- 1Department of Energy Plant Research Laboratory, Michigan State University, East Lansing, MI, United States
- 2Department of Biochemistry and Molecular Biology, Michigan State University, East Lansing, MI, United States
The conversion of sunlight into useable cellular energy occurs via the proton–coupled electron transfer reactions of photosynthesis. Light is absorbed by photosynthetic pigments and transferred to photochemical reaction centers to initiate electron and proton transfer reactions to store energy in a redox gradient and an electrochemical proton gradient (proton motive force, pmf), composed of a concentration gradient (ΔpH) and an electric field (Δψ), which drives the synthesis of ATP through the thylakoid FoF1-ATP synthase. Although ATP synthase structure and function are conserved across biological kingdoms, the number of membrane–embedded ion–binding c subunits varies between organisms, ranging from 8 to 17, theoretically altering the H+/ATP ratio for different ATP synthase complexes, with profound implications for the bioenergetic processes of cellular metabolism. Of the known c–ring stoichiometries, photosynthetic c–rings are among the largest identified stoichiometries, and it has been proposed that decreasing the c-stoichiometry could increase the energy conversion efficiency of photosynthesis. Indeed, there is strong evidence that the high H+/ATP of the chloroplast ATP synthase results in a low ATP/nicotinamide adenine dinucleotide phosphate (NADPH) ratio produced by photosynthetic linear electron flow, requiring secondary processes such as cyclic electron flow to support downstream metabolism. We hypothesize that the larger c subunit stoichiometry observed in photosynthetic ATP synthases was selected for because it allows the thylakoid to maintain pmf in a range where ATP synthesis is supported, but avoids excess Δψ and ΔpH, both of which can lead to production of reactive oxygen species and subsequent photodamage. Numerical kinetic simulations of the energetics of chloroplast photosynthetic reactions with altered c–ring size predicts the energy storage of pmf and its effects on the photochemical reaction centers strongly support this hypothesis, suggesting that, despite the low efficiency and suboptimal ATP/NADPH ratio, a high H+/ATP is favored to avoid photodamage. This has important implications for the evolution and regulation of photosynthesis as well as for synthetic biology efforts to alter photosynthetic efficiency by engineering the ATP synthase.
Introduction
Oxygenic photosynthetic membranes use light to excite electrons on special chlorophyll molecules to store energy in two forms. Redox energy is stored by light-driven extraction of electrons from water to reduce NADP+ to nicotinamide adenine dinucleotide phosphate (NADPH). Phosphorylation potential is stored by a chemiosmotic mechanism (Mitchell, 1961; Mitchell, 1966), coupling the light driven electron transfer reactions to the generation of a proton electrochemical gradient (proton motive force, pmf), which in turn drives the synthesis of ATP from ADP + Pi through an F-type ATP synthase (reviewed in (Boyer, 1997; Junge and Nelson, 2015).
In green algae and higher plant chloroplasts, pmf is stored across the thylakoid membrane in both transmembrane electric field (Δψ) and a proton concentration gradient (ΔpH) (Cruz et al., 2001), differing from mitochondrial respiratory membranes and most plama membranes, across which the pmf is primarily composed of Δψ (Kashket, 1981; Booth, 1985). Both components are thermodynamically interchangeable driving forces for the chloroplast ATP synthase (Hangarter and Good, 1982; Gräber et al., 1984) so that the total driving force for ATP synthesis can be described as:
where Δψi-o and ΔpHo-i represent the electric field and proton gradient calculated as the difference in concentrations between the inside (lumen) and outside (stroma), R is the universal gas constant, and F is Faraday’s constant.
During steady-state photosynthesis, pmf is generated by light-driven proton translocation and subsequently consumed by H+ efflux from the lumen through the ATP synthase, which are regulated in interdependent ways. The formation of pmf is also governed by electron transfer rates, which in turn are controlled by “photosynthetic control,” i.e., the slowing of plastoquinol (PQH2) oxidation ten–fold from pH 7.5 to 5.5 at the cytochrome b6f complex as lumen pH decreases below about 6.5 (Nishio and Whitmarsh, 1993; Hope et al., 1994; Takizawa et al., 2007). In effect, the formation of ΔpH and acidification of the lumen is self-controlled.
The consumption of pmf is regulated by control of ATP synthase activity (Kanazawa and Kramer, 2002; Cruz et al., 2005a; Takizawa et al., 2008), which at least in green algae and plants responds to decreases in the capacity of the cell to use photosynthetic energy by restricting the efflux of protons, resulting in buildup of pmf and subsequent acidification of the lumen, leading to increased photosynthetic control (reviewed in (Strand and Kramer, 2014) and activation of qE, the rapidly reversible form of nonphotochemical quenching (NPQ) (Niyogi and Truong, 2013). As will be discussed below, the impact and mechanisms of pmf regulation may be different in other photosynthetic lineages, as in cyanobacteria in which some evidence suggests that pH may not regulate NPQ (Kirilovsky and Kerfeld, 2012).
The buildup of thylakoid ΔpH can have additional effects on the photosynthetic machinery. Strong lumen acidification has been shown, in vitro, to release Ca2+ from the photosystem II (PSII) oxygen evolving complex (OEC) (Krieger and Weis, 1993), as well as slowing the release of protons from the OEC during PSII turnover (Zaharieva et al., 2011). Based on surveys of experimental data on both pH–mediated regulation and damage to photosynthetic proteins, it was proposed that pmf is regulated so that the lumen pH remains above about 5.5 except under environmental stresses (Kramer et al., 1999). In this case, maintaining sufficient free energy in ATP (ΔGATP) requires the storage of at least part of pmf in the form of Δψ (Kramer et al., 2004; Cruz et al., 2005b; Zhang et al., 2009; Davis et al., 2016). This requirement has been validated by observations that the Δψ/ΔpH ratio is sensitive to environmental stresses (Avenson et al., 2004; Zhang et al., 2009; Davis et al., 2016) and is controlled by specific ion transporters (Checchetto et al., 2012; Armbruster et al., 2014; Kunz et al., 2014; Duan et al., 2016; Herdean et al., 2016a; Herdean et al., 2016b; Schneider et al., 2016; Hohner et al., 2019).
While Δψ can support ATP synthesis in thylakoids with only moderate or no lumen acidification (Hangarter and Good, 1982; Gräber et al., 1984), a large amplitude Δψ also has important secondary effects, most importantly in decreasing the free energy barrier for charge recombination in photosynthetic reaction centers (Crofts et al., 1971; Vos et al., 1991; Davis et al., 2016), thus enhancing the production of reactive oxygen species (ROS), particularly singlet oxygen (1O2) by PSII (Davis et al., 2016). Substantial rates of 1O2 production can be observed even in wild-type plants during rapid fluctuations in actinic light, which generate large, transient amplitudes of Δψ, which occur more rapidly than feedback regulation of the light reactions (Davis et al., 2017). We surmise that, because the core electron transfer protein complexes of photosynthesis (PSII, cytochrome b6f, and PSI) are highly conserved across all oxygenic photosynthetic organisms (Hasan and Cramer, 2012; Cardona, 2015), the effects of pmf composition on these reactions are likely to represent a common (if not universal) constraint on photosynthetic energy storage, and that evolution will have selected for systems that can adequately balance the storage of pmf in Δψ and ΔpH to balance the needs for efficient ATP synthesis, homeostasis, and the avoidance of excess ROS production.
Natural Variation in the Stoichiometry of F0c-Subunits
ATP synthesis in F0F1 ATP synthases is thought to involve rotational movement of the membrane embedded F0 portion driven by a single ion binding by each c–subunit, which is coupled to the catalytic turnover of the F1 α3β3 hexamer to release three ATP molecules per full turnover of the complex (reviewed in Junge and Nelson, 2015). In this mechanism, a full turnover of the F1 enzyme is coupled to complete 360° rotation of the c–ring, generating three ATP per c-subunits, dictating that the number of protons required to generate three ATPs (n H+/ATP) will be equal to the number of c-subunits (Watt et al., 2010). It should be noted that the actual H+/ATP stoichiometries have not yet been validated by direct experimentation, and some measurements, based on thermodynamics of pmf and ΔGATP, suggest H+/ATP of about 4 for both spinach and Escherichia coli, independent of the c subunit stoichiometry (Turina et al., 2003; Steigmiller et al., 2008). On the other hand, the stoichiometries of bioenergetic processes are notoriously difficult to measure [see e.g., (Steigmiller et al., 2008; Ferguson, 2010)] and while we consider the actual stoichiometry as yet unresolved, the majority of evidence suggests that it should be predominantly controlled by the c subunit stoichiometry (Steigmiller et al., 2008). Despite differences in the number of c subunits in different organisms, the rotational catalysis mechanism appears to be conserved across biological kingdoms (von Ballmoos et al., 2009; Kuhlbrandt, 2019) and various bioenergetic membranes (Koumandou and Kossida, 2014). While the mechanisms of regulation and the absence/presence of certain peripheral subunits vary between species (Walker, 2013), the core subunits of both F0 and F1 portions are highly conserved, with the striking exception that the number of c subunits that compose the F0 ring varies from 8 to 17 (Figure 1) (Pogoryelov et al., 2012; Kuhlbrandt, 2019), implying that different species evolved to have widely different H+/ATP ratios.
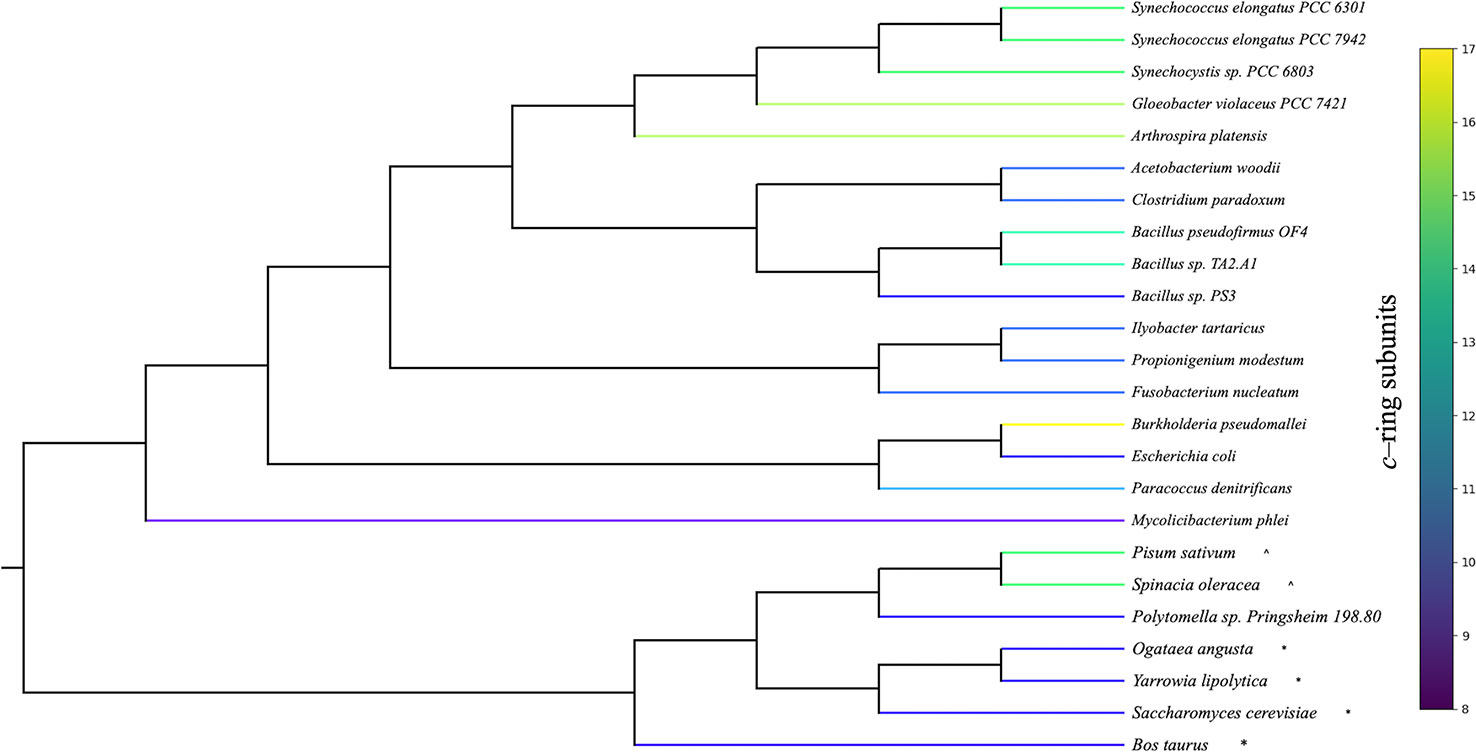
Figure 1 Phylogenetic organization of organisms with known adenosine triphosphate (ATP) synthase c–ring stoichiometries. Rooted phylogeny of organisms with experimentally determined c–ring stoichiometries retrieved from the National Center for Biotechnology Information (NCBI) taxonomy database. Organism branches colored according to the number of c subunits found in the ATP synthase c–ring. Cyanobacterial stoichiometries were determined from photosynthetic membranes, and stoichiometries from mitochondria (*) and chloroplasts (^) are indicated for eukaryotic organisms.
The determinants of c subunit stoichiometry are not yet fully understood (Ferguson, 2000; Ferguson, 2010). Each c subunit forms two membrane–spanning α–helices embedded within the membrane connected by an F1 facing loop (Vonck et al., 2002). Based on structural data from intact c–rings, amino acid differences near the N-terminal glycine motif may alter steric and chemical interactions between adjacent α–helices (Vonck et al., 2002), mediating how closely the c subunits can pack together (MacKenzie et al., 1997; Watt et al., 2010), with smaller amino acids providing closer packing and smaller c–ring stoichiometries (Pogoryelov et al., 2012). While the exact determinants for the c–ring stoichiometry is not yet evident, the size does appear to be genetically encoded, resulting from the c subunit primary sequence (Muller et al., 2001; Arechaga et al., 2002).
Expressing non–native c subunits, either from a different organism or by mutations, results in the assembly of functional chimeric ATP synthases (Laubinger et al., 1990; Suzuki et al., 2007; Liu et al., 2011; Pogoryelov et al., 2012). Supporting the role of c subunit primary sequence in determining ring size, replacing only the endogenous c subunit gene in E. coli ATP synthase with genes from other organisms resulted in c–ring stoichiometries matching the organism from which the exogenous c subunit was derived, rather than the host E. coli stoichiometry (Meier et al., 2005; Matthies et al., 2009). Importantly, the c–ring size appears to be determined solely by the sequence of the c subunits, and thus remains constant in a species, and does not vary with physiological state (Meyer Zu Tittingdorf et al., 2004; Ballhausen et al., 2009).
Adenosine Triphosphate Synthesis Energetics Are Impacted by c–Ring Stoichiometry
During catalytic turnover of the assembled ATP synthase, the overall rate limitation occurs in the F1 portion (Feniouk et al., 2004) and has been attributed to nucleotide binding and exchange (Panke and Rumberg, 1996). However, in thylakoids, the rate of ATP synthase turnover is strongly dependent on the amplitude of pmf (Kramer and Crofts, 1989). The ATP synthase is inactive at low pmf (Kaplan et al., 1967; Smith et al., 1976), but above the threshold pmf required to activate the complex, essentially linear (ohmic) with pmf (Smith et al., 1976; Hangarter and Good, 1982; Kramer and Crofts, 1989; Junesch and Graber, 1991). This implies that the rate-limiting step in vivo requires pmf, and thus changing the c stoichiometry should alter not only the thermodynamics (von Ballmoos et al., 2009; Watt et al., 2010; Silverstein, 2014) but also the kineics of ATP synthesis. Indeed, ATP synthases with different c–ring stoichiometries have different pmf activation thresholds, with larger c–ring complexes becoming active (Kaim and Dimroth, 1999) and having higher turnover rates at lower pmf amplitudes (Pogoryelov et al., 2012). It has been postulated that the kinetic effects of larger rings are due to increased torque resulting from the smaller step-wise rotation imposed by each proton translocation (von Ballmoos et al., 2008; von Ballmoos et al., 2009), much as shifting to a lower gear on a bicycle allows a rider to mount a steeper hill but at the cost of more energy input per distance traveled.
It is intriguing that the number of c subunits in photosynthetic organisms are all on the high end (13–15 subunits) of the determined c–ring stoichiometries (Seelert et al., 2000; Pogoryelov et al., 2005; Pogoryelov et al., 2007), including Gloeobacter violaceus PCC 7421, a phylogenetically ancestral, low–light requiring cyanobacterium originally isolated from calcareous rocks (Rippka et al., 1974; Nakamura et al., 2003), which was found to contain a c15 ring (Pogoryelov et al., 2007).
The energy required to catalyze the synthesis of ATP (ΔGATP) is given by:
where n is the H+/ATP ratio required to generate each molecule of ATP dictated by the number of c–subunits. Assuming the same ΔGATP between organisms, larger c–rings should overcome the energetic barrier for ATP production with a smaller pmf, but with a higher overall energy (H+) cost. The apparent high H+/ATP ratio in chloroplasts (n=4.67) decreases the pmf required to overcome ΔGATP, allowing photosynthesis to produce ATP at a lower relative pmf (Kaim and Dimroth, 1999), reducing the requirement to maintain a large pmf (either ΔpH or Δψ) during steady–state photosynthesis.
However, a higher H+/ATP implies that the output of ATP/NADPH for linear electron flow (LEF) will be lower, and in the case of chloroplasts, should result in 2.57 ATP/2 NADPH, below that needed to support the assimilatory reactions of the Calvin-Benson-Bassham (CBB) cycle (Allen, 2003). The resulting energy imbalance requires that chloroplasts activate processes to make up the differences in response to photosynthetic output capacity (Kramer and Evans, 2011). These include cyclic electron flow (reviewed in Strand et al., 2016), the water-water-cycle (Asada, 1999), the malate valve (Scheibe, 2004), as well as balancing the adenylate and electron (either ferredoxin or NADPH) requirements of other metabolic processes (Noctor and Foyer, 2000; Walker et al., 2014; Morales et al., 2018) all of which consume (directly or indirectly) photosynthetic energy. Thus, the large c stoichiometries in chloroplasts decrease energy efficiency both at the ATP synthase itself and in imposing a need for additional ATP producing reactions that decrease overall quantum efficiency of photosynthesis.
The Role of the Adenosine Triphosphate Synthase in Feedback Regulation of Photosynthesis
Whereas mitochondria have been found to store pmf primarily in Δψ, chloroplasts store a fraction of pmf as ΔpH; partly as a means of feedback regulation of the light reactions, chloroplasts have evolved mechanisms to alter the partitioning of pmf into ΔpH, probably to allow for lumen pH-induced regulation of light capture and electron flow to coordinate with downstream metabolic reactions and avoid over-reduction of PSI cofactors (Kanazawa et al., 2017), while maintaining sufficient Δψ to avoid over–acidification of the lumen (Kramer et al., 1999; Cruz et al., 2001). This has led some to hypothesize that the large c–ring stoichiometry in chloroplasts is required to accommodate a smaller Δψ (von Ballmoos et al., 2008). Using isolated ATP synthases incorporated into liposomes, the Δψ required to activate ATP synthesis activity was found to be inversely proportional to the c–ring size (Kaim and Dimroth, 1999), so that systems with larger stoichiometries should be able to produce ATP at lower pmf values. However, while this observation may explain a benefit of larger c–rings during induction, this fails to address why a large steady-state Δψ is not maintained by chloroplasts. Would the large pmf required for ATP synthesis with smaller c stoichiometries result in deleterious side reactions in the photosynthetic membrane, and if so, could this contribute to an apparent selection for larger c–rings?
Can c–Subunit Stoichiometry Be Tuned to Optimize the Thermodynamic Efficiency of Proton–Coupled Adenosine Triphosphate Synthesis?
Based on a flux model from available pmf and ATP substrate parameters, Silverstein (Silverstein, 2014) estimated that, with similar previous experimentally measured pmf and ΔGATP levels, the E. coli and bovine mitochondrial ATP synthases (c10 and c8, respectively) should convert pmf to ΔGATP with about 25% higher efficiency compared to the chloroplast ATP synthase (c14), and speculated that because photosynthetic organisms have access to readily available sunlight as an energy source, there may have been less evolutionary selection pressure to maximize the thermodynamic efficiency for ATP synthesis compared to organisms that rely on more scarce energy sources (fixed organic molecules). However, it is well known that photosynthetic organisms have adapted to grow in light-limiting conditions (Judd et al., 1964; Stomp et al., 2007; Scanlan et al., 2009), including low light requiring cyanobacteria which have been shown to also have large c-rings (Pogoryelov et al., 2007).
Here, we consider alternative reasons for why photosyntehtic organisms have evolved larger c-rings. As discussed elsewhere (Takizawa et al., 2007; Strand and Kramer, 2014), acidifying the thylakoid lumen can lead to pH–mediated downregulation of photosynthesis at least in plants and algae, or damage to photosynthetic components (Kramer et al., 1999), and robust mechanisms for maintaining pH homeostasis have evolved to maintain pmf predominantly as Δψ across other bioenergetic membranes (Kashket, 1981; Booth, 1985; Moore et al., 1985). Our previous work, however, showed that high Δψ can have deleterious effects on photosynthetic machinery (Davis et al., 2016). We therefore propose that a high H+/ATP stoichiometry was selected for because it allows photosynthesis to occur at high pmf while maintaining low Δψ and low ΔpH, thus preventing deleterious side reactions.
Methods
Computational Kinetic Simulations of Photosynthetic Light Reactions with Altered c–Ring Sizes
To explore the impact of ATP synthase c–ring stoichiometry, the photosynthetic light reactions were modelled using a previously published model for the basic photosynthetic light reactions of C3 plants (Davis et al., 2017). Briefly, the model includes ordinary differential equations (ODE) with defined rate constants for electron and proton transfer reactions for the light reactions of photosynthesis, including those that generate and affect the thylakoid pmf, as well as the biophysical properties of the thylakoid membrane, and the impacts of pmf storage as Δψ and ΔpH on the rate of electron transfer via regulation of b6f turnover, activation of the qE component of NPQ, and the influence of Δψ on PSII electron recombination. The code allows simulations over time during different conditions. The underlying code and expanded descriptions for all ODE can be found online at Github (https://github.com/protonzilla/Delta_Psi_Py). As the c–ring stoichiometry does not appear to change within an organism (Ballhausen et al., 2009), the simulations treat the H+/ATP ratio of the ATP synthase as constant for a given simulation, but can be changed between simulations. To investigate how the c–ring architecture impacts the light reactions, only the H+/ATP ratio was altered as a constant throughout each simulation. The resulting simulations are available as Supplementary Data Sheet 1, as well as an interactive Jupyter notebook available online at Github in which the simulations can be recreated.
Results and Discussion
Small c–Ring Architecture Limits Proton Motive Force Storage and Composition
Figure 2 shows outputs of our kinetic/thermodynamic simulations under conditions where the ATP synthase is active, but with no or very low net changes in proton flux, so that pmf approached equilibrium with ΔGATP over the course of the simulations. Physiologically this condition should occur at very low light, or when the chloroplast is placed in darkness but the ATP synthase regulatory thiols have not yet become fully oxidized. Thus, these estimates represent the minimum pmf needed to sustain ΔGATP.
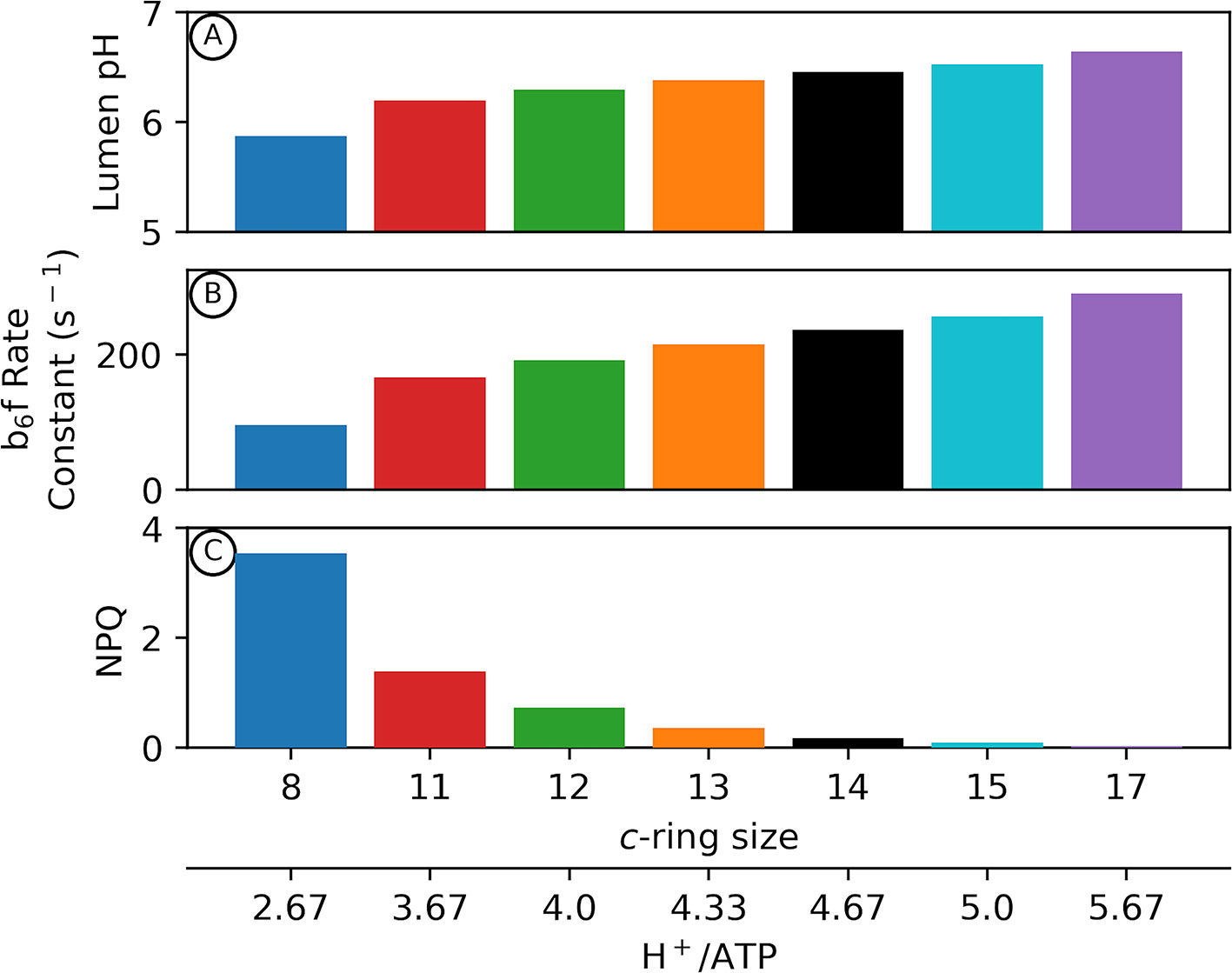
Figure 2 Adenosine triphosphate (ATP) synthase c–ring size impacts photosynthetic physiology in the dark. Kinetic modelling of photosynthetic light reactions with altered ATP synthase c subunit stoichiometry. Simulated responses of the light reactions were performed as in Davis et al., 2017, with all standard conditions held constant except for the number of ATP synthase c subunits. The pmf required to maintain equilibrium with ΔGATP in the dark is variable depending upon the number of c subunits in the ATP synthase c–ring (Eq. 2). Changes in lumen pH in the dark due to alterations in c–ring size (A) can decrease cytochrome b6f turnover rate (B) as well as activate pH–dependent nonphotochemical quenching (NPQ) in higher plants (C).
Assuming a ΔGATP of 40 kJ/mol (Giersch et al., 1980), a decrease in the number of c subunits from 14 (chloroplast) (Seelert et al., 2000) to 8 (Bos taurus mitochondria) (Watt et al., 2010) results in an increase in the pmf required to maintain ΔGATP equilibrium in the dark from ~89 to ~155 mV (Eq. 2). If the fraction of pmf is equally partitioned between Δψ and ΔpH, this results in a ΔpH at ΔGATP of about 0.75 units with a c14 ring (Eq. 1). Assuming stromal pH of 7.8, the lumen pH should reach about 7, where the violaxanthin de-epoxidase (VDE) and the PsbS protein are inactive, and the b6f complex is fully active (Takizawa et al., 2007), thus allowing for maximal photosynthetic efficiency at low light (Figure 2). By contrast, with a c8 ATP synthase, ΔpH at ΔGATP should reach 1.3 units and a lumen pH of 6.5, which is sufficiently acidic to activate VDE and protonate PsbS, thus activating qE, while slowing electron flow through the b6f complex, even in the dark (Figures 2B, C). Small c-rings have even more severe lumen pH-related effects if pmf is stored predominantly in ΔpH, as previously discussed (Kramer et al., 1999; Kramer et al., 2003).
Similarly, the Δψ required just to maintain ΔGATP equilibrium in the dark increases by ~33 mV when going from c14 to c8 if pmf was equally partitioned with ΔpH. During photosynthesis, pmf is held out of equilibrium from ΔGATP (see below) so that light-induced pmf generation should exacerbate these increases (Figure 3). Under this hypothetical smaller c8 operating structure, photosynthetic pmf would either need to be limited to a lower total pmf than its current c14 state, or require a dramatic shift in pmf partitioning into Δψ to avoid near immediate over-acidification of the thylakoid lumen below ~5.5 (ΔpH 2.3 units assuming stromal pH 7.8 in the light) (Kramer et al., 1999), or the evolution of a less pH-sensitive oxygen evolving complex (Krieger and Weis, 1993). A shift in partitioning in favor of Δψ could occur via genetic regulation of counter-ion movement through ion transport expression (Davis et al., 2017), or an increase in the buffering capacity of the lumen, though this might require massive remodelling of thylakoids, or the use of high concentrations of mobile buffering groups such as polyamines (Ioannidis et al., 2012).
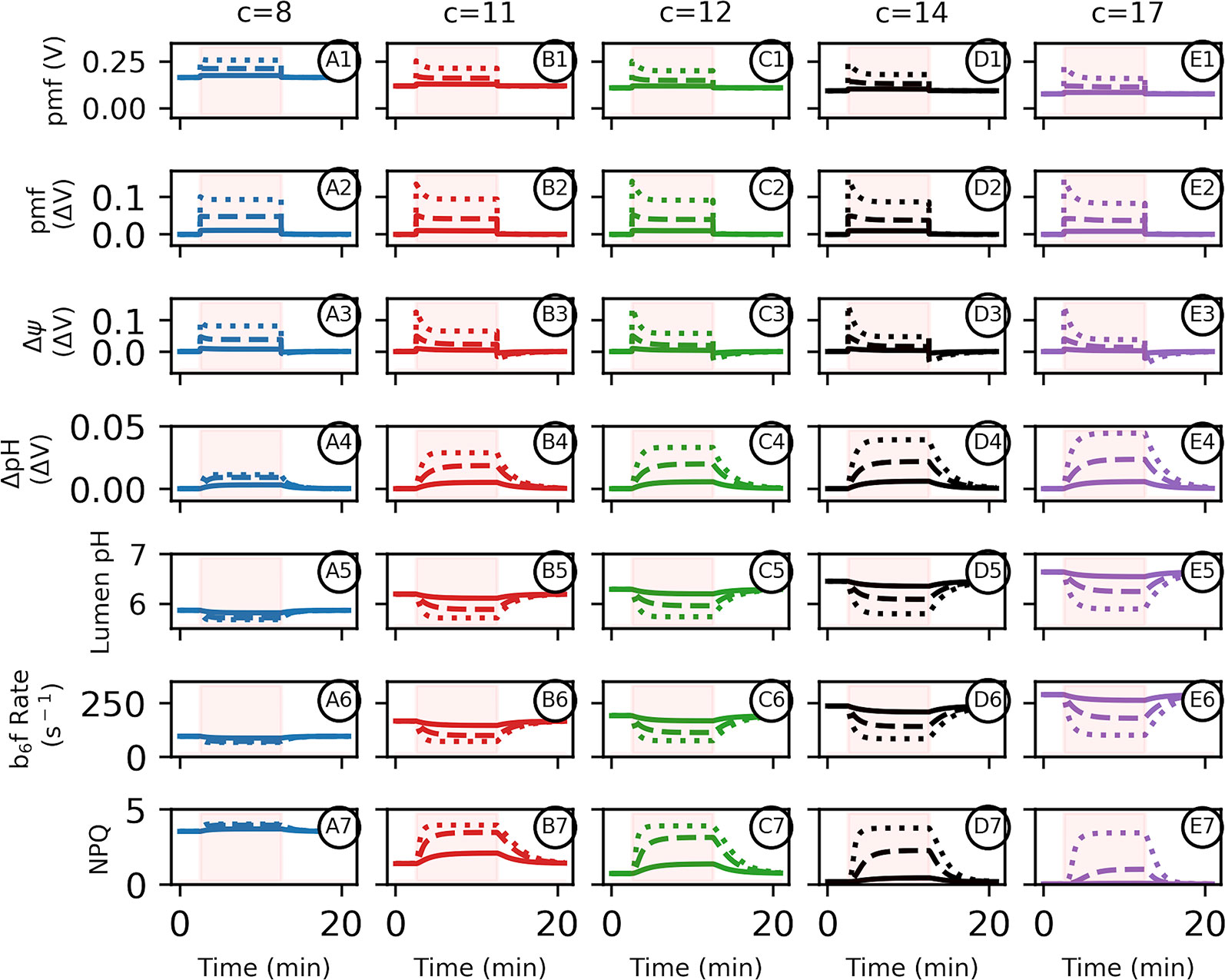
Figure 3 Altered adenosine triphosphate (ATP) synthase c-subunit stoichiometry limits proton motive force (pmf) composition and pH–mediated regulatory processes during photosynthesis. Simulated responses of the light reactions were performed as in Davis et al., 2017, with all standard conditions held constant except for the number of ATP synthase c-subunits. Simulations were performed using 10 min of static light at either 20 (solid lines), 100 (dashed lines), or 1,000 (dotted lines) μmol photons m−2s−1. Intervals of light excitation are indicated by shaded regions. (Panels 1–4) The light–induced pmf (1, 2A–D) of ATP synthases with c–stoichiometries of 8 (blue, column A), 11 (red, column B), 12 (green, column C), 14 (black, column D), or 17 (purple, column E) are shown in units of volts, so that a ΔpH of one is equivalent to 0.06 V. The total pmf (panel 2), Δψ (panel 3), and ΔpH (panel 4) are shown as light–induced changes relative to the pmf dark values indicated as ΔV from dark values, to emphasize light–induced ATP synthase constraints. (5) Light–induced changes in lumen pH due to photosynthetic activity. Light intensities and c–ring composition as in (1). (6) The relative rate constant for plastoquinol oxidation at the cytochrome b6f complex and (7) the extent of nonphotochemical quenching qE component for each c–ring size due to the light–induced changes in lumen pH.
While the kinetic/thermodynamic model used to analyse changes in c stoichiometries was based upon higher plant light chloroplasts, it should be noted that in cyanobacteria, where photosynthetic and respiratory electron transport share the same membrane and quinone pool (Mullineaux, 2014), the c subunit stoichiometry and pmf partitioning will impact electron transfer within both processes due to the shared role of b6f (Vermaas, 2001), though the pmf-dependencies of b6f turnover, OEC stability, or PSII recombination reactions have not been well explored in these species. However, as discussed above, cyanobacterial thylakoid ATP synthase c–rings are similar in size to chloroplast c–rings rather than bacterial respiratory rings (e.g., E. coli), possibly indicating that a large c–ring, with its corresponding limitations and advantages, while not necessarily optimized for respiration is less unfavorable to respiratory electron transfer than a small c–ring is unfavourable to photosynthetic electron transfer.
We next considered the effects of light activation of electron flow (Figure 3). With sufficiently high ATP synthase activity, it should be possible to maintain near equilibrium between pmf and ΔGATP even during photosynthesis. In vivo, however, the chloroplast ATP synthase activity is not sufficient to allow equilibration to occur, even under ideal conditions, and further down-regulation of control of ATP synthase activity under adverse conditions results in substantial disequilibrium with ΔGATP (Buchanan, 1980; Kanazawa and Kramer, 2002). This disequilibrium may have evolved to control lumen pH to activate photosynthetic control and qE (Kanazawa and Kramer, 2002; Avenson et al., 2004) while maintaining ATP homeostasis, or to maintain photosynthetic control by imposing rate limitations at the b6f complex. It could also represent a fundamental limitation in the kinetic properties of the ATP synthase, though in this case, it is not clear why this limitation could be overcome by over-expressing the complex. In the current simulations, we set ATP synthase activity constant at the highest levels we have observed in vivo based on analyses of the decay of the electrochromic shift in the dark (Zaks et al., 2012; Davis et al., 2017).
While the dark, initial pmf, which is set be in equilibrium with ΔGATP, increases with smaller c–rings (Figures 2 and 3), the total light-induced pmf (Figure 3 panel 2) is strongly limited to about the same extents regardless of the c–ring stoichiometry due to lumen pH–mediated photosynthetic control (Figure 3 panel 5) and qE. Therefore, the total light–induced pmf progressively decreases with smaller c–rings (Figure 3 panels 2–4 ). Thus, the only way to increase total pmf storage in small c-rings is to store a higher fraction as Δψ (Figure 3 panel 3). The light–induced pmf challenge is present at both low and high light intensities, with larger c–rings being preferential to photosynthetic electron transfer under all intensities. This is clearly seen in the rates of turnover of the b6f complex (Figure 3 panel 6). With larger c-rings, the lumen pH is above the pKa for PQH2 oxidation at low light, so photosynthetic control is low, but at high light, lumen acidification increases ΔpH and the turnover rate of the b6f complex decreases. In smaller c-rings, the lumen pH is initially sufficiently acidic that photosynthetic control is large even at low light, limiting electron flow and further acidification. Thus, variable photosynthetic control is lost with the smaller c-rings. Note that because PQH2 oxidation is the rate-limiting step in linear electron flow, b6f turnover can be estimated by the re-reduction kinetics of P700+. Given that ΔpH with smaller c-rings is saturated, we expect little effect of changing downstream reactions on photosynthetic control.
However, higher Δψ increases the rates of recombination reactions in PSII and 1O2 production (Figure 4) (Davis et al., 2016). The PSII recombination rate depends on the concentration of charge–separated states capable of recombining as well as the energetics of electron sharing between redox intermediates, with the rate changing exponentially with Δψ. For the charge-separated state(s) forming P+QA−, where P+ is the primary electron donor and QA the non-mobile PSII quinone, these changes correspond to:
where [S2QA− + S3QA−] represents the fraction of PSII containing donor and acceptor side states capable of recombining from QA−, kr is the intrinsic rate of recombination from S2/S3QA− with no Δψ, ΔEstab is the stabilization free energy of the charge-separated state S2/S3QA− expressed in eV, and f the distance between the charge-separated states normal to the membrane surface (Davis et al., 2016). As Δψ increases, the ΔEstab of charge separated states decreases (de Grooth and van Gorkom, 1981; Vos et al., 1991), leading to an increase in the rate of recombination. Therefore, even with relatively small changes in the amount of energy stored as Δψ, the velocity of recombination will increase dramatically. This likely limits the amount of energy that can be stored safely across photosynthetic membranes as Δψ, as electron recombination through back–reactions can lead to generation of ROS (Rutherford et al., 2012; Davis et al., 2016).
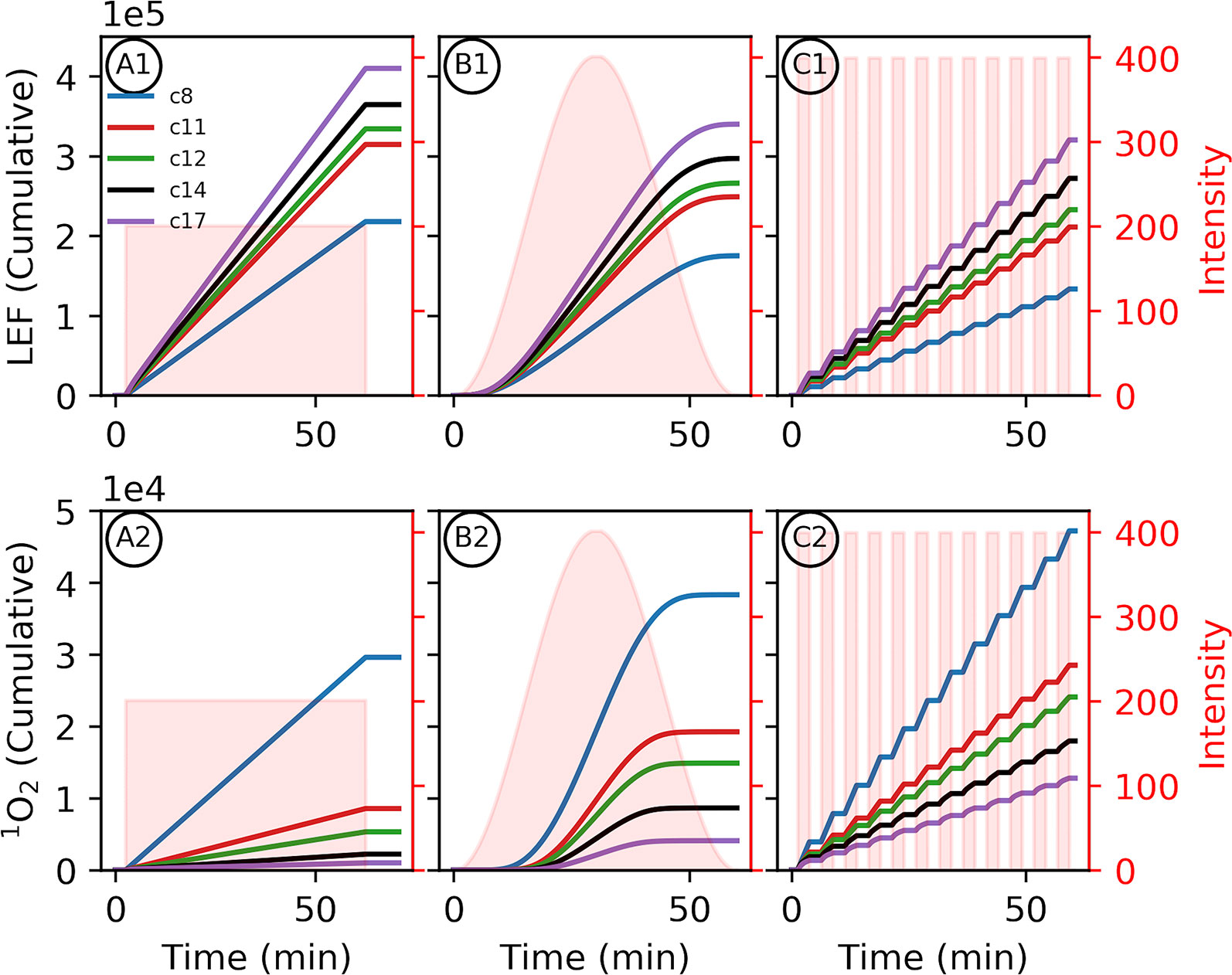
Figure 4 Altered proton motive force (pmf) composition due to c-subunit stoichiometry limits photosynthetic productivity. Simulated responses of the light reactions were performed as in Figure 3. Variability in environment was simulated with 1-h light profiles of static light (A1, 2), sinusoidal light (B1, 2), or square wave fluctuating light (C1, 2) to provide the same total illumination during the simulation. (1) The total outputs for linear electron flow (LEF) over the course of the light simulations and (2) 1O2 were integrated over the light treatment to give the cumulative totals. Shaded regions indicate the light profiles for each simulation.
As seen in Figure 3, relative to larger stoichiometries, the increased Δψ in smaller c–rings (Figure 3 panel 3) is expected to increase the probability of PSII recombination reactions and 1O2 generation. This small c–ring pitfall is exacerbated by the constitutive downregulation of electron transfer due to the lower lumen pH, limiting productive LEF and resulting in a more reduced quinone pool, which provides substrate for PSII recombination (Supplementary Figures 1–8). Dynamic light intensities (Figure 4), as would be expected in many natural environments, can induce large spikes in Δψ (Cruz et al., 2001; Davis et al., 2016) that can exacerbate PSII recombination-induced 1O2 production, and our simulations suggest that this effect is worsened as c subunit stoichiometry decreases. Simulations of one hour of illumination at light intensities of various dynamics but equal total photon flux, recapitulate the increased production of 1O2 with increasing intensity of light fluctuations (Figure 4 panel 2). Smaller c–rings, as with static light, produce even more 1O2 than the photosynthetic c14 with progressively more with more realistic, fluctuating light dynamics (Figure 4C). Coupled with the potential cellular damage from 1O2, small c–rings, even with higher light intensities than static light, result in progressively less LEF over the course of illumination in dynamic conditions due to premature downregulation of quinol oxidation relative to large stoichiometries (Figure 4 panel 1).
It therefore appears that a large c–ring stoichiometry, although arguably energetically inefficient in isolation (von Ballmoos et al., 2008; Silverstein, 2014), may in fact be far more physiologically efficient for photoautotrophic maintenance and organismal survival than a smaller stoichiometry. Additionally, the decrease in the chloroplast ATP synthase pmf activation threshold (Kaim and Dimroth, 1999) may have led to the additional advantage of photosynthetic ATP generation under even low light and low pmf conditions (Kramer and Crofts, 1989).
Are Large c-Rings an Evolutionary Adaptation to Utilize Large ΔpH and Synthesize Adenosine Triphosphate When Proton Motive Force Is Small?
Based on the arguments above for phototrophs, one might also expect to find higher c-stoichiometries in other organisms where pmf is constrained by similar needs to maintain physiologically permissive conditions. Interestingly, of the ATP synthase c-rings analyzed to date, the other group outside of oxygenic photoautotrophs that utilize larger (c13) rings are alkaliphilic bacteria (Meier et al., 2007; Preiss et al., 2010). Due to their high pH growth conditions, these organisms are subjected to an “inverted” ΔpH, acidic inside the cell relative to outside, and must generate a high Δψ in order to allow H+ driven ATP synthesis (Sturr et al., 1994; Olsson et al., 2003; Hicks et al., 2010). However, the inverted ΔpH works against total pmf, so that the operating pmf is lower than that in neutrophilic bacteria, possibly because there is a thermodynamic or structural limit to the amplitude of Δψ needed to counterbalance the negative ΔpH. Thus, a larger c-ring may be necessary to maintain sufficient pmf to overcome ΔGATP, which could explain the growth defects found in the alkaliphilic Bacillus pseudofirmus OF4 with engineered smaller c-rings (Liu et al., 2011; Preiss et al., 2013).
More examples of c-ring architecture combined with pmf [or sodium motive force (smf)] measurements could resolve why some organisms have evolved to use larger, theoretically less efficient H+/ATP ratios. The monophyly of oxygenic photosynthesis in a single group of bacteria (cyanobacteria) may have initiated c–ring constraints in chloroplasts. The pmf composition and dynamics in cyanobacteria has not yet been well studied, whereas an electrochromic shift of carotenoid pigments in the thylakoid membranes of eukaryotes (Bailleul et al., 2010), has allowed focused studies primarily in the green lineage (Bailleul et al., 2015). The recent report of a useable electrochromic shift in a cyanobacterium should make such studies possible (Viola et al., 2019).
While we emphasize the larger effects of changing c subunit stoichiometry by large amounts, but as expected, the effects of smaller changes on pmf are incremental, and thus we expect there to be subtler trade-offs. However, even small tradeoffs are expected to be important over evolutionary time scales. One additional, and interesting, tradeoff is that a c12 ring would be expected to balance the ATP/NADPH production by LEF with consumption by the CBB cycle, perhaps obviating the need for cyclic electron flow under some conditions. However, the overall ATP/NADPH demand is dynamic and should depend on which metabolic sinks are engaged (Heber, 1974; Krause and Heber, 1976; Edwards and Walker, 1983; Fernyhough et al., 1983; Kobayashi et al., 1995; Kramer and Evans, 2011), and thus energy balancing mechanisms should still be required. Making the rings are smaller than 12 subunits would introduce a different problem: an excess of ATP/NADPH production relative to consumption by the CBB cycle; interestingly, there are no established mechanisms to ameliorate this kind of imbalance (Kanazawa et al., 2020). This issue is more apparent (and acute) in cyanobacteria, which use dissipative oxygen reduction pathways to protect reactions centers from photodamage, but as a consequence introduce ATP/NADPH imbalances. Thus, a greater understanding of the pmf and ATP synthase architecture in cyanobacteria will enhance the understanding of bioenergetic interactions between photosynthetic electron transfer and ATP production. This understanding is crucial for any future synthetic biology approaches to alter photosynthetic ATP production either directly via the ATP synthase (Cardona et al., 2018), or indirectly via the H+/e− ratio of ATP/NADPH. These limitations and trade-offs, we predict, will likely hinder any gains in photosynthetic efficiency afforded by engineering smaller c–rings unless radical changes to the rest of photosynthesis are also made.
Data Availability Statement
The datasets generated for this study can be found on github (https://github.com/protonzilla/Delta_Psi_Py) as a detailed Jupyter (www.jupyter.org) notebook.
Author Contributions
GD and DK contributed to the design of simulations and interpretation of results. GD and DK contributed to the drafting and revising of the article.
Funding
Funding for GD and DK was supported by the US Department of Energy (DOE), Office of Basic Science, Basic Energy Sciences (BES) under award number DE-FG02-91ER20021.
Conflict of Interest
The authors declare that the research was conducted in the absence of any commercial or financial relationships that could be construed as a potential conflict of interest.
Supplementary Material
The Supplementary Material for this article can be found online at: https://www.frontiersin.org/articles/10.3389/fpls.2019.01778/full#supplementary-material
Supplementary Figure 1 | Comparison of pmf composition and pH–mediated regulatory processes during photosynthesis in naturally occurring photosynthetic c-rings and c12 ring to balance ATP/NADPH with carbon assimilation. Simulated responses of the light reactions were performed as in Davis et al., 2017, with all standard conditions held constant except for the number of ATP synthase c-subunits. Simulations were performed using 10 minutes of static light at either 20 (solid lines), 100 (dashed lines), or 1000 (dotted lines) μmol photons m-2s-1 as in Figure 1. Intervals of light excitation are indicated by shaded regions. (Panels 1-4) The light–induced pmf (1, 2A-D) of ATP synthases with c–stoichiometries of 12 (green, column A), 13 (orange, column B), 14 (black, column C), or 15 (cyan, column D) are shown in units of volts, so that a ΔpH of one is equivalent to 0.06 V. The total pmf (panel 2), Δψ (panel 3), and ΔpH (panel 4) are shown as light–induced changes relative to the pmf dark values indicated as ΔV from dark values, to emphasize light–induced ATP synthase constraints. (5) Light–induced changes in lumen pH due to photosynthetic activity. Light intensities and c–ring composition as in (1). (6) The relative rate constant for plastoquinol oxidation at the cytochrome b6f complex and (7) the extent of nonphotochemical quenching qE component for each c–ring size due to the light–induced changes in lumen pH.
Supplementary Figure 2 | Altered ATP synthase c8 stoichiometry impacts pmf composition and pH–mediated regulatory processes during photosynthesis under increasingly dynamic light environments. Simulated responses of the light reactions were performed as in Davis et al., 2017, with all standard conditions held constant except for the number of ATP synthase c-subunits. Simulations were performed using 1-hour of either static light (A), sinusoidal light (B), or square wave fluctuating light (C) with equal total photon flux over the total duration of each light treatment. Intervals of light excitation are indicated by shaded regions. (Panels 1-4) The light–induced pmf of ATP synthases with c–stoichiometries of 8 (blue) or 14 (black) are shown in units of volts, so that a ΔpH of one is equivalent to 0.06 V. The total pmf (panel 2), Δψ (panel 3), and ΔpH (panel 4) are shown as light–induced changes relative to the pmf dark values indicated as ΔV from dark values, to emphasize light–induced ATP synthase constraints. (5) Light–induced changes in lumen pH due to photosynthetic activity. Light intensities and c–ring composition as in (1). (6) The relative rate constant for plastoquinol oxidation at the cytochrome b6f complex and (7) the extent of nonphotochemical quenching qE component for each c–ring size due to the light–induced changes in lumen pH.
Supplementary Figure 3 | Altered ATP synthase c11 stoichiometry impacts pmf composition and pH–mediated regulatory processes during photosynthesis under increasingly dynamic light environments. Simulated responses of the light reactions were performed as in Davis et al., 2017, with all standard conditions held constant except for the number of ATP synthase c-subunits. Simulations were performed using 1-hour of either static light (A), sinusoidal light (B), or square wave fluctuating light (C) with equal total photon flux over the total duration of each light treatment. Intervals of light excitation are indicated by shaded regions. (Panels 1-4) The light–induced pmf of ATP synthases with c–stoichiometries of 11 (red) or 14 (black) are shown in units of volts, so that a ΔpH of one is equivalent to 0.06 V. The total pmf (panel 2), Δψ (panel 3), and ΔpH (panel 4) are shown as light–induced changes relative to the pmf dark values indicated as ΔV from dark values, to emphasize light–induced ATP synthase constraints. (5) Light–induced changes in lumen pH due to photosynthetic activity. Light intensities and c–ring composition as in (1). (6) The relative rate constant for plastoquinol oxidation at the cytochrome b6f complex and (7) the extent of nonphotochemical quenching qE component for each c–ring size due to the light–induced changes in lumen pH.
Supplementary Figure 4 | Altered ATP synthase c12 stoichiometry impacts pmf composition and pH–mediated regulatory processes during photosynthesis under increasingly dynamic light environments. Simulated responses of the light reactions were performed as in Davis et al., 2017, with all standard conditions held constant except for the number of ATP synthase c-subunits. Simulations were performed using 1-hour of either static light (A), sinusoidal light (B), or square wave fluctuating light (C) with equal total photon flux over the total duration of each light treatment. Intervals of light excitation are indicated by shaded regions. (Panels 1-4) The light–induced pmf of ATP synthases with c–stoichiometries of 12 (green) or 14 (black) are shown in units of volts, so that a ΔpH of one is equivalent to 0.06 V. The total pmf (panel 2), Δψ (panel 3), and ΔpH (panel 4) are shown as light–induced changes relative to the pmf dark values indicated as ΔV from dark values, to emphasize light–induced ATP synthase constraints. (5) Light–induced changes in lumen pH due to photosynthetic activity. Light intensities and c–ring composition as in (1). (6) The relative rate constant for plastoquinol oxidation at the cytochrome b6f complex and (7) the extent of nonphotochemical quenching qE component for each c–ring size due to the light–induced changes in lumen pH.
Supplementary Figure 5 | Altered ATP synthase c13 stoichiometry impacts pmf composition and pH–mediated regulatory processes during photosynthesis under increasingly dynamic light environments. Simulated responses of the light reactions were performed as in Davis et al., 2017, with all standard conditions held constant except for the number of ATP synthase c-subunits. Simulations were performed using 1-hour of either static light (A), sinusoidal light (B), or square wave fluctuating light (C) with equal total photon flux over the total duration of each light treatment. Intervals of light excitation are indicated by shaded regions. (Panels 1-4) The light–induced pmf of ATP synthases with c–stoichiometries of 13 (orange) or 14 (black) are shown in units of volts, so that a ΔpH of one is equivalent to 0.06 V. The total pmf (panel 2), Δψ (panel 3), and ΔpH (panel 4) are shown as light–induced changes relative to the pmf dark values indicated as ΔV from dark values, to emphasize light–induced ATP synthase constraints. (5) Light–induced changes in lumen pH due to photosynthetic activity. Light intensities and c–ring composition as in (1). (6) The relative rate constant for plastoquinol oxidation at the cytochrome b6f complex and (7) the extent of nonphotochemical quenching qE component for each c–ring size due to the light–induced changes in lumen pH.
Supplementary Figure 6 | Altered ATP synthase c15 stoichiometry impacts pmf composition and pH–mediated regulatory processes during photosynthesis under increasingly dynamic light environments. Simulated responses of the light reactions were performed as in Davis et al., 2017, with all standard conditions held constant except for the number of ATP synthase c-subunits. Simulations were performed using 1-hour of either static light (A), sinusoidal light (B), or square wave fluctuating light (C) with equal total photon flux over the total duration of each light treatment. Intervals of light excitation are indicated by shaded regions. (Panels 1-4) The light–induced pmf of ATP synthases with c–stoichiometries of 15 (cyan) or 14 (black) are shown in units of volts, so that a ΔpH of one is equivalent to 0.06 V. The total pmf (panel 2), Δψ (panel 3), and ΔpH (panel 4) are shown as light–induced changes relative to the pmf dark values indicated as ΔV from dark values, to emphasize light–induced ATP synthase constraints. (5) Light–induced changes in lumen pH due to photosynthetic activity. Light intensities and c–ring composition as in (1). (6) The relative rate constant for plastoquinol oxidation at the cytochrome b6f complex and (7) the extent of nonphotochemical quenching qE component for each c–ring size due to the light–induced changes in lumen pH.
Supplementary Figure 7 | Altered ATP synthase c17 stoichiometry impacts pmf composition and pH–mediated regulatory processes during photosynthesis under increasingly dynamic light environments. Simulated responses of the light reactions were performed as in Davis et al., 2017, with all standard conditions held constant except for the number of ATP synthase c-subunits. Simulations were performed using 1-hour of either static light (A), sinusoidal light (B), or square wave fluctuating light (C) with equal total photon flux over the total duration of each light treatment. Intervals of light excitation are indicated by shaded regions. (Panels 1-4) The light–induced pmf of ATP synthases with c–stoichiometries of 17 (purple) or 14 (black) are shown in units of volts, so that a ΔpH of one is equivalent to 0.06 V. The total pmf (panel 2), Δψ (panel 3), and ΔpH (panel 4) are shown as light–induced changes relative to the pmf dark values indicated as ΔV from dark values, to emphasize light–induced ATP synthase constraints. (5) Light–induced changes in lumen pH due to photosynthetic activity. Light intensities and c–ring composition as in (1). (6) The relative rate constant for plastoquinol oxidation at the cytochrome b6f complex and (7) the extent of nonphotochemical quenching qE component for each c–ring size due to the light–induced changes in lumen pH.
Supplementary Figure 8 | Altered pmf composition due to c-subunit stoichiometry limits photosynthetic productivity. Simulated responses of the light reactions were performed as in figures 3 and 4. Variability in environment was simulated with 1-hour light profiles of static light (A1, 2), sinusoidal light (B1, 2), or square wave fluctuating light (C1, 2) to provide the same total illumination during the simulation. (1) The total outputs for linear electron flow (LEF) over the course of the light simulations and (2) 1O2 were integrated over the light treatment to give the cumulative totals. Shaded regions indicate the light profiles for each simulation.
References
Allen, J. F. (2003). Cyclic, pseudocyclic and noncyclic photophosphorylation: new links in the chain. Trends Plant Sci. 8, 15–19. doi: 10.1016/s1360-1385(02)00006-7
Arechaga, I., Butler, P. J. G., Walker, J. E. (2002). Self-assembly of ATP synthase subunit c rings. FEBS Lett. 515, 189–193. doi: 10.1016/S0014-5793(02)02447-X
Armbruster, U., Carrillo, L. R., Venema, K., Pavlovic, L., Schmidtmann, E., Kornfeld, A., et al. (2014). Ion antiport accelerates photosynthetic acclimation in fluctuating light environments. Nat. Commun. 5, 5439. doi: 10.1038/ncomms6439
Asada, K. (1999). THE water-water cycle in chloroplasts: scavenging of active oxygens and dissipation of excess photons. Annu. Rev. Plant Physiol. Plant Mol. Biol. 50, 601–639. doi: 10.1146/annurev.arplant.50.1.601
Avenson, T. J., Cruz, J. A., Kramer, D. M. (2004). Modulation of energy-dependent quenching of excitons in antennae of higher plants. Proc. Natl. Acad. Sci. U.S.A. 101, 5530–5535. doi: 10.1073/pnas.0401269101
Bailleul, B., Cardol, P., Breyton, C., Finazzi, G. (2010). Electrochromism: a useful probe to study algal photosynthesis. Photosynth. Res. 106, 179–189. doi: 10.1007/s11120-010-9579-z
Bailleul, B., Berne, N., Murik, O., Petroutsos, D., Prihoda, J., Tanaka, A., et al. (2015). Energetic coupling between plastids and mitochondria drives CO2 assimilation in diatoms. Nature 524, 366–369. doi: 10.1038/nature14599
Ballhausen, B., Altendorf, K., Deckers-Hebestreit, G. (2009). Constant c10 ring stoichiometry in the Escherichia coli ATP synthase analyzed by cross-linking. J. Bacteriol. 191, 2400–2404. doi: 10.1128/JB.01390-08
Boyer, P. D. (1997). The ATP synthase–a splendid molecular machine. Annu. Rev. Biochem. 66, 717–749. doi: 10.1146/annurev.biochem.66.1.717
Buchanan, B. B. (1980). Role of light in the regulation of chloroplast enzymes. Annu. Rev. Plant Physiol. Plant Mol. Biol. 31, 341–374. doi: 10.1146/annurev.pp.31.060180.002013
Cardona, T., Shao, S., Nixon, P. J. (2018). Enhancing photosynthesis in plants: the light reactions. Essays Biochem. 62, 85–94. doi: 10.1042/EBC20170015
Cardona, T. (2015). A fresh look at the evolution and diversification of photochemical reaction centers. Photosynth. Res. 126, 111–134. doi: 10.1007/s11120-014-0065-x
Checchetto, V., Segalla, A., Allorent, G., La Rocca, N., Leanza, L., Giacometti, G. M., et al. (2012). Thylakoid potassium channel is required for efficient photosynthesis in cyanobacteria. Proc. Natl. Acad. Sci. U. S. A. 109, 11043–11048. doi: 10.1073/pnas.1205960109
Crofts, A. R., Wraight, C. A., Fleischmann, D. E. (1971). Energy conservation in photochemical reactions of photosynthesis and its relation to delayed fluorescence. FEBS Lett. 15, 89–100. doi: 10.1016/0014-5793(71)80031-5
Cruz, J. A., Sacksteder, C. A., Kanazawa, A., Kramer, D. M. (2001). Contribution of electric field (Δψ) to steady-state transthylakoid proton motive force (pmf) in vitro and in vivo. Control of pmf parsing into Δψ and ΔpH by ionic strength. Biochemistry 40, 1226–1237. doi: 10.1021/bi0018741
Cruz, J. A., Avenson, T. J., Kanazawa, A., Takizawa, K., Edwards, G. E., Kramer, D. M. (2005a). Plasticity in light reactions of photosynthesis for energy production and photoprotection. J. Exp. Bot. 56, 395–406. doi: 10.1093/jxb/eri022
Cruz, J. A., Kanazawa, A., Treff, N., Kramer, D. M. (2005b). Storage of light-driven transthylakoid proton motive force as an electric field (Δψ) under steady-state conditions in intact cells of Chlamydomonas reinhardtii. Photosynth. Res. 85, 221–233. doi: 10.1007/s11120-005-4731-x
Davis, G. A., Kanazawa, A., Schottler, M. A., Kohzuma, K., Froehlich, J. E., Rutherford, A. W., et al. (2016). Limitations to photosynthesis by proton motive force-induced photosystem II photodamage. Elife 5. doi: 10.7554/eLife.16921
Davis, G. A., Rutherford, A. W., Kramer, D. M. (2017). Hacking the thylakoid proton motive force for improved photosynthesis: modulating ion flux rates that control proton motive force partitioning into Δψ and ΔpH. Philos. Trans. R. Soc. L. B. Biol. Sci. 372. doi: 10.1098/rstb.2016.0381
de Grooth, B. G., van Gorkom, H. J. (1981). External electric field effects on prompt and delayed fluorescence in chloroplasts. Biochim. Biophys. Acta 635, 445–456. doi: 10.1016/0005-2728(81)90104-3
Duan, Z., Kong, F., Zhang, L., Li, W., Zhang, J., Peng, L. (2016). A bestrophin-like protein modulates the proton motive force across the thylakoid membrane in Arabidopsis. J. Integr. Plant Biol. 58, 848–858. doi: 10.1111/jipb.12475
Edwards, G., Walker, D. A. (1983). C3, C4: Mechanisms, and cellular and environmental regulation, of photosynthesis (Oxford, UK: Blackwell Scientific Publications).
Feniouk, B. A., Kozlova, M. A., Knorre, D. A., Cherepanov, D. A., Mulkidjanian, A. Y., Junge, W. (2004). The proton-driven rotor of ATP synthase: ohmic conductance (10 fS), and absence of voltage gating. Biophys. J. 86, 4094–4109. doi: 10.1529/biophysj.103.036962
Ferguson, S. J. (2000). ATP synthase: what dictates the size of a ring? Curr. Biol. 10, R804–R808. doi: 10.1016/S0960-9822(00)00765-X
Ferguson, S. J. (2010). ATP synthase: from sequence to ring size to the P/O ratio. Proc. Natl. Acad. Sci. U.S.A. 107, 16755–16756. doi: 10.1073/pnas.1012260107
Fernyhough, P., Foyer, C., Horton, P. (1983). The influence of metabolic state on the level of phosphorylation of the light-harvesting chlorophyll-protein complex in chloroplasts isolated from maize mesophyll. Biochim. Biophys. Acta 725, 155–161. doi: 10.1016/0005-2728(83)90235-9
Giersch, C., Heber, U., Kobayashi, Y., Inoue, Y., Shibata, K., Heldt, H. W. (1980). Energy charge, phosphorylation potential and proton motive force in chloroplasts. Biochim. Biophys. Acta 590, 59–73. doi: 10.1016/0005-2728(80)90146-2
Gräber, P., Junesch, U., Schatz, G. H. (1984). Kinetics of proton-transport-coupled ATP-synthesis in chloroplasts. Activation of the ATPase by an artificially generated ΔpH and Δψ. Berichte Der. Bunsen-Gesellschaft-Physical Chem. Chem. Phys. 88, 599–608. doi: 10.1002/bbpc.19840880706
Hangarter, R. P., Good, N. E. (1982). Energy thresholds for ATP synthesis in chloroplasts. Biochim. Biophys. Acta 681, 397–404. doi: 10.1016/0005-2728(82)90181-5
Hasan, S. S., Cramer, W. A. (2012). On rate limitations of electron transfer in the photosynthetic cytochrome b6f complex. Phys. Chem. Chem. Phys. 14, 13853–13860. doi: 10.1039/c2cp41386h
Heber, U. (1974). Metabolite exchange between chloroplasts and cytoplasm. Annu. Rev. Plant Physiol. Plant Mol. Biol. 25, 393–421. doi: 10.1146/annurev.pp.25.060174.002141
Herdean, A., Nziengui, H., Zsiros, O., Solymosi, K., Garab, G., Lundin, B., et al. (2016a). The Arabidopsis thylakoid chloride channel AtCLCe functions in chloride homeostasis and regulation of photosynthetic electron transport. Front. Plant Sci. 7, 115. doi: 10.3389/fpls.2016.00115
Herdean, A., Teardo, E., Nilsson, A. K., Pfeil, B. E., Johansson, O. N., Unnep, R., et al. (2016b). A voltage-dependent chloride channel fine-tunes photosynthesis in plants. Nat. Commun. 7, 11654. doi: 10.1038/ncomms11654
Hicks, D. B., Liu, J., Fujisawa, M., Krulwich, T. A. (2010). F1F0-ATP synthases of alkaliphilic bacteria: lessons from their adaptations. Biochim. Biophys. Acta 1797, 1362–1377. doi: 10.1016/j.bbabio.2010.02.028
Hohner, R., Correa Galvis, V., Strand, D. D., Voelkner, C., Kraemer, M., Messer, M., et al. (2019). Photosynthesis in Arabidopsis thaliana is unaffected by the function of the vacuolar K+ channel TPK3. Plant Physiol. doi: 10.1104/pp.19.00255
Hope, A. B., Valente, P., Matthews, D. B. (1994). Effects of pH on the kinetics of redox reactions in and around the cytochrome bf complex in an isolated system. Photosynth. Res. 42, 111–120. doi: 10.1007/bf02187122
Ioannidis, N. E., Cruz, J. A., Kotzabasis, K., Kramer, D. M. (2012). Evidence that putrescine modulates the higher plant photosynthetic proton circuit. PLoS One 7, e29864. doi: 10.1371/journal.pone.0029864
Judd, D. B., Macadam, D. L., Wyszecki, G. (1964). Spectral distribution of typical daylight as a function of correlated color temperature. J. Opt. Soc. Am. 54, 1031. doi: 10.1364/josa.54.001031
Junesch, U., Graber, P. (1991). The rate of ATP-synthesis as a function of delta pH and delta psi catalyzed by the active, reduced H(+)-ATPase from chloroplasts. FEBS Lett. 294, 275–278. doi: 10.1016/0014-5793(91)81447-G
Junge, W., Nelson, N. (2015). ATP synthase. Annu. Rev. Biochem. 84, 631–657. doi: 10.1146/annurev-biochem-060614-034124
Kaim, G., Dimroth, P. (1999). ATP synthesis by F-type ATP synthase is obligatorily dependent on the transmembrane voltage. EMBO J. 18, 4118–4127. doi: 10.1093/emboj/18.15.4118
Kanazawa, A., Kramer, D. M. (2002). In vivo modulation of nonphotochemical exciton quenching (NPQ) by regulation of the chloroplast ATP synthase. Proc. Natl. Acad. Sci. U.S.A. 99, 12789–12794. doi: 10.1073/pnas.182427499
Kanazawa, A., Ostendorf, E., Kohzuma, K., Hoh, D., Strand, D. D., Sato-Cruz, M., et al. (2017). Chloroplast ATP synthase modulation of the thylakoid proton motive force: implications for photosystem i and photosystem ii photoprotection. Front. Plant Sci. 8, 719. doi: 10.3389/fpls.2017.00719
Kanazawa, A., Davis, G. A., Fisher, N., Neofotis, P., Kramer, D. M. (2020). “Diversity in photoprotection and energy balancing in terrestrial and aquatic phototrophs,” in Photosynthesis in Algae. Eds. Larkum, A. W., Grossman, A. R., Raven, J. A. (Dordrecht: Springer).
Kaplan, J. H., Uribe, E., Jagendorf, A. T. (1967). ATP hydrolysis caused by acid-base transition of spinach chloroplasts. Arch. Biochem. Biophys. 120, 365–370. doi: 10.1016/0003-9861(67)90252-4
Kashket, E. R. (1981). Proton motive force in growing Streptococcus lactis and Staphylococcus aureus cells under aerobic and anaerobic conditions. J. Bacteriol. 146, 369–376. doi: 10.1128/JB.146.1.369-376.1981
Kirilovsky, D., Kerfeld, C. A. (2012). The orange carotenoid protein in photoprotection of photosystem II in cyanobacteria. Biochim. Biophys. Acta 1817, 158–166. doi: 10.1016/j.bbabio.2011.04.013
Kobayashi, Y., Kaiser, W., Heber, U. (1995). Bioenergetics of carbon assimilation in intact chloroplasts: Coupling of proton to electron transport at the ratio H+/e=3 is incompatible with H+/ATP=3 in ATP synthesis. Plant Cell Physiol. 36, 1629–1637. doi: 10.1093/oxfordjournals.pcp.a078930
Koumandou, V. L., Kossida, S. (2014). Evolution of the F0F1 ATP synthase complex in light of the patchy distribution of different bioenergetic pathways across prokaryotes. PLoS Comput. Biol. 10, e1003821. doi: 10.1371/journal.pcbi.1003821
Kramer, D. M., Crofts, A. R. (1989). Activation of the chloroplast ATPase measured by the electrochromic change in leaves of intact plants. Biochim. Biophys. Acta 976, 28–41. doi: 10.1016/s0005-2728(89)80186-0
Kramer, D. M., Evans, J. R. (2011). The importance of energy balance in improving photosynthetic productivity. Plant Physiol. 155, 70–78. doi: 10.1104/pp.110.166652
Kramer, D. M., Sacksteder, C. A., Cruz, J. A. (1999). How acidic is the lumen? Photosynth. Res. 60, 151–163. doi: 10.1023/a:1006212014787
Kramer, D. M., Cruz, J. A., Kanazawa, A. (2003). Balancing the central roles of the thylakoid proton gradient. Trends Plant Sci. 8, 27–32. doi: 10.1016/S1360-1385(02)00010-9
Kramer, D. M., Avenson, T. J., Edwards, G. E. (2004). Dynamic flexibility in the light reactions of photosynthesis governed by both electron and proton transfer reactions. Trends Plant Sci. 9, 349–357. doi: 10.1016/j.tplants.2004.05.001
Krause, G. H., Heber, U. (1976). “Energetics of intact chloroplasts,” in Topics in photosynthesis - Volume 1. The intact chloroplast. Ed. Barber, J. (Amsterdam, Netherlands: Elsevier/North-Holland Biomedical Press, The Netherlands), 171–214.
Krieger, A., Weis, E. (1993). The role of calcium in the pH-dependent control of photosystem II. Photosynth. Res. 37, 117–130. doi: 10.1007/bf02187470
Kuhlbrandt, W. (2019). Structure and Mechanisms of F-Type ATP Synthases. Annu. Rev. Biochem. doi: 10.1146/annurev-biochem-013118-110903
Kunz, H. H., Gierth, M., Herdean, A., Satoh-Cruz, M., Kramer, D. M., Spetea, C., et al. (2014). Plastidial transporters KEA1, -2, and -3 are essential for chloroplast osmoregulation, integrity, and pH regulation in Arabidopsis. Proc. Natl. Acad. Sci. U.S.A. 111, 7480–7485. doi: 10.1073/pnas.1323899111
Laubinger, W., Deckers-Hebestreit, G., Altendorf, K., Dimroth, P. (1990). A hybrid adenosinetriphosphatase composed of F1 of Escherichia coli and F0 of Propionigenium modestum is a functional sodium ion pump. Biochemistry 29, 5458–5463. doi: 10.1021/bi00475a008
Liu, J., Fackelmayer, O. J., Hicks, D. B., Preiss, L., Meier, T., Sobie, E. A., et al. (2011). Mutations in a helix-1 motif of the ATP synthase c-subunit of Bacillus pseudofirmus OF4 cause functional deficits and changes in the c-ring stability and mobility on sodium dodecyl sulfate-polyacrylamide gel electrophoresis. Biochemistry 50, 5497–5506. doi: 10.1021/bi2005009
MacKenzie, K. R., Prestegard, J. H., Engelman, D. M. (1997). A transmembrane helix dimer: structure and implications. Science 276 (5309), 131–133. doi: 10.1126/science.276.5309.131
Matthies, D., Preiss, L., Klyszejko, A. L., Muller, D. J., Cook, G. M., Vonck, J., et al. (2009). The c13 ring from a thermoalkaliphilic ATP synthase reveals an extended diameter due to a special structural region. J. Mol. Biol. 388, 611–618. doi: 10.1016/j.jmb.2009.03.052
Meier, T., Yu, J., Raschle, T., Henzen, F., Dimroth, P., Muller, D. J. (2005). Structural evidence for a constant c11 ring stoichiometry in the sodium F-ATP synthase. FEBS J. 272, 5474–5483. doi: 10.1111/j.1742-4658.2005.04940.x
Meier, T., Morgner, N., Matthies, D., Pogoryelov, D., Keis, S., Cook, G. M., et al. (2007). A tridecameric c ring of the adenosine triphosphate (ATP) synthase from the thermoalkaliphilic Bacillus sp. strain TA2.A1 facilitates ATP synthesis at low electrochemical proton potential. Mol. Microbiol. 65, 1181–1192. doi: 10.1111/j.1365-2958.2007.05857.x
Meyer Zu Tittingdorf, J. M. W., Rexroth, S., Schafer, E., Schlichting, R., Giersch, C., Dencher, N. A., et al. (2004). The stoichiometry of the chloroplast ATP synthase oligomer III in Chlamydomonas reinhardtii is not affected by the metabolic state. Biochim. Biophys. Acta 1659, 92–99. doi: 10.1016/j.bbabio.2004.08.008
Mitchell, P. (1961). Coupling of phosphorylation to electron and hydrogen transfer by a chemi-osmotic type of mechanism. Nature 191, 144–148. doi: 10.1038/191144a0
Mitchell, P. (1966). Chemiosmotic coupling in oxidative and photosynthetic phosphorylation. Biol. Rev. Camb. Philos. Soc. 41, 445–502. doi: 10.1111/j.1469-185X.1966.tb01501
Moore, A. L., Rich, P. R., Douce, R., Day, R. A. (1985). “Organization of the Respiratory Chain and Oxidative Phosphorylation,” in Higher Plant Cell Respiration. Eds. Douce, R., Day, D. A. (Berlin: Springer), 134–172.
Morales, A., Yin, X., Harbinson, J., Driever, S. M., Molenaar, J., Kramer, D. M., et al. (2018). In silico analysis of the regulation of the photosynthetic electron transport Chain in C3 Plants. Plant Physiol. 176, 1247–1261. doi: 10.1104/pp.17.00779
Muller, D. J., Dencher, N. A., Meier, T., Dimroth, P., Suda, K., Stahlberg, H., et al. (2001). ATP synthase: constrained stoichiometry of the transmembrane rotor. FEBS Lett. 504, 219–222. doi: 10.1016/S0014-5793(01)02708-9
Mullineaux, C. W. (2014). Co-existence of photosynthetic and respiratory activities in cyanobacterial thylakoid membranes. Biochim. Biophys. Acta 1837, 503–511. doi: 10.1016/j.bbabio.2013.11.017
Nakamura, Y., Kaneko, T., Sato, S., Mimuro, M., Miyashita, H., Tsuchiya, T., et al. (2003). Complete genome structure of Gloeobacter violaceus PCC 7421, a cyanobacterium that lacks thylakoids. DNA Res. 10, 137–145. doi: 10.1093/dnares/10.4.137
Nishio, J. N., Whitmarsh, J. (1993). Dissipation of the proton electrochemical potential in intact chloroplasts (II. The pH gradient monitored by cytochrome f reduction kinetics). Plant Physiol. 101, 89–96. doi: 10.1104/pp.101.1.89
Niyogi, K. K., Truong, T. B. (2013). Evolution of flexible non-photochemical quenching mechanisms that regulate light harvesting in oxygenic photosynthesis. Curr. Opin. Plant Biol. 16, 307–314. doi: 10.1016/j.pbi.2013.03.011
Noctor, G., Foyer, C. H. (2000). Homeostasis of adenylate status during photosynthesis in a fluctuating environment. J. Exp. Bot. 51 Spec No, 347–356. doi: 10.1093/jexbot/51.suppl_1.347
Olsson, K., Keis, S., Morgan, H. W., Dimroth, P., Cook, G. M. (2003). Bioenergetic properties of the thermoalkaliphilic Bacillus sp. strain TA2.A1. J. Bacteriol. 185, 461–465. doi: 10.1128/JB.185.2.461-465.2003
Panke, O., Rumberg, B. (1996). Kinetic modelling of the proton translocating CF0CF1-ATP synthase from spinach. FEBS Lett. 383, 196–200. doi: 10.1016/0014-5793(96)00246-3
Pogoryelov, D., Yu, J., Meier, T., Vonck, J., Dimroth, P., Muller, D. J. (2005). The c15 ring of the Spirulina platensis F-ATP synthase: F1/F0 symmetry mismatch is not obligatory. EMBO Rep. 6, 1040–1044. doi: 10.1038/sj.embor.7400517
Pogoryelov, D., Reichen, C., Klyszejko, A. L., Brunisholz, R., Muller, D. J., Dimroth, P., et al. (2007). The oligomeric state of c rings from cyanobacterial F-ATP synthases varies from 13 to 15. J. Bacteriol. 189, 5895–5902. doi: 10.1128/jb.00581-07
Pogoryelov, D., Klyszejko, A. L., Krasnoselska, G. O., Heller, E. M., Leone, V., Langer, J. D., et al. (2012). Engineering rotor ring stoichiometries in the ATP synthase. Proc. Natl. Acad. Sci. U.S.A. 109, E1599–E1608. doi: 10.1073/pnas.1120027109
Preiss, L., Yildiz, O., Hicks, D. B., Krulwich, T. A., Meier, T. (2010). A new type of proton coordination in an F(1)F(o)-ATP synthase rotor ring. PLoS Biol. 8, e1000443. doi: 10.1371/journal.pbio.1000443
Preiss, L., Klyszejko, A. L., Hicks, D. B., Liu, J., Fackelmayer, O. J., Yildiz, O., et al. (2013). The c-ring stoichiometry of ATP synthase is adapted to cell physiological requirements of alkaliphilic Bacillus pseudofirmus OF4. Proc. Natl. Acad. Sci. U.S.A. 110, 7874–7879. doi: 10.1073/pnas.1303333110
Rippka, R., Waterbury, J., Cohenbazire, G. (1974). A cyanobacterium which lacks Thylakoids. Arch. Microbiol. 100, 419–436. doi: 10.1007/bf00446333
Rutherford, A. W., Osyczka, A., Rappaport, F. (2012). Back-reactions, short-circuits, leaks and other energy wasteful reactions in biological electron transfer: redox tuning to survive life in O(2). FEBS Lett. 586, 603–616. doi: 10.1016/j.febslet.2011.12.039
Scanlan, D. J., Ostrowski, M., Mazard, S., Dufresne, A., Garczarek, L., Hess, W. R., et al. (2009). Ecological genomics of marine picocyanobacteria. Microbiol. Mol. Biol. Rev. 73, 249–24+. doi: 10.1128/mmbr.00035-08
Scheibe, R. (2004). Malate valves to balance cellular energy supply. Physiol. Plant. 120, 21–26. doi: 10.1111/j.0031-9317.2004.0222.x
Schneider, A., Steinberger, I., Herdean, A., Gandini, C., Eisenhut, M., Kurz, S., et al. (2016). The evolutionarily conserved protein PHOTOSYNTHESIS AFFECTED MUTANT71 is required for efficient manganese uptake at the thylakoid membrane in Arabidopsis. Plant Cell 28, 892–910. doi: 10.1105/tpc.15.00812
Seelert, H., Poetsch, A., Dencher, N. A., Engel, A., Stahlberg, H., Muller, D. J. (2000). Structural biology. Proton-powered turbine of a plant motor. Nature 405, 418–419. doi: 10.1038/35013148
Silverstein, T. P. (2014). An exploration of how the thermodynamic efficiency of bioenergetic membrane systems varies with c-subunit stoichiometry of F1F0 ATP synthases. J. Bioenerg. Biomembr. 46, 229–241. doi: 10.1007/s10863-014-9547-y
Smith, D. J., Stokes, B. O., Boyer, P. D. (1976). Probes of initial phosphorylation events in ATP synthesis by chloroplasts. J. Biol. Chem. 251, 4165–4171.
Steigmiller, S., Turina, P., Graber, P. (2008). The thermodynamic H+/ATP ratios of the H+-ATPsynthases from chloroplasts and Escherichia coli. Proc. Natl. Acad. Sci. U. S. A. 105, 3745–3750. doi: 10.1073/pnas.0708356105
Stomp, M., Huisman, J., Stal, L. J., Matthijs, H. C. (2007). Colorful niches of phototrophic microorganisms shaped by vibrations of the water molecule. ISME J. 1, 271–282. doi: 10.1038/ismej.2007.59
Strand, D. D., Kramer, D. M. (2014). “Control of Non-Photochemical Exciton Quenching by the Proton Circuit of Photosynthesis,” in Non-Photochemical Quenching and Energy Dissipation in Plants, Algae and Cyanobacteria. Eds. DemmigAdams, B., Garab, G., Adams, W., Govindjee (Dordrecht: Springer), 387–408. doi: 10.1007/978-94-017-9032-1_18
Strand, D. D., Fisher, N., Kramer, D. M. (2016). Distinct Energetics and Regulatory Functions of the Two Major Cyclic Electron Flow Pathways in Chloroplasts. Ed. Kirchhoff Wymondham, H. (Norfolk, United Kingdom: Caister Academic Press).
Sturr, M. G., Guffanti, A. A., Krulwich, T. A. (1994). Growth and bioenergetics of alkaliphilic Bacillus firmus OF4 in continuous culture at high pH. J. Bacteriol. 176, 3111–3116. doi: 10.1128/jb.176.11.3111-3116.1994
Suzuki, T., Ozaki, Y., Sone, N., Feniouk, B. A., Yoshida, M. (2007). The product of uncI gene in F1Fo-ATP synthase operon plays a chaperone-like role to assist c-ring assembly. Proc. Natl. Acad. Sci. U. S. A. 104, 20776–20781. doi: 10.1073/pnas.0708075105
Takizawa, K., Cruz, J. A., Kanazawa, A., Kramer, D. M. (2007). The thylakoid proton motive force in vivo. Quantitative, non-invasive probes, energetics, and regulatory consequences of light-induced pmf. Biochim. Biophys. Acta 1767, 1233–1244. doi: 10.1016/j.bbabio.2007.07.006
Takizawa, K., Kanazawa, A., Kramer, D. M. (2008). Depletion of stromal P(i) induces high “energy-dependent” antenna exciton quenching (qE) by decreasing proton conductivity at CF(O)-CF(1) ATP synthase. Plant Cell Env. 31, 235–243. doi: 10.1111/j.1365-3040.2007.01753.x
Turina, P., Samoray, D., Graber, P. (2003). H+/ATP ratio of proton transport-coupled ATP synthesis and hydrolysis catalysed by CF0F1-liposomes. EMBO J. 22, 418–426. doi: 10.1093/emboj/cdg073
Vermaas, W. F. J. (2001). “Photosynthesis and Respiration in Cyanobacteria,” in eLS (American Cancer Society). doi: 10.1038/npg.els.0001670
Viola, S., Bailleul, B., Yu, J., Nixon, P., Selles, J., Joliot, P., et al. (2019). Probing the electric field across thylakoid membranes in cyanobacteria. Proc. Natl. Acad. Sci. U. S. A. 116, 21900–21906. doi: 10.1073/pnas.1913099116
von Ballmoos, C., Cook, G. M., Dimroth, P. (2008). Unique rotary ATP synthase and its biological diversity. Annu. Rev. Biophys. 37, 43–64. doi: 10.1146/annurev.biophys.37.032807.130018
von Ballmoos, C., Wiedenmann, A., Dimroth, P. (2009). Essentials for ATP synthesis by F1F0 ATP synthases. Annu. Rev. Biochem. 78, 649–672. doi: 10.1146/annurev.biochem.78.081307.104803
Vonck, J., von Nidda, T. K., Meier, T., Matthey, U., Mills, D. J., Kuhlbrandt, W., et al. (2002). Molecular architecture of the undecameric rotor of a bacterial Na+-ATP synthase. J. Mol. Biol. 321, 307–316. doi: 10.1016/S0022-2836(02)00597-1
Vos, M. H., van Gorkom, H. J., van Leeuwen, P. J. (1991). An electroluminescence study of stabilization reactions in the oxygen-evolving complex of Photosystem II. Biochim. Biophys. Acta 1056, 27–39. doi: 10.1016/s0005-2728(05)80069-6
Walker, B. J., Strand, D. D., Kramer, D. M., Cousins, A. B. (2014). The response of cyclic electron flow around photosystem I to changes in photorespiration and nitrate assimilation. Plant Physiol. 165, 453–462. doi: 10.1104/pp.114.238238
Walker, J. E. (2013). The ATP synthase: the understood, the uncertain and the unknown. Biochem. Soc. Trans. 41, 1–16. doi: 10.1042/bst20110773
Watt, I. N., Montgomery, M. G., Runswick, M. J., Leslie, A. G., Walker, J. E. (2010). Bioenergetic cost of making an adenosine triphosphate molecule in animal mitochondria. Proc. Natl. Acad. Sci. U.S.A. 107, 16823–16827. doi: 10.1073/pnas.1011099107
Zaharieva, I., Wichmann, J. M., Dau, H. (2011). Thermodynamic limitations of photosynthetic water oxidation at high proton concentrations. J. Biol. Chem. 286, 18222–18228. doi: 10.1074/jbc.M111.237941
Zaks, J., Amarnath, K., Kramer, D. M., Niyogi, K. K., Fleming, G. R. (2012). A kinetic model of rapidly reversible nonphotochemical quenching. Proc. Natl. Acad. Sci. U.S.A. 109, 15757–15762. doi: 10.1073/pnas.1211017109
Zhang, R., Cruz, J. A., Kramer, D. M., Magallanes-Lundback, M. E., Dellapenna, D., Sharkey, T. D. (2009). Moderate heat stress reduces the pH component of the transthylakoid proton motive force in light-adapted, intact tobacco leaves. Plant. Cell Environ. 32, 1538–1547. doi: 10.1111/j.1365-3040.2009.02018.x
Keywords: photosynthesis, adenosine triphosphate synthase, proton motive force, singlet oxygen, electron transfer, bioenergetics
Citation: Davis GA and Kramer DM (2020) Optimization of ATP Synthase c–Rings for Oxygenic Photosynthesis. Front. Plant Sci. 10:1778. doi: 10.3389/fpls.2019.01778
Received: 11 September 2019; Accepted: 20 December 2019;
Published: 30 January 2020.
Edited by:
Cornelia Spetea, University of Gothenburg, SwedenReviewed by:
Mark Aurel Schöttler, Max Planck Institute of Molecular Plant Physiology, GermanyMikko Tikkanen, University of Turku, Finland
Copyright © 2020 Davis and Kramer. This is an open-access article distributed under the terms of the Creative Commons Attribution License (CC BY). The use, distribution or reproduction in other forums is permitted, provided the original author(s) and the copyright owner(s) are credited and that the original publication in this journal is cited, in accordance with accepted academic practice. No use, distribution or reproduction is permitted which does not comply with these terms.
*Correspondence: David M. Kramer, a3JhbWVyZDhAbXN1LmVkdQ==