- 1Key Laboratory of Aquatic Botany and Watershed Ecology, Wuhan Botanical Garden, Center of Plant Ecology, Core Botanical Gardens, Chinese Academy of Sciences, Wuhan, China
- 2Aix Marseille Univ CNRS, BIP UMR 7281, IMM, FR 3479, Marseille, France
- 3University of Chinese Academy of Sciences, Beijing, China
- 4Institute of Hydrobiology, Chinese Academy of Sciences, Wuhan, China
Acclimation to variable CO2 was studied in floating leaves of the freshwater monocot Ottelia cordata grown in either low or high CO2. The most striking anatomical variations responding to high CO2 included the enlarged upper epidermal cells and the decreased area of epidermal chloroplasts. Stomata that distributed on the upper surface, and the stomatic chamber area, showed no significant response to high CO2. pH-drift experiments indicated that floating leaves of O. cordata were able to use bicarbonate regardless of CO2 concentrations. Photosynthetic enzyme activities and patterns of organic acids fluctuation confirmed that floating leaves of O. cordata can operate CAM only at low CO2, and perform C4-like metabolism at both high and low CO2. Overall, the present results imply that the floating leaves of O. cordata does not just rely on the atmospheric CO2 for its inorganic carbon, but is also dependent on CO2 and bicarbonate in the water. By showing these effects of CO2 variation, we highlight the need for further experimental studies on the regulatory mechanisms in O. cordata floating leaves, that prevent futile cycling among the three CO2 concentrating mechanisms (bicarbonate use, C4, and CAM metabolism) and the strategy for exploiting atmospheric CO2, as well as studies on the detailed biochemical pathway for C4 and CAM metabolism in this species.
Introduction
Since preindustrial times, the atmospheric CO2 concentration has increased from ~280 ppm to currently over 400 ppm and is predicted to reach 800 ppm by the year 2100 (IPCC, 2014). The increasing concentration of atmospheric CO2 might influence plant traits, such as growth, photosynthesis, as well as the morphology and anatomy of leaves (Long et al., 2004). The responses of terrestrial plants to increasing atmospheric CO2 have been studied extensively (e.g. Long et al., 2004; Ainsworth and Long, 2005), however, studies on aquatic plants are sparse and mainly focus on marine species (Johnson et al., 2013; Koch et al., 2013). The effects of increasing atmospheric CO2 on freshwater macrophytes appear to be more intricate and might be species- and water body- specific (Hussner et al., 2019). Increasing the atmospheric CO2 will increase the availability of dissolved inorganic carbon (DIC) and decrease the pH in some aquatic ecosystems; this will also increase the proportion of CO2 and HCO3- within the pool of DIC, within a given pH range (Pedersen et al., 2013). Nevertheless, in some cases such as the productive lakes, the aquatic ecosystems are pulled out of the air-equilibrium either by rapid photosynthesis that decrease CO2 concentrations or rapid decomposition of litter in the sediment that increase CO2 concentrations in the water. This can generate diel changes in CO2 concentrations of over 100-fold as well as the seasonal changes (Maberly, 1996; Schippers et al., 2004). Generally, photosynthesis in submerged aquatic plants is more sensitive and responsive to increasing CO2 than HCO3- (Maberly and Madsen, 1998).
As to photosynthesis in submerged aquatic plants, CO2 is the preferred form of carbon and a number of species could only use CO2. However, about 50% of tested species have evolved strategies to use HCO3- to handle CO2 limitation (Iversen et al., 2019). The problem of limited inorganic carbon supply is caused by several factors (Maberly and Gontero, 2017): (1) the CO2 diffusion rate in water is ~10 000 times lower than in air, which hinder the CO2 transport into freshwater plants through the boundary layer; (2) when the requirement for inorganic carbon by photosynthesis exceeds the supply from the environment, especially in productive systems, the CO2 can be depleted and its concentration close to zero. Thus, in addition to HCO3- use, freshwater plants have evolved a diversity of CO2 concentrating mechanisms (CCMs) to increase further the efficiency of carbon utilization. Although much less widespread than HCO3- use, some freshwater macrophytes possess a type of C4 (Bowes, 2011) or crassulacean acid metabolism (CAM)-like metabolism (Keeley, 1998). Both C4 and CAM implement primary carbon fixation via the enzyme phosphoenolpyruvate carboxylase (PEPC), that is active during either day for C4 or during night for CAM. Unlike terrestrial C4 plants, in aquatic species, C4 metabolism can be induced under the limitation of inorganic carbon, or be constitutive independent of the CO2 concentration (Maberly and Gontero, 2017). The frequency of aquatic species with CAM is ~9% (Maberly and Gontero, 2018), and the utilization of CO2 can occur during daytime, allowing the freshwater CAM plants to assimilate CO2 continuously (Keeley, 1998; Madsen et al., 2002), which differs from the terrestrial CAM plants. CAM metabolism is a plastic process in freshwater plants (Bowes and Salvucci, 1989), and CO2 concentration can affect its activity (Klavsen and Maberly, 2010; Zhang et al., 2014). Except the amelioration strategies described above, exploitation strategies are another diversity of strategies to minimize the carbon constraint in aquatic plants that involve morphological and anatomical features to give access to higher availability of free CO2 from the sediment or the atmosphere (Klavsen et al., 2011; Maberly and Gontero, 2018). Floating and aerial leaves of some amphibious species are the examples with exploitation strategies that could exploit more CO2 from the atmosphere (Sand-Jensen et al., 1992; Sand-Jensen and Frost-Christensen, 1998). The compilation of CCMs in aquatic macrophytes showed that less than 25% species with an ability to use HCO3- have alternative strategies, which mainly comprise C4 and access to atmospheric CO2; nevertheless, CAM and C4 do not usually coexist in one species (Maberly and Madsen, 2002; Maberly and Gontero, 2017). The submerged freshwater plant, Ottelia alismoides, is hitherto the only known species to operate three CCMs (HCO3- use, C4 and CAM metabolism) in the same tissue (Zhang et al., 2014; Shao et al., 2017).
In addition to the responses of CCMs to variable CO2 availability, the size and anatomical structure of plant leaves are also influenced by CO2 concentration (Radoglou and Jarvis, 1990). Enhanced CO2 generally increases the size of terrestrial plants (Pritchard et al., 1999). In Brassica juncea, high CO2 increased the thickness of the upper and lower epidermis, as well as the mesophyll cells of leaves (Uprety et al., 2001). While, in sorghum, the increase in growth CO2 resulted in a marked decrease in the thickness of the bundle sheath (Watling et al., 2000). In O. alismoides, high CO2 increased the thicknesses of the epidermal layers, the air space and mesophyll cells (Han et al., 2020). To our knowledge, there is little information in the literature concerning the effects of varying CO2 availability on leaf structure and the relationships between structure and function in aquatic plants.
Ottelia cordata (Wallich) Dandy, a member of the Hydrocharitaceae, is an aquatic macrophyte distributed in China, Cambodia, Myanmar and Thailand (Wang et al., 2019; Li et al., 2020). It is a perennial and heterophyllous plant with linear or lanceolate submersed leaves and ovate-cordate floating leaves. Cultivation experiments showed that during its early growth phases, O. cordata is totally submerged and it only grows submerged leaves in the first year; at the second year and after, it only grows floating leaves, which is the only part of the plant that comes into contact with air (He and Sun, 1990). It was shown recently that O. cordata floating leaf is a HCO3- user and perhaps operate CAM (Cao et al., 2019). The aim here was to quantify the extent of plasticity of CCMs and leaf anatomy in this species, in response to varying CO2 availability in the water, to evaluate further possible effects of CO2 enrichment on this species.
Materials and Methods
Plant Material
Healthy O. cordata plants were collected randomly from a wild population at Haikou, Hainan Province, China. After collection, in order to eliminate the differences caused by environmental heterogeneity, the plants were cultured in plastic pots with aseptic soil and filled with water, and were illuminated with FSL T5/865 28 W fluorescence tubes in a growth room located in Wuhan Botanical Garden, with ambient temperature (13°C~17°C) and ~130 μmol photon m-2 s-1 (Li-Cor underwater sensor, UWQ, connected to a Li-Cor LI-1400 data logger) at the water surface, with a 14-h light (08:00–22:00)/10-h dark photoperiod. The light intensity condition was chosen as a trade-off between having sufficient light for photosynthesis to avoid the effects induced by low light levels on anatomy and photosynthetic physiology, and not so much light to avoid causing photodamage when at low CO2 concentrations. The water depth was maintained at ~30 cm during the acclimation. The transplanted plants were cultured for 3~4 weeks before they were used in the experiments.
Response of O. cordata Floating Leaves to Different CO2 Concentrations
In the different CO2 acclimated experiments, the pots of O. cordata plants were put into the white plastic tanks (65 cm × 45 cm × 35 cm) containing tap water and incubated at two CO2 concentrations as described previously (Huang et al., 2018). In the high CO2 treatment (HC), the target pH of 7.0 was maintained between 6.52 and 7.06 by bubbling the medium with pure CO2 under the control of a microcomputer pH controller (model 6311, Jenco Instruments, USA), producing CO2 concentrations between 380~1603 μM with a mean of 941 μM. A low CO2 (LC) concentration was produced by the natural photosynthesis of the plants which depleted the inorganic carbon of the water, and increased pH from 8.09 to 8.91, with a CO2 concentration range of 3~28 µM and a mean of 15 µM. The acclimation of different CO2 with four replicate pots per treatment, lasted four weeks in the growth room. Over the whole period of acclimation, alkalinity, pH and temperature were measured to calculate the CO2 concentration using the equations reported in Maberly (1996). More information of the conditions in the treatments is shown in Table 1. Following the different CO2 acclimation, there were newly floating leaves emerged, which were harvested for anatomical structure observation and physiological parameters measurement.
Leaf Anatomy Observation by Light Microscopy and Transmission Electron Microscopy
The youngest fully developed floating leaves in both CO2 treatments were sampled at 19:00 and were completed within half an hour. Then the leaves were sliced into 3 mm× 3 mm leaf fragments along the midrib. The segments were fixed immediately in 2.5% glutaraldehyde (pH 7.4) at 4°C overnight and then post-fixed in 1% OsO4 at 4°C for 2.5 h. The semithin sections and ultrathin sections of the leaf samples were obtained according to the method of Han et al. (2020). Semithin sections were stained with methylene blue and observed using a digital light microscope (Motic BA310). Quantitative characteristics of leaf structures including the area and size of the epidermal and mesophyll cells, as well as the air space and stomatic chamber, were measured using the Motic Images Plus 2.0 ML software. Ultrathin sections were examined and photographed under a TEM (HT7700, Hitachi, Japan). The area and size of chloroplasts and starch grains in the electron micrographs were measured with ImageJ software. At least 10 epidermal/mesophyll cells from both the upper and lower surfaces were measured per leaf from the digitized images.
pH-Drift Experiments and Drift Parameters
The ability of O. cordata floating leaves to use HCO3- was assessed in pH-drift experiments (Maberly and Spence, 1983). About 0.2~0.3 g fresh weight (FW) of leaves collected from different CO2 acclimated plants, rinsed in clean tap water and then incubated in 70-ml test bottles with 50 ml of test solution (equimolar concentrations of NaHCO3 and KHCO3 at an overall concentration of 1 mM). The bottles with plant leaves were sealed with glass stoppers and incubated in a growth room at 25°C and ~130 µmol photon m-2 s-1 PAR. After ~24 h continuous irradiance, the final pH of the medium was measured with pH electrode (model IP-600-9 Jenco Instruments, USA) connected to a microcomputer pH controller. The final alkalinity of the solution was measured by Gran titration (Zhang et al., 2014). The total Ci remaining at the end of pH-drift, which represents the dissolved inorganic carbon comprising free CO2, HCO3- and CO32-, was designated as CT. The concentration of CT, CO2 and HCO3- in the solution were calculated from the alkalinity, temperature and pH (Maberly, 1996). The quotient of CT/alkalinity reflects the ability of O. cordata floating leaves to deplete inorganic carbon (Maberly and Spence, 1983).
Photosynthetic Enzyme Activity Measurement
Floating leaves were harvested at 21:00 h (dusk) before the lights turn off and at 07:00 h (dawn) before the lights turn on and immediately frozen in liquid nitrogen for further measurement of photosynthetic enzyme activity. The activity of ribulose 1,5-bisphosphate carboxylase–oxygenase (Rubisco), phosphoenol pyruvate carboxylase (PEPC), pyruvate phosphate dikinase (PPDK) and NADP-malic enzyme (NADP-ME), were determined according to the methods described in Zhang et al. (2014), and were assessed from the rates of NADH disappearance or appearance at 340 nm and 25°C, using a microplate reader (Tecan M200 PRO, Austria).
CAM Activity and Organic Acids Content
Fresh O. cordata floating leaves (0.2~0.5 g), were collected at 21:00 h (dusk) and 07:00 h (dawn) and immediately frozen in liquid nitrogen for further measurement of acidity and organic acids. The acidity was measured according to the method of Zhang et al. (2014).
The organic acids in O. cordata floating leaves were extracted and detected based on the methods described previously (Pedersen et al., 2011). About 0.2 g frozen leaves were ground and extracted with ice-cold 5% (v/v) perchloric acid using a mortar and pestle. The homogenized samples were centrifuged at 8,000g for 10 min, the supernatant was collected and the procedure was repeated once with ice-cold 5% perchloric acid. The supernatants were mixed and adjusted to pH 3.0~3.5 using saturated K2CO3 solution, and then centrifuged again at 4,000g for 5 min. The resulting supernatant was filtered through a 0.22-μm filter and transferred to vials for quantification with high performance liquid chromatography (HPLC). The component of organic acids in the extraction was separated in an Athena C18-WP (4.6 mm × 250 mm) column (CNW Technologies GmbH, Germany) maintained at a constant temperature of 30°C. The mobile phase consisted of 25 mM KH2PO4 (pH 2.5) with methanol at a constant flow rate of 0.8 ml min-1. The concentration of organic acids was detected at 220 nm with a photodiode array detector (PDA) coupled to a HPLC (Waters e2695 system, Milford, MA, USA). The quantitative determination of the organic acids was obtained by analyzing the chromatographic data using Data System based on the corresponding retention time and peak area of the different organic acids.
Chlorophyll, Dry Weight, and Leaf Area
The content of chlorophyll a and b in O. cordata floating leaves were determined spectrophotometrically at 649 and 665 nm (TU-1810PC, Purkinje General, China) according to the method described in Shao et al. (2017). Dry weight (DW) was measured after the leaves were dried for 48 h at 80°C. Projected (1-sided) leaf area was calculated from digital photographs with AreaAna software (Huazhong University of Sciences and Technology, China).
Statistical Analysis
The data were analyzed using SPSS 16.0 (SPSS Inc., Chicago, USA). The significance of CO2 treatment and sample time were determined with two-way ANOVA. The independent sample t-tests were used for the comparisons on the effects of CO2 concentrations on the morphological and anatomical characteristics as well as the pH-drift traits. Pearson correlation was used to test the correlation between different enzyme activities and the significance level was set at 5%.
Results
Chlorophyll, FW/DW, and Leaf Area
High CO2 did not have a statistically significant effect on the chlorophyll content, FW/DW and leaf area in O. cordata floating leaves, when compared with that of low CO2 (p>0.05; Table 2).
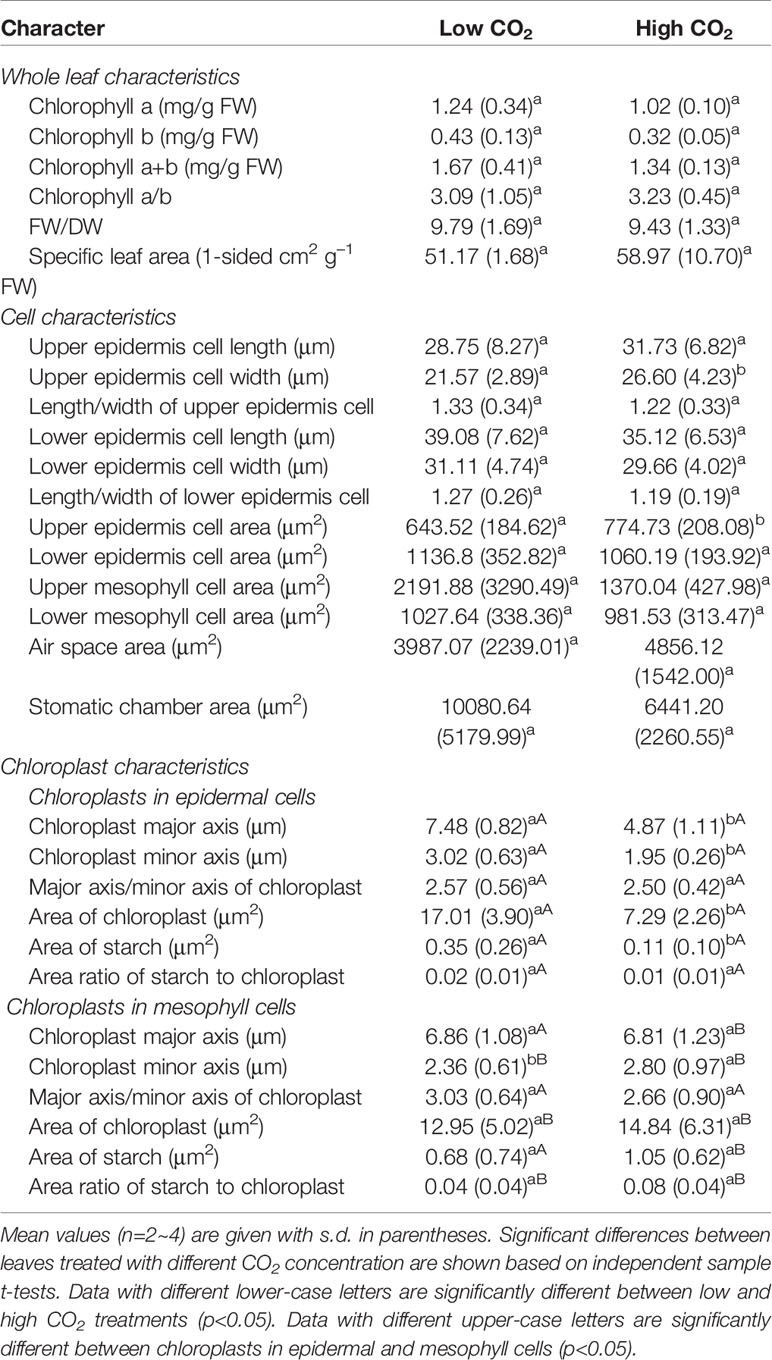
Table 2 Effects of low and high CO2 concentrations on the morphological and anatomical characteristics of the O. cordata floating leaves.
Anatomical Structure and Ultrastructure of Chloroplast
The leaf anatomy of O. cordata floating leaves under high and low CO2 were shown in Figure 1 and the basic structure of leaves did not vary with CO2 concentrations. The surface of O. cordata floating leaf was relatively flat and smooth. Transverse section observation showed that the upper and lower epidermis was single-layered rectangular cells, closely arranged (Figures 1A–F). The area and width of the upper epidermal cells in leaves grown under high CO2 were notably larger than those under low CO2 (p<0.05; Table 2), however there was no significant difference in lower epidermal cells (p>0.05; Table 2). The length and length/width ratio of the upper and lower epidermal cells were not significantly affected by CO2 concentrations (p>0.05; Table 2). Stomata only distributed on the upper epidermis of floating leaves of O. cordata (Figures 1B, E). The mesophyll tissues were developed, and there were air spaces interspersed among the lower mesophyll cells. The differentiation between palisade tissues and spongy tissues was present (Figures 1B, C, E, F). There was no significant difference in the area of air spaces, stomatic chamber, upper and lower mesophyll cells between high and low CO2 treated leaves (p>0.05; Table 2).
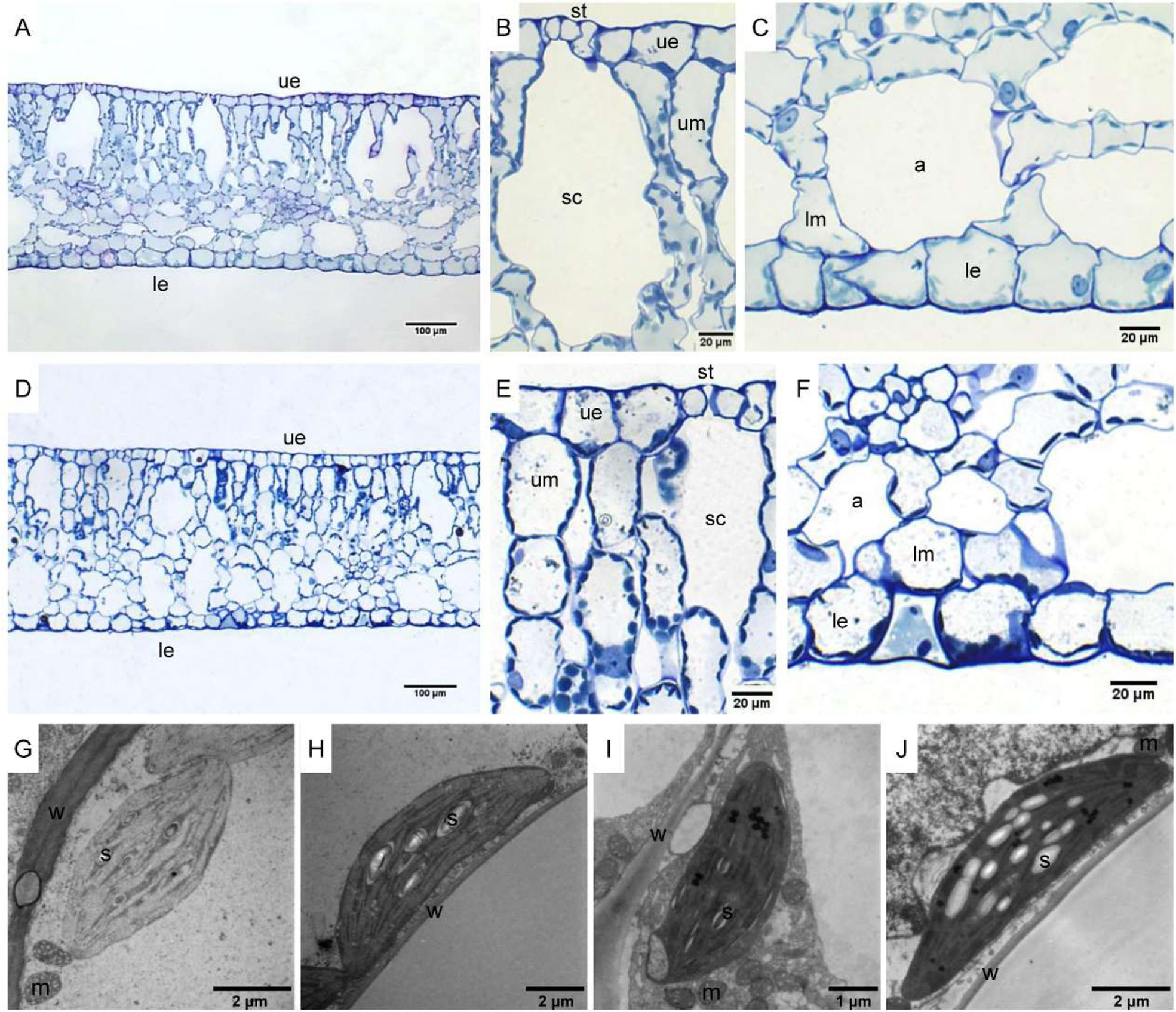
Figure 1 Leaf anatomy and chloroplast ultrastructure of O. cordata floating leaves grown at low and high CO2. (A–C) Anatomy of O. cordata floating leaf grown under low CO2. (D–F) Anatomy of O. cordata floating leaf grown under high CO2. (G) Chloroplast in epidermal cells under low CO2. (H) Chloroplast in mesophyll cells under low CO2. (I) Chloroplast in epidermal cells under high CO2. (J) chloroplast in mesophyll cells under high CO2. a, air space; le, lower epidermal cell; lm, lower mesophyll cell; m, mitochondria; s, starch; st, stoma; sc, stomatic chamber; ue, upper epidermal cell; um, upper mesophyll cell; w, cell wall.
Considering chloroplasts can differ in cells responding to the variation of environmental factors, we evaluated chloroplast shape, size and ultrastructure in both epidermal and mesophyll cells grown at high and low CO2. In leaves grown at low and high CO2, the chloroplasts in epidermal cells were spindle-shaped (Figures 1G, I). The epidermal chloroplasts under low CO2 (Figure 1G) had longer length for the major and minor axis, bigger size and more starch area occupied in the chloroplast, when compared with those at high CO2 (Figure 1I; p<0.05; Table 2). However, the ratio of major axis length and minor axis length of the chloroplast and the area ratio of starch to chloroplast were not notably influenced by CO2 concentrations (p>0.05; Table 2). For the mesophyll chloroplast, when compared with low CO2 condition, except the slight but significant increased minor axis length at high CO2 (p<0.05; Figures 1H, J; Table 2), there was no other significant difference on the ultrastructure of this type of chloroplast (p>0.05; Table 2). The comparison between these two types of chloroplasts showed that, at low CO2, the epidermal chloroplasts had significantly larger area but lower area ratio of starch to chloroplast than mesophyll chloroplasts (p<0.05; Table 2). When at high CO2, the epidermal chloroplasts had significantly smaller size and area, as well as the smaller starch area and lower area ratio of starch to chloroplast than mesophyll chloroplasts (p<0.05; Table 2).
pH-Drift Characteristics
The pH-drift experiments provided clear evidence for HCO3- use in both CO2 conditions in O. cordata floating leaves (Table 3). Both high and low CO2 treated O. cordata floating leaves raised pH and reduced the concentration of free CO2 and HCO3- in the medium significantly from the initial values of 7.9, 23 μM and 0.99 mM. Final pH ranged from 10.33 for high CO2 acclimation to 10.62 for low CO2 acclimation. Correspondingly, the high and low CO2 acclimated leaves depleted CO2 and HCO3- concentrations to 17 and 4 nM, 0.18 and 0.08 mM, respectively. The [CO2], [HCO3-], and [CT] remaining at the end of pH-drift were significantly higher at high CO2 condition as compared with low CO2 acclimation (p<0.05). The quotient of CT/Alk, used to estimate the carbon uptake ability, was significantly lower in low CO2 acclimated leaves (p<0.05), indicating that the ability of using HCO3- was more efficient under low CO2 condition.
Key Photosynthetic Enzymes Activity
Rubisco activity was 4.4-fold higher in low CO2 compared to high CO2 acclimated O. cordata floating leaves at dawn (p<0.05; Table 4), in contrast at dusk the difference was not statistically significant (p>0.05; Figure 2A). PEPC activity was 1.5-fold higher in low CO2 compared to high CO2 leaves (p<0.05; Table 4), at both dusk and dawn (Figure 2B). In high CO2 acclimated O. cordata floating leaves, the PEPC : Rubisco ratio was 1.7 at the end of day and increased to 4.3 by the end of night. For low CO2 conditions, the ratio was 1.1 at the end of day and increased slightly to 1.5 by the end of night (Figure 2C, Table 4). PPDK showed a similar pattern to PEPC (Figure 2D). The CO2 concentration triggered a 1.3-fold increase in O. cordata floating leaves at low compared to high CO2 at dusk (p<0.05), but had a slightly and not significantly promotion at dawn (p>0.05). The activity of the decarboxylating enzyme NADP-ME did not vary with the CO2 concentrations (Figure 2E). There was a significant correlation between activity of PEPC vs. Rubisco and PPDK vs. PEPC (p<0.05; Figures 2F, G). Activity of NADP-ME did not correlate with changes in activity of PEPC (data not shown).
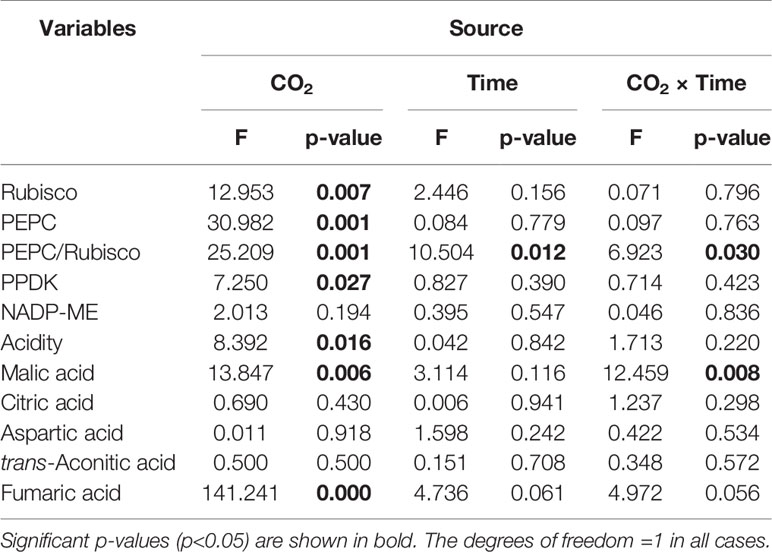
Table 4 Two-way ANOVA results for physiological parameters in O. cordata, with CO2 concentration and time as factors.
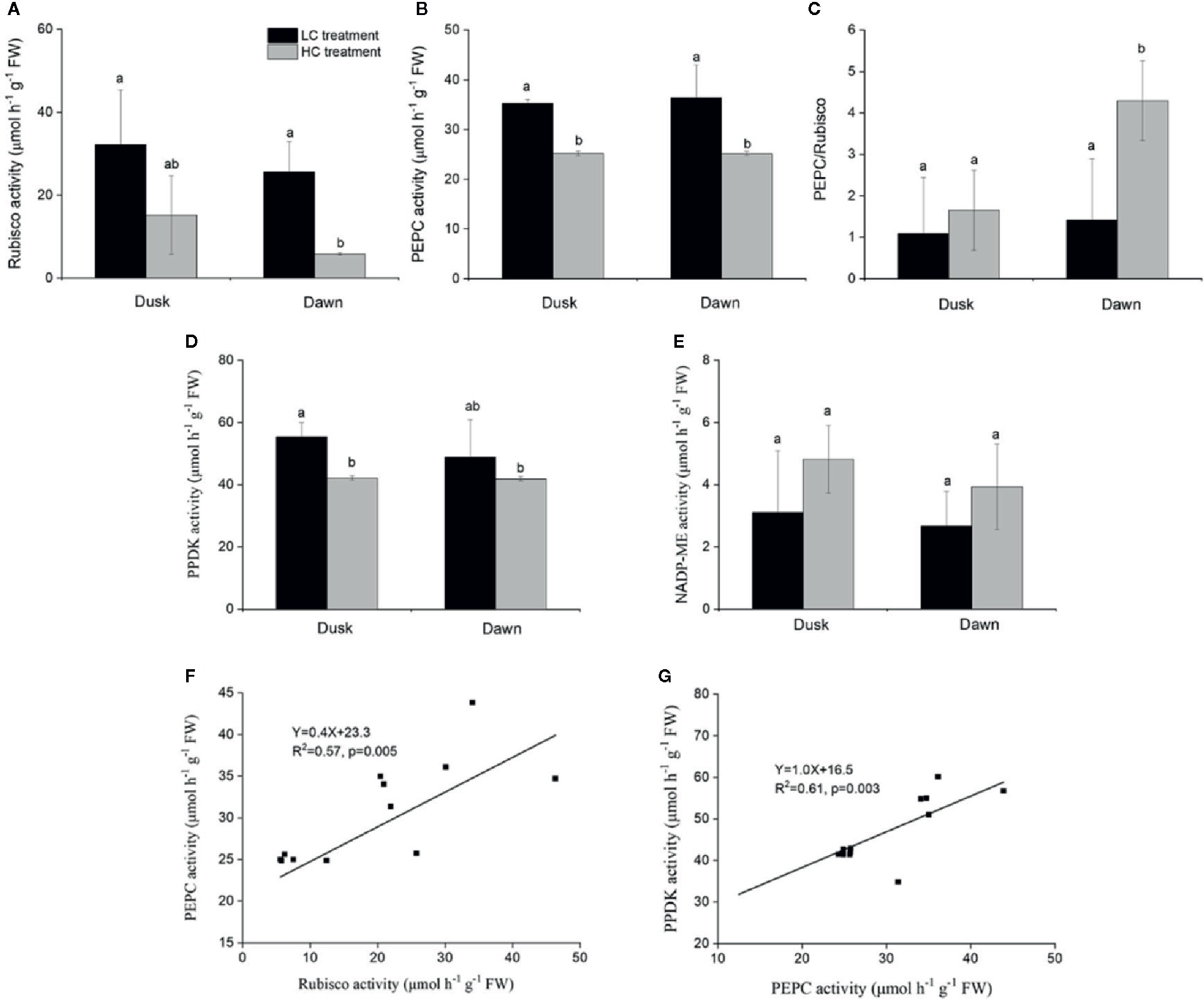
Figure 2 Influence of CO2 concentrations on activities of enzymes from O. cordata floating leaves collected at dusk (21:00 h, before the lights turn off) and dawn (07:00 h, before the lights turn on), as well as the correlations between activity of Rubisco, pyruvate phosphate dikinase (PPDK) and activity of phosphoenolpyruvate carboxylase (PEPC) at dusk and dawn, grown at low and high concentrations of CO2. (A) Rubisco activity. (B) PEPC activity. (C) Ratio of PEPC to Rubisco activity. (D) PPDK activity. (E) NADP-malic enzyme (NADP-ME) activity. (F) Correlation between activity of Rubisco and activity of PEPC. (G) Correlation between activity of PPDK and activity of PEPC. In (A~E), the mean values (n=3~4) with their s.d. are shown. Different letters show significant differences (p<0.05, post hoc Duncan’s test) among treatments. In (F~G), each point represents the activity measured from an individual sample from high CO2 and low CO2 acclimated plants. Pearson correlation was used to test the correlation between activity of PEPC and Rubisco, PPDK and PEPC.
Daily Oscillations of Acidity and Organic Acids
The CAM capacity in O. cordata floating leaves was assessed initially by measuring the diel change in acidity. Across the high and low CO2 conditions, acidity levels varied between 35 and 89 μequiv g-1 FW at dusk and between 54 and 74 μequiv g-1 FW at dawn (Figure 3A). There was a significant difference between dusk and dawn acidity levels in O. cordata floating leaves at low CO2 of about 20 μequiv g-1 FW (p<0.05), in contrast there was no evidence for diel acidity variation at high CO2.
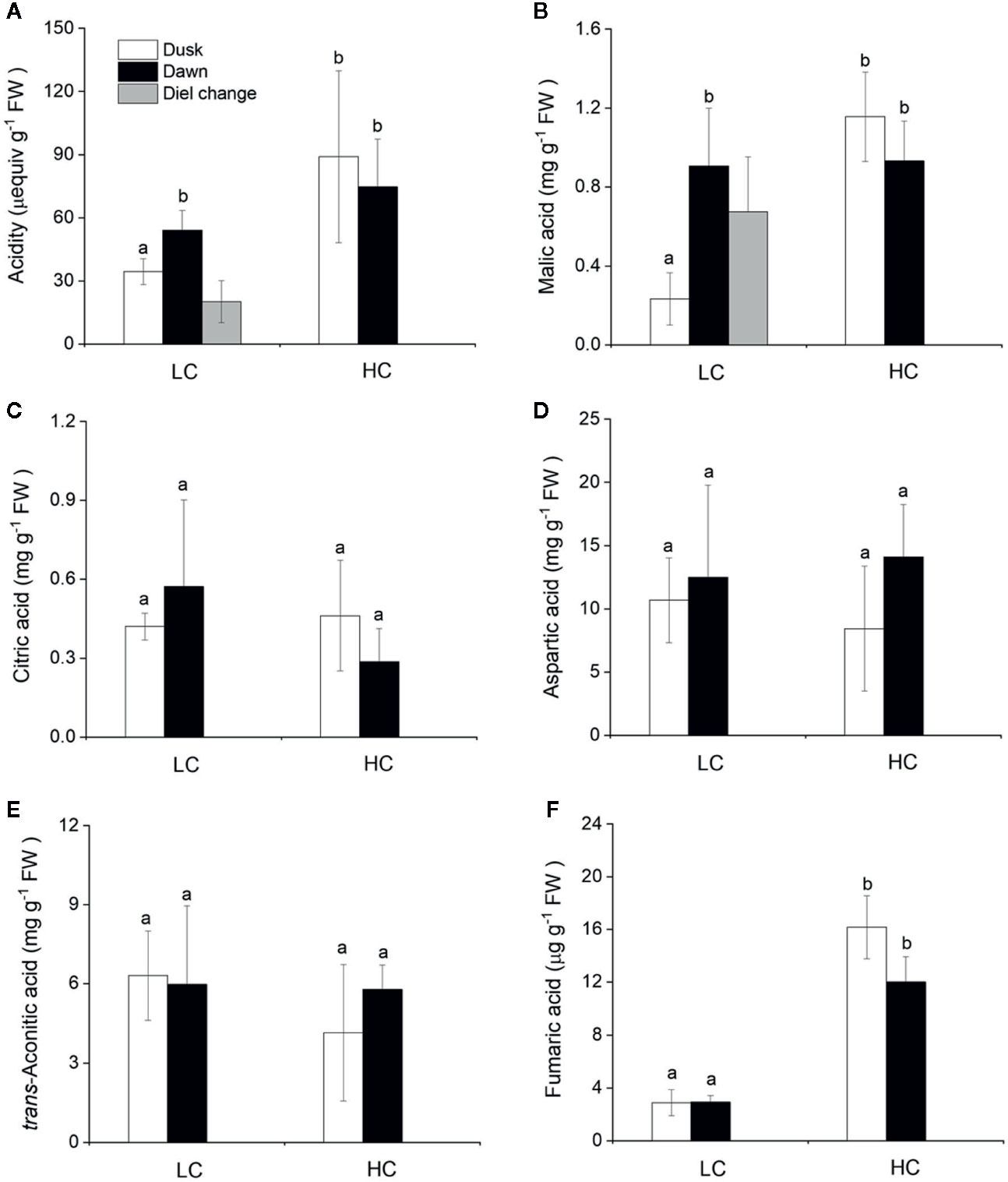
Figure 3 Influence of CO2 concentrations on acidity and concentrations of organic acids from O. cordata floating leaves collected at dusk (21:00 h, before the lights turn off) and dawn (07:00 h, before the lights turn on). (A) Acidity. (B) Malic acid. (C) Citric acid. (D) Aspartic acid. (E) trans-Aconitic acid. (F) Fumaric acid. LC means low CO2; HC means high CO2. The mean values (n=3) with their s.d. are shown. Different letters indicate significant differences (p<0.05, post hoc Duncan’s test) among treatments.
We also analyzed organic acids levels in floating leaves of O.cordata at dusk and dawn. The malic acid concentrations did not differ between the two time points at high CO2 (p>0.05; Figure 3B, Table 4). In contrast, leaf tissues of low CO2 treatment showed significant dusk/dawn oscillation in malic acid content. The concentration of malic acid at dusk was only 25% of that at dawn, showing an obvious depletion of the malate pool during day period at low CO2 (p<0.05; Figure 3B, Table 4). However, the accumulation of malic acid at dawn did not change with the concentrations of CO2 (p>0.05). Concentrations of citric acid, aspartic acid and trans-aconitic acid in the leaf tissue did not change between the two sampling times and CO2 conditions (p>0.05; Figures 3C–E, Table 4). In contrast, the concentration of fumaric acid in high CO2 acclimated O. cordata floating leaves was 5.5-fold higher at dusk and 4.1-fold higher at dawn than in low CO2 (p<0.05), but with no significant differences between the two sampling times at both CO2 treatments (p>0.05; Figure 3F, Table 4).
Discussion
Responses of Anatomy of O. cordata Floating Leaves to Variable CO2
Through the transverse section observation of the O. cordata floating leaves, some anatomical changes were observed following acclimation with different CO2. This is in agreement with previous reports in terrestrial or aquatic plants (Thomas and Harvey, 1983; Bray and Reid, 2002; Driscoll et al., 2006; Han et al., 2020). The most striking change occurred in O. cordata floating leaves responding to high CO2 is the increased transverse-section area and width of upper epidermal cells. A similar increase of the area of upper epidermal cell in O. alismoides grown under elevated CO2 was found (Han et al., 2020). In maize, the epidermal cell size from the paradermal view was also shown to be highly responsive to environmental CO2 concentration (Driscoll et al., 2006). This would suggest that the enlarged epidermal cells are formed in response to high CO2. This response may have come about to have consequences for photosynthesis possibly by expanding CO2 diffusion through the upper epidermal cell membrane. Nevertheless, in some species, such as in Phaseolus vulgaris L. seedlings (Radoglou and Jarvis, 1992), the epidermal cells remained constant in size irrespective of CO2 treatment.
Stomata were found to be distributed on the upper surface of O. cordata floating leaves. Generally, most submerged leaves do not possess stomata, or, where they do exist but they are nonfunctional (Sculthorpe, 1967). However, floating leaves of aquatic plants, such as the aquatic pteridophyte Marsilea, water lilies, and Potamogeton octandrus have stomata on the upper surface (Lin and Yang, 1999; Rudall and Knowles, 2013; Li et al., 2019). The stomata of terrestrial plants generally distribute more on lower epidermis than upper epidermis in order to reduce water loss. However, in aquatic plants, the distribution of stomata on the upper epidermis is obviously greater than that on the lower epidermis, since the upper surface of the floating leaf is accessible to the air. Floating leaves of aquatic plants is one of the examples with exploitation strategies to overcome the problem of carbon limitation that could exploit more constant and available CO2 from the atmosphere based on stomata (Maberly and Gontero, 2018). Observations of the ultrathin sections showed that the epidermal chloroplast had higher sensitivity to high CO2 than the mesophyll chloroplast in O. cordata floating leaves, which differs from the responses in O. alismoides (Han et al., 2020), where both epidermal and mesophyll chloroplasts showed sensitive responses to CO2 elevation and the size of both type of chloroplasts were increased. However, it should be noted that, in both O. cordata and O. alismoides, the area ratio of starch to chloroplast was much greater in mesophyll than in epidermal cells regardless of the CO2 concentrations.
Responses of CCMs in O. cordata Floating Leaves to Variable CO2
The pH-drift experiment confirmed the results reported by Cao et al. (2019), that floating leaves of O. cordata are able to use HCO3-. As a general trend, amphibious heterophyllous plants, in contrast to consistently submerged rooted macrophytes, are not able to use HCO3- for photosynthesis (Madsen and Sand-Jensen, 1991). However, clearly, O. cordata floating leaves exhibited substantial capacity for HCO3- utilization, irrespective of the CO2 concentrations during acclimation. Moreover, the HCO3- utilization efficiency was relatively similar as that in other truly submerged bicarbonate user, such as O. alismoides (Zhang et al., 2014; Shao et al., 2017). Considering the much higher concentration of free CO2 (380~1603 μM) in high CO2 acclimated conditions, the finding of relatively efficient HCO3- extraction in O. cordata floating leaves grown at high CO2 was also surprising, because at least some aquatic plants decrease investment in the capacity for HCO3- utilization when living in an environment with sufficient CO2 (Maberly and Spence, 1983; Madsen and Sand-Jensen, 1987). From a cost-benefit point of view, under high CO2 conditions, investment in a CO2-concentrating system could be expected not to be profitable since the benefit in terms of carbon gain will most likely be restricted (Maberly and Gontero, 2017). However, Madsen and Maberly (1991) reported that the HCO3- user Ranunculus peltatus still benefit significantly from HCO3- usage even at very high CO2 concentrations (greater than 200 μM). Overall, the patterns of inorganic carbon depletion in the pH-drift system confirm that the floating leaves of O. cordata are a constitutive bicarbonate user.
Based on the enzymatic activity analysis, O. cordata floating leaves have the carboxylating, PEP regenerating and decarboxylating enzymes that are needed for operating a C4 pathway. We firstly compared the activities of Rubisco, PEPC, and PPDK, there are hints of possible C4 metabolism exist in O. cordata floating leaves. Under both CO2 acclimation conditions, the activity of PEPC was greater than that of Rubisco and the ratio of PEPC to Rubisco was about 1.5 and 4 in low and high CO2 conditions, respectively. The ratio for O. cordata is lower than Hydrilla verticillata, but similar to those reported for O. alismoides, O. acuminata, and Egeria densa, all of which are regarded as C4 aquatic plants (Casati et al., 2000; Bowes, 2011; Shao et al., 2017). In contrast, the PEPC/Rubisco ratio in terrestrial C3 plants and aquatic plants that lack a biochemical concentrating mechanism is substantially less than 1 (Zhang et al., 2014). In addition, the activity of PPDK, that regenerates PEP to ensure the supply of substrate for PEPC, was significantly greater at low vs. high CO2 and equivalent to that of PEPC. Thus, PPDK should be able to support PEPC activity. As the potential decarboxylating enzymes, the NADP-ME activity was not significantly different at low vs. high CO2. Previous work has shown that H. verticillata (Magnin et al., 1997) and E. densa (Casati et al., 2000) belong to the NADP-ME C4-subtype. O. alismoides and O. acuminata are the first reports of aquatic plants that belong to the NAD-ME C4-subtype (Zhang et al., 2014).
C4 metabolism in some freshwater macrophytes is induced or up-regulated under low/limited CO2 conditions, such as in H. verticillata and E. densa (Casati et al., 2000; Bowes, 2011). In Eleocharis vivipara, the C4 cycle is present when its leaves are in air but absent when in water (Murphy et al., 2007). In the present study, it seems that the C4 cycle is not abolished in high CO2 acclimated O. cordata floating leaves, which implies that C4 is constitutive in O. cordata floating leaves, like the other known two constitutive C4 species in the genus Ottelia: O. alismoides and O. acuminata (Zhang et al., 2014; Shao et al., 2017; Han et al., 2020). C4 plants require structural and functional coordination to operate C4 efficiently. For H. verticillata, it performs single-cell C4 photosynthesis, where C4 production and decarboxylation occur in different parts of a cell (Bowes et al., 2002). For O. alismoides, a dual-cell C4 model was assumed, where the epidermal cells produce C4 product and the mesophyll cells decarboxylate the C4 product; however, it is also possible that both processes could occur within the mesophyll cells (Han et al., 2020). For O. cordata, further research is needed to establish the precise location of key photosynthetic enzymes involved in the primary CO2 fixation and decarboxylation, which could help to clarify the specific structural basis for C4 cycle, as well as to confirm the C4-subtype in this species.
Aquatic CAM metabolism was firstly found in the freshwater lycophyte Isoetes howellii (Keeley, 1981), and subsequently found in other freshwater angiosperms such as Crassula helmsii (Newman and Raven, 1995), Littorella uniflora (Robe and Griffiths, 2000), O. alismoides (Zhang et al., 2014) and Deinostema violaceum (Yin et al., 2017). In CAM plants, a high nocturnal PEPC activity allows malic acid to be produced in the dark. In O. cordata floating leaves, PEPC remains active at night and its nocturnal activity was very high. Under low CO2 condition, the PEPC : Rubisco ratio was ~2, which was consistent with nocturnal carboxylation as a consequence of CAM activity. In addition, the diel fluctuations of acidity have been considered to be an important indicator to estimate CAM activity (Keeley, 1998). In the present study, only low CO2 acclimated O. cordata floating leaves exhibited marked diurnal titratable acidity fluctuation, ~20 µequiv g-1 FW, which was slightly lower than that found in O. alismoides (up to 34 µequiv g-1 FW) (Zhang et al., 2014). It is also lower than Vallisneria americana and V. spiralis, which present evidence for CAM operation with diel acidity changes up to 42 and 51 µequiv g-1 FW, respectively (Keeley, 1998). Nevertheless, similar or lower values have been reported in a number of other putative freshwater CAM species (Webb et al., 1988; Keeley, 1998). In contrast, when grown at high CO2, O. cordata floating leaves showed a small but not statistically significant diel change in acidity, which indicates the absence of CAM. The field experiments performed by Cao et al. (2019), where the samples of O. cordata floating leaves were collected from their natural stands with the environmental CO2 concentration ~120 μM, showed the presence of CAM with 12.5 µequiv g-1 FW diel acidity change. This different performance of CAM activity between O. cordata floating leaves grown in situ and in laboratory cultures, might be the consequence of complex environmental variables in the field. CAM activity in aquatic plants, could be affected by a combination of factors including CO2 concentration, light intensity, temperature, as well as the leaf aging (Maberly and Gontero, 2017; Huang et al., 2018). Thus, the results presented here confirmed that CAM metabolism is present but facultative in O. cordata floating leaves.
Generally, malate is the primary product of CAM photosynthesis and presents substantial diel fluctuations (Keeley, 1998; Igamberdiev and Eprintsev, 2016). At night, the final C4 product malic acid is accumulated and stored in the vacuole; during day, malate comes out of the vacuole and is decarboxylated to produce CO2 (Borland and Taybi, 2004). In O. cordata floating leaves, we detected five types of organic acids and only malic acid revealed marked diurnal fluctuations at low CO2, the other four types of organic acid including citric acid, aspartic acid, trans-aconitic acid and fumaric acid were quite stable throughout the day. The significant decrease of malic acid during day suggests that it was most likely decarboxylated to produce CO2 for photosynthesis in O. cordata floating leaves when grown at low CO2, as it is in most of the aquatic CAM plants (Keeley, 1998). The nocturnal accumulation level of citric acid is similar with malic acid, however, the role of citrate accumulation in CAM plants is still unclear. Lüttge (1988) reported that CAM plants would transform a part of stored malate to citrate via tricarboxylic acid cycle (TCA cycle) to maintain their metabolic balance. Winter and Smith (1996) reported that citrate may serve to increase the pH-buffering capacity in the vacuoles of CAM plants, which would enhance accumulation of malate. The present data showed that the ratio of nocturnal citric acid accumulation to malic acid accumulation was 33% and 67% for high and low CO2 acclimated O. cordata floating leaves, respectively. In the CAM plant Euphorbia milii, citrate and malate accumulated equally (Herrera, 2013); whereas, Miszalski et al. (2013) found that in the CAM tree Clusia hilariana, malate and citrate experienced independent fluctuations, which were related to photosynthesis and respiration, respectively. In addition, in O. cordata floating leaves, aspartic acid and trans-aconitic acid presented much higher level than malic acid, but did not respond to CO2 availability, regardless of dusk and dawn. During metabolism the intensity of converting reactions among different carbon-compounds can cause preferential and higher accumulation of other organic acids than malic acid (Igamberdiev and Eprintsev, 2016). Moreover, aspartic acid content might be partially contributed by its derivative -asparagine, since these two amino acids are not separated quite well on the Athena C18-WP column.
Conclusions
We conclude that the anatomical structure and CCMs in O. cordata floating leaves exhibits responses to CO2 availability. High CO2 acclimation increased the size of upper epidermal cells, but decreased the area of chloroplast in epidermal cells. Under high CO2, O. cordata floating leaves are able to use HCO3- in addition to CO2 as an inorganic carbon source for photosynthesis, and can perform C4 metabolism. When exposed to low CO2, it also exhibits CAM cycling characteristics. Whether this species could perform other strategies under different CO2 conditions, such as the uptake of CO2 from sediment, is not known yet. Overall, the floating leaves of O. cordata present flexible and efficient ability to adjust structure and CCMs for utilization of inorganic carbon, allowing this species to form dense biomass and to be successfully distributed in shallow waters (He and Sun, 1990). Further studies are needed to establish the detailed biochemical pathway for C4 and CAM metabolism in this species, as well as the regulatory mechanism among the three CCMs and the exploitation strategies for exploiting atmospheric CO2, responding to the variable CO2 availability in the habitat.
Data Availability Statement
All datasets presented in this study are included in the article/supplementary material.
Author Contributions
WH and SH designed the experiments. WH, SH, and ZX performed the experiments and collected the data. WH, SH, and WL analyzed and prepared the manuscript. All authors contributed to the article and approved the submitted version.
Funding
This work was supported by the Strategic Priority Research Program of the Chinese Academy of Sciences (Grant No. XDB31000000) and the National Natural Science Foundation of China (Grant No. 31970368).
Conflict of Interest
The authors declare that the research was conducted in the absence of any commercial or financial relationships that could be construed as a potential conflict of interest.
Acknowledgments
We thank Hongsheng Jiang, Yu Cao, Yu Cheng, and Zhenwei Lu for assistance in the field sampling. We thank Dr. Brigitte Gontero and Dr. Stephen Maberly for valuable advices and language polishing during the preparation of the article. The authors are in debt to the Chinese Academy of Sciences (CAS) for the scholarship awarded to WH. We also thank reviewers and editors for valuable suggestions on the manuscript.
References
Ainsworth, E. A., Long, S. P. (2005). What have we learned from 15 years of free-air CO2 enrichment (FACE)? A meta-analytic review of the responses of photosynthesis, canopy properties and plant production to rising CO2. New Phytol. 165, 351–372. doi: 10.1111/j.1469-8137.2004.01224.x
Borland, A. M., Taybi, T. (2004). Synchronization of metabolic processes in plants with Crassulacean acid metabolism. J. Exp. Bot. 55, 1255–1265. doi: 10.1093/jxb/erh105
Bowes, G., Salvucci, M. E. (1989). Plasticity in the photosynthetic carbon metabolism of submersed aquatic macrophytes. Aquat. Bot. 34, 233–266. doi: 10.1016/0304-3770(89)90058-2
Bowes, G., Rao, S. K., Estavillo, G. M., Reiskind, J. B. (2002). C4 mechanisms in aquatic angiosperms: comparisons with terrestrial C4 systems. Funct. Plant Biol. 29, 379–392. doi: 10.1071/pp01219
Bowes, G. (2011). “Single-cell C4 photosynthesis in aquatic plants,” in C4 photosynthesis and related CO2 concentrating mechanisms. Eds. Raghavendra, A. S., Sage, R. F. (New York: Springer), 63–80.
Bray, S., Reid, D. M. (2002). The effect of salinity and CO2 enrichment on the growth and anatomy of the second trifoliate leaf of Phaseolus vulgaris. Can. J. Bot. 80, 349–359. doi: 10.1139/b02-018
Cao, Y., Liu, Y., Ndirangu, L., Li, W., Xian, L., Jiang, H. S. (2019). The analysis of leaf traits of eight Ottelia populations and their potential ecosystem functions in karst freshwaters in China. Front. Plant Sci. 9, 1938. doi: 10.3389/fpls.2018.01938
Casati, P., Lara, M. V., Andreo, C. S. (2000). Induction of a C4-like mechanism of CO2 fixation in Egeria densa, a submersed aquatic species. Plant Physiol. 123, 1611–1622. doi: 10.1104/pp.123.4.1611
Driscoll, S., Prins, A., Olmos, E., Kunert, K., Foyer, C. (2006). Specification of adaxial and abaxial stomata, epidermal structure and photosynthesis to CO2 enrichment in maize leaves. J. Exp. Bot. 57, 381–390. doi: 10.1093/jxb/erj030
Han, S., Maberly, S. C., Gontero, B., Xing, Z., Li, W., Jiang, H., et al. (2020). Structural basis for C4 photosynthesis without Kranz anatomy in leaves of the submerged freshwater plant Ottelia alismoides. Ann. Bot. 125, 8697–879. doi: 10.1093/aob/mcaa005
He, J., Sun, X. (1990). Ottelia emersa Z.C. Zhao et R.L. Luo (Hydrocharitaceae) - a Synonym of O. cordata (Wallich) Dandy. Aquat. Bot. 36, 395–398. doi: 10.1016/0304-3770(90)90056-q
Herrera, A. (2013). Crassulacean acid metabolism-cycling in Euphorbia milii. AoB Plants 5, plt014. doi: 10.1093/aobpla/plt014
Huang, W. M., Shao, H., Zhou, S. N., Zhou, Q., Fu, W. L., Zhang, T., et al. (2018). Different CO2 acclimation strategies in juvenile and mature leaves of Ottelia alismoides. Photosynth. Res. 138, 219–232. doi: 10.1007/s11120-018-0568-y
Hussner, A., Smith, R., Mettler-Altmann, T., Hill, M. P., Coetzee, J. (2019). Simulated global increases in atmospheric CO2 alter the tissue composition, but not the growth of some submerged aquatic plant bicarbonate users growing in DIC rich waters. Aquat. Bot. 153, 44–50. doi: 10.1016/j.aquabot.2018.11.009
Igamberdiev, A. U., Eprintsev, A. T. (2016). Organic acids: the pools of fixed carbon involved in redox regulation and energy balance in higher plants. Front. Plant Sci. 7, 1042. doi: 10.3389/fpls.2016.01042
IPCC (2014). “Climate Change. Synthesis Report”, in Contribution of Working Groups I, II and III to the Fifth Assessment Report of the Intergovernmental Panel on Climate Change, vol. 151 . Eds. Core Writing Team, Pachauri, R. K., Meyer, L. A. (Geneva, Switzerland: IPCC).
Iversen, L. L., Winkel, A., Baastrup-Spohr, L., Hinke, A. B., Alahuhta, J., Baattrup-Pedersen, A., et al. (2019). Catchment properties and the photosynthetic trait composition of freshwater plant communities. Science 366, 878–881. doi: 10.1126/science.aay5945
Johnson, V., Brownlee, C., Rickaby, R., Graziano, M., Milazzo, M., Hall-Spencer, J. (2013). Responses of marine benthic microalgae to elevated CO2. Mar. Biol. 160, 1813–1824. doi: 10.1007/s00227-011-1840-2
Keeley, J. E. (1981). Isoetes howellii: a submerged aquatic CAM plant? Am. J. Bot. 68, 420–424. doi: 10.1002/j.1537-2197.1981.tb06380.x
Keeley, J. E. (1998). CAM photosynthesis in submerged aquatic plants. Bot. Rev. 64, 121–175. doi: 10.1007/BF02856581
Klavsen, S. K., Maberly, S. C. (2010). Effect of light and CO2 on inorganic carbon uptake in the invasive aquatic CAM-plant Crassula helmsii. Funct. Plant Biol. 37, 737–747. doi: 10.1071/FP09281
Klavsen, S. K., Madsen, T. V., Maberly, S. C. (2011). Crassulacean acid metabolism in the context of other carbon-concentrating mechanisms in freshwater plants: a review. Photosynth. Res. 109, 269–279. doi: 10.1007/s11120-011-9630-8
Koch, M., Bowes, G., Ross, C., Zhang, X. H. (2013). Climate change and ocean acidification effects on seagrasses and marine macroalgae. Global Change Biol. 19, 103–132. doi: 10.1111/j.1365-2486.2012.02791.x
Li, X., He, D., Guo, Y. (2019). Morphological structure and physiological research of heterophylly in Potamogeton octandrus. Plant Syst. Evol. 305, 223–232. doi: 10.1007/s00606-019-1564-1
Li, Z. Z., Ngarega, B. K., Lehtonen, S., Gichira, A. W., Karichu, M. J., Wang, Q. F., et al. (2020). Cryptic diversity within the African aquatic plant Ottelia ulvifolia (Hydrocharitaceae) revealed by population genetic and phylogenetic analyses. J. Plant Res. 133, 373–381. doi: 10.1007/s10265-020-01175-2
Lin, B., Yang, W. (1999). Blue light and abscisic acid independently induce heterophyllous switch in Marsilea quadrifolia. Plant Physiol. 119, 429–434. doi: 10.1104/pp.119.2.429
Long, S. P., Ainsworth, E. A., Rogers, A., Ort, D. R. (2004). Rising atmospheric carbon dioxide: plants FACE the future. Annu. Rev. Plant Biol. 55, 591–628. doi: 10.1146/annurev.arplant.55.031903.141610
Lüttge, U. (1988). Day-night changes of citric-acid levels in crassulacean acid metabolism: phenomenon and ecophysiological significance. Plant Cell Environ. 11, 445–451. doi: 10.1111/j.1365-3040.1988.tb01782.x
Maberly, S. C., Gontero, B. (2017). Ecological imperatives for aquatic CO2-concentrating mechanisms. J. Exp. Bot. 68, 3797–3814. doi: 10.1093/jxb/erx201
Maberly, S. C., Gontero, B. (2018). “Trade-offs and synergies in the structural and functional characteristics of leaves photosynthesizing in aquatic environments,” in The Leaf: A Platform for Performing Photosynthesis. Eds. W.W.A, III, Terashima, I. (New York: Springer), 307–343.
Maberly, S., Madsen, T. (1998). Affinity for CO2 in relation to the ability of freshwater macrophytes to use HCO3–. Funct. Ecol. 12, 99–106. doi: 10.1046/j.1365-2435.1998.00172.x
Maberly, S. C., Madsen, T. V. (2002). Freshwater angiosperm carbon concentrating mechanisms: processes and patterns. Funct. Plant Biol. 29, 393–405. doi: 10.1071/pp01187
Maberly, S., Spence, D. (1983). Photosynthetic inorganic carbon use by freshwater plants. J. Ecol. 71, 705–724. doi: 10.2307/2259587
Maberly, S. C. (1996). Diel, episodic and seasonal changes in pH and concentrations of inorganic carbon in a productive lake. Freshwater Biol. 35, 579–598. doi: 10.1111/j.1365-2427.1996.tb01770.x
Madsen, T. V., Maberly, S. C. (1991). Diurnal variation in light and carbon limitation of photosynthesis by two species of submerged freshwater macrophyte with a differential ability to use bicarbonate. Freshwater Biol. 26, 175–187. doi: 10.1111/j.1365-2427.1991.tb01727.x
Madsen, T. V., Sand-Jensen, K. (1987). Photosynthetic capacity, bicarbonate affinity and growth of Elodea canadensis exposed to different concentrations of inorganic carbon. OIKOS 50, 176–182. doi: 10.2307/3565998
Madsen, T. V., Sand-Jensen, K. (1991). Photosynthetic carbon assimilation in aquatic macrophytes. Aquat. Bot. 41, 5–40. doi: 10.1016/0304-3770(91)90037-6
Madsen, T. V., Olesen, B., Bagger, J. (2002). Carbon acquisition and carbon dynamics by aquatic isoetids. Aquat. Bot. 73, 351–371. doi: 10.1016/S0304-3770(02)00030-X
Magnin, N. C., Cooley, B. A., Reiskind, J. B., Bowes, G. (1997). Regulation and localization of key enzymes during the induction of Kranz-less, C4-type photosynthesis in Hydrilla verticillata. Plant Physiol. 115, 1681–1689. doi: 10.1104/pp.115.4.1681
Miszalski, Z., Kornas, A., Rozpądek, P., Fischer-Schliebs, E., Lüttge, U. (2013). Independent fluctuations of malate and citrate in the CAM species Clusia hilariana Schltdl. under low light and high light in relation to photoprotection. J. Plant Physiol. 170, 453–458. doi: 10.1016/j.jplph.2012.11.005
Murphy, L. R., Barroca, J., Franceschi, V. R., Lee, R., Roalson, E. H., Edwards, G. E., et al. (2007). Diversity and plasticity of C4 photosynthesis in Eleocharis (Cyperaceae). Funct. Plant Biol. 34, 571–580. doi: 10.1071/fp06296
Newman, J. R., Raven, J. A. (1995). Photosynthetic carbon assimilation by Crassula helmsii. Oecologia 101, 494–499. doi: 10.1007/BF00329429
Pedersen, O., Rich, S. M., Pulido, C., Cawthray, G. R., Colmer, T. D. (2011). Crassulacean acid metabolism enhances underwater photosynthesis and diminishes photorespiration in the aquatic plant Isoetes australis. New Phytol. 190, 332–339. doi: 10.1111/j.1469-8137.2010.03522.x
Pedersen, O., Colmer, T. D., Sand-Jensen, K. (2013). Underwater photosynthesis of submerged plants–recent advances and methods. Front. Plant Sci. 4:140. doi: 10.3389/fpls.2013.00140
Pritchard, S. G., Rogers, H. H., Prior, S. A., Peterson, C. M. (1999). Elevated CO2 and plant structure: a review. Global. Change Biol. 5, 807–837. doi: 10.1046/j.1365-2486.1999.00268.x
Radoglou, K., Jarvis, P. (1990). Effects of CO2 enrichment on four poplar clones. I. Growth and leaf anatomy. Ann. Bot. 65, 617–626. doi: 10.1093/oxfordjournals.aob.a087978
Radoglou, K., Jarvis, P. (1992). The effects of CO2 enrichment and nutrient supply on growth morphology and anatomy of Phaseolus vulgaris L. seedlings. Ann. Bot. 70, 245–256. doi: 10.1093/oxfordjournals.aob.a088466
Robe, W., Griffiths, H. (2000). Physiological and photosynthetic plasticity in the amphibious, freshwater plant, Littorella uniflora, during the transition from aquatic to dry terrestrial environments. Plant Cell Environ. 23, 1041–1054. doi: 10.1046/j.1365-3040.2000.00615.x
Rudall, P. J., Knowles, E. V. (2013). Ultrastructure of stomatal development in early-divergent angiosperms reveals contrasting patterning and pre-patterning. Ann. Bot. 112, 1031–1043. doi: 10.1093/aob/mct169
Sand-Jensen, K., Frost-Christensen, H. (1998). Photosynthesis of amphibious and obligately submerged plants in CO2-rich lowland streams. Oecologia 117, 31–39. doi: 10.1007/s004420050628
Sand-Jensen, K., Pedersen, M. F., Nielsen, S. L. (1992). Photosynthetic use of inorganic carbon among primary and secondary water plants in streams. Freshwater Biol. 27, 283–293. doi: 10.1111/j.1365-2427.1992.tb00540.x
Schippers, P., Mooij, W. M., Vermaat, J. E., de Klein, J. (2004). The effect of atmospheric carbon dioxide elevation on plant growth in freshwater ecosystems. Ecosystems 7, 63–74. doi: 10.1007/s10021-003-0195-z
Shao, H., Gontero, B., Maberly, S. C., Jiang, H. S., Cao, Y., Li, W., et al. (2017). Responses of Ottelia alismoides, an aquatic plant with three CCMs, to variable CO2 and light. J. Exp. Bot. 68, 3985–3995. doi: 10.1093/jxb/erx064
Thomas, J. F., Harvey, C. N. (1983). Leaf anatomy of four species grown under continuous CO2 enrichment. Bot. Gaz. 144, 303–309. doi: 10.1086/337377
Uprety, D., Dwivedi, N., Mohan, R., Paswan, G. (2001). Effect of elevated CO2 concentration on leaf structure of Brassica juncea under water stress. Biol. Plantarum 44, 149–152. doi: 10.1023/A:1017959429783
Wang, H. X., Guo, J. L., Li, Z. M., Yu, Y. H., Zhang, Y. H. (2019). Characterization of the complete chloroplast genome of an endangered aquatic macrophyte, Ottelia cordata (Hydrocharitaceae). Mitochondrial DNA B 4, 1839–1840. doi: 10.1080/23802359.2019.1612719
Watling, J. R., Press, M. C., Quick, W. P. (2000). Elevated CO2 induces biochemical and ultrastructural changes in leaves of the C4cereal sorghum. Plant Physiol. 123, 1143–1152. doi: 10.1104/pp.123.3.1143
Webb, D., Rattray, M., Brown, J. (1988). A preliminary survey for crassulacean acid metabolism (CAM) in submerged aquatic macrophytes in New Zealand. New Zeal. J. Mar. Fresh. 22, 231–235. doi: 10.1080/00288330.1988.9516295
Winter, K., Smith, J. (1996). “Crassulacean acid metabolism: current status and perspectives,” in Crassulacean Acid Metabolism, Biochemistry, Ecophysiology and Evolution. Eds. Winter, K., Smith, J. A. C. (New York: Springer), 389–426.
Yin, L., Li, W., Madsen, T. V., Maberly, S. C., Bowes, G. (2017). Photosynthetic inorganic carbon acquisition in 30 freshwater macrophytes. Aquat. Bot. 140, 48–54. doi: 10.1016/j.aquabot.2016.05.002
Keywords: CO2 availability, C4, crassulacean acid metabolism, bicarbonate use, CO2 concentrating mechanisms, organic acids
Citation: Huang W, Han S, Xing Z and Li W (2020) Responses of Leaf Anatomy and CO2 Concentrating Mechanisms of the Aquatic Plant Ottelia cordata to Variable CO2. Front. Plant Sci. 11:1261. doi: 10.3389/fpls.2020.01261
Received: 04 May 2020; Accepted: 31 July 2020;
Published: 14 August 2020.
Edited by:
Reimund Goss, Leipzig University, GermanyReviewed by:
Matheus Bianconi, The University of Sheffield, United KingdomRoxana Khoshravesh, University of New Mexico, United States
Copyright © 2020 Huang, Han, Xing and Li. This is an open-access article distributed under the terms of the Creative Commons Attribution License (CC BY). The use, distribution or reproduction in other forums is permitted, provided the original author(s) and the copyright owner(s) are credited and that the original publication in this journal is cited, in accordance with accepted academic practice. No use, distribution or reproduction is permitted which does not comply with these terms.
*Correspondence: Wei Li, bGl3ZWlAd2JnY2FzLmNu
†These authors have contributed equally to this work