Coram, T., Filley, T., and Vyn, T. J. (2017). Applicability of a “multi-stage pulse labeling” 15N approach to phenotype N dynamics in maize plant components during the growing season. Front. Plant Sci. 8:1360. doi: 10.3389/fpls.2017.01360
DeBruin, J. L., Hemphill, B., and Schussler, J. R. (2018). Silk development and kernel set in maize as related to nitrogen stress. Crop Sci. 58, 2581–2592. doi: 10.2135/cropsci2018.03.0160
Eagle, A. J., Olander, L. P., Locklier, K. L., Heffernan, J. B., and Bernhardt, E. S. (2017). Fertilizer management and environmental factors drive N 2 O and NO 3 losses in corn: a meta-analysis. Soil Sci. Soc. Am. J. 81, 1191–1202. doi: 10.2136/sssaj2016.09.0281
Echarte, L., Rothstein, S., and Tollenaar, M. (2008). The response of leaf photosynthesis and dry matter accumulation to nitrogen supply in an older and a newer maize hybrid. Crop Sci. 48:656. doi: 10.2135/cropsci2007.06.0366
Echarte, L., and Tollenaar, M. (2006). Kernel set in maize hybrids and their inbred lines exposed to stress. Crop Sci. 46, 870–878. doi: 10.2135/cropsci2005.0204
Friedrich, J. W., and Schrader, L. E. (1979). N Deprivation in maize during grain-filling. Ii. Remobilization of 15n and 35s and the relationship between n and s accumulation 1. Agron. J. 71, 466–472. doi: 10.2134/agronj1979.00021962007100030021x
Gallais, A., and Coque, M. (2005). Genetic variation and selection for nitrogen use efficiency in maize: a synthesis. Maydica 50:531.
Gallais, A., Coque, M., Le Gouis, J., Prioul, J. L., Hirel, B., and Quillere, I. (2007). Estimating the proportion of nitrogen remobilization and of postsilking nitrogen uptake allocated to maize kernels by nitrogen-15 labeling. Crop Sci. 47, 685–691. doi: 10.2135/cropsci2006.08.0523
Gallais, A., Coque, M., Quilléré, I., Prioul, J. L., and Hirel, B. (2006). Modelling postsilking nitrogen fluxes in maize (Zea mays) using 15N-labelling field experiments. New Phytol. 172, 696–707. doi: 10.1111/j.1469-8137.2006.01890.x
Gonzalez-Dugo, V., Durand, J. L., and Gastal, F. (2010). Water deficit and nitrogen nutrition of crops. a review. Agron Sus. Dev. 30, 529–544. doi: 10.1051/agro/2009059
Goron, T. L., Watts, S., Shearer, C., and Raizada, M. N. (2015). Growth in turface® clay permits root hair phenotyping along the entire crown root in cereal crops and demonstrates that root hair growth can extend well beyond the root hair zone. BMC Res. Notes 8:143. doi: 10.1186/s13104-015-1108-x
Hisse, I. R., D’Andrea, K. E., and Otegui, M. E. (2019). Source-sink relations and kernel weight in maize inbred lines and hybrids: responses to contrasting nitrogen supply levels. Field Crops Res. 230, 151–159. doi: 10.1016/j.fcr.2018.10.011
Jacobs, B. C., and Pearson, C. J. (1991). Potential yield of maize, determined by rates of growth and development of ears. Field Crops Res. 27, 281–298. doi: 10.1016/0378-4290(91)90067-6
Kim, K.-I., Clay, D. E., Carlson, C. G., Clay, S. A., and Trooien, T. (2008). Do synergistic relationships between nitrogen and water influence the ability of corn to use nitrogen derived from fertilizer and soil? Agron. J. 100, 551–556. doi: 10.2134/agronj2007.0064
Kosgey, J. R., Moot, D. J., Fletcher, A. L., and McKenzie, B. A. (2013). Dry matter accumulation and post-silking N economy of ‘stay-green’ maize (Zea mays L.) hybrids. Eur. J. Agron. 51, 43–52. doi: 10.1016/j.eja.2013.07.001
Lemaire, G., Charrier, X., and Hébert, Y. (1996). Nitrogen uptake capacities of maize and sorghum crops in different nitrogen and water supply conditions. Agron 16, 231–246. doi: 10.1051/agro:19960403
Lemcoff, J. H., and Loomis, R. S. (1986). Nitrogen influences on yield determination in maize 1. Crop Sci. 26, 1017–1022. doi: 10.2135/cropsci1986.0011183X002600050036x
Lemcoff, J. H., and Loomis, R. S. (1994). Nitrogen and density influences on silk emergence, endosperm development, and grain yield in maize (Zea mays L.). Field Crops Res. 38, 63–72. doi: 10.1016/0378-4290(94)90001-9
Liedgens, M., Soldati, A., Stamp, P., and Richner, W. (2000). Root development of maize (Zea mays L.) as observed with minirhizotrons in lysimeters. Crop Sci. 40, 1665–1672. doi: 10.2135/cropsci2000.4061665x
Liu, C. A., Zhou, L. M., Jia, J. J., Wang, L. J., Si, J. T., Li, X., et al. (2014). Maize yield and water balance is affected by nitrogen application in a film-mulching ridge–furrow system in a semiarid region of China. Eur. J. Agron. 52, 103–111. doi: 10.1016/j.eja.2013.10.001
Mariotti, A., Germon, J. C., Hubert, P., Kaiser, P., Letolle, R., Tardieux, A., et al. (1981). Experimental determination of nitrogen kinetic isotope fractionation: some principles; illustration for the denitrification and nitrification processes. Plant Soil 62, 413–430. doi: 10.1007/BF02374138
Masclaux-Daubresse, C., Daniel-Vedele, F., Dechorgnat, J., Chardon, F., Gaufichon, L., and Suzuki, A. (2010). Nitrogen uptake, assimilation and remobilization in plants: challenges for sustainable and productive agriculture. Ann. Bot. 105, 1141–1157. doi: 10.1093/aob/mcq028
McMurtrie, R. E., and Näsholm, T. (2018). Quantifying the contribution of mass flow to nitrogen acquisition by an individual plant root. New Phytol. 218, 119–130. doi: 10.1111/nph.14927
Monneveux, P., Zaidi, P. H., and Sanchez, C. (2005). Population density and low nitrogen affects yield-associated traits in tropical maize. Crop Sci. 45, 535–545. doi: 10.2135/cropsci2005.0535
Muchow, R. C. (1988). Effect of nitrogen supply on the comparative productivity of maize and sorghum in a semi-arid tropical environment III. grain yield and nitrogen accumulation. Field Crops Res. 18, 31–43. doi: 10.1016/0378-4290(88)90057-3
Mueller, S. M., Camberato, J. J., Messina, C., Shanahan, J., Zhang, H., and Vyn, T. J. (2017). Late-split nitrogen applications increased maize plant nitrogen recovery but not yield under moderate to high nitrogen rates. Agron. J. 109, 2689–2699. doi: 10.2134/agronj2017.05.0282
Mueller, S. M., and Vyn, T. J. (2016). Maize plant resilience to N stress and post-silking N capacity changes over time: a review. Front. Plant Sci. 7:53. doi: 10.3389/fpls.2016.00053
Mueller, S. M., and Vyn, T. J. (2018). Physiological constraints to realizing maize grain yield recovery with silking-stage nitrogen fertilizer applications. Field Crops Res. 228, 102–109. doi: 10.1016/j.fcr.2018.08.025
Ning, P., Fritschi, F. B., and Li, C. (2017). Temporal dynamics of post-silking nitrogen fluxes and their effects on grain yield in maize under low to high nitrogen inputs. Field Crops Res. 204, 249–259. doi: 10.1016/j.fcr.2017.01.022
Ning, P., Yang, L., Li, C., and Fritschi, F. B. (2018). Post-silking carbon partitioning under nitrogen deficiency revealed sink limitation of grain yield in maize. J. Exp. Bot. 69, 1707–1719. doi: 10.1093/jxb/erx496
O’Brien, J. M., Hamilton, S. K., Podzikowski, L., and Ostrom, N. (2012). The fate of assimilated nitrogen in streams: an in situ benthic chamber study. Freshwater Biol. 57, 1113–1125. doi: 10.1111/j.1365-2427.2012.02770.x
Osaki, M. (1995). Comparison of productivity between tropical and temperate maize: I. leaf senescence and productivity in relation to nitrogen nutrition. Soil Sci. Plant Nutr. 41, 439–450. doi: 10.1080/00380768.1995.10419606
Palta, J. A., Kobata, T., Turner, N. C., and Fillery, I. R. (1994). Remobilization of carbon and nitrogen in wheat as influenced by postanthesis water deficits. Crop Sci. 34, 118–124. doi: 10.2135/cropsci1994.0011183X003400010021x
Pan, W. L., Camberato, J. J., Moll, R. H., Kamprath, E. J., and Jackson, W. A. (1995). Altering source-sink relationships in prolific maize hybrids: consequences for nitrogen uptake and remobilization. Crop Sci. 35, 836–845. doi: 10.2135/cropsci1995.0011183X003500030034x
Paponov, I. A., and Engels, C. (2005). Effect of nitrogen supply on carbon and nitrogen partitioning after flowering in maize. J. Plant Nutr. Soil Sci. 168, 447–453. doi: 10.1002/jpln.200520505
Pfeffer, A., Stewart, G., Janovicek, K., and Deen, W. (2010). Evaluation of canopy reflectance technology using a delta yield approach. Agron J. 102, 1453–1461. doi: 10.2134/agronj2010.0092
Plénet, D., and Lemaire, G. (2000). Relationships between dynamics of nitrogen uptake and dry matter accumulation in maize crops. determination of critical N concentration. Plant Soil 216, 65–82. doi: 10.1023/A:1004783431055
Portela, S. I., Andriulo, A. E., Sasal, M. C., Mary, B., and Jobbágy, E. G. (2006). Fertilizer vs. organic matter contributions to nitrogen leaching in cropping systems of the Pampas: 15N application in field lysimeters. Plant Soil 289, 265–277. doi: 10.1007/s11104-006-9134-z
Premalal, S. B. (1997). Aggregate Size Effects on Early Season Corn (Zea mays L.) Root Growth and Biomass Accumulation. Ph.D Thesis, University of Guelph, Guelph.
Rajcan, I., and Tollenaar, M. (1999). Source: sink ratio and leaf senescence in maize:: II. Nitrogen metabolism during grain filling. Field Crops Res. 60, 255–265. doi: 10.1016/S0378-4290(98)00143-9
Sadras, V. O., and Lemaire, G. (2014). Quantifying crop nitrogen status for comparisons of agronomic practices and genotypes. Field Crops Res. 164, 54–64. doi: 10.1016/j.fcr.2014.05.006
Schussler, J. R., and Westgate, M. E. (1994). Increasing assimilate reserves does not prevent kernel abortion at low water potential in maize. Crop Sci. 34, 1569–1576. doi: 10.2135/cropsci1994.0011183X003400060028x
Subedi, K. D., and Ma, B. L. (2005). Nitrogen uptake and partitioning in stay-green and leafy maize hybrids. Crop Sci. 45, 740–747. doi: 10.2135/cropsci2005.0740
Ta, C. T., and Weiland, R. T. (1992). Nitrogen partitioning in maize during ear development. Crop Sci. 32, 443–451. doi: 10.2135/cropsci1992.0011183X003200020032x
Teixeira, E. I., George, M., Herreman, T., Brown, H., Fletcher, A., Chakwizira, E., et al. (2014). The impact of water and nitrogen limitation on maize biomass and resource-use efficiencies for radiation, water and nitrogen. Field Crops Res. 168, 109–118. doi: 10.1016/j.fcr.2014.08.002
Tollenaar, M. (1977). Sink source relationships during reproductive development in maize. a review. Maydica 22, 49–75. doi: 10.1093/jxb/eru041
Triboi, E., and Triboi-Blondel, A. M. (2002). Productivity and grain or seed composition: a new approach to an old problem. Euro. J. Agron. 16, 163–186. doi: 10.1016/S1161-0301(01)00146-0
Vega, C. R., Andrade, F. H., Sadras, V. O., Uhart, S. A., and Valentinuz, O. R. (2001). Seed number as a function of growth. a comparative study in soybean, sunflower, and maize. Crop Sci. 41, 748–754. doi: 10.2135/cropsci2001.413748x
Wolfe, D. W., Henderson, D. W., Hsiao, T. C., and Alvino, A. (1988). Interactive water and nitrogen effects on senescence of maize. I. Leaf area duration, nitrogen distribution, and yield. Agron J. 80, 859–864. doi: 10.13287/j.1001-9332.201702.007
Worku, M., Bänziger, M., Friesen, D., and Horst, W. J. (2007). Nitrogen uptake and utilization in contrasting nitrogen efficient tropical maize hybrids. Crop Sci. 47, 519–528. doi: 10.1371/journal.pone.0199492
Yan, P., Chen, Y., Sui, P., Vogel, A., and Zhang, X. (2018). Effect of maize plant morphology on the formation of apical kernels at different sowing dates and under different plant densities. Field Crops Res. 223, 83–92. doi: 10.1016/j.fcr.2018.04.008
Yang, L., Guo, S., Chen, F., Yuan, L., and Mi, G. (2017). Effects of pollination-prevention on leaf senescence and post-silking nitrogen accumulation and remobilization in maize hybrids released in the past four decades in China. Field Crops Res. 203, 106–113. doi: 10.1016/j.fcr.2016.12.022
Yang, L., Guo, S., Chen, Q., Chen, F., Yuan, L., and Mi, G. (2016). Use of the stable nitrogen isotope to reveal the source-sink regulation of nitrogen uptake and remobilization during grain filling phase in maize. PLoS One 11:e0162201. doi: 10.1371/journal.pone.0162201
Keywords: grain nitrogen sources, maize, nitrogen remobilization, nitrogen stress, post-silking nitrogen uptake, water stress, yield
Citation: Nasielski J, Earl H and Deen B (2019) Luxury Vegetative Nitrogen Uptake in Maize Buffers Grain Yield Under Post-silking Water and Nitrogen Stress: A Mechanistic Understanding. Front. Plant Sci. 10:318. doi: 10.3389/fpls.2019.00318
Received: 02 November 2018; Accepted: 27 February 2019;
Published: 26 March 2019.
Edited by:
Victoria Fernandez, Polytechnic University of Madrid, SpainReviewed by:
Sarah M. Mueller, CiBO Technologies, United StatesJason DeBruin, Corteva Agriscience, United States
Copyright © 2019 Nasielski, Earl and Deen. This is an open-access article distributed under the terms of the Creative Commons Attribution License (CC BY). The use, distribution or reproduction in other forums is permitted, provided the original author(s) and the copyright owner(s) are credited and that the original publication in this journal is cited, in accordance with accepted academic practice. No use, distribution or reproduction is permitted which does not comply with these terms.
*Correspondence: Bill Deen, YmRlZW5AdW9ndWVscGguY2E=