- 1Department of Soil, Plant and Environmental Quality, Institute of Agricultural Sciences, ICA-CSIC, Madrid, Spain
- 2Centro Nacional de Biotecnología, CNB-CSIC, Madrid, Spain
- 3Brookhaven National Laboratory, Upton, NY, United States
- 4Centro de Estudios Avanzados en Zonas Áridas, CEAZA, La Serena, Chile
Mercury (Hg) is extremely toxic for all living organisms. Hg-tolerant symbiotic rhizobia have the potential to increase legume tolerance, and to our knowledge, the mechanisms underlying Hg tolerance in rhizobia have not been investigated to date. Rhizobial strains of Ensifer medicae, Rhizobium leguminosarum bv. trifolii and Bradyrhizobium canariense previously isolated from severely Hg-contaminated soils showed different levels of Hg tolerance. The ability of the strains to reduce mercury Hg2+ to Hg0, a volatile and less toxic form of mercury, was assessed using a Hg volatilization assay. In general, tolerant strains displayed high mercuric reductase activity, which appeared to be inducible in some strains when grown at a sub-lethal HgCl2 concentration. A strong correlation between Hg tolerance and mercuric reductase activity was observed for E. medicae strains, whereas this was not the case for the B. canariense strains, suggesting that additional Hg tolerance mechanisms could be playing a role in B. canariense. Transcript abundance from merA, the gene that encodes mercuric reductase, was quantified in tolerant and sensitive E. medicae and R. leguminosarum strains. Tolerant strains presented higher merA expression than sensitive ones, and an increase in transcript abundance was observed for some strains when bacteria were grown in the presence of a sub-lethal HgCl2 concentration. These results suggest a regulation of mercuric reductase in rhizobia. Expression of merA genes and mercuric reductase activity were confirmed in Medicago truncatula nodules formed by a sensitive or a tolerant E. medicae strain. Transcript accumulation in nodules formed by the tolerant strain increased when Hg stress was applied, while a significant decrease in expression occurred upon stress application in nodules formed by the Hg-sensitive strain. The effect of Hg stress on nitrogen fixation was evaluated, and in our experimental conditions, nitrogenase activity was not affected in nodules formed by the tolerant strain, while a significant decrease in activity was observed in nodules elicited by the Hg-sensitive bacteria. Our results suggest that the combination of tolerant legumes with tolerant rhizobia constitutes a potentially powerful tool in the bioremediation of Hg-contaminated soils.
Introduction
Mercury is the most toxic heavy metal, and mercury contamination is becoming a major problem worldwide, in both wild ecosystems and agricultural soils. Environmental mercury contamination can be due to natural processes (volcanism, soil erosion, geothermal activities, and wild fires), but is mostly the result of anthropogenic activities, such as mining or the increasing use of phytochemicals in agriculture (Dash and Das, 2012; Wu et al., 2014; Paris et al., 2015; Marx et al., 2016). The most prevalent forms of mercury in the environment and in living organisms are metallic mercury (Hg0), mercuric mercury (Hg2+), and methylmercury (CH3Hg+), which is formed as a result of the methylation of Hg2+ by some microorganisms found in soil and water (Dash and Das, 2012; Tchounwou et al., 2012; Naguib et al., 2018). All forms of Hg are toxic, but Hg0 is volatile and the least toxic mercury state (Barkay et al., 2003). The toxicity of Hg and other heavy metals is due to complex interactions with cellular macromolecules (Azevedo and Rodriguez, 2012). In bacteria, plants and other organisms, the presence of metals might cause the formation of free radicals, generating oxidative stress (Mudipalli, 2008), and replacement of essential metals in pigments or enzymes, thus affecting their optimal activity (Malayeri et al., 2008; Mathema et al., 2011). Mercury appears to enter in both prokaryotic and eukaryotic cells through ion channels, competing with essential metals such as copper, iron, or zinc (Blazka and Shaikh, 1992; Bridges and Zalups, 2005). It has a high affinity for the sulfhydryl groups of amino acids, causing the alteration and loss of function of enzymes and sulfur-containing antioxidants (Patra and Sharma, 2000; Nies, 2003). Consequently, high bioaccumulation of mercury presents considerable problems in plants and animals, and results in prominent biomagnification in the trophic chain (David et al., 2012; Lavoie et al., 2013).
Some microorganisms have developed different strategies to protect themselves against mercury, such as extrusion by ATPases, cation antiport systems, immobilization with anionic ligands or precipitation of colloids (Robinson and Tuovinen, 1984; Osborn et al., 1997; Naguib et al., 2018). A common strategy to many bacteria is the enzymatic reduction of Hg2+ to less toxic and volatile Hg0, which is mediated by the bacterial mercuric reductase enzyme (MerA) encoded by the merA gene, which is usually part of the mer operon that contains several genes involved in mercury detoxification. Operon components and organization may be different in different bacteria (Mathema et al., 2011; Boyd and Barkay, 2012; Freedman et al., 2012; Naguib et al., 2018).
Mercury-tolerant microorganisms capable of detoxifying mercury have a potential for bioremediation of mercury-contaminated water and soil (Barkay et al., 2003). Therefore, the identification of tolerant, mercury-detoxifying bacteria is highly relevant. Deficiencies in nutrients, such as nitrogen, are the main limiting factors in the revegetation and/or phytoremediation of poor, marginal and polluted soils. Rhizobia are gram-negative soil bacteria that fix atmospheric nitrogen in the symbiotic root nodules that they form with leguminous plants. Once bacteria are released inside the host plant cell, they differentiate into bacteroids, the nitrogen-fixing form. Both host plant and rhizobia benefit from this interaction because the plant obtains nitrogen as ammonia (NH3), which is rapidly protonated to ammonium (NH4+), while the bacteria receive carbon substrates (succinate and malate) as a way to rapidly obtain energy (Udvardi and Poole, 2013). This is why the use of rhizobia-legume symbioses has been proposed to be a useful tool for the bioremediation of nutrient-depleted heavy metal-contaminated soils (Hao et al., 2014). The symbiotic system has actually been successfully used in soils contaminated with different heavy metals (Dary et al., 2010; Fagorzi et al., 2018; Bellabarba et al., 2019). Thus, the identification of Hg-tolerant rhizobia strains capable of detoxifying mercury has become of great interest to be used in conjunction with tolerant legumes in Hg-polluted soils.
One of the largest deposits of mercury in the world is the Almadén mine district in Central Spain, where the primary mineral is cinnabar (mercury sulfide). Centuries of mining caused the dispersion of the metal in the environment surrounding Almadén, leading to elevated mercury concentrations in soils and plants (Martínez-Coronado et al., 2011; Lominchar et al., 2015). To our knowledge, the tolerance and detoxification mechanisms present in Hg-resistant rhizobia have not yet been described. The aim of this study was to analyze rhizobial strains collected from Hg-contaminated soils of Almadén, which presented different tolerance to Hg, in order to identify whether mercury tolerance was due to their capacity to reduce Hg2+ to Hg0. Bacteria strains of Ensifer (formerly Sinorhizobium) medicae, Rhizobium leguminosarum bv. trifolii, and Bradyrhizobium canariense were previously isolated from nodules in host plants growing in Hg-contaminated soils (Nonnoi et al., 2012; Ruiz-Díez et al., 2012, 2013). We hypothesize that significant differences in mercury reductase activity will be detected between strains having low vs. high mercury tolerance in free living conditions and that the enzyme might be active and provide a protective effect on nitrogen fixation in legume nodules in the presence of Hg stress.
Materials and Methods
Bacteria and Growth Media
Bacteria used in this study were previously isolated from legume nodules that were grown in Hg-contaminated soil (see Nonnoi et al., 2012; Ruiz-Díez et al., 2012, 2013 for specific information regarding each strain). Twenty-one R. leguminosarum bv. trifolii strains and 23 Ensifer medicae strains were isolated from nodules of Trifolium and Medicago species, respectively, which were growing in different sites near the abandoned Almadén mercury mine in Spain. Seven B. canariense strains were isolated from nodules of Lupinus, Spartium, Adenocarpus, and Ornithopus species collected from the Almadén area or from non-contaminated sites. E. medicae and R. leguminosarum strains were grown in Tryptone Yeast (TY) medium, and B. canariense strains were grown in Vincent medium (Vincent, 1970).
Rhizobial Tolerance to Mercury: Minimum Inhibitory Concentration
In all experiments described in this work, HgCl2 was used as the source of Hg. Hereafter, Hg, Hg tolerance, Hg-tolerant, Hg-sensitive, Hg stress, Hg concentration always refers to HgCl2. Rhizobial strains were grown in liquid medium at 28°C until an OD600 of 0.7. To determine the lowest HgCl2 concentration that completely inhibits bacterial growth, minimum inhibitory concentration (MIC), 2 μl of bacterial culture was inoculated on agar solid medium with increasing HgCl2 concentrations: 25, 50, 75, 100, 125, 150, 175, 200, 225, 250, and 275 μM (Nonnoi et al., 2012). The inoculated plates were incubated at 28°C. E. medicae and R. leguminosarum are fast-growing rhizobia that, in our experimental conditions, showed visible growth after 2 d when grown in the absence of HgCl2. B. canariense is a slow-growing rhizobium and growth was not evident before 7 d. Thus, MIC was determined after 2 d for E. medicae and R. leguminosarum, and after 7 d for B. canariense.
Mercury Volatilization Assay
The ability of rhizobia to reduce Hg2+ to Hg0 was analyzed by an X-ray film method that detects mercury volatilization. When Hg0 is generated, mercury vapor reacts with silver (Ag+) contained in the X-ray film, it shows a dark spot (Nakamura and Nakahara, 1988). Rhizobia strains were grown in liquid medium in the absence of HgCl2 or a concentration of 4 μM HgCl2 in order to determine an induction of mercuric reductase activity by mercury. Cultures were grown at 28°C until the concentration reached an OD600 of 0.7. Then, 4 ml of each culture were centrifuged at 2,000 × g for 15 min at 20°C. The pellet was resuspended in 50 μl of reaction medium that contained 50 μM HgCl2 (Nakamura and Nakahara, 1988). Controls contained the reaction medium without bacteria. Reaction mixtures were loaded onto a multi-well plate, and an X-ray film was placed on the plate in the dark (Nakamura and Nakahara, 1988). Films were developed after 4 h. Reaction medium without bacteria was used as a negative control. A densitometry analysis of the X-ray films was performed using the ImageJ software.
Transcriptional Analyses of Mercuric Reductases
E. medicae strains AMp08 (tolerant) and VMo01 (sensitive) and R. leguminosarum strains STf07 (tolerant) and VTc11 (sensitive) were grown in liquid TY medium in the absence or presence of mercury (4 μM HgCl2). When cultures reached an OD600 of 0.8, we directly added the Qiagen RNAprotect Bacteria Reagent to the bacterial culture in a 1:2 ratio (culture: RNA stabilizer). The mixtures were vortexed for 5 s and incubated for 5 min at room temperature (23°C) and then centrifuged at 5,000 × g for 10 min at 20°C. Pellets were frozen in liquid nitrogen. Bacterial lysis was performed by resuspension and incubation of the cell pellet in 1 mg/ml lysozyme from chicken egg whites (Sigma-Aldrich) in Tris–EDTA buffer, pH 8.0. Total RNA was extracted using the Qiagen RNeasy Mini Kit. For gene expression in bacteroids, nodules of M. truncatula were frozen in liquid nitrogen and the tissue was ground with using the MM40 (Retsch) mixer mill. RNA was extracted using the Qiagen RNeasy Plant Mini Kit according to the manufacturer’s protocol. The isolated RNA was subjected to DNase treatment. The quality and the quantity of the extracts were firstly analyzed with a NanoDrop 1,000 spectrophotometer (Thermo Scientific), and subsequently, RNA quality was assessed with an Agilent 2,100 Bioanalyzer.
For quantitative real time PCR (qPCR), reverse transcription was performed using the High-Capacity cDNA Reverse Transcription Kit (Applied Biosystems). Reactions were performed in a 7300 Real Time PCR System (Applied Biosystem). Each qPCR reaction contained 7.5 μl of SYBR Green PCR master mix (PE Applied Biosystem), 5 μl of cDNA, and 10 ng/μl of each primer in a final volume of 15 μl. Minus-reverse transcriptase (–RT) controls of the RNA samples were included in real-time RT-PCR experiments, in order to detect and estimate genomic DNA contamination; only samples free of genomic DNA contamination were included. Primers were designed using Primer Express Software v3.0 (PE Applied Biosystems). Primers used to amplify E. medicae merA1 and merA2 genes and R. leguminosarum merA2 are listed in Table 1. Genes encoding 16S rRNA genes were used as endogenous controls. Thermocycling conditions were as follows: an initial denaturing time of 10 min at 95°C, followed by 40 PCR cycles consisting of 95°C for 15 s and 60°C for 1 min. The data were analyzed using the 7,300 System Software (Applied Biosystems). And the relative quantification was carried out according to the comparative CT method (Pfaffl, 2001), as described in the Applied Biosystems StepOneTM Real-Time PCR System. Three independent biological experiments were performed with three technical replicates per sample. Each nodule sample contained nodules pooled from 4–6 plants. Differences were considered significant when the fold-change was ≥2 and SD bars did not overlap.
Phylogenetic Analysis
Alignment and phylogenetic analysis of mercuric reductase (MerA) protein sequences of selected rhizobia were performed using the Geneious 6.01 software (Kearse et al., 2012). The neighbor-joining method (Saitou and Nei, 1987) was applied to construct a phylogenetic tree. The evolutionary distances were computed with the Jukes-Cantor genetic distance amino acid substitution model. Branch support was generated using bootstrapping of 100 resampled trees.
Plant Material, Inoculation, Growth Conditions, and Mercury Stress
Medicago truncatula cv. Parabinga plants were used in this study. M. truncatula seeds were scarified with 96% sulfuric acid for 6 min, and then immersed in commercial bleach for 1 min, washed 6 times with sterile distilled water and left in water for 1 h. Sterilized seeds germinated overnight on Petri dishes containing 1% agar/water. Germinated seeds were inoculated during sowing with 1 ml of bacteria grown to exponential phase (OD600 = 0.8). Two E. medicae strains were used: AMp08, the strain that displayed the highest mercury tolerance, and VMo01, a sensitive strain that showed an increase in mercuric reductase activity when grown at a sub-lethal mercury concentration (4 μM HgCl2).
Plants were grown in pots filled with vermiculite in a growth chamber (25/19°C, 16/8 h photoperiod) and irrigated with nitrogen-free nutrient solution (Hoagland and Arnon, 1938). After 5 weeks, plants were watered with nutrient solution containing 0 mM or 500 μM HgCl2. Plant material was collected 24 h after treatment application.
Nitrogenase Activity
Nitrogen fixation activity was estimated by the acetylene reduction assay (ARA), as described by Shvaleva et al. (2010). Although the use of a “closed” system for measuring acetylene reduction can lead to an acetylene-induced decline in nitrogenase activity, it is appropriate for comparative measurements, especially when assay time is short. Nodulated roots were introduced in 15.5 ml tubes and 10% of volume was replaced by acetylene. After 1 h of incubation, gas samples were taken and analyzed for ethylene content using a gas chromatographer (Perkin–Elmer 8310, United States) using nitrogen as a carrier gas. We took into account the volume of the nodulated roots after measurements were taken; nodules were detached and weighed to calculate the nitrogenase activity per gram of nodule. Three independent biological experiments were performed. In each experiment, 7–10 plants per treatment were analyzed. Statistics analysis were performed with IBM SPSS Statistics 25 (SPSS Inc., Chicago, IL, United States), using a mixed linear model to analyze the effect of Hg on the nitrogenase activity (p < 0.05).
Results
Mercury Tolerance and Mercuric Reductase Activity in Free-Living Rhizobia
Mercury tolerance of rhizobia strains was tested on solid medium by measuring MIC following treatment with Hg (Table 2). Four E. medicae strains (AMp08, AMp10, NMp01, y SMp01) and three R. leguminosarum strains (STf07, STf08, and STf09) are considered Hg-tolerant (Nieto et al., 1989) as their growth was not inhibited in 0.1 mM Hg (MIC > 100 μM). All B. canariense strains were sensitive to mercury. L7-AH was the most Hg-tolerant B. canariense strain (MIC = 50 μM), while ISLU-16 and L-3, which were obtained from non-contaminated sites, were the most Hg-sensitive strains. The MIC values obtained for B. canariense in solid medium were higher than those previously reported, which had been measured in liquid medium (Ruiz-Díez et al., 2012, 2013). Some possible reasons that might explain the observed differences are considered below in the Discussion section.
The X-ray film assays revealed the ability of the strains to reduce Hg2+ to more volatile Hg0. Mercuric reductase activity values were obtained from densitometry analyses of the X-ray films. In general E. medicae and R. leguminosarum tolerant strains showed high mercuric reductase activities. Additionally, many of the strains showed increased mercuric reductase activity when they were grown in the presence of a low mercury concentration. The mercuric reductase activity assays performed with B. canariense strains showed that some strains displayed mercuric reductase activity. In some cases, activity increased when the strains had been cultivated in the presence of 4 μM HgCl2 (Table 2). Strain SP-7C1, which presented the highest mercury reductase activity, was not the most tolerant strain. Strains ISLU-16, L-3, and L-7Q displayed very low reductase activity. Moreover, their growth in liquid medium was completely inhibited by 4 μM Hg, in agreement with the MIC values previously reported (Ruiz-Díez et al., 2012, 2013).
In order to estimate the association of mercury tolerance of the rhizobial strains with their mercuric reductase activity, we performed a densitometry analysis of the rows in the X-ray films corresponding to the bacteria grown without mercury (rows 1 and 3). The densitometry values were plotted against the MIC values for E. medicae (Figure 1A), R. leguminosarum bv. trifolii (Figure 1B) and B. canariense (Figure 1C) strains. A strong correlation (R2 = 0.83) was observed in the case of E. medicae, while no correlation was found for the B. canariense strains (R2 = 0.41). We considered that the R2 value for R. leguminosarum might not be reliable, as there were only three strains that presented some degree of tolerance. It should also be noted that the X-ray film assay is a semi-quantitative assay, and consequently, the activity values obtained by densitometry analysis should be considered to be approximations.
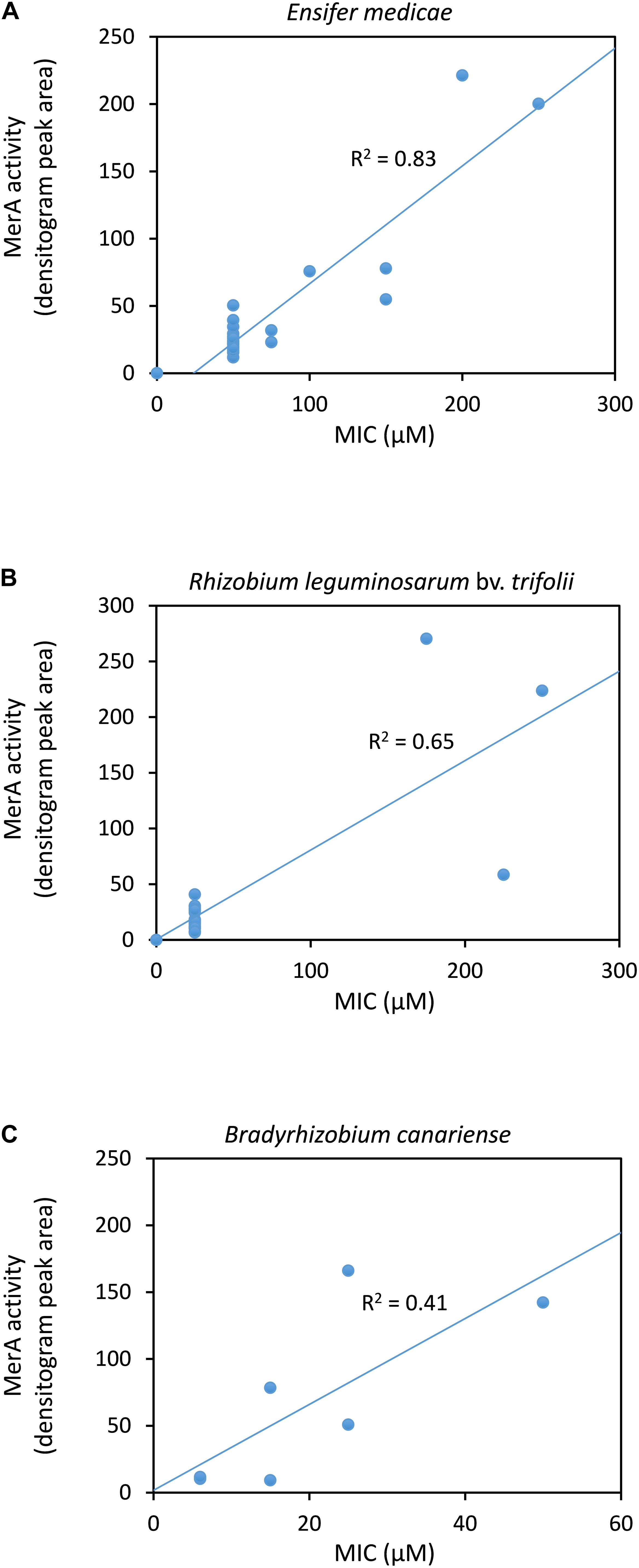
Figure 1. Correlation between mercuric reductase activity and mercury tolerance of rhizobial strains. (A) Ensifer medicae. (B) Rhizobium leguminosarum bv. trifolii. (C) Bradyrhizobium canariense. Mercuric reductase values were obtained from the densitometry analyses of the X-ray films. The coefficients of determination R2 are indicated.
Expression of merA Genes in Free-Living Rhizobia
Our assays indicated that mercuric reductase activity was induced in some strains when grown in sublethal mercury concentrations (4 μM HgCl2). Two E. medicae strains, VMo01 (sensitive) and Amp08 (tolerant), and two R. leguminosarum strains, VTc11 (sensitive), and STf07 (tolerant) were used to determine whether the differences in activity between strains and the mercury-induced increase in activity observed corresponded with merA gene expression levels. Sequenced E. medicae WSM419 strain has two mercuric reductase genes, one located on the chromosome (merA1) and another one on the symbiotic plasmid pSymB (merA2). R. leguminosarum WSM1325 has only one mercuric reductase gene (merA2) located on plasmid pR132502. To compare the expression levels of the merA genes in the different strains grown in the absence or presence of a sub-lethal mercury concentration, qPCR was performed. In E. medicae, merA1 expression was higher in the tolerant strain (AMp08) than in the sensitive strain (VMo01) when grown in the absence of HgCl2 (Figure 2A). The tolerant strain did not show a significant increase in expression when grown in the presence of HgCl2. In contrast, the expression of the merA1 gene significantly increased when the sensitive strain was cultivated in the presence of HgCl2. It appeared that induction ocurred due to the presence of the metal in the growth medium. E. medicae merA2 expression patterns were similar to those of merA1 (Figure 2B). In the absence of Hg, expression was higher in the tolerant strain (AMp08) and a significant induction occurred when the sensitive strain (VMo01) was grown in the presence of mercury. Expression of R. leguminosarum merA2 gene was higher in the tolerant strain (STf07) than in the sensitive strain (VTc11). There was an increase in transcript accumulation in both strains when they were grown in the presence of mercury (Figure 2C).
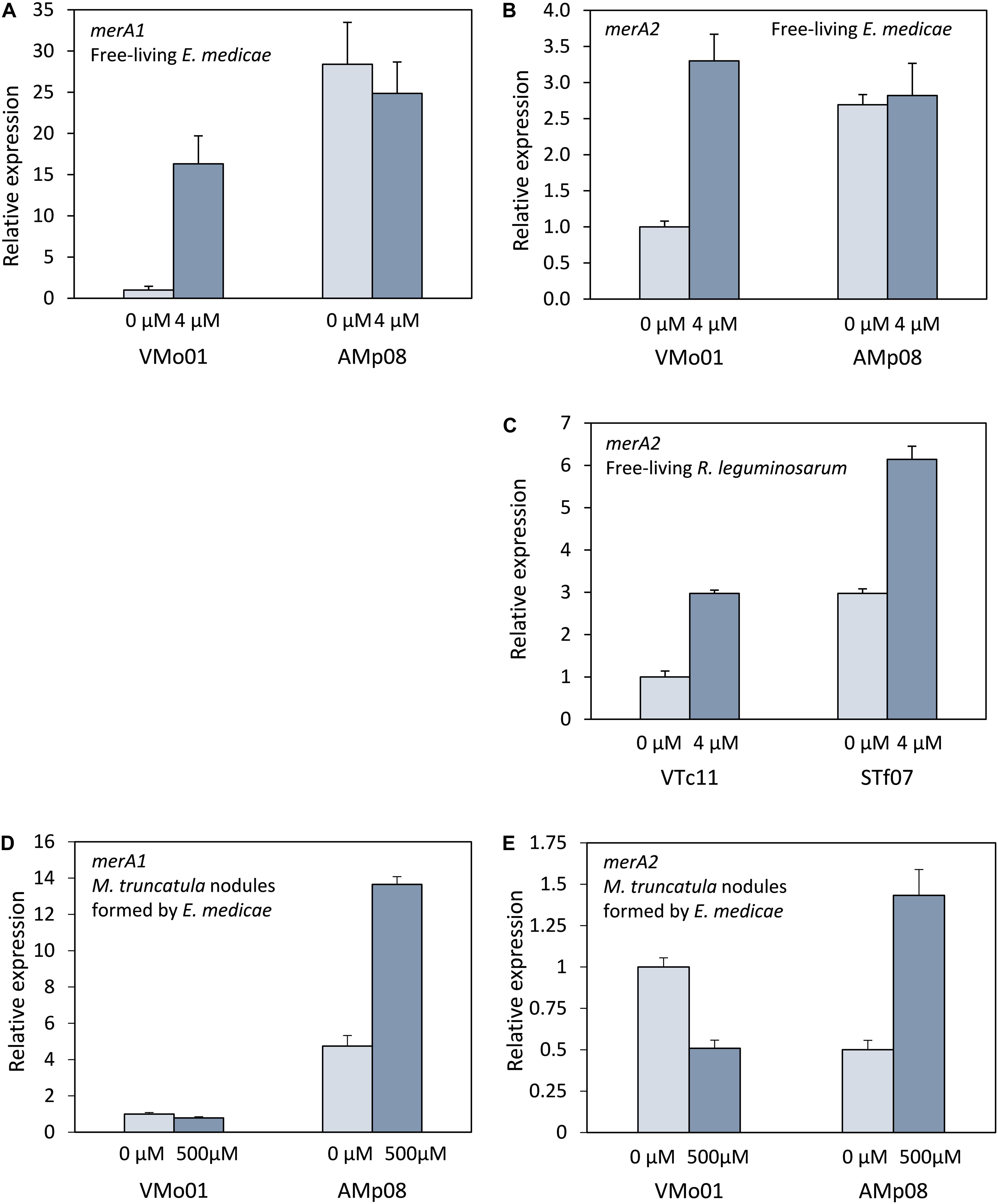
Figure 2. Expression of merA in free-living rhizobia and in Medicago truncatula nodules. (A) merA1 expression in free-living E. medicae strains VMo01 (sensitive) and AMp08 (tolerant). (B) merA2 expression in free-living E. medicae strains VMo01 and AMp08. (C) merA2 expression in free-living R. leguminosarum bv. homologs were identified in some species trifolii strains VTc11 (sensitive) and STf07 (tolerant). (D) merA1 expression in M. truncatula nodules formed by E. medicae strains VMo01 or AMp08. (E) merA2 expression in M. truncatula nodules formed by E. medicae strains VMo01 or AMp08. Free-living bacteria were grown in the absence (0 μM) or presence of 4 μM HgCl2. Three independent biological experiments were performed. Five week-old nodulated M. truncatula plants were watered with nutrient solution containing 0 or 500 μM HgCl2 and harvested after 24 h. Three independent biological experiments were performed. Each biological sample contained nodules pooled from 4–6 plants. Three technical replicates per sample were performed. Mean values ± SD are represented. Differences were considered significant when fold change was ≥2 and SD bars did not overlap.
merA Genes Expression, Mercuric Reductase Activity and Nitrogen Fixation in M. truncatula Nodules
The symbiotic interaction of rhizobia with legume roots leads to the formation of a new plant organ, the root nodule. Inside the nodule, bacteria differentiate into bacteroids, which are able to fix atmospheric nitrogen. In this process, some bacterial genes are inactivated, while others are overexpressed. Inactivation of the merA genes in bacteroids would imply the absence of mercuric reductase in the nodule and thus, the loss of an important mercury detoxification mechanism that could increase the plant tolerance to the metal. M. truncatula plants were inoculated with either E. medicae VMo01 (sensitive) or Amp08 (tolerant) strains, and after 5 weeks, when nodules are mature and active in nitrogen fixation, mercury stress was applied. We performed qPCR to check whether merA1 and merA2 were expressed in bacteroids, and to detect any differences in expression between the rhizobial strains or the mercury treatment. Both merA genes were expressed in the nodule. Similar to what we had observed for free living bacteria, merA1 expression (Figure 2D) was higher in the tolerant strain than in the sensitive one. In contrast, the tolerant strain showed an induction when mercury stress was applied, whereas the sensitive strain did not show any induction. In control (untreated with Hg) nodules, merA2 expression was somewhat higher in the sensitive strain. While mercury stress led to a decrease in merA2 expression in the sensitive strain, the tolerant strain showed an induction of gene expression (Figure 2E). Although the conditions were not comparable, these results contrast with the expression in free-living bacteria, where the tolerant strain showed a higher expression but there was not an induction, whereas the sensitive strain showed an induction when grown in the presence of HgCl2. The merA transcripts in the bacteroids were translated into active mercuric reductase, as activity could be detected in crushed nodules (data not shown). However, the mercuric reductase assay was not suitable to quantify differences in activity between strains, or between control and mercury-stressed nodules. The assay results were not reproducible most likely due to heterogeneity of the crushed nodule tissue.
Nitrogenase activity was analyzed in nodules of M. truncatula plants inoculated with E. medicae strains VMo01 (sensitive) or AMp08 (tolerant) (Figure 3). The symbiotic performance of plants inoculated with the sensitive or the tolerant strains was similar, as there were no significant differences in nitrogenase activity in non-stressed plants. Additionally, no significant differences were observed in root and shoot fresh weight due to the inoculated strain (data not shown). Nodules formed by the sensitive strain showed a significant decrease in nitrogenase activity (66%) when mercury stress was applied compared to control nodules, while no significant differences were observed for plants inoculated with the tolerant strain.
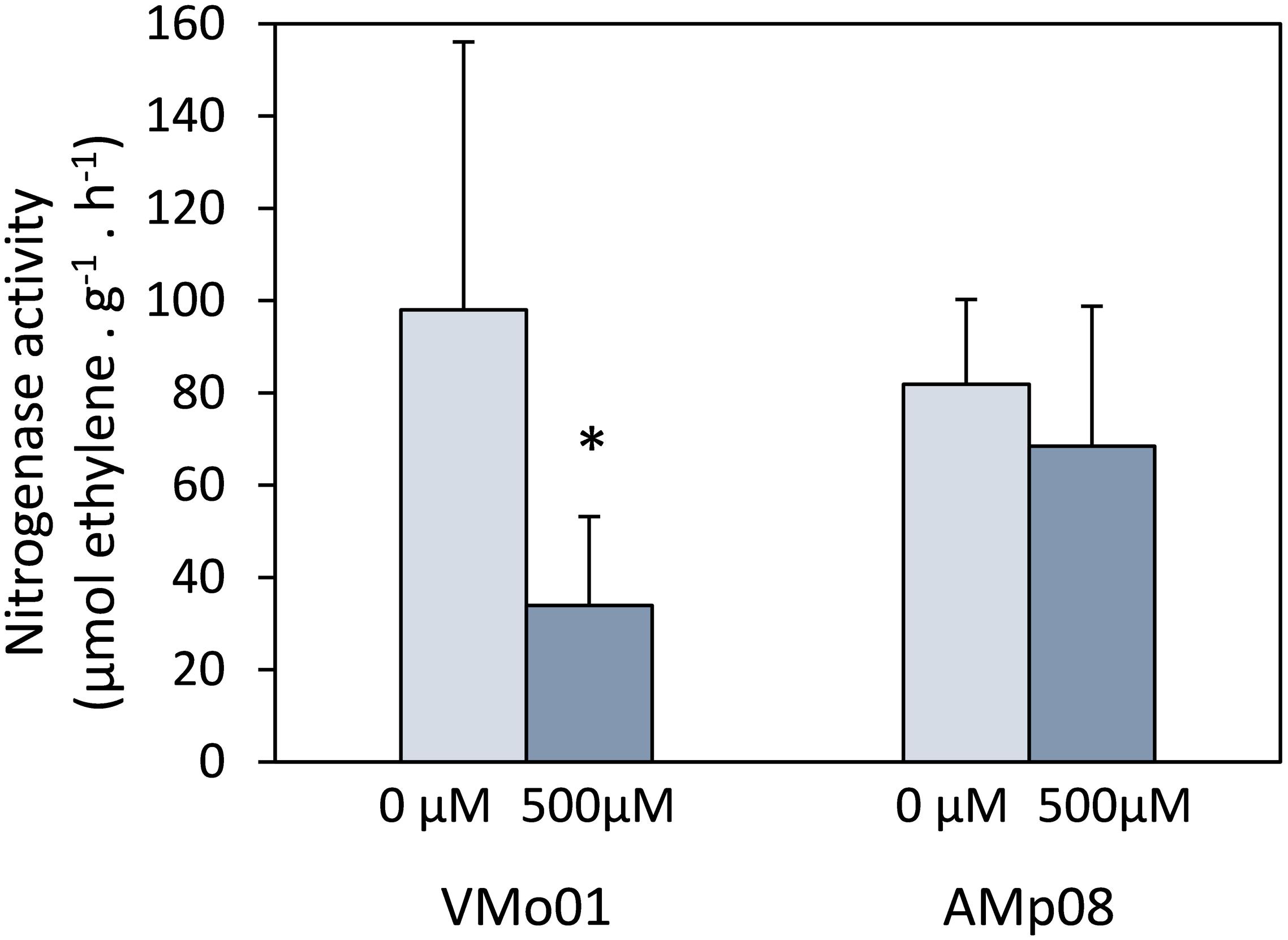
Figure 3. Nitrogenase activity per gram of nodule in control and Hg-treated M. truncatula nodules inoculated with E. medicae strains VMo01 (sensitive) and AMp08 (tolerant). Five week-old plants were watered with nutrient solution containing 0 or 500 μM HgCl2 and harvested after 24 h. Three independent biological experiments were performed. In each experiment, 7–10 plants per treatment were analyzed. Mean values ± SD are represented. An asterisk indicates significant differences (p < 0.05) between control and Hg-treated nodules.
To examine the prevalence and conservation of the merA genes among sequenced rhizobia, we performed a phylogenetic analysis. Widespread conservation of merA genes across rhizobia species was observed, as it was present in all species examined, including species from the genera Agrobacterium, Bradyrhizobium, Mesorhizobium, Rhizobium, and Ensifer (Figure 4). Two MerA homologs were identified in some species of the genera Ensifer and Mesorhizobium that were located in different clades. With a few exceptions, there was good correspondence between the MerA phylogeny and rhizobial systematics, where species of the same genus clustered together.
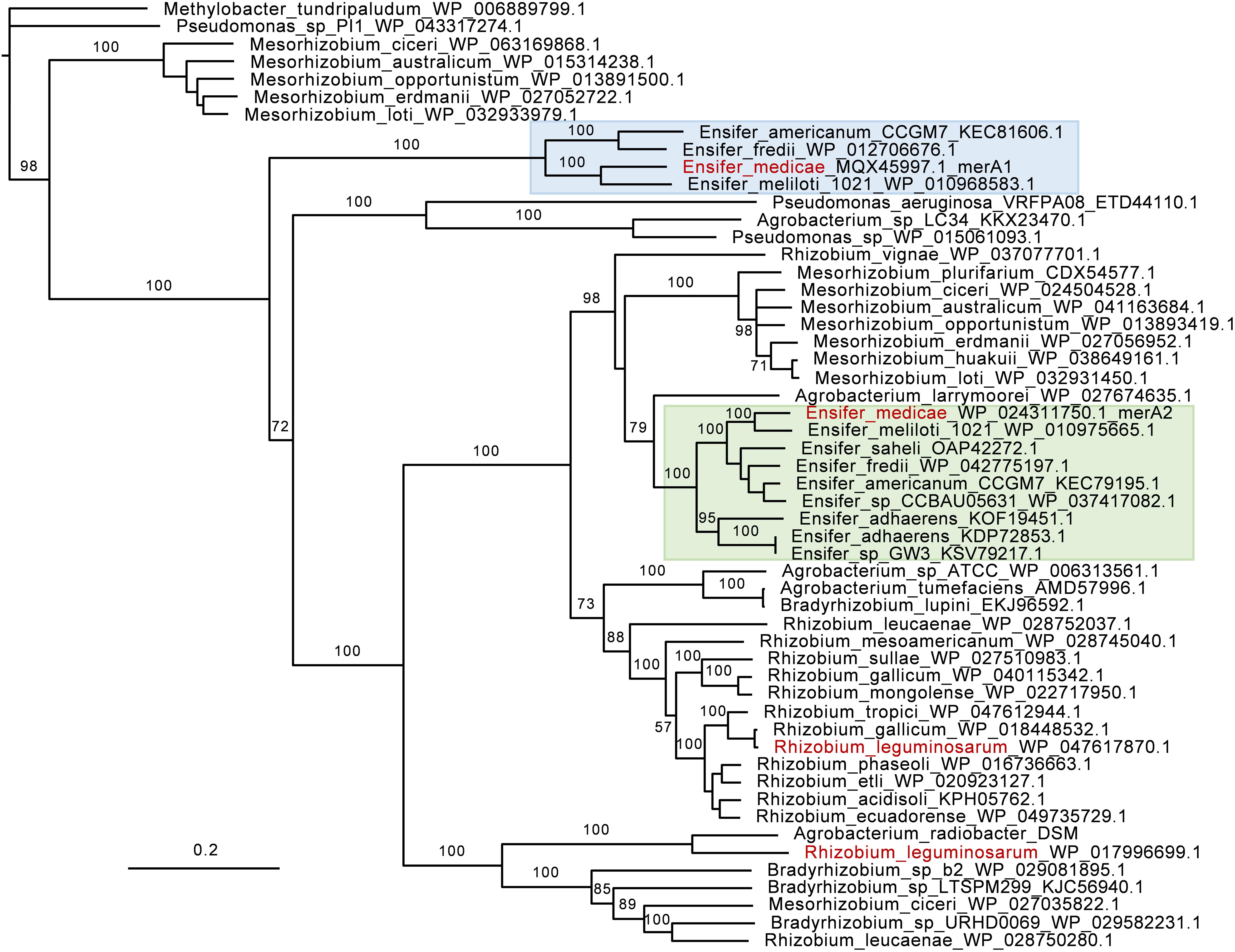
Figure 4. Phylogeny of mercuric reductase (MerA) in rhizobia. GenBank accession numbers are indicated. The phylogeny is based on amino acid sequences. The tree was rooted using two non-rhizobia MerA from Methlybacter tundripaludum and Pseudomonas sp. as outgroup sequences. The Ensifer sp. clades for MerA1 and MerA2 homologes are highlighted in blue and green, respectively. Ensifer medicae and Rhizobium leguminosarum are shown in red text.
Discussion
In this study, we analyzed the tolerance to Hg of several rhizobial strains collected from Hg-contaminated soils, and evaluated the role of mercuric reductase activity in the mercury tolerance of free-living bacteria and in the maintenance of nitrogen fixation in legume nodules subjected to mercury stress. The MIC values of all the strains used in this study were carefully re-evaluated, as in some preliminary mercuric reductase assays, some strains, which were initially considered as non-tolerant, displayed substantial mercuric reductase activity. The MIC values determined here for most E. medicae strains were similar to, although slightly higher than those reported in a previous study (Nonnoi et al., 2012), with a few of them that were considerably higher than those described earlier by Nonnoi et al. (2012). These differences could be attributed to modifications that might have occurred during the sub-culturing processes, in which inactive merA genes in the freshly collected strains might have been activated. The MIC values for R. leguminosarum were nearly identical to those reported previously (Nonnoi et al., 2012). The MIC values that we obtained for the B. canariense strains were consistently higher than those published earlier (Ruiz-Díez et al., 2012, Ruiz-Díez et al., 2013). The previously published MIC values for the B. canariense strains were determined in liquid medium, while in the present work, solid medium was used. The observed differences could be related to the fact that bacteria have the ability to form biofilms when they grow attached to a surface. Such biofilms are formed mainly by exopolysaccharides (EPS). The EPS matrix works as a diffusion barrier that slows down penetration of compounds such as toxic agents, antibiotics, and metals. Thus, the EPS matrix on the agar plates could be the cause for the observed higher tolerance to mercury. On the contrary, when bacteria are grown in suspension in liquid medium, they are dispersed and are more sensitive to toxic substances (Mah and O’Toole, 2001; Teitzel and Parsek, 2003). The presence of sensitive and tolerant strains in contaminated soils is common and might be due to several factors, such as the presence of micro-niches in the soil (Nonnoi et al., 2012).
A comparative promoter analysis could help determine a mechanism to explain the differences in mercuric reductase activity between strains, but this will require new genome assemblies and genome wide expression of sensitive and tolerant strains, data that are not available at the moment. Additionally, cis-regulatory differences could be also likely to explain differences between tolerant and sensitive strains; this would require experimental evidence rather than simple sequence comparisons. We are actually planning to test these hypotheses. Nevertheless, we feel that we have made a convincing case showing that the more tolerant E. medicae strains had significantly higher expression than non-tolerant strains, which is what we set out to do.
To our knowledge, there are no reports on the mechanisms that might account for mercury tolerance in rhizobia. Regarding the mer operon, variations in structure and organization have been found in different bacteria (Boyd and Barkay, 2012; Naguib et al., 2018). No generic mer operons have been identified up to now in the sequenced rhizobia genomes found in databases. Only merA and merR homologs have been identified in some of these genomes. The presence of merA, in the absence of any transporter (merT) and/or regulatory genes (merR), does not necessary imply effective Hg2+ reduction and mercury tolerance, as it has been observed in some bacteria lacking mer transporter genes (Osborn et al., 1997).
The mercuric reductase assays showed that the highly tolerant E. medicae strains presented the highest activities. We found a strong correlation between MIC values and mercuric reductase activity for this species. Others authors have also observed mercury tolerance involving mercury reductase activity in other types of bacteria lacking mer specific transporter genes, and it was postulated that protein transporters not specific to the mer system could be involved in mercury transport (Wang et al., 2009). In contrast, no correlation between MIC values and mercuric reductase activity was found for the B. canariense strains. This lack of correlation suggests that B. canariense might have other mercury tolerance mechanisms, such as the exopolysaccharide production mentioned above. In fact, the colonies formed by B. canariense strains appeared clearly more viscous or gelatinous than those formed by E. medicae or R. leguminosarum. This Hg-binding EPS mechanism most likely presents some intra-species variation, as the composition of the EPS matrix might vary, resulting in different MIC values among B. canariense strains. Due to the limited number of tolerant strains, the data for R. leguminosarum appear insufficient to determine whether there was a correlation between tolerance and mercuric reductase activity. In fact, it cannot be ruled out that different additional mechanisms might contribute to Hg tolerance in any of the three species.
When bacteria were grown in the presence of a sub-lethal Hg concentration, an increase in mercuric reductase activity could be observed for some strains. Most bacterial mercury detoxification systems are related to an induction phenomenon (Wang et al., 2009), and it is known that the expression of the merA gene, which encodes the MerA enzyme, can be induced by the presence of mercury (Freedman et al., 2012). Generic mer operons might carry regulatory genes. MerR is a transcriptional repressor which attaches to the promotor-operator region of the operon and acts as repressor or activator of the operon in the absence and presence of Hg2+, respectively (Barkay et al., 2003; Boyd and Barkay, 2012). We did not identify merR homologs in E. medicae or R. leguminosarum sequenced strains. Nonetheless, our results show a correspondence between mercuric reductase activity and merA expression, as both increased when bacteria were grown in the presence of a low Hg concentration, and merA expression in tolerant strains was higher than in sensitive strains. We analyzed merA expression in two E. medicae strains and two R leguminosarum strains with different Hg tolerance, and the results were similar for both species, suggesting the existence of a Hg-dependent regulation of merA expression and MerA activity. However, we cannot exclude that other strains might display different behavior.
It is known that when the differentiation from bacteria to bacteroids happens, there are physiological and genetic changes (Ampe et al., 2003). Some genes are induced while others are repressed or fully inactivated in the bacteroid. Here we showed that merA genes were expressed inside M. truncatula nitrogen-fixing nodules and the MerA was active. The expression patterns differed from those observed in free-living bacteria. Besides the clear differences between both experiments, the changes in genetic regulation that occur in the differentiation process could also explain the differences in merA gene transcription between the free-living E. medicae bacteria and bacteroids.
The expression of merA1 and merA 2 in the different zones of the nodule was analyzed using the data of Roux et al. (2014) for expression of Ensifer meliloti genes in sections of M. truncatula nodules. merA1 presented very low expression in the uninfected meristem. Expression in the FII zone, where the number of bacteria should be low, was high and similar to that of the infected zone FIII. This could indicate that merA1 expression is lower in bacteroids than in undifferenciated bacteria, or could be due to some contamination of FII sections with infected cells. merA2 displayed low expression in uninfected tissues (FI and FIId), and high expression in the fixation zone FIII.
Tolerant rhizobia strains constitute a promising bioremediation tool as they can survive in the presence of high metal concentrations (Hao et al., 2014). According to our results, a tolerant strain appears to be more efficient in reducing mercury on account of a higher basal merA expression. We and other groups have previously described that metal-tolerant rhizobia help maintain nitrogen fixation under metal stress conditions (Shvaleva et al., 2010; Rangel et al., 2017). Quiñones et al. (2013) showed in a long-term experiment that Lupinus albus plants grown in the presence of Hg and inoculated with a Hg-tolerant strain were more tolerant than those inoculated with a sensitive strain. This was likely due to the preservation of nitrogen fixation under mercury stress, but additional factors were involved. When the lupin plants were inoculated with a Hg-sensitive strain, nodulation was severely affected by mercury and a drastic drop in nitrogenase activity was observed. However, in their experimental conditions it was difficult to determine whether mercury was affecting nitrogen fixation itself, or the decrease in activity was a consequence of poor nodulation or formation of non-effective nodules. For these reasons, we designed a short-term experiment to affect nitrogen fixation alone, to show that the tolerance of the strain was important to maintain nitrogenase activity under mercury stress conditions. The present results suggest that nitrogen fixation is differentially affected by mercury stress depending on the mercury tolerance of the inoculated rhizobia.
The Hg-tolerant E. medicae strain used in this work and its protective effect on nitrogen fixation in the presence of Hg stress suggest a potential use as a bioremediation tool in Hg-contaminated environments. This could be exploited using the bacteria alone, due to their high mercuric reductase activity, or in association with tolerant M. truncatula varieties. In fact, we have analyzed M. truncatula germplasm for Hg tolerance (García de la Torre et al., 2013) and identified some Hg-tolerant cultivars. Moreover, M. truncatula is a forage legume that produces high biomass and has a good soil coverage. As a result, we consider that the combination of tolerant E. medicae strains and tolerant M. truncatula varieties has the potential to become a powerful tool for detoxification of Hg-contaminated soils.
Data Availability Statement
The original contributions presented in the study are included in the article/Supplementary Material, further inquiries can be directed to the corresponding author.
Author Contributions
TC, MML, and JJP contributed conception and design of the study. GA and PH determined the MIC values and the mercuric reductase activity of free living bacteria. TC, BP, GA, and PH designed primers and performed the qPCR assays. VL-D carried out the nitrogenase activity assays. DG-R and HPV determined the mercuric reductase activity in nodules. PT and DB contributed to the setup of the mercuric reductase assay. TP and TC contributed to the phylogeny analyses and to the discussion section of the manuscript. GA and DG-R wrote the first draft of the manuscript. JJP supervised and contributed to all the experiments in the manuscript. All authors contributed to manuscript revision, read, and approved the submitted version.
Funding
This work was supported by a grant from Agencia Estatal de Investigación (AEI) (AGL2017-88381-R to JJP and MML). DG-R was supported by a JAE Intro fellowship from Consejo Superior de Investigaciones Científicas (CSIC) (JAEINT19_EX_0082). We acknowledge support of the publication fee (25%) by the CSIC Open Access Publication Support Initiative through its Unit of Information Resources for Research (URICI).
Conflict of Interest
The authors declare that the research was conducted in the absence of any commercial or financial relationships that could be construed as a potential conflict of interest.
Acknowledgments
We thank Dr. Mercedes Fernández-Pascual for kindly providing the B. canariense strains.
Supplementary Material
The Supplementary Material for this article can be found online at: https://www.frontiersin.org/articles/10.3389/fpls.2020.560768/full#supplementary-material
References
Ampe, F., Kiss, E., Sabourdy, F., and Batut, J. (2003). Transcriptome analysis of Sinorhizobium meliloti during symbiosis. Genome Biol. 4:R15. doi: 10.1186/gb-2003-4-2-r15
Azevedo, R., and Rodriguez, E. (2012). Phytotoxicity of mercury in plants: a review. J. Bot. 2012:848614. doi: 10.1155/2012/848614
Barkay, T., Miller, S. M., and Summers, A. O. (2003). Bacterial mercury resistance from atoms to ecosystems. FEMS Microbiol. Rev. 27, 355–384. doi: 10.1016/S0168-6445(03)00046-9
Bellabarba, A., Fagorzi, C., diCenzo, G. C., Pini, F., Viti, C., and Checcucci, A. (2019). Deciphering the symbiotic plant microbiome: translating the most recent discoveries on rhizobia for the improvement of agricultural practices in metal-contaminated and high saline lands. Agronomy 9:529. doi: 10.3390/agronomy9090529
Blazka, M. E., and Shaikh, Z. A. (1992). Cadmium and mercury accumulation in rat hepatocytes: interactions with other metal ions. Toxicol. Appl. Pharmacol. 113, 118–125. doi: 10.1016/0041-008X(92)90015-K
Boyd, E. S., and Barkay, T. (2012). The mercury resistance operon: from an origin in a geothermal environment to an efficient detoxification machine. Front. Microbiol. 3:349. doi: 10.3389/fmicb.2012.00349
Bridges, C. C., and Zalups, R. K. (2005). Molecular and ionic mimicry and the transport of toxic metals. Toxicol. Appl. Pharmacol. 204, 274–308. doi: 10.1016/j.taap.2004.09.007
Dary, M., Chamber-Pérez, M. A., Palomares, A. J., and Pajuelo, E. (2010). “In situ” phytostabilisation of heavy metal polluted soils using Lupinus luteus inoculated with metal resistant plant-growth promoting rhizobacteria. J. Hazard. Mater. 177, 323–330. doi: 10.1016/j.jhazmat.2009.12.035
Dash, H. R., and Das, S. (2012). Bioremediation of mercury and the importance of bacterial mer genes. Int. Biodeterior. Biodegradation 75, 207–213. doi: 10.1016/j.ibiod.2012.07.023
David, I. G., Matache, M. L., Tudorache, A., Chisamera, G., Rozylowicz, L., and Radu, G. L. (2012). Food chain biomagnification of heavy metals in samples from the lower prut floodplain natural park. Environ. Eng. Manag. J. 11, 69–73. doi: 10.30638/eemj.2012.010
Fagorzi, C., Checcucci, A., diCenzo, G. C., Debiec-Andrzejewska, K., Dziewit, L., Pini, F., et al. (2018). Harnessing rhizobia to improve heavy-metal phytoremedation by legumes. Genes 9:542. doi: 10.3390/genes9110542
Freedman, Z., Zhu, C., and Barkay, T. (2012). Mercury resistance and mercuric reductase activities and expression among chemotrophic thermophilic Aquificae. Appl. Environ. Microbiol. 78, 6568–6575. doi: 10.1128/AEM.01060-12
García de la Torre, V. S., Coba de la Peña, T., Lucas, M. M., and Pueyo, J. J. (2013). Rapid screening of Medicago truncatula germplasm for mercury tolerance at the seedling stage. Environ. Exp. Bot. 91, 90–96. doi: 10.1016/j.envexpbot.2013.03.004
Hao, X., Taghavi, S., Xie, P., Orbach, M. J., Alwathnani, H. A., Rensing, C., et al. (2014). Phytoremediation of heavy and transition metals aided by legume-rhizobia symbiosis. Int. J. Phytoremediation 16, 179–202. doi: 10.1080/15226514.2013.773273
Hoagland, D. R., and Arnon, D. I. (1938). The Water Culture Method for Growing Plants Without Soil. Berkeley: University of California.
Kearse, M., Moir, R., Wilson, A., Stones-Havas, S., Cheung, M., Sturrock, S., et al. (2012). Geneious basic: an integrated and extendable desktop software platform for the organization and analysis of sequence data. Bioinformatics 28, 1647–1649. doi: 10.1093/bioinformatics/bts199
Lavoie, R. A., Jardine, T. D., Chumchal, M. M., Kidd, K. A., and Campbell, L. M. (2013). Biomagnification of mercury in aquatic food webs: a worldwide meta-analysis. Environ. Sci. Technol. 47, 13385–13394. doi: 10.1021/es403103t
Lominchar, M. A., Sierra, M. J., and Millán, R. (2015). Accumulation of mercury in Typha domingensis under field conditions. Chemosphere 119, 994–999. doi: 10.1016/j.chemosphere.2014.08.085
Mah, T. F., and O’Toole, G. A. (2001). Mechanisms of biofilm resistance to antimicrobial agents. Trends Microbiol. 9, 34–39. doi: 10.1016/S0966-842X(00)01913-2
Malayeri, B. E., Chehregani, A., Yousefi, N., and Lorestani, B. (2008). Identification of the hyper accumulator plants in copper and iron mine in Iran. Pakistan J. Biol. Sci. 11, 490–492. doi: 10.3923/pjbs.2008.490.492
Martínez-Coronado, A., Oyarzun, R., Esbrí, J. M., Llanos, W., and Higueras, P. (2011). Sampling high to extremely high Hg concentrations at the Cerco de Almadenejos, Almadén mining district (Spain): the old metallurgical precinct (1794 to 1861 AD) and surrounding areas. J. Geochem. Explor. 109, 70–77. doi: 10.1016/j.gexplo.2010.04.007
Marx, S. K., Rashid, S., and Stromsoe, N. (2016). Global-scale patterns in anthropogenic Pb contamination reconstructed from natural archives. Environ. Pollut. 213, 283–298. doi: 10.1016/j.envpol.2016.02.006
Mathema, V. B., Thakuri, B. C., and Sillanpää, M. (2011). Bacterial mer operon-mediated detoxification of mercurial compounds: a short review. Arch. Microbiol. 193, 837–844. doi: 10.1007/s00203-011-0751-4
Mudipalli, A. (2008). Metals (Micro nutrients or toxicants) & global health. Indian J. Med. Res. 128, 331–334.
Naguib, M. M., El-Gendy, A. O., and Khairalla, A. S. (2018). Microbial diversity of mer operon genes and their potential roles in mercury bioremediation and resistance. Open Biotechnol. J. 12, 56–77. doi: 10.2174/1874070701812010056
Nakamura, K., and Nakahara, H. (1988). Simplified X-ray film method for detection of bacterial volatilization of mercury chloride by Escherichia coli. Appl. Environ. Microbiol. 54, 2871–2873. doi: 10.1128/AEM.54.11.2871-2873.1988
Nies, D. H. (2003). Efflux-mediated heavy metal resistance in prokaryotes. FEMS Microbiol. Rev. 27, 313–339. doi: 10.1016/S0168-6445(03)00048-2
Nieto, J. J., Fernández-Castillo, R., Márquez, M. C., Ventosa, A., Quesada, E., and Ruiz-Berraquero, F. (1989). Survey of metal tolerance in moderately halophilic eubacteria. Appl. Environ. Microbiol. 55, 2385–2390. doi: 10.1128/AEM.55.9.2385-2390.1989
Nonnoi, F., Chinnaswamy, A., García de la Torre, V. S., Coba de la Peña, T., Lucas, M. M., and Pueyo, J. J. (2012). Metal tolerance of rhizobial strains isolated from nodules of herbaceous legumes (Medicago spp. and Trifolium spp.) growing in mercury-contaminated soils. Appl. Soil Ecol. 61, 49–59. doi: 10.1016/j.apsoil.2012.06.004
Osborn, A. M., Bruce, K. D., Strike, P., and Ritchie, D. A. (1997). Distribution, diversity and evolution of the bacterial mercury resistance (mer) operon. FEMS Microbiol. Rev. 19, 239–262. doi: 10.1111/j.1574-6976.1997.tb00300.x
Paris, J. R., King, R. A., and Stevens, J. R. (2015). Human mining activity across the ages determines the genetic structure of modern brown trout (Salmo trutta L.) populations. Evol. Appl. 8, 573–585. doi: 10.1111/eva.12266
Patra, M., and Sharma, A. (2000). Mercury toxicity in plants. Bot. Rev. 366, 379–422. doi: 10.1007/BF02868923
Pfaffl, M. W. (2001). A new mathematical model for relative quantification in real-time RT-PCR. Nucleic Acids Res. 29, 2002–2007. doi: 10.1093/nar/29.9.e45
Quiñones, M. A., Ruiz-Díez, B., Fajardo, S., López-Berdonces, M. A., Higueras, P. L., and Fernández-Pascual, M. (2013). Lupinus albus plants acquire mercury tolerance when inoculated with an Hg-resistant Bradyrhizobium strain. Plant Physiol. Biochem. 73, 168–175. doi: 10.1016/j.plaphy.2013.09.015
Rangel, W. M., Thijs, S., Janssen, J., Oliveira Longatti, S. M., Bonaldi, D. S., Ribeiro, P. R. A., et al. (2017). Native rhizobia from Zn mining soil promote the growth of Leucaena leucocephala on contaminated soil. Int. J. Phytoremediation 19, 142–156. doi: 10.1080/15226514.2016.1207600
Robinson, J. B., and Tuovinen, O. H. (1984). Mechanisms of microbial resistance and detoxification of mercury and organomercury compounds: physiological, biochemical, and genetic analyses. Microbiol. Rev. 48, 95–124. doi: 10.1128/mmbr.48.2.95-124.1984
Roux, B., Rodde, N., Jardinaud, M. F., Timmers, T., Sauviac, L., Cottret, L., et al. (2014). An integrated analysis of plant and bacterial gene expression in symbiotic root nodules using laser-capture microdissection coupled to RNA sequencing. Plant J. 77, 817–837. doi: 10.1111/tpj.12442
Ruiz-Díez, B., Fajardo, S., Quiñones, M. A., López, M. A., Higueras, P., and Fernández-Pascual, M. (2013). “Selección de cepas de rhizobia tolerantes a mercurio por su capacidad de bioacumulación y su efectividad en leguminosas arbustivas,” in Microorganisms for Future Agriculture (Abstracts of the II Iberoamerican Conference on Beneficial Plant-Microorganism-Environment Interactions), ed. M. Megía, (Seville: Publicaciones de la Universidad de Sevilla), 454–455.
Ruiz-Díez, B., Quiñones, M. A., Fajardo, S., López, M. A., Higueras, P., and Fernández-Pascual, M. (2012). Mercury-resistant rhizobial bacteria isolated from nodules of leguminous plants growing in high Hg-contaminated soils. Appl. Microbiol. Biotechnol. 96, 543–554. doi: 10.1007/s00253-011-3832-z
Saitou, N., and Nei, M. (1987). The neighbor-joining method: a new method for reconstructing phylogenetic trees. Mol. Biol. Evol. 4, 406–425. doi: 10.1093/oxfordjournals.molbev.a040454
Shvaleva, A., Coba de la Peña, T., Rincón, A., Morcillo, C. N., García de la Torre, V., Lucas, M. M., et al. (2010). Flavodoxin overexpression reduces cadmium-induced damage in alfalfa root nodules. Plant Soil 326, 109–121. doi: 10.1007/s11104-009-9985-1
Tchounwou, P. B., Yedjou, C. G., Patlolla, A. K., and Sutton, D. J. (2012). Heavy metals toxicity and the environment. EXS 101, 133–164. doi: 10.1007/978-3-7643-8340-4_6
Teitzel, G. M., and Parsek, M. R. (2003). Heavy metal resistance of biofilm and planktonic Pseudomonas aeruginosa. Appl. Environ. Microbiol. 69, 2313–2320. doi: 10.1128/AEM.69.4.2313-2320.2003
Udvardi, M., and Poole, P. S. (2013). Transport and metabolism in legume-rhizobia symbioses. Annu. Rev. Plant Biol. 64, 781–805. doi: 10.1146/annurev-arplant-050312-120235
Vincent, J. M. (1970). A Manual for the Practical Study of Root-nodule Bacteria. Oxford: Blackwell Scientific, doi: 10.1002/jobm.19720120524
Wang, Y., Freedman, Z., Lu-Irving, P., Kaletsky, R., and Barkay, T. (2009). An initial characterization of the mercury resistance (mer) system of the thermophilic bacterium Thermus thermophilus HB27. FEMS Microbiol. Ecol. 67, 118–129. doi: 10.1111/j.1574-6941.2008.00603.x
Keywords: rhizobia, mercury, mercuric reductase, merA, nitrogen fixation, nodule, Ensifer medicae, Medicago truncatula
Citation: Arregui G, Hipólito P, Pallol B, Lara-Dampier V, García-Rodríguez D, Varela HP, Tavakoli Zaniani P, Balomenos D, Paape T, Coba de la Peña T, Lucas MM and Pueyo JJ (2021) Mercury-Tolerant Ensifer medicae Strains Display High Mercuric Reductase Activity and a Protective Effect on Nitrogen Fixation in Medicago truncatula Nodules Under Mercury Stress. Front. Plant Sci. 11:560768. doi: 10.3389/fpls.2020.560768
Received: 10 May 2020; Accepted: 22 December 2020;
Published: 14 January 2021.
Edited by:
Brigitte Mauch-Mani, Université de Neuchâtel, SwitzerlandReviewed by:
Clarisse Brígido, University of Evora, PortugalLee-Feng Chien, National Chung Hsing University, Taiwan
Copyright © 2021 Arregui, Hipólito, Pallol, Lara-Dampier, García-Rodríguez, Varela, Tavakoli Zaniani, Balomenos, Paape, Coba de la Peña, Lucas and Pueyo. This is an open-access article distributed under the terms of the Creative Commons Attribution License (CC BY). The use, distribution or reproduction in other forums is permitted, provided the original author(s) and the copyright owner(s) are credited and that the original publication in this journal is cited, in accordance with accepted academic practice. No use, distribution or reproduction is permitted which does not comply with these terms.
*Correspondence: José J. Pueyo, amoucHVleW9AY3NpYy5lcw==