- 1Division of Biological Science, Graduate School of Science and Technology, Nara Institute of Science and Technology, Ikoma, Japan
- 2Division for Research Strategy, Institute for Research Initiatives, Nara Institute of Science and Technology, Ikoma, Japan
The parasitic witchweed Striga hermonthica causes devastating damage to crops in sub-Saharan Africa, yet the mechanism of its parasitism is not well understood. Parasitic plants form a special organ called a haustorium to obtain water and nutrients from host plants. The haustorium is induced by host-derived small molecules, collectively named haustorium-inducing factors (HIFs). The most active HIF known to date is 2,6-dimethoxy-p-benzoquinone (DMBQ), originally isolated from sorghum root extracts. It has been suggested that DMBQ is produced by oxidation of its precursor, syringic acid, and that reactive oxygen species (ROS) and peroxidases are involved in the process. However, the roles of ROS in haustorium formation after HIF recognition remain to be elucidated. Here, we investigated the effects of various inhibitors of ROS and ROS-regulating enzymes on haustorium formation in S. hermonthica. Inhibitors of NADPH oxidases and peroxidases inhibited haustorium formation during treatment with DMBQ, syringic acid, and host root extracts, suggesting that ROS production and/or regulation via NADPH oxidases and peroxidases are essential for haustorium formation. We observed hydrogen peroxide accumulation in the haustorium upon treatment with various HIFs. Our results suggest that ROS and ROS-regulating enzymes are indispensable in downstream signaling of HIFs for haustorium formation.
Introduction
Striga hermonthica, a noxious parasitic plant belonging to the Orobanchaceae family, infects economically important cereals, such as sorghum, maize, pearl millet, and rice, and causes huge damage to world agriculture, especially in sub-Saharan Africa. The estimated annual yield losses are over one billion USD, negatively affecting the livelihoods of more than 100 million people (Ejeta, 2007). However, the infection mechanism of S. hermonthica remains poorly understood and thus effective control methods for this pest have not yet been established.
S. hermonthica is an obligate root parasite, depriving the host plant of water and nutrients. Striga identifies the presence of potential hosts at the time of its germination by recognition of host-derived strigolactones (Yoshida and Shirasu, 2012). After germination, the Striga radicle grows toward host roots and forms a special invasive organ, the haustorium, at the tip of its radical. Haustorium formation is characterized by the swelling of the radicle tip and proliferation of hair cells, haustorial hairs, on the surface. The haustorium functions in host attachment, invasion and nutrient transfer, and its formation is critical for the survival of Striga as an obligate parasite (Yoshida et al., 2016). Haustorium formation is initiated after recognition of haustorium-inducing factors (HIFs) derived from host plants. The Striga radicle then halts its growth, and cell expansion and division occur at its tip to form a bulge-like structure. At the same time, epidermal cells on the haustorium-forming sites start to proliferate haustorial hairs (O'Malley and Lynn, 2000). When haustoria are induced by HIFs in vitro, the bulged haustorium structure is formed within 24 h, and stops further development. Upon infection of a living host, haustoria attach to host tissues and internally invade host roots. Inside host tissues, the parasitic haustoria differentiate to form intrusive cells, some of which further differentiate into xylem cells to connect the host xylem with their own upon entry into the host stele (Yoshida et al., 2016). Overall, haustorium formation involves a cascade of molecular and cellular events controlled in a highly precise spatial-temporal manner.
HIFs are known to be derived from host roots. In 1986, Chang and Lynn identified 2,6-dimethoxy-1,4-benzoquinone (DMBQ) as a HIF from root extracts of sorghum, a Striga host plant (Chang and Lynn, 1986). In addition to DMBQ, structurally similar quinones and flavonoids were reported to induce haustoria of Striga and the facultative parasitic plants Triphysaria versicolor and Phtheirospermum japonicum (Albrecht et al., 1999; Cui et al., 2018). Structure-activity relationship studies in Striga asiatica found that HIFs fall within a range of certain redox potentials, suggesting that redox potential is critical for HIF activity (Smith et al., 1996). More recently, we have shown that phenolic compounds with one or two methoxy groups at the 3- and 5-positions and an hydroxyl group at the 4-position have activity for haustorium induction in S. hermonthica (Cui et al., 2018). Plant cell wall lignin polymers are composed of such phenolic compounds, suggesting that the HIFs may originate from lignin. Alteration of the lignin composition in plants affects haustorium-inducing activity, indicating that HIFs indeed originate from lignin biosynthesis or degradation pathways (Cui et al., 2018). Syringic acid, a phenolic acid potentially produced from the degradation of cell wall lignin, can be oxidized and converted to DMBQ (Kim et al., 1998). Based on this data, Keyes et al. (2001) proposed a model in which host cell wall phenolic acid constituents are oxidized by Striga-derived hydrogen peroxide (H2O2) and peroxidase to produce DMBQ. They showed that reduction of H2O2 by the H2O2-scavenging enzyme catalase reduced the haustorium formation rate upon treatment with syringic acid but not with DMBQ (Keyes et al., 2000). Thus, in the model, the reactive oxygen species (ROS) produced by Striga are the rate-limiting molecules for the conversion of phenolic acids to DMBQ (Keyes et al., 2000). Furthermore, quinone oxidoreductases (QRs) have been reported to play an important role in haustorium induction of T. versicolor and in P. japonicum (Bandaranayake et al., 2010; Ishida et al., 2017). Because the QR protein reduces one or two electrons and produces ROS, it was proposed that ROS may also be involved in the haustorium-induction signal (Bandaranayake et al., 2010).
ROS are highly reactive metabolites containing oxygen and are produced in various metabolic processes. Representative ROS are superoxide (), hydroxyl radical (·OH), H2O2, and singlet oxygen (1O2). Because of their high reactivity, accumulation of ROS causes oxidative stress and adversely affects most living organisms (Mittler, 2017); however, a moderate amount of ROS is necessary for growth and for signaling induction in response to environmental stress in plants. In Arabidopsis, treatment with ROS inhibitors impairs root growth (Dunand et al., 2007). ROS also work as important signals in the immune response to pathogens in plants (Mittler, 2017). Upon pathogen infection, pathogen-associated molecular patterns are recognized by cell surface receptors and activation of calcium channels causes an influx of calcium ions into cells. Subsequent activation of NADPH oxidase by calcium-dependent kinase produces H2O2, which functions as a signaling molecule (Kadota et al., 2015; Qi et al., 2017). Peroxidases catalyze the production or regulation of ROS, depending on the catalytic activity of each peroxidase isoform, and also contribute to the pathogen response, cell wall lignification and cell elongation (Kim et al., 2010; Das and Roychoudhury, 2014; Shigeto and Tsutsumi, 2016). Despite the importance of ROS regulation in plant growth and development, the roles of ROS in host-parasitic plant interaction remain poorly understood.
In this study, we aimed to investigate the roles of ROS and related enzymes in haustorium-induction signaling in S. hermonthica. Our pharmacological study found that NADPH oxidases and peroxidases have important roles in haustorium formation before and after perception of HIF signals.
Materials and Methods
Plant Materials
Striga hermonthica seeds were kindly provided by Prof. A. G Babiker (National Research Center, Khartoum, Sudan). Approximately 50–100 mg of Striga seeds were placed into 1.5 ml plastic tubes and sterilized with 20% commercial bleach solution (Kao Ltd., Japan; final concentration of sodium hypochlorite was about 0.6%). The seeds in the bleach solution were mixed by vortexing, left to stand for several seconds to precipitate, and the supernatant was removed. This sterilization procedure was repeated 10 times. The Striga seeds were then rinsed 10 times with sterile water and transferred to 9 cm petri dishes with moistened glass fiber filter paper (GE Healthcare UK Ltd., Little Chalfont, UK). For preconditioning, the petri dish was sealed using surgical tape and incubated at 25°C for 7 d in the dark. Preconditioned Striga seeds were treated with 10 nM strigol (Hirayama and Mori, 1999) at 25°C for 1 d in the dark, and then subjected to haustorium-inducing factor treatments.
For preparation of rice root extracts, rice (Oryza sativa subsp. japonica cv. Koshihikari and cv. Nipponbare) seeds were briefly washed with 70% ethanol, sterilized with 10% commercial bleach solution (Kao Ltd., Japan; final concentration of sodium hypochlorite was about 0.3%) for 30 min and washed at least three times using sterilized water. For germination, the sterilized seeds were transferred to 9 cm petri dishes with moistened filter paper (Advantec Co Ltd., Tokyo, Japan) and incubated at 25°C for 7 d under 16 h light (35 μmol/sm2)/8 h dark conditions. The rice roots were collected, ground under liquid nitrogen to a fine powder and suspended in sterilized water to a concentration of 5% (w/v). The rice extract solution was filter-sterilized and mixed with agar medium to the final concentration indicated.
Chemicals and Reagents
Chemicals used in this study were obtained from the following suppliers; DMBQ (Sigma-Aldrich, Osaka, Japan), syringic acid (Sigma-Aldrich), salicylhydroxamic acid (SHAM, Tokyo Chemical Industry Co., Ltd., Tokyo, Japan), potassium iodide (Wako Pure Chemical Industries, Ltd., Osaka, Japan), potassium benzoate (Wako Pure Chemical Industries, Ltd.), L-ascorbic acid (Wako Pure Chemical Industries, Ltd.), diphenyleneiodunium (DPI, Funakoshi Frontiers Life Science, Tokyo, Japan), umbelliferone (Tokyo Chemical Industry Co., Ltd.), 6,7-dihydroxycoumarin (esculetin, Sigma-Aldrich), phenylarsine oxide (PAO, Sigma-Aldrich), and sodium diethyldithiocarbamate trihydrate (DDC, Sigma-Aldrich). Each chemical was dissolved in DMSO or water at a stock concentration of 10 mM and stored at −20°C until use (Supplementary Table S1).
Enzymes used in this study were obtained from the indicated supplier; superoxide dismutase (SOD, Nacalai Tesque, Inc., Kyoto, Japan), catalase (Wako Pure Chemical Industries, Ltd.), and horse radish peroxidase (HRP, Wako Pure Chemical Industries, Ltd.). SOD, HRP, and catalase were dissolved in phosphate buffer (pH 7.0) at a stock concentration of 104, 104, and 105 U/ml, respectively, and stored at −20°C until use. H2O2 was obtained from Nacalai Tesque.
Visualization of ROS
Intracellular localization of H2O2 was visualized using carboxy-H2DFFDA (Thermo Fisher). Strigol-treated Striga seeds were transferred to plastic 96-well plates with 20–30 seeds per well. One hundred microliter of DMBQ (Sigma-Aldrich) or syringic acid (Sigma-Aldrich) were added to each well at a final concentration of 10 μM and placed at 25°C in the dark. For the control treatment, an equal amount of water was used. After 24 h, the solution in each well was removed and the seedlings were stained with 100 μl of 20 μM carboxy-H2DFFDA reagent for 30 min. The stained seedlings were rinsed with sterile water a few times and observed under a confocal microscope (Leica TCS SP5), using 460 and 508 nm as excitation and emission wavelengths, respectively. For time-lapse photographs, germinated Striga seedlings were mounted on a glass-bottom dish (Matsunami Glass Ind., Ltd., Osaka, Japan) with 10 μM DMBQ and 20 μM carboxy-H2DFFDA, and immediately observed under a Leica TCS SP5 microscope. Time-lapse photos were taken every 30 min for 24 h with 460 and 508 nm excitation and fluorescence wavelengths, respectively.
For visualization, haustoria were induced by 10 μM DMBQ or syringic acid for 24 h and the seedlings were stained with 0.5 mg/ml Nitroblue tetrazolium chloride (NBT: Roche) for 30 min. The stained seedlings were washed with sterile water a few times and observed under light microscopy.
For visualization of NO− and ·OH, Striga haustoria induced by 10 μM DMBQ or syringic acid for 24 h were stained with 10 μM diaminoflurescein-2-diacetate (DAF-2 DA, Goryo Chemical, Inc., Sapporo, Japan) or 10 μM aminophenyl fluorescein (APF, Goryo Chemical, Inc.), respectively, for 30 min, and were then washed with sterile water a few times. The Striga seedlings were observed under a confocal microscope (Leica TCS SP5), using 460 and 508 nm as excitation and fluorescence wavelengths, respectively.
Treatment With ROS Inhibitors and ROS-Related Enzymes
Strigol-treated seeds were transferred to 96-well plates with 20–30 seeds per well. Ten micrometer DMBQ (Sigma-Aldrich) or syringic acid (Sigma-Aldrich), or 0.5% rice root extracts, were added to the germinated Striga seeds together with various ROS inhibitors or ROS-related enzymes. The plates were sealed using surgical tape and kept at 25°C in the dark for 24 h. Haustorium formation was observed under a stereo microscope (Zeiss Stemi-2000) and the haustorium formation rate (expressed as a percentage) was calculated as the number of plants that formed an haustorium divided by the total number of plants used in the experiment. At least three biological replicates were performed for each experiment. Statistically significant differences between samples were detected by either a Student's t-test or a Tukey HSD (honest significant difference) test using Excel or R, respectively.
Results
Effects of ROS Scavengers on Haustorium Induction
To investigate the importance of ROS-scavenging enzymes on haustorium induction, we performed haustorium induction assays with catalase and SOD, which scavenge H2O2 and , respectively. Striga seedlings were exposed to the HIFs, DMBQ, and syringic acid, in the presence of these enzymes for 24 h, and the haustorium formation rate was calculated. Without the addition of enzyme, the haustorium formation rate was almost over 90% with either 10 μM DMBQ or syringic acid. Upon catalase treatment at 104 U/ml, the haustorium formation rate was reduced to 60% in either the DMBQ or syringic acid treatments (Figure 1A). These results indicate that the ROS-scavenger catalase affected haustorium formation in a similar way when induced by either DMBQ or syringic acid.
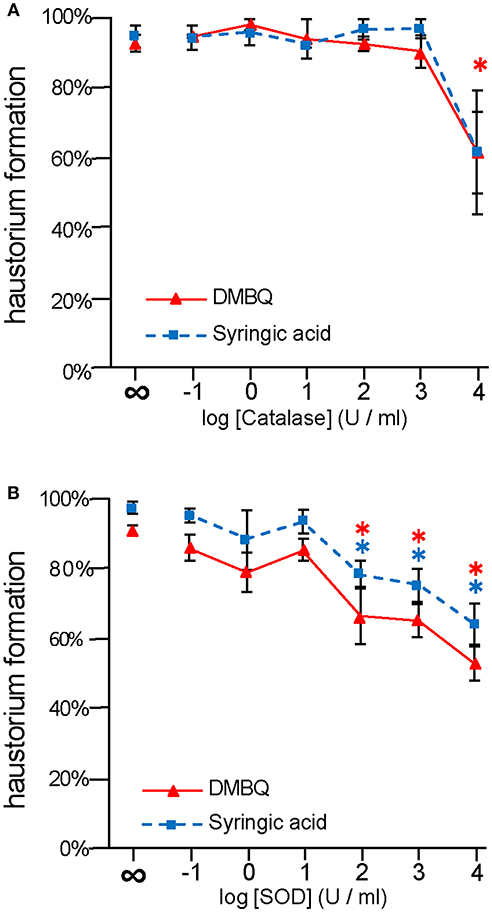
Figure 1. Effects of external application of ROS-scavenging enzymes on haustorium formation. S. hermonthica seedlings were exposed to DMBQ or syringic acid with the ROS-scavenging enzymes, catalase (A) or SOD (B). Ten micrometer of DMBQ (A,B) and 100 μM (A) or 10 μM (B) of syringic acid were used in each experiment to allow comparison with a previous report on catalase (Keyes et al., 2000). After 24 h exposure, haustorium formation rates were evaluated. Experiments were performed at least twice independently with similar results. Red triangles and blue squares indicate treatment with DMBQ and syringic acid, respectively, and error bars indicate standard error (SE: n = 3). ∞ indicates without enzyme control. Asterisks indicate statistically significant differences between samples with and without the indicated enzymes as detected by a Student's t-test (*P < 0.05, red asterisk, DMBQ; blue asterisk, syringic acid).
The haustorium formation rate in the presence of DMBQ or syringic acid was reduced to 60 and 70% at 103 U/ml SOD, respectively. With the addition of 104 U/ml SOD it was reduced to 50% in the presence of DMBQ and 30% in the presence of syringic acid (Figure 1B). These results suggest that removal of from the solution by SOD interfered with haustorium formation. Although there were slight differences in the inhibitory effect of SOD between DMBQ and syringic acid, it appears that was necessary for haustorium formation when induced by either HIFs.
Localization of ROS During Haustorium Formation in S. hermonthica
The above experiments indicate that the presence of ROS is important for haustorium induction. To test whether such ROS accumulate in the parasite tissues, we visualized H2O2 and upon haustorium induction. We used carboxy-H2DFFDA, which is activated by endogenous esterase in presence of H2O2 to emit a green fluorescence, for detecting H2O2 accumulation in living cells. Although this probe can also react with other ROS, it has been widely used in plants to monitor real-time H2O2 production in plants due to its high sensitivity (Swanson et al., 2011). Striga haustoria were induced by DMBQ or syringic acid, stained with carboxy-H2DFFDA, and observed under fluorescence confocal microscopy. In the control, water-treated Striga seedlings, a moderate H2O2 signal was detected at the maturation zone of roots and no detectable signal was observed at the root tips (Figure 2A). In contrast to this, haustorium-forming Striga, which were treated with DMBQ or syringic acid, strongly accumulated H2O2 in the haustorial hairs (Figures 2B,C). Next, we observed a time course of H2O2 accumulation during haustorium formation. The swelling of the radicle tip and proliferation of haustorial hairs were observed approximately 7 h after DMBQ treatment, while at this time point, no significant florescence signal indicating H2O2 accumulation was detected except a very weak signal at the tip of emerging haustorium. The green fluorescence started to appear in haustorial hairs at 14 h after DMBQ treatment and ultimately covered the whole haustorium surface at 24 h (Figure 3 and Supplementary Movies S1A,B). These results indicated that accumulation of H2O2 did not precede haustorium formation and was accompanied by morphological changes in the Striga radicles.
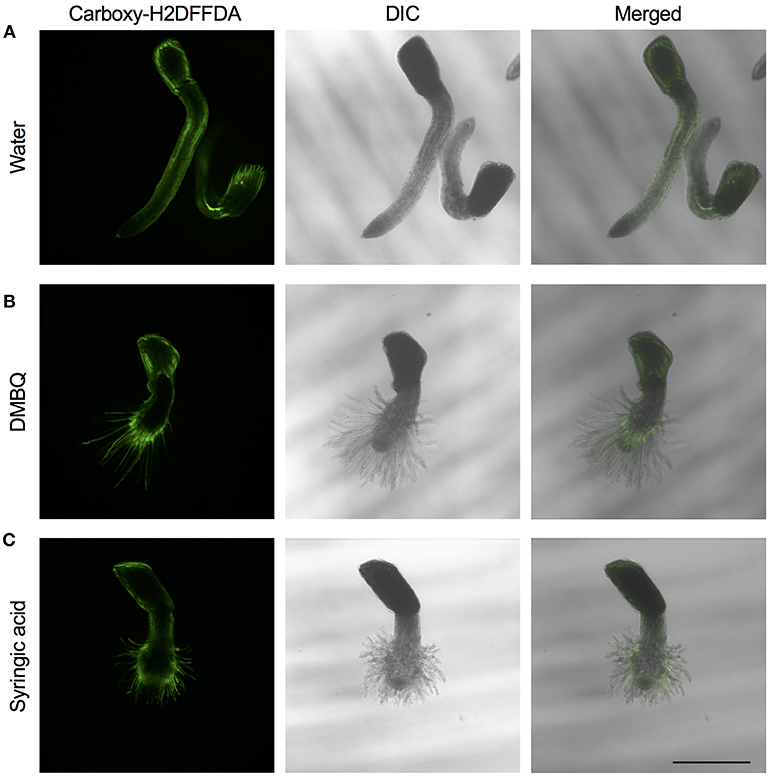
Figure 2. Visualization of H2O2 accumulation in haustoria of S. hermonthica. S. hermonthica seedlings were untreated (A) or treated with 10 μM DMBQ (B) or 10 μM syringic acid (C) for 24 h and accumulation of H2O2 was visualized by carboxy-H2DFFDA staining. Fluorescence images (left panels), DIC images (middle panels), and overlay images (right panels) are shown. Scale bar indicates 500 μm. The experiments were repeated at least three times and representative images are shown.
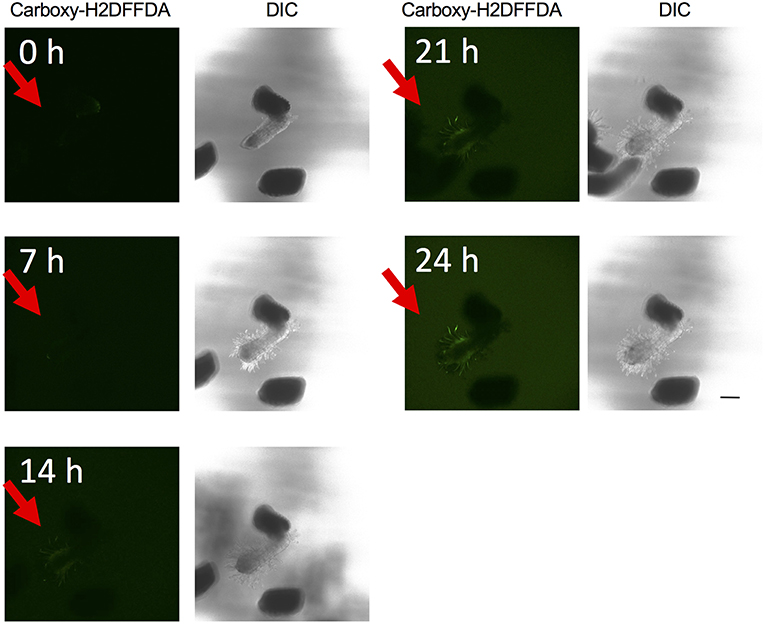
Figure 3. Time course visualization of H2O2 accumulation. H2O2 accumulation was observed by carboxy-H2DFFDA staining (green) at 0, 7, 14, 21, and 24 h after haustorium induction by DMBQ. Each time point is composed of a fluorescence image (left) and a DIC image (right). Each image was derived from the same laser power and exposure time. Red arrows indicate S. hermonthica radicles. The scale bar indicates 200 μm.
For detection of , Striga haustoria were stained with NBT. In the roots of water-treated Striga seedlings, low levels of NBT staining were observed throughout the plant, with strong staining at the root tip (Figure 4A). After haustorium induction by DMBQ or syringic acid, the haustorium tip region was strongly stained by NBT, while the other plant parts including haustorial hairs showed moderate staining (Figures 4B,C). These results suggested that, despite major morphological changes after haustorium induction, was mostly localized at the tips of radicles or haustoria.
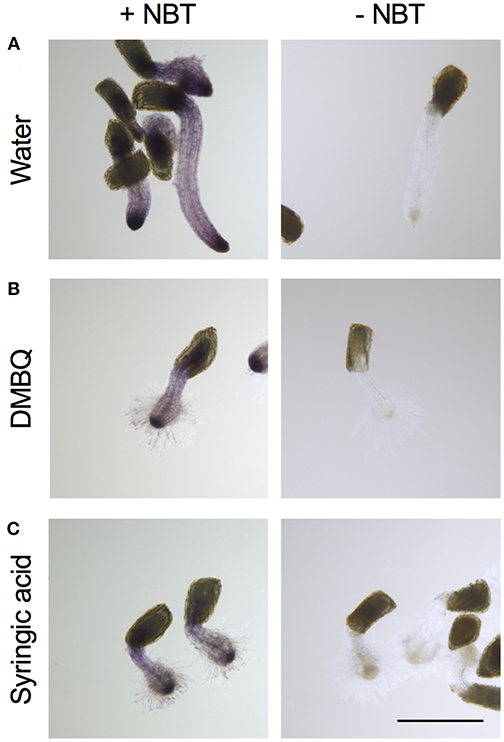
Figure 4. Visualization of accumulation in haustoria of S. hermonthica. S. hermonthica seedlings were untreated (A) or treated with 10 μM DMBQ (B) or 10 μM syringic acid (C) for 24 h and stained with NBT to visualize . NBT-stained seedlings (left panels) and no-staining control (right panels) are shown. Scale bar indicates 500 μm. The experiments were repeated at least three times and representative images are shown.
We also investigated the localization of NO− and ·OH, using DAF-2 DA and APF, respectively. Although NO− signals were observed at a slightly higher level in haustoria than in control roots, these ROS signals were very faint in both the radial tip and the haustorium under our experimental conditions (Supplementary Figure S1). To make conclusions about the accumulation of NO- and ·OH during haustorium formation, a more sensitive assay system is required.
Effects of ROS Inhibitors on Haustorium Induction in S. hermonthica
To further investigate the involvement of ROS in haustorium induction, various ROS scavengers and inhibitors targeting ROS-regulating enzymes were tested during HIF treatments. We used seven inhibitors or modulators with different efficacies; SHAM (a peroxidase inhibitor), potassium iodide (KI) and potassium benzoate (scavengers of H2O2), DPI and PAO [NADPH oxidase inhibitors; (Liszkay et al., 2004)], DDC (an inhibitor of SOD), L-ascorbic acid [an oxygen radical scavenger; (Chen et al., 2013)] and umbelliferone and 6,7-dihydroxycoumarin (esculetin) [peroxidase modulators; (Dunand et al., 2007)]. Germinated Striga seedlings were exposed to various concentrations of each ROS or enzyme inhibitor together with HIFs for 24 h and the haustorium formation rate was calculated. The inhibitors themselves did not induce haustorium except esculetin, which showed a slight haustorium-inducing activity at 100 μM (Figure 5).
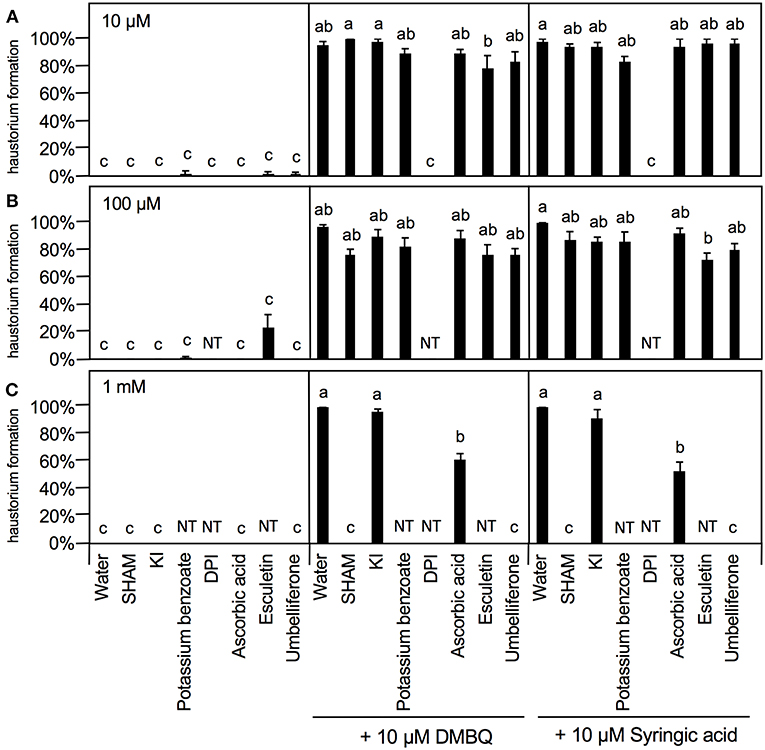
Figure 5. Effects of ROS or ROS-regulating enzyme inhibitors on haustorium formation. Haustorium formation rates were calculated after 24 h treatment with 10 μM (A), 100 μM (B), 1 mM (C) of the inhibitors indicated, in the presence of 10 μM DMBQ or syringic acid. Error bars indicate SE (n = 3), and NT, not tested. Different lower-case letters represent significant differences as determined by a Tukey HSD test (p < 0.05).
At 10 μM, none of the ROS inhibitors displayed any significant inhibitory effects on haustorium formation, except for DPI, which almost entirely blocked haustorium formation (Figure 5A). At 100 μM, esculetin alone significantly reduced haustorium formation by 20% with 10 μM syringic acid treatment (Figure 5B). At 1 mM, SHAM and umbelliferone completely inhibited haustorium formation induced by either DMBQ or syringic acid (Figure 5C, Supplementary Figure S2). Furthermore, 1 mM ascorbic acid reduced the haustorium formation rate to about 60% in both DMBQ and syringic acid treatments (Figure 5C and Supplementary Figure S2). A high concentration (1 mM) of esculetin and potassium benzoate was not tested, because 1 mM esculetin strongly inhibited root tip growth and turned the root tip brown (Supplementary Figure S2), and potassium benzoate was difficult to solubilize at this concentration. The concentration-dependent effects of SHAM were further analyzed. 250 and 500 μM SHAM decreased the haustorium formation rate to about 20 and 0%, respectively (Supplementary Figure S3A). Because KI, a H2O2 scavenger, was not effective at a concentration of 1 mM, we tested higher concentrations. Treatment with 5 mM KI had almost no effect on root growth of Striga seedlings without HIFs (Supplementary Figure S2) and decreased the haustorium formation rate to approximately 20% in both DMBQ and syringic acid treatments (Figure 6A). In addition, we tested PAO as another NADPH oxidase inhibitor. PAO inhibited haustorium formation at 10 μM (Supplementary Figure S3B). These results indicate that NADPH oxidase inhibitors, peroxidase inhibitors and H2O2 scavengers effectively inhibited haustorium formation in S. hermonthica. Because NADPH oxidase produces that is converted to H2O2 by SOD, we examined the effects of the SOD inhibitor, DDC, on haustorium induction. DDC treatment at 150 μM decreased the haustorium formation rate to 10% (Figure 6B), suggesting that H2O2 has a pivotal role in haustorium formation in S. hermonthica.
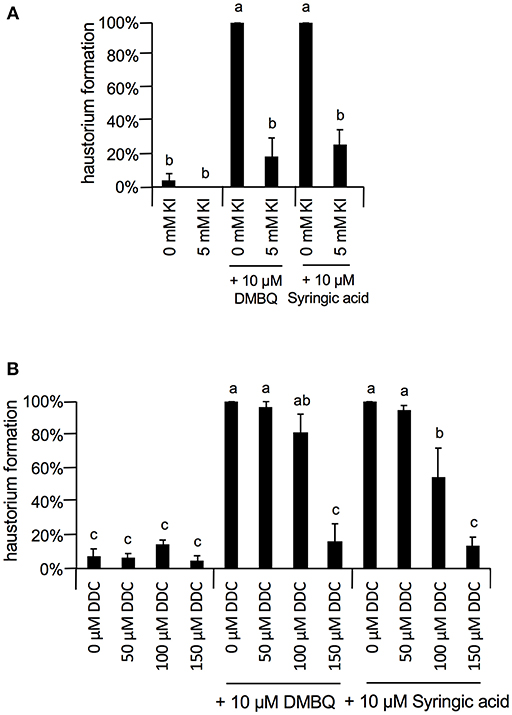
Figure 6. Effects of Kl and DDC on haustorium formation. (A) Effect of 5 mM KI on haustorium induction by DMBQ or syringic acid. (B) Concentration-dependent inhibition of DDC on haustorium formation induced by DMBQ or syringic acid. Error bars indicate SE (n = 3), and NT, not tested. Different lower-case letters represent significant differences as determined by a Tukey HSD test (p < 0.05).
The effects of ROS inhibitors on haustorium formation were also examined for rice root extract-induced haustoria, using extracts from the cultivars Nipponbare and Koshihikari. Nipponbare is a Striga-tolerant cultivar, while Koshihikari is susceptible (Gurney et al., 2006; Yoshida and Shirasu, 2009). The major HIFs produced by rice root extracts have not been identified to date and it is possible that compounds other than DMBQ or syringic acid are responsible for haustorium induction in this plant. At a concentration of 0.5%, the extracts of both cultivars induced haustoria by over 90% (Supplementary Figure S4). Like the results from haustorium induction by DMBQ or syringic acid, haustorium formation was inhibited by 10 μM DPI, 1 mM SHAM, and 1 mM umbelliferone and partially inhibited by 1 mM ascorbic acid (Supplementary Figure S4). The effects of ascorbic acid were slightly but significantly different between susceptible and resistant cultivars, which may reflect the difference in HIFs produced by the extracts of these two cultivars. Overall, the effects of the inhibitors were similar in both the chemical-induced and root extract-induced haustoria, indicating that haustorium induction by rice root extracts occurred in a similar manner to the induction by DMBQ and syringic acid, at least in regards to ROS function.
As the strongest inhibition was observed with 10 μM DPI (Figure 7), we investigated the concentration-dependent inhibition of haustorium formation by this NADPH oxidase inhibitor. The inhibitory effects of DPI were confirmed at 1 μM with a reduction of 60 and 80% in DMBQ and syringic acid treatment, respectively (Figure 7A). Inhibition was not detected at 0.1 μM (Figure 7A). Because DPI is an NADPH oxidase inhibitor and NADPH oxidase is involved in and subsequent H2O2 production, we investigated whether inhibition of haustorium formation by DPI could be restored by exogenous H2O2 application. Application of exogenous H2O2 with DPI did not restore the rate of haustorium formation, either induced by DMBQ or syringic acid (Figure 7B). These results indicate that in planta H2O2 generation by NADPH oxidases is crucial for haustorium formation.
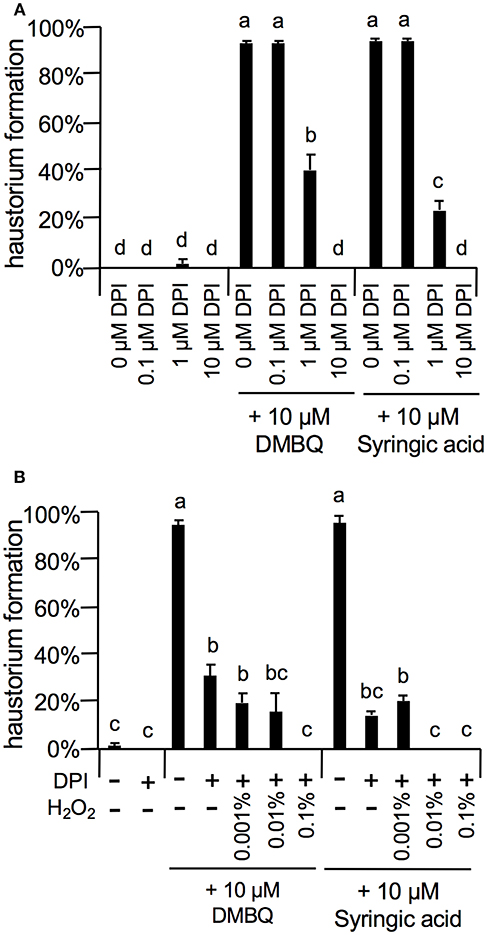
Figure 7. Effects of DPI and external H2O2 on haustorium formation. (A) Haustorium formation rates upon treatment with lower concentrations of DPI in the presence of 10 μM DMBQ or syringic acid. (B) Effects of external H2O2 on haustorium formation rates treated with 1 μM DPI in the presence of 10 μM DMBQ or syringic acid. Error bars indicate SE (n = 3). NT, not tested. Different lower-case letters represent significant differences as determined by a Tukey HSD test (p < 0.05).
Effects of Exogenous Peroxidase on Haustorium Formation
Previous studies suggest that peroxidases, together with H2O2, function in catalyzing the oxidation reaction that converts syringic acid to DMBQ (Kim et al., 1998; Keyes et al., 2000). However, our inhibition experiments above indicated that peroxidases may also be involved in haustorium formation by DMBQ. To further test the effect of peroxidase, we applied an exogenous peroxidase and observed its effect on haustorium formation by DMBQ and syringic acid.
As 10 μM DMBQ and syringic acid showed almost saturated haustorium induction rates (over 90%, Figures 5–8), lower concentrations of these molecules were first tested to compare the activity of DMBQ and syringic acid. One, 0.1, and 0.05 μM DMBQ treatment resulted in an approximately 60, 20, and 10% haustorium formation rate, respectively. On the other hand, 1 μM syringic acid induced haustoria at a rate of 40%, and almost no haustorium-induction activity was observed at concentrations of 0.1 μM or less (Figure 8A). These results suggest that DMBQ has a higher haustorium-induction activity than syringic acid in S. hermonthica.
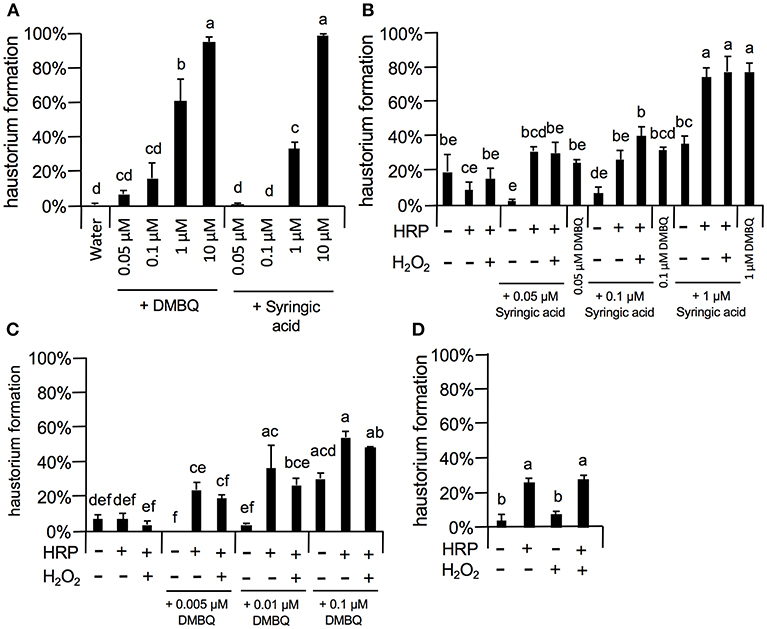
Figure 8. Effects of external peroxidase on haustorium formation by DMBQ and syringic acid. (A) Concentration-dependent haustorium formation rates of DMBQ and syringic acid treatments. S. hermonthica seedlings were exposed to 0.05, 0.1, 1, and 10 μM of DMBQ or syringic acid, and haustorium formation rates were calculated after 24 h. (B,C) Striga seedlings were exposed to a combination of 0.01 U/μl HRP and 0.001% H2O2 in the presence of various concentrations of syringic acid (B) or DMBQ (C) and haustorium formation rates were calculated after 24 h. (D) S. hermonthica seedlings were exposed to a high concentration of HRP at 0.1 U/μl and 0.001% H2O2, and haustorium formation rates were calculated after 24 h. Error bars indicate SE (n = 3). NT, not tested. Different lower-case letters represent significant differences as detected by a Tukey HSD test (p < 0.05).
Next, to assess the haustorium formation-promoting effects of peroxidase, HRP was applied with DMBQ or syringic acid (Figures 8B,C). When HRP (10 U/ml HRP) was applied together with syringic acid at various concentrations, the haustorium formation rate was significantly increased to similar levels of that induced by DMBQ. For example, application of HRP with syringic acid (0.1 μM) increased the haustorium formation rate to 30%, similar to the rate induced by 0.1 μM DMBQ alone. These results support the proposed model where syringic acid is converted to DMBQ by Striga peroxidase (Keyes et al., 2000), at least as measured by haustorium-induction activity. Interestingly, HRP also increased haustorium formation rates with DMBQ (Figure 8C), suggesting that peroxidase has a direct effect on DMBQ or on Striga's perception of HIFs. In contrast to HRP, the addition of H2O2, the co-substrate of peroxidase, did not enhance haustorium formation, possibly due to the presence of H2O2 from Striga (Figures 8B,C). Surprisingly, higher concentrations of HRP (102 U/ml) in the absence of HIFs also induced haustorium formation at up to 20% (Figure 8D), whereas H2O2 alone did not promote haustorium induction. These results suggest that HRP itself can enhance the haustorium formation rate of S. hermonthica at high concentrations.
Discussion
The Role of ROS in Haustorium Formation
Although DMBQ was identified as a HIF from sorghum root extracts (Chang and Lynn, 1986), the mode of action of HIFs is poorly understood. Keyes et al. (2001) reported that the haustorium formation rate in S. asiatica was reduced by catalase treatment when haustoria were induced by syringic acid but not by DMBQ, and concluded that H2O2 is important for the conversion of syringic acid to DMBQ during haustorium formation [Figure 9A; (Keyes et al., 2001)]. However, our results show that catalase (a H2O2 scavenger) and SOD (an scavenger) decrease the rate of haustorium formation even when DMBQ was used as a HIF (Figure 1). These results suggest that ROS have important roles not only in the conversion of syringic acid to DMBQ, but also in the haustorium formation processes provoked by DMBQ (Figure 9B).
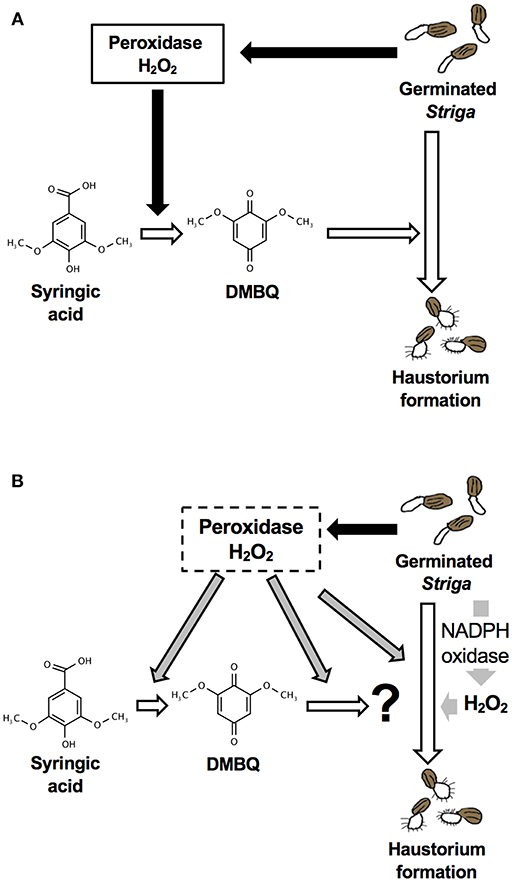
Figure 9. Models for haustorium induction in S. hermonthica. (A) A model from previous studies [modified from (Keyes et al., 2001)]. DMBQ is produced from syringic acid by Striga-derived peroxidase and H2O2. DMBQ acts as a signal for Striga seedlings to form a haustorium. (B) A model suggested by this study. Striga-derived peroxidase can convert syringic acid to DMBQ, which may be further converted to a compound with higher haustorium-inducing activity. In addition, peroxidase enhances haustorium formation independently from HIFs. NADPH oxidase and H2O2 production have a pivotal role in haustorium formation in Striga.
In Arabidopsis, it was reported that H2O2 accumulates at the root tip, especially at the surface of epidermal cells and root hairs in the differentiation zone, and that accumulates in the whole root, and more strongly in the meristem and elongation zone of roots (Dunand et al., 2007). In this study using carboxy-H2DFFDA staining, we did not observe H2O2 accumulation at the radicle tip of Striga before haustorium induction. was detected in the whole plant at low levels and at the radicle tips at high levels, regardless of haustorium formation. In the time-lapse study of H2O2 accumulation, the root tip swelling signature of early haustorium formation was initiated before H2O2 accumulation became apparent (Figure 3). Later, the H2O2 signal was primarily detected in haustorial hairs, similar to that in growing root hairs of normal plants, which agrees with a previous report that haustorial hairs are specialized root hairs in parasitic plants (Cui et al., 2016). Therefore, it is possible that the observed H2O2 accumulated at later stages of haustorium formation may represent its developmental function on cell growth, that involves cell wall modification and expansion. Previous studies in Striga asiatica reported that H2O2 strongly accumulated at the radicle tip and disappeared about 2 h after HIF treatment (Keyes et al., 2007; Fuller et al., 2017). In contrast to this, we observed almost no H2O2 fluorescence at the radicle tip before the haustorium induction treatment, with major H2O2 accumulation appearing after 10–15 h of haustorium induction (Figure 3). It is worth noting that the previous study used S. asiatica whereas we used S. hermonthica, therefore, the time course of H2O2 accumulation may be different among different Striga species. In addition, we could not detect NO− and ·OH in haustoria. Given the characteristic of short half-life of ROS molecules and cell type-dependent permeability of probes, however, further experiments with higher time resolution and different marker combinations are needed to clarify whether various ROS molecules appear at very early stage of haustorium formation.
In our experiments using various ROS inhibitors, the NADPH oxidase inhibitors DPI and PAO strongly inhibited haustorium formation induced by DMBQ and syringic acid. In addition, treatment with a SOD inhibitor, DDC, also inhibited haustorium formation, indicating that H2O2 production is involved in haustorium formation. However, the effect of DPI could not be restored by addition of external H2O2, suggesting that in planta H2O2 generation via NADPH oxidase activity, which is probably regulated in temporal or spatial manners, is required for haustorium induction. NADPH oxidase enzymes are membrane proteins localized on either the plasma membrane or the chloroplast membrane and are encoded by 10 Respiratory Burst Oxidase Homolog (RBOH) genes in Arabidopsis. RBOH genes are expressed in different tissues, among which RBOH A-C, G, and I are expressed specifically in the root elongation zone (Zimmermann et al., 2004; Sagi and Fluhr, 2006). In S. asiatica, Liang et al. (2016) reported that expression of one of the RBOH genes (SaNOX1) decreased at the early stages (~2 h) of haustorium development accompanied by a H2O2 reduction at the root tip (Liang et al., 2016). However, it is possible that S. hermonthica has several RBOH homologs and that each RBOH gene has a distinct physiological function. In future, it would be interesting to verify the function of each RBOH gene in S. hermonthica during haustorium formation in Striga. Overall, these results suggest that haustorium induction involves NADPH oxidase-mediated ROS production.
The Role of Peroxidase in Haustorium Formation
Previous studies proposed that peroxidases are involved in the conversion of syringic acid to DMBQ (Kim et al., 1998). We observed that haustorium-inducing activity was greater with DMBQ treatment than with syringic acid treatment, and that application of HRP increased the haustorium formation rate with syringic acid to a level similar to that of DMBQ. Thus, this result supports the model in which syringic acid is converted to DMBQ by peroxidase. However, HRP also increased the haustorium formation rate with DMBQ treatment. This result suggests that DMBQ may be converted into an unknown compound that has even greater haustorium-induction activity. Alternatively, peroxidase may promote the secretion of another HIF from Striga, or raise its sensitivity to HIFs. In our experiments, 102 U/ml HRP induced a haustorium formation rate of 20% without any additional HIFs. The presence of high concentrations of HRP may have caused cell wall degradation in the Striga seedlings, releasing small amounts of haustorium-inducing quinones and phenolics. This hypothesis is supported by our previous study in which lignin polymers induced haustorium formation in S. hermonthica in the presence of ligninolytic enzymes (Cui et al., 2018). The other possibility is that HRP may directly target the HIF perception signaling pathway, the details of which remain unclear to date. Considering the negative effects of peroxidase inhibitors on haustorium induction, it appears that peroxidases may function to promote haustorium formation.
In conclusion, this study revealed that the haustorium formation rate is affected by the external application of ROS scavengers, NADPH oxidase inhibitors and peroxidase inhibitors, suggesting the importance of endogenous ROS in haustorium formation. Although a previous model indicated the importance of ROS in the production of DMBQ (Figure 9A), our results indicate that ROS, especially H2O2, are indispensable signals acting downstream of DMBQ. Specifically, a certain level of H2O2 production by endogenous NADPH oxidase is important in haustorium formation (Figure 9B). In addition, peroxidases may act downstream of DMBQ to enhance haustorium formation signals (Figure 9B). The effects of ROS inhibitors were similar with treatments using DMBQ, syringic acid and rice root extracts, suggesting that these HIFs target the same pathway or have a similar mode of action. Finally, this study provides evidence that ROS have important roles in haustorium formation downstream of the DMBQ signal. In addition, we propose that ROS inhibitors may be useful as the basis for future Striga control reagents.
Data Availability
All datasets generated for this study are included in the manuscript and/or the Supplementary Files.
Author Contributions
SW, SC, and SY conceived the idea of this study, designed the experiments and wrote the manuscript. SW and SC performed the experiments and data analysis.
Funding
This work was partly supported by KAKENHI grant number 17K15142 to SC, and 18H02464 and 18H04838 to SY. This study was also partly supported by the International Atomic Energy Agency Research Contract Number 20645.
Conflict of Interest Statement
The authors declare that the research was conducted in the absence of any commercial or financial relationships that could be construed as a potential conflict of interest.
Acknowledgments
We thank Prof. Abdel G. E. Babiker (Environment and Natural Resources and Desertification Research Institute, Sudan) for providing the S. hermonthica seeds, and Prof. Kenji Mori for providing strigol.
Supplementary Material
The Supplementary Material for this article can be found online at: https://www.frontiersin.org/articles/10.3389/fpls.2019.00328/full#supplementary-material
Supplementary Figure S1. Visualization of NO− and ·OH. For visualization of NO− (A–C) and ·OH (D–F), haustoria of S. hermonthica induced by 10 μM DMBQ (B,E) or syringic acid (C,F) for 24 h were stained with 10 μM DAF-2 DA (A–C) or 10 μM APF (D–F) for 30 min. Radicals grown in water (A, D) were observed as a control. Left and right panels show confocal microscope images and DIC images, respectively. The scale bar indicates 500 μm.
Supplementary Figure S2. Images of S. hermonthica seedlings treated with ROS inhibitors and DMBQ or syringic acid. Germinated S. hermonthica seedlings were exposed to each inhibitor at indicated concentrations with or without (control) 10 μM DMBQ or syringic acid and observed after 24 h of treatment. White arrowheads indicate brownish radicle tip by high concentration of esculetin treatment.
Supplementary Figure S3. Effects of SHAM and PAO on haustorium formation. (A) Concentration-dependent inhibition of SHAM on haustorium formation induced by DMBQ or syringic acid. (B) Effect of PAO on haustorium induction by DMBQ or syringic acid. Error bars indicate SE (n = 3), and NT, not tested. Different lower-case letters represent significant differences as determined by a Tukey HSD test (p < 0.05).
Supplementary Figure S4. Effects of ROS inhibitors on haustorium formation induced by rice root extracts. Haustorium formation rates were calculated after S. hermonthica seedlings were treated with 0.5% root extracts from cultivars Nipponbare and Koshihikari in the absence or presence of various ROS inhibitors. The concentration of each chemical is shown at the top left of each graph. Error bars indicate SE (n = 3), and NT, not tested. Different lower-case letters represent significant differences as determined by a Tukey HSD test (p < 0.05).
Supplementary Table S1. Chemicals used in this study.
Supplementary Movie S1. Time-lapse movie of H2O2 accumulations during haustorium formation. Time-lapse images H2O2 accumulation visualized by carboxy-H2DFFDA staining were taken every 30 min after with (A) or without (B) DMBQ treatment for 24 h.
References
Albrecht, H., Yoder, J. I., and Phillips, D. A. (1999). Flavonoids promote haustoria formation in the root parasite Tiriphysaria versicolor. Am. Soc. Plant Physiol. 119, 585–591. doi: 10.1104/pp.119.2.585
Bandaranayake, P. C. G., Filappova, T., Tomilov, A., Tomilova, N. B., Jamison-McClung, D., Ngo, Q., et al. (2010). A single-electron reducing quinone oxidoreductase is necessary to induce haustorium development in the root parasitic plant Triphysariam. Plant Cell 22, 1404–1419. doi: 10.1105/tpc.110.074831
Chang, M., and Lynn, D. G. (1986). The haustorium and the chemistry of host recognition in parasitic angiosperms. J. Chem. Ecol. 12, 561–579. doi: 10.1007/BF01020572
Chen, Y.-H., Chao, Y.-Y., Hsu, Y. Y., and Kao, C. H. (2013). Heme oxygenase is involved in H2O2-induced lateral root formation in apocynin-treated rice. Plant Cell Rep. 32, 219–226. doi: 10.1007/s00299-012-1356-3
Cui, S., Wada, S., Tobimatsu, Y., Takeda, Y., Saucet, S. B., Takano, T., et al. (2018). Host lignin composition affects haustorium induction in the parasitic plants Phtheirospermum japonicum and Striga hermonthica. New Phytol. 218, 710–723. doi: 10.1111/nph.15033
Cui, S., Wakatake, T., Hashimoto, K., Saucet, S. B., Toyooka, K., Yoshida, S., et al. (2016). Haustorial hairs are specialized root hairs that support parasitism in the facultative parasitic plant Phtheirospermum japonicum. Plant Physiol. 170, 1492–1503. doi: 10.1104/pp.15.01786
Das, K., and Roychoudhury, A. (2014). Reactive oxygen species (ROS) and response of antioxidants as ROS-scavengers during environmental stress in plants. Front. Environ. Sci. 2, 1–13. doi: 10.3389/fenvs.2014.00053
Dunand, C., Crèvecoeur, M., and Penel, C. (2007). Distribution of superoxide and hydrogen peroxide in Arabidopsis root and their influence on root development: possible interaction with peroxidases. New Phytol. 174, 332–341. doi: 10.1111/j.1469-8137.2007.01995.x
Ejeta, G. (2007). “The Striga scourge in Africa: a growing pandemic,” in Integrating New Technologies for Striga Control, Towards Ending the Witch-Hunt, eds. G. Ejeta, and J. Gressel (London: World Scientific Publishing Co.), 3–16.
Fuller, A. W., Young, P., Pierce, B. D., Kitson-Finuff, J., Jain, P., Schneider, K., et al. (2017). Redox-mediated quorum sensing in plants. PLoS ONE 12:e0182655. doi: 10.1371/journal.pone.0182655
Gurney, A. L., Slate, J., Press, M. C., and Scholes, J. D. (2006). A novel form of resistance in rice to the angiosperm parasite Striga hermonthica. New Phytol. 169, 199–208. doi: 10.1111/j.1469-8137.2005.01560.x
Hirayama, K., and Mori, K. (1999). Plant bioregulators, 5. Synthesis of (+)-strigol and (+)-orobanchol, the germination stimulants, and their stereoisomers by employing lipase-catalyzed asymmetric acetylation as the key step. European J. Org. Chem. 1999, 2211–2217. doi: 10.1002/(SICI)1099-0690(199909)1999:9<2211::AID-EJOC2211>3.0.CO;2-O
Ishida, J. K., Yoshida, S., and Shirasu, K. (2017). Quinone oxidoreductase 2 is involved in haustorium development of the parasitic plant Phtheirospermum japonicum. Plant Signal. Behav. 12:e1319029. doi: 10.1080/15592324.2017.1319029
Kadota, Y., Shirasu, K., and Zipfel, C. (2015). Regulation of the NADPH oxidase RBOHD during plant immunity. Plant Cell Physiol. 56, 1472–1480. doi: 10.1093/pcp/pcv063
Keyes, W. J., O'Malley, R. C., Kim, D., and Lynn, D. G. (2000). Signaling organogenesis in parasitic angiosperms: xenognosin generation, perception, and response. J. Plant Growth Regul. 19, 217–231. doi: 10.1007/s003440000024
Keyes, W. J., Palmer, A. G., Erbil, W. K., Taylor, J. V., Apkarian, R. P., Weeks, E. R., et al. (2007). Semagenesis and the parasitic angiosperm Striga asiatica. Plant J. 51, 707–716. doi: 10.1111/j.1365-313X.2007.03171.x
Keyes, W. J., Taylor, J. V., Apkarian, R. P., and Lynn, D. G. (2001). Dancing together. Social controls in parasitic plant development. Plant Physiol. 127, 1508–1512. doi: 10.1104/pp.010753
Kim, D., Kocz, R., Boone, L., Keyes, W. J., and Lynn, D. G. (1998). On becoming a parasite: evaluating the role of wall oxidases in parasitic plant development. Chem. Biol. 5, 103–117. doi: 10.1016/S1074-5521(98)90144-2
Kim, M. J., Ciani, S., and Schachtman, D. P. (2010). A peroxidase contributes to ros production during Arabidopsis root response to potassium deficiency. Mol. Plant 3, 420–427. doi: 10.1093/mp/ssp121
Liang, L., Liu, Y., Jariwala, J., Lynn, D. G., and Palmer, A. G. (2016). Detection and adaptation in parasitic angiosperm host selection. Am. J. Plant Sci. 7, 1275–1290. doi: 10.4236/ajps.2016.78123
Liszkay, A., van der Zalm, E., and Schopfer, P. (2004). Production of reactive oxygen intermediates (O 2 2, H 2 O 2, and OH) by maize roots and their role in wall loosening and elongation growth. Plant Physiol. 136, 3114–3123. doi: 10.1104/pp.104.044784
O'Malley, R. C., and Lynn, D. G. (2000). Expansin message regulation in parasitic angiosperms: marking time in development. Plant Cell 12, 1455–1465. doi: 10.1105/tpc.12.8.1455
Qi, J., Wang, J., Gong, Z., and Zhou, J. M. (2017). Apoplastic ROS signaling in plant immunity. Curr. Opin. Plant Biol. 38, 92–100. doi: 10.1016/j.pbi.2017.04.022
Sagi, M., and Fluhr, R. (2006). Production of reactive oxygen species by plant NADPH oxidases. Plant Physiol. 141, 336–340. doi: 10.1104/pp.106.078089
Shigeto, J., and Tsutsumi, Y. (2016). Diverse functions and reactions of class III peroxidases. New Phytol. 209, 1395–1402. doi: 10.1111/nph.13738
Smith, C. E., Ruttledge, T., Zeng, Z., O'Malley, R. C., and Lynn, D. G. (1996). A mechanism for inducing plant development: the genesis of a specific inhibitor. Proc. Natl. Acad. Sci. U.S.A. 93, 6986–6991. doi: 10.1073/pnas.93.14.6986
Swanson, S. J., Choi, W. G., Chanoca, A., and Gilroy, S. (2011). In vivo imaging of Ca2+, pH, and reactive oxygen species using fluorescent probes in plants. Annu. Rev. Plant Biol. 62, 273–297. doi: 10.1146/annurev-arplant-042110-103832
Yoshida, S., Cui, S., Ichihashi, Y., and Shirasu, K. (2016). The haustorium, a specialized invasive organ in parasitic plants. Annu. Rev. Plant Biol. 67, 643–667. doi: 10.1146/annurev-arplant-043015-111702
Yoshida, S., and Shirasu, K. (2009). Multiple layers of incompatibility to parasitic witch weed, Striga hermonthica. New Phytol. 183, 180–189. doi: 10.1111/j.1469-8137.2009.02840.x
Yoshida, S., and Shirasu, K. (2012). Plants that attack plants: molecular elucidation of plant parasitism. Curr. Opin. Plant Biol. 15, 708–713. doi: 10.1016/j.pbi.2012.07.004
Keywords: parasitic plant, ROS, peroxidase, haustorium, inhibitor, DMBQ, Striga
Citation: Wada S, Cui S and Yoshida S (2019) Reactive Oxygen Species (ROS) Generation Is Indispensable for Haustorium Formation of the Root Parasitic Plant Striga hermonthica. Front. Plant Sci. 10:328. doi: 10.3389/fpls.2019.00328
Received: 11 December 2018; Accepted: 28 February 2019;
Published: 22 March 2019.
Edited by:
Christophe Dunand, Université Toulouse III Paul Sabatier, FranceReviewed by:
Honghong Wu, University of California, Riverside, United StatesAnkush Prasad, Palacký University Olomouc, Czechia
Copyright © 2019 Wada, Cui and Yoshida. This is an open-access article distributed under the terms of the Creative Commons Attribution License (CC BY). The use, distribution or reproduction in other forums is permitted, provided the original author(s) and the copyright owner(s) are credited and that the original publication in this journal is cited, in accordance with accepted academic practice. No use, distribution or reproduction is permitted which does not comply with these terms.
*Correspondence: Satoko Yoshida, c2F0b2tveUBicy5uYWlzdC5qcA==