- 1Genetics and Genomics of Plants, CeBiTec and Faculty of Biology, Bielefeld University, Bielefeld, Germany
- 2Algae Biotechnology and Bioenergy, CeBiTec and Faculty of Biology, Bielefeld University, Bielefeld, Germany
Flavonol synthase (FLS) is a key enzyme for the formation of flavonols, which are a subclass of the flavonoids. FLS catalyzes the conversion of dihydroflavonols to flavonols. The enzyme belongs to the 2-oxoglutarate-dependent dioxygenases (2-ODD) superfamily. We characterized the FLS gene family of Brassica napus that covers 13 genes, based on the genome sequence of the B. napus cultivar Express 617. The goal was to unravel which BnaFLS genes are relevant for seed flavonol accumulation in the amphidiploid species B. napus. Two BnaFLS1 homeologs were identified and shown to encode bifunctional enzymes. Both exhibit FLS activity as well as flavanone 3-hydroxylase (F3H) activity, which was demonstrated in vivo and in planta. BnaFLS1-1 and -2 are capable of converting flavanones into dihydroflavonols and further into flavonols. Analysis of spatio-temporal transcription patterns revealed similar expression profiles of BnaFLS1 genes. Both are mainly expressed in reproductive organs and co-expressed with the genes encoding early steps of flavonoid biosynthesis. Our results provide novel insights into flavonol biosynthesis in B. napus and contribute information for breeding targets with the aim to modify the flavonol content in rapeseed.
Introduction
Rapeseed (Brassica napus L.) is the second most important oil crop worldwide (Nesi et al., 2008; OECD-FAO and Connell, 2015). The high oil (∼50%) and protein (∼25%) content of B. napus seed is the result of decades of extensive breeding aiming to improve its nutritional quality and agronomical yield (Nesi et al., 2008). Still, the presence of anti-nutritional components, like phenolic compounds or glucosinolates, render rapeseed protein essentially unusable for human consumption (Wang et al., 2018; Hald et al., 2019). While glucosinolate break-down products cause metabolic disturbances, phenolics can impair digestibility and cause a strong bitter off-taste (Nesi et al., 2008; Wanasundara et al., 2016; Hald et al., 2019). The glucosinolates amount in seeds have been greatly reduced through breeding of double zero lines with improved nutraceutical properties (Nesi et al., 2008). However, breeding of low phenolic lines with optimal compositions for the use of rapeseed protein as edible vegetable product is difficult. The reason is the great diversity of phenolic compounds and their involvement in many processes which impact plant fitness (Auger et al., 2010; Wang et al., 2018). Phenolics can be beneficial for human health due to their antioxidant activity, thereby facilitating the prevention of cardiovascular diseases and cancer (Wang et al., 2018). On the other hand, phenolics can (i) impair digestibility, (ii) cause undesired dark color, and (iii) cause bitter off-taste derived from kaempferol-derivatives (Auger et al., 2010; Hald et al., 2019). Therefore, breeding of low or high phenolic cultivars depends on their economic use, e.g., use as seed oil/animal feed or edible vegetable (Wang et al., 2018).
Flavonoids are a major group of phenolics and belong to a diverse class of plant specialized metabolites comprising over 9,000 different substances (Williams and Grayer, 2004; Grotewold, 2006). They are derived from flavonoid biosynthesis (Figure 1), which branch of from the phenylalanine-based general phenylpropanoid pathway (Hahlbrock and Scheel, 1989). Flavonoids are classified in different subgroups, namely chalcones, flavones, flavandiols, anthocyanins, proanthocyanidins (PAs), aurones, and flavonols (Winkel-Shirley, 2001). Flavonols define the largest subgroup of flavonoids, mainly due to a plethora of glycosylation patterns (Zhang et al., 2013). They are classified in e.g., kaempferols and quercetins depending on the hydroxylation pattern of the B ring (Winkel-Shirley, 2001). Flavonols are colorless for the human eye but absorb in the ultraviolet (UV) range. After light treatment, they accumulate in their glycosylated form in the vacuole of epidermal and mesophyll cells or on occasion in epicuticular waxes (Weisshaar and Jenkins, 1998; Winkel-Shirley, 2001; Agati et al., 2009). Their biosynthesis is largely influenced by environmental cues such as temperature and UV light (Winkel-Shirley, 2002; Olsen et al., 2009). Flavonols have several physiological functions in plants including antimicrobial properties, UV protection, modulation of auxin transport, male fertility, and flower pigmentation together with anthocyanins (Harborne and Williams, 2000; Peer and Murphy, 2007).
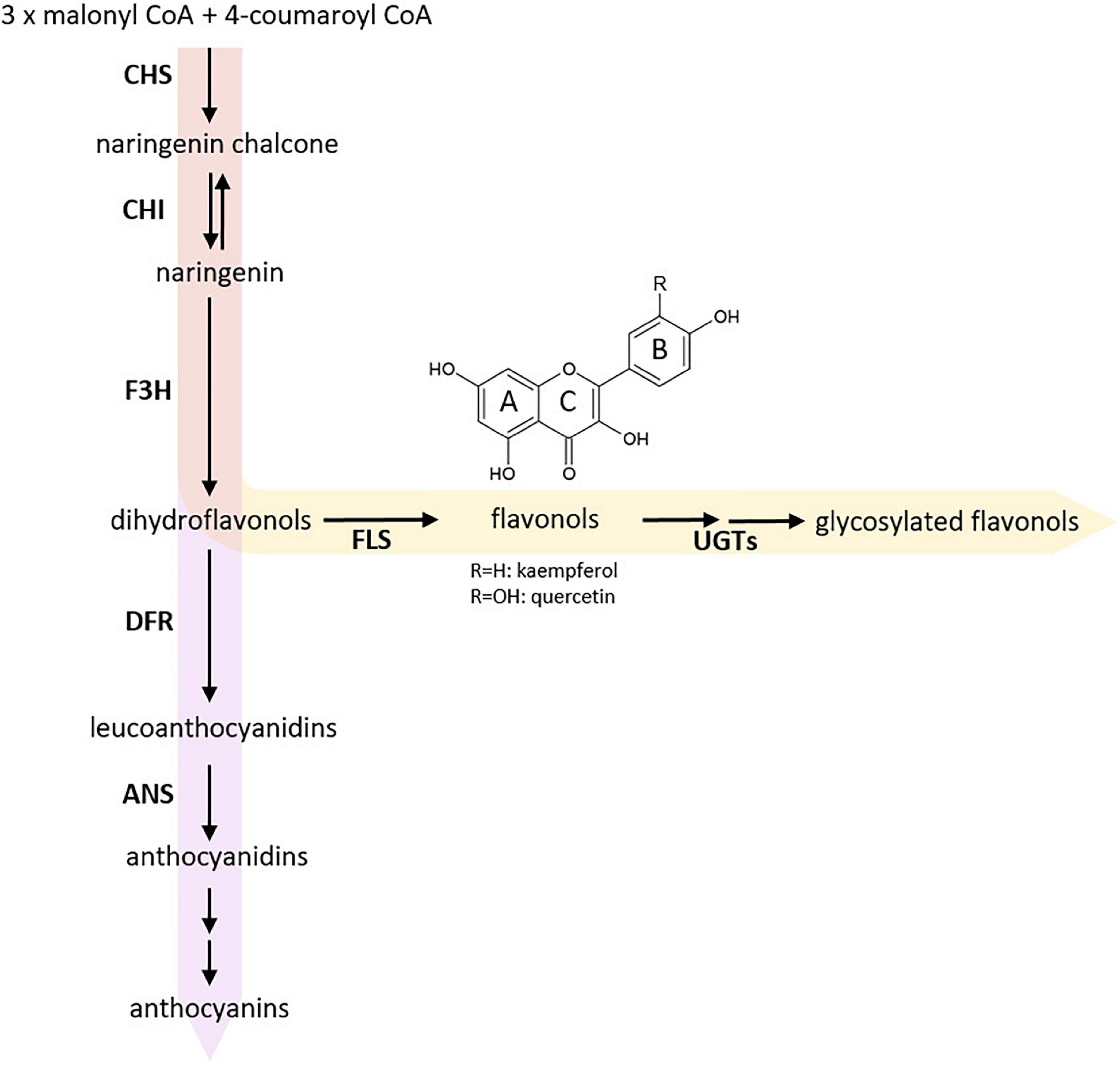
Figure 1. Simplified scheme of flavonoid biosynthesis. The flavonol biosynthesis pathway (highlighted via an orange arrow) is part of the flavonoid biosynthesis, which also includes the anthocyanin pathway (highlighted via a violet arrow) (modified after Winkel-Shirley, 2001). The metabolic flux into the flavonol biosynthesis is influenced by dihydroflavonol 4-reductase (DFR) as it competes with FLS for substrates. Enzyme names are abbreviated as follows: chalcone synthase (CHS), chalcone isomerase (CHI), flavanone 3-hydroxylase (F3H), flavonol synthase (FLS), UDP-glycosyltransferases (UGTs), anthocyanidin synthase (ANS).
The central enzyme of flavonol biosynthesis is flavonol synthase (FLS). FLS converts a dihydroflavonol into the corresponding flavonol by introducing a double bond between C-2 and C-3 of the C-ring (Figure 1; Forkmann et al., 1986; Holton et al., 1993). FLS activity was first identified in irradiated parsley cells (Britsch et al., 1981). Several studies identified more than one FLS gene in the genome of a given species, including Zea mays (Falcone Ferreyra et al., 2012), Musa acuminata (Busche et al., 2021), Vitis vinifera (Downey et al., 2003; Fujita et al., 2006), Fressica hybrida (Shan et al., 2020), and Arabidopsis thaliana (Pelletier et al., 1997; Owens et al., 2008). In A. thaliana, which is evolutionary closely related to B. napus, most genes of the central enzymes of the flavonoid biosynthesis are encoded by single-copy genes. However, FLS marks an exception as there are six genes annotated in the A. thaliana genome sequence (Pelletier et al., 1997; Owens et al., 2008). Only FLS1 encodes a functional FLS, thus being the major contributor to flavonol production in A. thaliana (Wisman et al., 1998). It has been postulated that the AthFLS gene family derived from recent gene duplication events and is currently undergoing a pseudogenization process to eliminate “unnecessary” gene copies (Preuss et al., 2009; Stracke et al., 2009). The Brassicaceae-lineage specific whole genome triplication followed by diploidization after divergence from the common ancestor of A. thaliana and B. napus (Wang et al., 2011; Chalhoub et al., 2014) suggests that the amphidiploid B. napus harbors an even larger FLS family, which formally may cover up to 36 members. So far, six FLS genes have been identified for the A-subgenome donor B. rapa (Guo et al., 2014), while the C-subgenome donor B. oleracea has not yet been studied in detail. Up to now, the exact size of the B. napus FLS gene family remains unknown. Previous studies on the flavonol biosynthesis in B. napus were mainly focused on metabolites (Auger et al., 2010) or covered transcriptomic and phylogenetic analysis of genes preceding the FLS reaction in the flavonol pathway (Qu et al., 2016).
Some FLSs have been characterized as bifunctional enzymes, exhibiting FLS and F3H activity (Figure 1), e.g., in A. thaliana (Prescott et al., 2002; Owens et al., 2008), Oriza sativa (Park et al., 2019), Citrus unshiu (Lukacin et al., 2003), and Ginkgo biloba (Xu et al., 2012). FLS has been classified as a 2-oxoglutarate-dependent dioxygenase (2-ODD), similar to flavanone 3-hydroxylase (F3H) and anthocyanidin synthase (ANS). The three enzymes display partial amino acid (aa) sequence similarity and overlapping functions (Prescott and John, 1996; Cheng et al., 2014). The non-heme cytosolic 2-ODD enzymes require 2-oxoglutarate as co-substrate, while ferrous iron acts as co-factor (Cheng et al., 2014). FLS and ANS are relatively closely related with 50-60% aa sequence similarity, while F3H share less than 35% similarity with FLS and ANS (Lukacin et al., 2003; Cheng et al., 2014). ANS, an enzyme catalyzing a late step in the flavonoid biosynthesis pathway (Figure 1), can have both FLS and F3H activity (Welford et al., 2001; Cheng et al., 2014). Therefore, ANS contributes to flavonol production, although (at least in A. thaliana) to a much lesser extent than FLS (Preuss et al., 2009). In addition, 2-ODDs display species-specific substrate specificities and affinities (Preuss et al., 2009; Park et al., 2017; Jiang et al., 2020).
The transcriptional regulation of flavonol biosynthesis is mainly achieved by the combinatorial action(s) of MYB11, MYB12, and MYB111, which belong to subgroup 7 (SG7) of the R2R3-MYB transcription factor family (Mehrtens et al., 2005; Stracke et al., 2007). However, the myb11/myb12/myb111 triple mutant of A. thaliana retains its pollen flavonol composition (Stracke et al., 2010). This led to the discovery of MYB99, MYB21, and MYB24, which together control flavonol biosynthesis in anthers and pollen (Battat et al., 2019; Shan et al., 2020). MYB21, MYB24, and the SG7 MYBs function as independent transcriptional activators (Mehrtens et al., 2005; Stracke et al., 2007; Shan et al., 2020). The SG7 MYBs can activate all genes belonging to flavonol biosynthesis including CHS, CHI, F3H, and FLS (Mehrtens et al., 2005; Stracke et al., 2007). Recently, direct activation of AthFLS1 by AthMYB21 and AthMYB24 was shown in A. thaliana (Shan et al., 2020).
In this study, we characterize 13 members of the BnaFLS gene family, which is one of the largest FLS enzyme families analyzed to date. We separated the BnaFLS genes from F3H and ANS genes of B. napus. Only one FLS gene has been characterized so far in B. napus (Vu et al., 2015). We demonstrate that both BnaFLS1 homeologs encode bifunctional enzymes, exhibiting FLS and F3H activity, while two BnaFLS3 homeologs encode proteins with solely F3H activity. Moreover, we provide insights into the spatio-temporal transcription of BnaFLSs and present hypotheses about the mechanisms underlying FLS bifunctionality. Thus, our study provides novel insights into the flavonol biosynthesis of B. napus and supports targeted engineering of flavonol content, e.g., to enable the use of rapeseed protein in human consumption.
Materials and Methods
Plant Material
We used B. napus Express 617, a dark-seeded winter cultivar (Lee et al., 2020). B. napus was first grown in the greenhouse under long day conditions and then transferred outside for natural vernalization, followed by additional growth outside. A. thaliana Columbia 0 (Col-0, NASC ID N1092) and Nössen-0 (Nö-0, NASC ID N3081) were used as wildtype controls. The f3h mutant (tt6-2, GK-292E08, NASC ID N2105575, Col-0 background) (Appelhagen et al., 2014) and the ans/fls1 double mutant (synonym ldox/fls1-2, ldox: SALK_028793, NASC ID N2105579, Col-0 background; fls1-2: RIKEN_PST16145, Nö-0 background) (Stracke et al., 2009) were used for the generation of transgenic lines. A. thaliana plants were grown in the greenhouse under a 16-h-light/8-h-dark cycle at 22°C before transformation.
Identification of BnaFLS Candidate Genes
BnaFLS homologs were identified with KIPEs v0.255 as described previously (Pucker et al., 2020). KIPEs was run with a minimal BLAST hit similarity of 40% to reduce the number of fragmented peptides derived from possible mis-annotations. As bait, peptide sequences from the sequence collection of functional F3H, FLS, and ANS sequences described in KIPEs were used. As subject species, the peptide sequence sets of several Brassica species were used (Supplementary Table 1). The alignment was constructed with MAFFT v.7 (Katoh and Standley, 2013) and trimmed to minimal alignment column occupancy of 10%. Next, a phylogenetic tree was built with FastTree v2.1.10 (Price et al., 2009) using 10,000 rounds of bootstrapping, including the bait sequences and 2-ODD-like sequences from A. thaliana derived from Kawai et al., 2014 (Supplementary File 1). The phylogenetic tree was visualized with FigTree v1.4.31 (Supplementary Figure 1). Classification of BnaFLS candidates was generated based on the corresponding A. thaliana orthologs.
Sequence-Specific Analyses of BnaFLS Candidates and Secondary Structure Modeling
A comprehensive summary about gene-specific features of BnaFLS candidates is summarized in Supplementary Table 2. GSDS 2.0 (Hu et al., 2015) was used to generate gene structure plots. Literature knowledge was used to identify MYB-recognition elements (MRE) within 1 kbp upstream of the translational start site of BnaFLS candidates (Supplementary Figure 2). The conserved MRE consensus sequence 5′-AcCTACCa-3′, identified as a SG7 recognition motif (Hartmann et al., 2005; Stracke et al., 2007) and the sequence motifs important for the binding of AthMYB21 (MYBPZM: 5′-CCWACC-3′) and AthMYB24 (MYBCORE: 5′-CNGTTR-3′) to AthFLS1 were used for screening (Battat et al., 2019; Shan et al., 2020).
Theoretical isoelectric points, as well as molecular weight values of the BnaFLS protein sequences were calculated with ExPASY V (Gasteiger et al., 2005; Supplementary Table 3). In addition, SignalP v. 5.0 (Almagro Armenteros et al., 2019b) and TargetP v. 2.0 (Almagro Armenteros et al., 2019a) were used to infer the presence of signal peptides and N-terminal presequences of BnaFLS candidates, respectively (Supplementary Tables 4, 5). TMHMM v. 2.0 (Krogh et al., 2001) was used to predict transmembrane regions within BnaFLS sequences (Supplementary Table 2). Finally, Plant-mPLoc v. 2.0 (Chou and Shen, 2010) was used to predict the subcellular localization of BnaFLS candidates (Supplementary Table 2). Amino acid sequence identities of BnaFLSs compared to FLS homologs of A. thaliana, B. rapa, and B. oleracea were calculated based on a MAFFT alignment (Supplementary Table 6).2 Protein sequence alignments were visualized at http://espript.ibcp.fr/ESPript/ESPript/index.php v. 3.0 (Robert and Gouet, 2014) using the AthFLS1 pdb file derived from Pucker et al., 2020. Functionally relevant amino acid residues and motifs for FLS and F3H activity were highlighted.
In silico secondary structure models of relevant BnaFLS candidates were generated via I-TASSER (Roy et al., 2010) and visualized with Chimera v. 1.13.1 (Pettersen et al., 2004). The AthF3H PDB file derived from Pucker et al., 2020 was used for visualization. The generated PDB files of this work can be accessed via Supplementary File 2.
Gene Expression Analysis: Ribonucleic Acid Extraction, Library Construction, and Sequencing
Ribonucleic acid (RNA) samples were isolated from seeds and leaves using the NucleoSpin® RNA Plant kit (Macherey-Nagel, Düren, Germany) according to manufacturer’s instructions. Seed samples of the B. napus cultivar Express 617 were collected 23 and 35 days after flowering (DAF), while leave samples were collected 35 DAF. Samples were collected in triplicates. The RNA quality was validated using NanoDrop and Agilent 2100 to confirm the purity, concentration, and integrity, respectively. Based on 1 μg of total RNA, sequencing libraries were constructed following the TruSeq v2 protocol. Three seed and leaf samples per genotype were processed. Single end sequencing of 82 nt was performed on an Illumina NextSeq 500 at the Sequencing Core Facility of the Center for Biotechnology (CeBiTec) at Bielefeld University.
Gene Expression Analysis and Co-expression Analysis Using Brassica napus RNA-Seq Data
Read quality was assessed by FastQC (Andrews, 2018), revealing reads of good quality reaching a phred score of 35 or above. Next, reads were mapped to the Express 617 reference genome sequence (Lee et al., 2020) using STAR v. 2.7.1a (Dobin et al., 2013). STAR was run in basic mode allowing maximal 5% mismatches per read length and using a minimum of 90% matches per read length. These read mappings were used to manually correct the functional annotation of the BnaFLS candidates (Supplementary File 3). The corresponding corrected annotation file was used for downstream analysis.
Beside the newly generated RNA-Seq data, publicly available RNA-Seq data sets were used and retrieved from the Sequence Read Archive3 via fastq-dump v. 2.9.64 to analyze the expression of the candidate genes across various organs (Supplementary Table 7). Kallisto v. 0.44 (Bray et al., 2016) was used with default parameters to quantify transcripts abundance. The heatmap was constructed with a customized python script (see text footnote 2) using mean transcripts per millions (TPMs) per organ. Condition-independent co-expression analysis was performed to identify co-expressed genes using Spearman’s correlation coefficient (see text footnote 2) by incorporating 696 RNA-Seq data sets (Supplementary Table 8). To filter for strong co-expression the Spearman’s correlation coefficient threshold was set to 0.7 as suggested by Usadel et al. (2009).
Functional Annotation of Brassica napus Express 617 Genes
Genes were functionally annotated by transferring the A. thaliana Araport11 (Cheng et al., 2017) functional annotation to the B. napus Express 617 gene models. The annotation was used for the co-expression analysis. OrthoFinder v. 2.3.7 (Emms and Kelly, 2019) was applied using default parameters to identify orthogroups between the representative peptide sequences of Araport11 and the B. napus Express 617 peptide sequences as previously defined (Pucker et al., 2017). Remaining non-annotated genes were functionally annotated by using reciprocal best blast hits (RBHs) and best blast hits (BBHs) as described previously (Pucker et al., 2016; Supplementary Table 9).
Generation of BnaFLSs Constructs
All constructs generated in this work were produced via Gateway cloning technique according to manufacturer’s instructions and verified by DNA sequencing (Supplementary Table 10). Total RNA from leaves and seeds of Express 617 was extracted as described above (see section “Gene Expression Analysis: Ribonucleic Acid Extraction, Library Construction, and Sequencing”). Complementary DNA (cDNA) was synthesized with the ProtoScriptTM Reverse Transcriptase kit (Invitrogen, Karlsruhe, Germany) using ∼1 μg of total RNA and 1 μl of oligo (dT) and 1 μl of random-hexamer primers. cDNA fragments corresponding to the full-length ORFs of the candidate genes were then amplified via PCR with Q5® High-Fidelity Polymerase PCR kit (NEB, Frankfurt am Main, Germany) using gene-specific gateway primers (Supplementary Table 9). The sizes of the amplification products were analyzed by gel electrophoresis and visualized by ethidium bromide on a 1% agarose gel. The amplicons were purified from the PCR reagent tube via the NucleoSpin® Gel and PCR Clean-up Kit (Macherey-Nagel, Düren, Germany).
The purified cDNA fragments corresponding to the full-length ORFs of the candidate genes were then recombined into pDONRTM /Zeo (Invitrogen, Karlsruhe, Germany) using the Gateway BP Clonase II Enzyme Mix (Invitrogen, Karlsruhe, Germany) and the attB recombination sites of the respective gateway primers (Supplementary Table 10). Each entry clone was then used to transfer the CDS into the destination vector pLEELA (Jakoby et al., 2004) or pDEST17 (Invitrogen) via the Gateway LR Clonase II Enzyme Mix (Invitrogen, Karlsruhe, Germany). In pLEELA, the rapeseed coding sequences are under control of a double 35S promoter. pDEST17 was used for heterologous protein expression during the in vivo E. coli bioconversion assay under the control of the T7 promotor. The following constructs were available from previous studies: pDEST17-AthF3H, pDEST17-AthFLS1 (Busche et al., 2021), pDONR-AthFLS3, pDONR-AthFLS5, pDONR-AthANS (Preuss et al., 2009). The respective BnaFLS CDS sequences are listed in Supplementary File 4.
Flavanone 3-Hydroxylase and Flavonol Synthase Bioconversion Assay in E. coli
The bioconversion assay in E. coli subsequent HPTLC analysis of the methanolic extracts were performed as described in Busche et al. (2021). Successful heterologous expression of the recombinant proteins via SDS-PAGE was shown (Supplementary Figure 3).
Generation of Complementation Lines
The generated pLEELA-BnaFLSX constructs were used to transform the A. thaliana f3h knock out mutant, as well as the ans/fls1 double mutant using the A. tumefaciens strain GV3101:pM90RK (Koncz and Schell, 1986) according to the floral dip protocol (Clough and Bent, 1998). Selection of T1 plants was carried out by BASTA selection. Surviving plants were genotyped for the respective wildtype and mutant alleles, as well as the insertion of the transgene into the genome and its expression via PCR and RT-PCR (Supplementary Table 10). The genotyping for the presence of the transgene was repeated with T2 plants. T2 plants were used for the generation of flavonol-containing methanolic extracts as described below (see section “Flavonol Content Analysis by High-Performance Thin-Layer Chromatography”). T2 plants of the transformed ans/fls1 mutants and T3 plants of the transformed f3h mutants were used for DPBA-staining of young seedlings (see section “In situ Flavonoid Staining of Whole Seedlings”).
Flavonol Content Analysis by High-Performance Thin-Layer Chromatography
The flavonol glycosides were extracted and analyzed as previously described (Stracke et al., 2009). A. thaliana stems were homogenized in 80% methanol and incubated for 15 min at 70°C and then centrifuged for 10 min at 16,100 × g. The supernatants were vacuum-dried at 60°C and sediments were dissolved in 1 μl of 80% methanol mg–1 starting material for HPTLC analysis. In total, 3 μl of each sample were spotted on silica-60 HPTLC-plates. The A. thaliana accessions Col-0 and Nössen-0, as well as the ans/fls1 double mutant were used as controls for the ans/fls1 A. thaliana complementation lines. For the f3h complementation lines, Col-0 and the f3h mutant were used as controls. The mobile phase consisted of a mixture of 66.7% ethyl acetate, 8% formic acid, 8% acetic acid, and 17.3% water. Flavonoid compounds were detected as described before (Stracke et al., 2009).
In situ Flavonoid Staining of Whole Seedlings
The visualization of flavonoids via DPBA-staining with whole seedlings was performed as described (Stracke et al., 2007), with the following minor adaptations: the bleached seedlings were stained to saturation in a freshly prepared aqueous solution of 0.25% (w/v) DPBA, 0.01% (v/v) Triton X-100, and 20% ethanol (v/v).
Results
Flavonol Synthase Family of Brassica napus
We identified a monophyletic group of 13 BnaFLS candidates through phylogenetic analysis using F3H, ANS, and 2-ODD-like protein sequences as outgroup to classify members of the 2-ODD family (Figure 2, Supplementary Figure 1, and Supplementary Table 2) of B. napus. The BnaFLS candidates were further classified within the FLS gene family based on their phylogenetic relationship to their most likely A. thaliana orthologs (Figure 2). Thereby, we identified two BnaFLS1, two BnaFLS2, five BnaFLS3, and four BnaFLS4 candidates in the B. napus cultivar Express 617. BnaFLS1-1 was identified on chromosome C09, while its homeolog BnaFLS1-2 is located on chromosome A09.
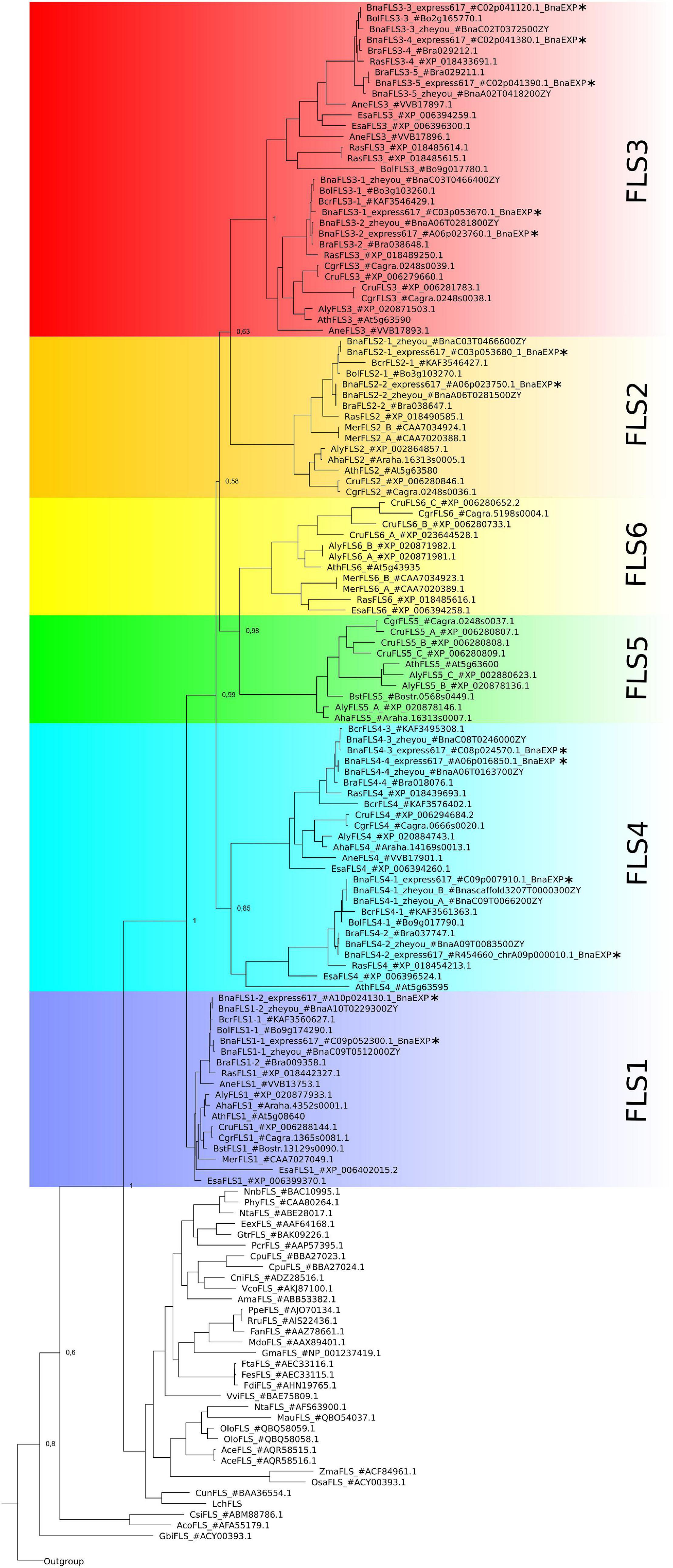
Figure 2. Phylogeny of BnaFLS candidates and previously described FLS sequences. Relative bootstrap-values are shown next to relevant nodes. The phylogenetic tree is based on amino acid sequences. FLS family members of B. napus Express 617 are marked with an asterisk. The outgroup comprises the 2-ODD members ANS and F3H, as well as 2-ODD-like sequences (Supplementary Figure 1).
The genomic structure of the BnaFLS candidate genes comprises 3-4 exons and the encoded proteins display a length range from 270 to 336 amino acids (aa) (Table 1, Supplementary Figure 4, and Supplementary Table 2). Considering the chromosomal rearrangements as described for the cultivar Darmor-bzh (Chalhoub et al., 2014), homeologs were identified (Table 1).
No FLS5 and FLS6 homologs were identified in B. rapa, B. oleracea, and B. napus (Figure 2). Additionally screened B. napus cultivars (Gangan, No2127, Quinta, Shenglii, Tapidor, Westar, ZS11, Zheyou7) were in line with these results. As a FLS6 homolog is present in Raphanus sativus, a very close relative to B. rapa, B. oleracea and B. napus, the latter three might have lost FLS6 very recently. FLS5 was not found in the analyzed species of Brassiceae, Arabideae, Eutremeae, and Coluteocarpeae, while at least one copy was present in Camelineae and Boechereae indicating that FLS5 might have recently emerged in the latter tribes.
Organ- and Temporal-Specific Expression of BnaFLS Candidates
The expression of all BnaFLS candidate genes was analyzed by newly generated and publicly available RNA-Seq data (Table 2 and Supplementary Table 7). As seeds are the major organ for agronomical relevance, we screened for BnaFLS candidates expressed in seeds. In total, five genes were found to be expressed in seeds: BnaFLS1-1, BnaFLS1-2, BnaFLS2-1, BnaFLS3-3, and BnaFLS3-4. These five BnaFLS candidate genes revealed organ- and seed developmental-specific expression patterns (Table 2).
Both BnaFLS1 candidates revealed similar expression patters, showing the highest expression in late anther development, petals, and seeds. The expression of both BnaFLS1s tend to increase in siliques from 10 to 40 days after flowering (DAF). A similar expression pattern was observed in the seed coat revealing a development dependent expression. The biggest differences in BnaFLS1-1 and BnaFLS1-2 expression were observed in the embryo, where BnaFLS1-2 is higher expressed compared to BnaFLS1-1 indicating organ-specific transcriptional regulation at least for this organ. In contrast to BnaFLS1s, both BnaFLS3s are only marginally expressed in anthers and petals. While the expression of BnaFLS1s peaks during late seed and silique development, the expression of both BnaFLS3s peak in the early developmental stages. BnaFLS3-4 is highly expressed during seed coat development. Contrasting expression patterns of BnaFLS3-3 and BnaFLS3-4 were identified in e.g., seed coat samples indicating again organ-specific transcriptional regulation. BnaFLS2-1 was only marginally expressed in all analyzed organs, showing the highest expression in seed coat and roots. In summary, these findings indicate a role of BnaFLS1-1, BnaFLS1-2, BnaFLS2-1, BnaFLS3-3, and BnaFLS3-4 in seeds.
The five BnaFLS candidates expressed in seeds were used for downstream in-depth sequence- and functional analysis of the encoded proteins. The candidates revealed similar genomic structures and an alternative splice variant of BnaFLS2-1 was detected (Figure 3, Supplementary Table 2, and Supplementary Figure 5).

Figure 3. Genomic structure of BnaFLS candidates expressed in seeds. The exon-intron structure of BnaFLS candidates is shown. The exons are split into coding sequences (CDS, black) and untranslated regions (UTR, gray) and are displayed by rectangles, introns are displayed as black connecting lines.
BnaFLS1-1 and BnaFLS1-2 Are Co-expressed With Major Players of the Flavonoid Biosynthesis
To get first insights into which biological pathways the five BnaFLS candidates expressed in seeds might be involved, we identified co-expressed genes (Supplementary Tables 11–15). Interestingly, the genes with the most similar expression pattern to BnaFLS1-1 are part of the flavonoid biosynthesis or the general phenylpropanoid pathway, including 4CL, CHS, CHI, F3H, F3′H, FLS1-2, UGT84A2, GSTF12, and MYB111. Similar results were obtained for BnaFLS1-2, which is co-expressed with homolog(s) of 4CL, CHS, CHI, F3H, FLS1-1, UGT84A2, and MYB111. Both BnaFLS1 genes contain the conserved subgroup 7 MYB-recognition element (MRE) motif in their putative promotor sequences (Supplementary Figure 2).
BnaFLS3-4 was identified to be co-expressed with genes which mostly lack a functional annotation. However, BnaFLS3-4 is strongly co-expressed with a MYB61 homolog. AthMYB61 is a known regulator of seed coat development. For BnaFLS2-1 (Spearman’s correlation coefficient < 0.59) and BnaFLS3-3 (Spearman’s correlation coefficient < 0.69) no genes with strong co-expression could be identified. This is likely due to the very weak expression of BnaFLS2-1 and the broad expression pattern of BnaFLS3-3 (Table 2).
BnaFLS Candidates Share High Amino Acid Sequence Identity to Arabidopsis thaliana 2-ODD Orthologs
To shed light on the potential functionalities of the BnaFLS candidates, the encoded proteins were compared to the well-characterized 2-ODD-members FLS, F3H, and ANS from A. thaliana (Table 3). BnaFLS1-1 and BnaFLS1-2 share > 91% sequence identity to AthFLS1, while BnaFLS2-1 has 57.4% sequence identity to AthFLS2. BnaFLS3-2 and BnaFLS3-3 revealed a sequence identity of 66.8% to AthFLS3. When comparing all BnaFLS candidates to AthF3H and AthANS, the protein identity ranged from 26.7-31 to 33.6-38.4%, respectively. The two BnaFLS1 candidates share 98.2% sequence identity, differing in 6 aa positions, while both BnaFLS3 candidates have 97.4% sequence identity, differing in 8 aa positions. The high sequence similarity between the BnaFLS candidates and their respective AthFLS orthologs implies close structural relationships and related functions.
BnaFLS Candidates Carry Residues Important for Flavonol Synthase and Flavanone 3-Hydroxylase Activity
The five BnaFLS candidates expressed in seeds were analyzed with respect to conserved amino acids and motifs important for FLS functionality (Figure 4). Both BnaFLS1 candidates contain all conserved amino acids and motifs. All remaining candidates lack the motifs potentially important for FLS activity, namely “SxxTxLVP”-, “CPQ/RPxLAL”-, and the N-terminal “PxxxIRxxxEQP,” in parts or completely. However, all BnaFLS candidates possess the conserved residues for ferrous iron- and 2-oxoglutarate-binding. Only BnaFLS2-1 revealed three amino acid exchanges in the five substrate binding residues analyzed, which are H103N, K173R, and E266D. BnaFLS3-3 and BnaFLS3-4 carry a G235A (G261 in AthFLS1) amino acid exchange. As some FLSs are bifunctional showing F3H-side activity, BnaFLS candidates were additionally screened for residues important for F3H activity (Figure 4). Besides the previously described G235A exchange of both BnaFLS3 candidates, all five BnaFLS candidates possess the residues described to play a role for F3H activity. The high conservation of relevant motifs and amino acids suggested both FLS1 candidates to be bifunctional. Due to the incomplete motifs and exchanges in conserved amino acids of BnaFLS3-3, BnaFLS3-4, and BnaFLS2-1 the FLS and/or F3H activity of these candidates might be affected.
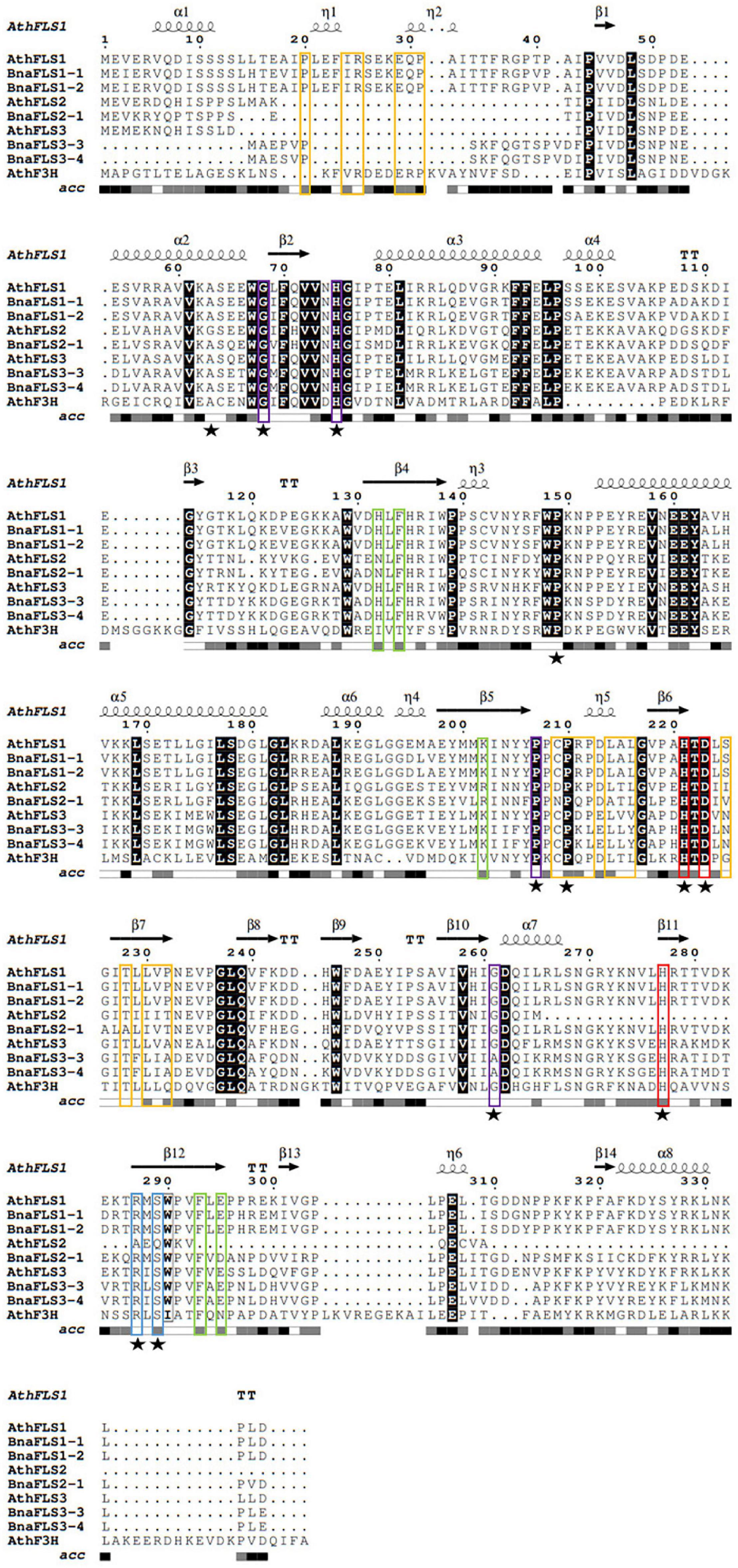
Figure 4. Multiple sequence alignment of BnaFLS candidates relevant for seed flavonol accumulation. Conserved amino acids and motifs important for FLS functionality were labeled as followed: the “PxxxIRxxxEQP,” “CPQ/RPxLAL,” and “SxxTxLVP” motifs are shown in orange, while residues involved in substrate-, ferrous iron-, and 2-oxoglutarate-binding are marked in green, red, and blue, respectively. Residues important for proper folding and/or highly conserved across 2-ODDs are labeled in violet. Residues relevant for F3H activity are marked with a black star. Black background indicates perfect conservation across all sequences. Secondary structure information is derived from an in silico model of AthFLS1 predicted by I-TASSER. acc = relative accessibility.
Moreover, all BnaFLS candidates were predicted to contain no transmembrane helices, signal peptides or N-terminal presequences (mitochondrial-, chloroplast-, thylakoid luminal transfer peptide) and are therefore assumed and predicted to be located in the cytoplasm (Supplementary Tables 2, 4, 5).
Functional Characterization of BnaFLS Candidates
For the functional characterization of BnaFLS1-1, BnaFLS1-2, BnaFLS2-1, BnaFLS3-3, and BnaFLS3-4 in vivo bioconversion assays in E. coli as well as analysis of stablely transformed A. thaliana knock out mutants were performed. The reproducibility of the bioconversion assay was ensured by showing that the observed functionalities of the well-known 2-ODD members AthFLS1, AthFLS3, AthFLS5, AthF3H, and AthANS match literature-based knowledge (Supplementary Figure 6). As expected, AthF3H showed clear F3H activity. In line with previous reports, AthFLS1 was identified as bifunctional possessing FLS activity and F3H side activity and AthANS showed FLS and F3H side activity. None of these activities could be detected for AthFLS5. Although AthFLS3 was reported to have FLS activity under extended assay conditions in E. coli, we could not detect FLS or F3H activity.
BnaFLS1-1 and BnaFLS1-2 Are Bifunctional Enzymes Exhibiting Flavanone 3-Hydroxylase and Flavonol Synthase Activity
The predictions reported above were experimentally validated for BnaFLS1-1 and BnaFLS1-2, which were indeed bifunctional. Both enzymes can generate dihydrokaempferol and kaempferol (Figures 5A,B). To validate bifunctionality in planta, flavonol glycosides of the ans/fls1 A. thaliana double mutants transgenic for BnaFLS1-1 and BnaFLS1-2 were analyzed via HPTLC. In line with the bioconversion assay results, the in planta analysis revealed successful complementation of the ans/fls1 A. thaliana double knock out mutant by BnaFLS1-1 or BnaFLS1-2, restoring the A. thaliana wildtype phenotype (Figure 5C). Additionally, DPBA-staining of young seedlings was used to visualize flavonoid derivatives under UV illumination, including kaempferol (green) and quercetin derivatives (yellow, orange). This in situ validation revealed a restoration of the wildtype phenotype by BnaFLS1-1 and BnaFLS1-2 compared to the f3h and ans/fls1 knock out mutants (Figures 5D,E). Collectively, these results showed that BnaFLS1-1 and BnaFLS1-2 encode bifunctional enzymes, which exhibit FLS and F3H activity.
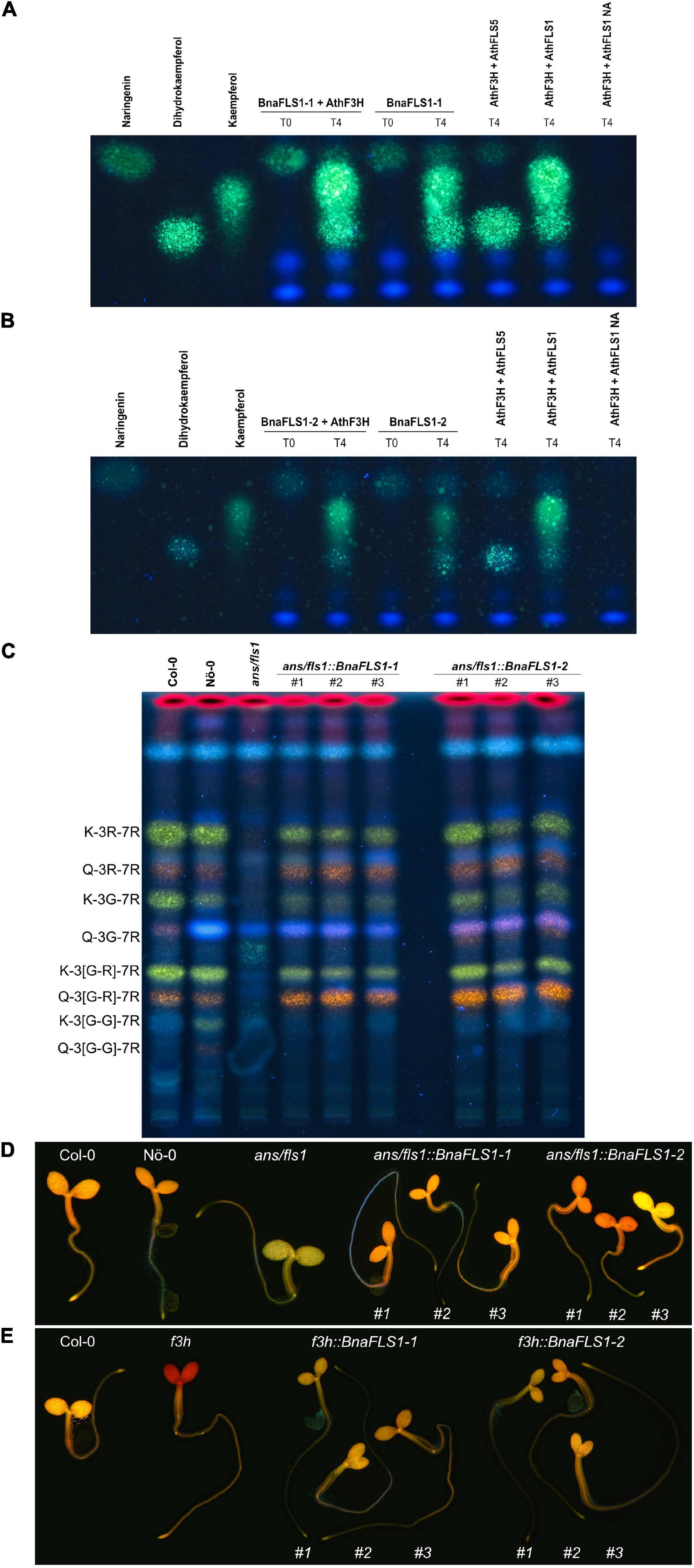
Figure 5. BnaFLS1-1 and BnaFLS1-2 are bifunctional enzymes exhibiting F3H and FLS activity. (A,B) Bioconversion assay results based on a HPTLC using extracts from E. coli expressing recombinant BnaFLS1-1 or BnaFLS1-2. The substrate of F3H naringenin, as well as the FLS substrate dihydrokaempferol and the product kaempferol were used as standards. AthFLS1 served as positive control and AthFLS5 as negative control. In the last sample no Nargingenin (NA) was supplemented. (C) HPTLC on silica gel-60 plates of methanolic extracts of stem of Col-0, Nö-0, ans/fls1 A. thaliana knock out mutant, and three independent T2 ans/fls1 A. thaliana knock out BnaFLS1-1 and BnaFLS1-2 complementation lines followed by DPBA staining, applied in this order. Pictures were taken under UV illumination. Kaempferol- and quercetin derivatives are green and orange respectively, while sinapate derivates are faint blue, dihydrokaempferol derivates are turquois, and chlorophylls appear red. The following flavonoid derivates are labeled: kaempferol-3-O-rhamnoside-7-O-rhamnoside (K-3R-7R), quercetin-3-O-rhamnoside-7-O-rhamnoside (Q-3R-7R), kaempferol-3-O-glucoside-7-O-rhamnoside (K-3G-7R), quercetin-3-O-glucoside-7-O-rhamnoside (Q-3G-7R), kaempferol-3-O-glucorhamnosid-7-O-rhamnoside (K-3[G-R]-7R), quercetin-3-O-glucorhamnosid-7-O-rhamnoside (Q-3[G-R]-7R), kaempferol-3-O-gentiobioside-7-O-rhamnoside (K-3[G-G]-7R), and quercetin-3-O-gentiobioside-7-O-rhamnoside (Q-3[G-G]-7R). (D,E) Flavonol staining in young seedlings of Col-0, Nö-0, ans/fls1 double and f3h single A. thaliana knock out mutant, as well as representative pictures of three independent T2 ans/fls1 A. thaliana knock out BnaFLS1-1 and BnaFLS1-2 complementation lines and three independent T3 f3h A. thaliana knock out BnaFLS1-1 and BnaFLS1-2 complementation lines. Flavonols in norflurazon-bleached seedlings were stained with DPBA until saturation and imaged by epifluorescence microscopy. Orange color indicates the accumulation of quercetin derivates. Photos of representative seedlings are shown.
BnaFLS Family Members With Divergent Enzyme Functionalities
Interestingly, only BnaFLS1-1 and BnaFLS1-2 revealed FLS activity out of the five BnaFLS candidates expressed in seeds. While neither F3H nor FLS activity could be detected for BnaFLS2-1 (Supplementary Figure 7), both BnaFLS3 candidates showed F3H activity in vivo and in planta, thus they can convert naringenin to dihydroflavonols (Figures 6A–E). However, no FLS activity could be detected for both BnaFLS3s (Figures 6A–E). These findings validate the predictions based on the presence of almost all important residues for F3H activity for both BnaFLS3s, with G235A (G261 in AthFLS1) being the only exception (Figure 4).
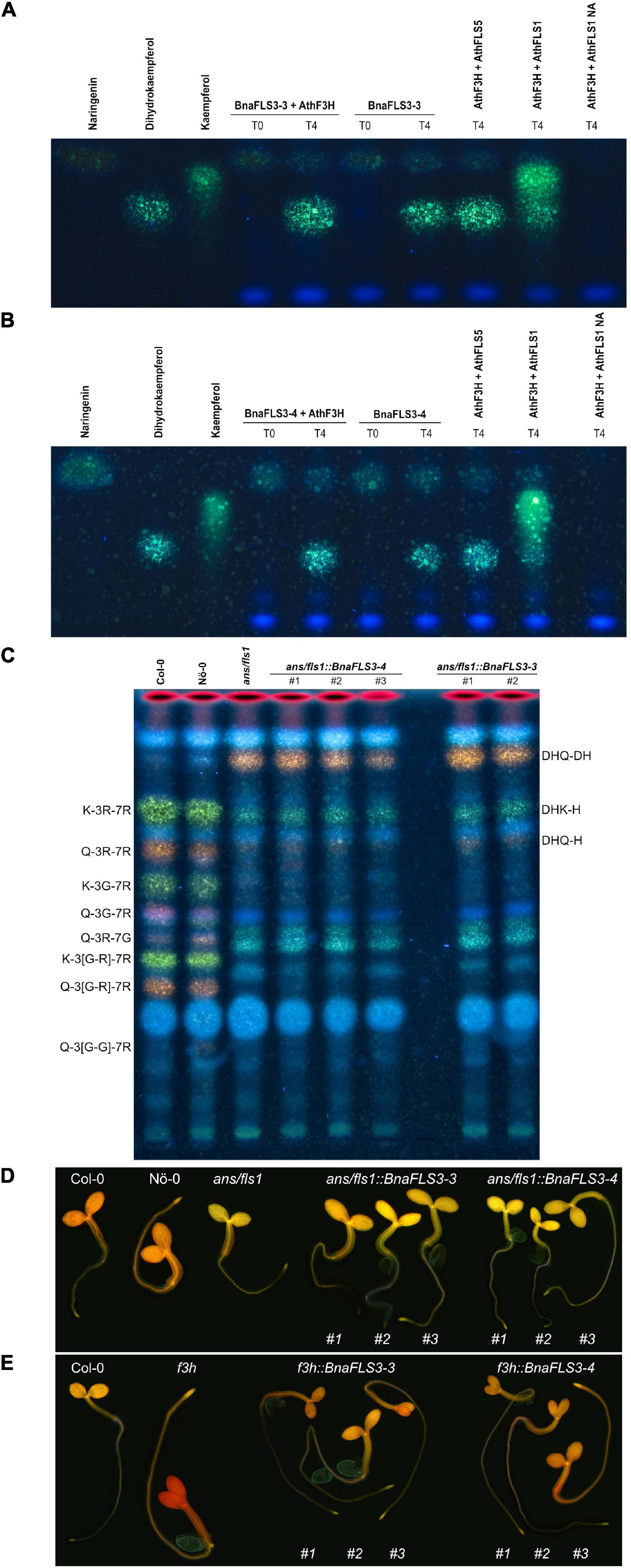
Figure 6. BnaFLS3-3 and BnaFLS3-4 exhibit F3H activity. See Figure 5 for detailed figure description. (A) Bioconversion assay results of BnaFLS3-3 and (B) BnaFLS3-4. (C) The following flavonoid derivates were additionally labeled: dihydroquercetin-deoxyhexoside (DHQ-DH), dihydrokaempferol-hexoside (DHK-H), dihydroquercetin-hexoside (DHQ-H), quercetin-3-O-rhamnoside-7-O-glucoside (Q-3R-7G). (D,E) Flavonol staining in young seedlings.
Structural Modeling Revealed Three Major Differences of the Bifunctional Enzymes Compared to Monofunctional Ones
To investigate whether the bifunctionality of both BnaFLS1s compared to both BnaFLS3s, which showed only F3H activity, might be based on structural differences in silico, 3D models were generated (Figures 7A–F). The BnaFLS1s showed three major differences compared to both BnaFLS3s, which offer insights into the potential mechanisms of bifunctionality: (i) Both BnaFLS3 models revealed a shorter N-terminus compared to BnaFLS1s, resulting in the loss of the presumably FLS-specific “PxxxIRxxxEQP”-motif and α-helices (Figures 4, 7). (ii) The amino acid G261 proposed to be involved in proper folding is only present in both BnaFLS1s, while BnaFLS3s carry an alanine at this position. This residue is located between the transition of a beta-sheet from the jellyroll core structure to an α-helix. The hydrophobic side chain of alanine likely reduces the space in the catalytic center. (iii) Both BnaFLS3s show only partial overlaps with the “SxxTxLVP”- and “CPQ/RPxLAL”-FLS-specific sequence motifs (Figure 4). However, these mismatches do not have a substantial effect on the overall secondary structure in these regions (Figures 7E,F). Moreover, an extended N-terminus is not essential for F3H activity since it is absent in BnaFLS3-3 and BnaFLS3-4 (Figures 7D–F).
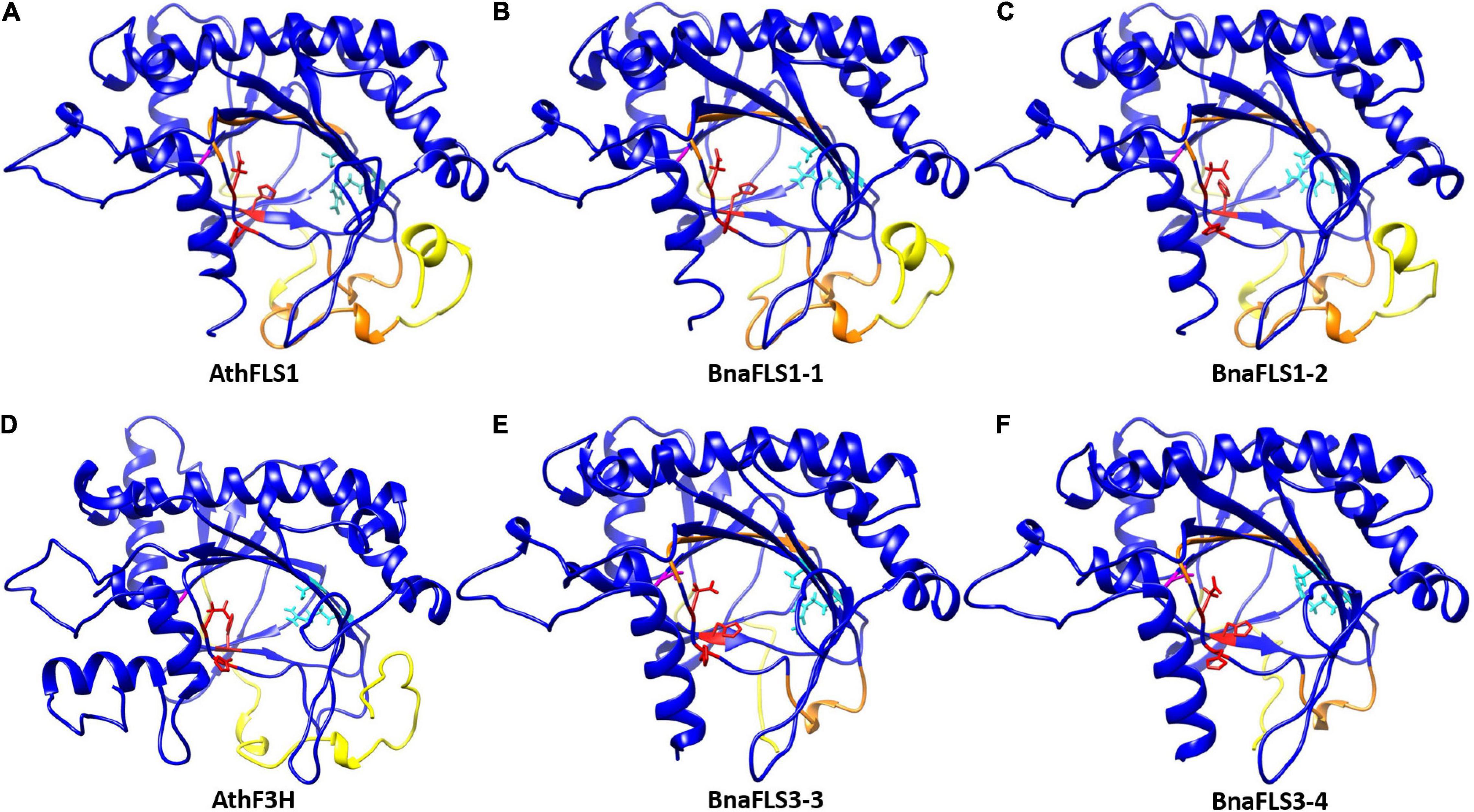
Figure 7. 3D secondary structure models of BnaFLS1s and BnaFLS3s. Homology models of (A) AthFLS1, (B) BnaFLS1-1, (C) BnaFLS1-2, (D) AthF3H, (E) BnaFLS3-3, and (F) BnaFLS3-4 modeled via I-TASSER are shown looking into the center of the jellyroll motif. Ferrous iron-coordinating residues are shown in red, 2-oxoglutarate binding residues are marked in cyan, and the corresponding position of G261 in AthFLS1 is shown in magenta. The N-terminus divergence between BnaFLS1s and BnaFLS3s is marked in yellow (corresponding to amino acids 1-42 in AthFLS1). Orange regions compromise regions postulated to be specific for FLS.
Discussion
Phylogeny of BnaFLS Gene Family Members
Although flavonols are of agronomical, ornamental, nutritional, and health importance, the major players of the flavonol biosynthesis in the oil and protein crop B. napus have not been investigated in great detail yet. So far, only one FLS gene was identified via transient expression in tobacco (Vu et al., 2015). However, as there are several members of the BnaFLS gene family expressed in seeds, it is necessary to characterize the encoding enzymes to infer which genes contribute to flavonol biosynthesis in B. napus seeds.
The members of the BnaFLS gene family are more closely related to each other than to any of the other 2-ODDs, which is in line with the results for the AthFLS gene family (Owens et al., 2008). In contrast to the AthFLS gene family, which is located on chromosome 5 in close proximity (Owens et al., 2008), the members of the BnaFLS gene family are distributed across seven chromosomes. Considering the chromosomal rearrangements described for B. napus cultivar Darmor-bzh (Chalhoub et al., 2014) and also the chromosomal positions of the B. rapa (Guo et al., 2014) and B. oleracea (Parkin et al., 2014) FLS genes, high local synteny of the FLS loci to those of B. napus Express 617 was identified. This syntenic relation allowed the assignment of 6 homeologous pairs of the B. napus FLS gene family. The homeolog pair BnaFLS3-3 and BnaFLS3-4 is located on the pseudochromosome C02 and clusters together with one additional unassigned BnaFLS3-5 homolog. The position of BnaFLS3-4 and BnaFLS3-5 on C02 in the Express 617 assembly likely derives from a mis-assembly as inferred by manual curation of the locus and the frequent assignment of the respective homologs to A02 in other long-read B. napus cultivar assemblies like Westar and Shengli (Song et al., 2020). Moreover, the respective B. rapa homologs BraFLS3-4 (Bra029212) and BraFLS3-5 (Bra029211) are located on A02. BraFLS3-4 and BraFLS3-5 are assumed to originate from duplication of the syntenic AthFLS2 to AthFLS5 tandem array. This duplication is part of the whole genome duplication (WGD), but only some genes of the array were retained in B. rapa (Guo et al., 2014). BraFLS3-5 is assumed to be derived from a gene duplication event of BraFLS3-4 (Guo et al., 2014), which is underlined by the close proximity of the two homologs BnaFLS3-4 and BnaFLS3-5 (only 2.9 kbp apart in the Express 617 assembly, see Table 1). Moreover, BraFLS2-2 (Bra038647) and BraFLS3-2 (Bra038648) are assumed to have emerged by WGD events as described before (Guo et al., 2014). A similar originating mechanism is assumed for BolFLS2-1 (Bo3g103270) and BolFLS3-1 (Bo3g103260) and thus for their respective homologs BnaFLS3-1 and BnaFLS2-1. Therefore, these ancient duplication events shaped the B. napus FLS gene family.
In A. thaliana FLS5 encodes a full-length protein, which contains amino acid exchanges important for hydrogen bonding of the substrate most likely resulting in a non-functional polypeptide (Owens et al., 2008; Preuss et al., 2009). In line with our results, no FLS5 homolog was identified in B. rapa (Guo et al., 2014). However, we could also not detect FLS5 in Brassiceae, Arabideae, Eutremeae, and Coluteocarpeae, but FLS5 was detected in the Camelineae, which include A. thaliana, as well as in Boechereae. Thus, we postulate that FLS5 emerged after the divergence of the common ancestor of the parental species of B. napus (B. rapa and B. oleracea) and A. thaliana rather than that it was frequently lost after the WGD events of the tandem array as postulated by Guo et al., 2014.
FLS6 was characterized as a pseudogene in A. thaliana (Owens et al., 2008; Stracke et al., 2009) and no FLS6 homolog was identified in B. rapa (Guo et al., 2014). These findings are in line with our results showing that FLS6 was lost very recently in B. rapa and B. oleracea and consequently is not present in B. napus, since FLS6 is still present in Raphanus sativus. As FLS6 was identified as pseudogene and FLS5 is known to encode a non-functional protein in A. thaliana (Owens et al., 2008; Preuss et al., 2009; Stracke et al., 2009), the parental species B. oleracea and B. rapa have already eliminated these “unnecessary” genes.
However, some BnaFLS genes are retained as they still encode functional proteins like BnaFLS3-3 and BnaFLS3-4, which encode for proteins with F3H activity. Importantly, both BnaFLS3s show a higher sequence identity with functional FLSs compared to F3H homologs, although exhibiting only F3H activity. This fact provides clear evidence that a classification solely based on amino acid sequences is not sufficient to infer functionalities of FLS family members and very likely 2-ODDs in general.
The BnaFLS Gene Family Contains Two Bifunctional Flavonol Synthases
Bifunctionality has so far not been reported for a FLS from B. napus. By using two independent methods, we demonstrated bifunctionality of the two BnaFLS1 homeologs, which exhibit F3H and FLS activity. Thus, BnaFLS1-1 and BnaFLS1-2 are responsible for flavonol production in planta. We hypothesize that the respective orthologs of B. oleracea (Bo9g174290) and B. rapa (Bra009358) are bifunctional enzymes as well (Supplementary Table 6). Moreover, two additional members of the BnaFLS gene family have been functionally characterized. Interestingly, BnaFLS3-3 and BnaFLS3-4 revealed only F3H activity, while no FLS activity was detected. By incorporating sequence and structural analyses of 3D secondary structure models of BnaFLS1s vs. BnaFLS3s, we proposed a set of evolutionary events underlying the mechanisms of bifunctionality. Both BnaFLS3s lack several amino acids at the beginning of the N-terminus, which could cause the loss of FLS activity as it harbors the “PxxxIRxxxEQP” motif. This motif was previously proposed to be important for FLS activity as it distinguishes FLS from 2-oxoglutarate-/FeII-dependent dioxygenases with other substrate specificities (Owens et al., 2008; Stracke et al., 2009). Additional support for the relevance of this N-terminal region is provided by an AthFLS1 protein lacking the first 21 amino acids which showed no FLS activity (Pelletier et al., 1999; Owens et al., 2008). Moreover, amino acid exchanges in the “CPQ/RPxLAL”- and “SxxTxLVP”-motif in both BnaFLS3s possibly impact FLS activity. In addition, both BnaFLS3s carry a G235A (G261 in AthFLS1) amino acid exchange in comparison to BnaFLS1s, which might be relevant for bifunctionality as this exchange reduced the activity of a mutated Citrus unshiu FLS by 90% (Wellmann et al., 2002). This glycine is conserved across 2-ODDs and is suggested to play a role in proper folding (Wellmann et al., 2002). In accordance, we identified the A235 of BnaFLS3s and G261 of BnaFLS1 located between the transition of a beta-sheet from the jellyroll core structure to an α-helix, thereby the hydrophobic side chain of the alanine might reduce the space in the catalytic center. We propose that FLS bifunctionality is likely influenced by a combination of the identified motifs and residues rather than a single causative change as observed before for other flavonoid enzymes (Gebhardt et al., 2007; Seitz et al., 2007). The impact of each motif or amino acid on FLS bifunctionality needs further investigations that go beyond this study. As these sequence differences of BnaFLS3s do not abolish F3H activity, we uncovered that a truncated N-terminus and G261 are not essential for F3H activity. This is of importance as G261 was reported to be important for F3H activity (Britsch et al., 1993) while it may only play a minor role in conservation of F3H activity.
In addition to the FLS activity of BnaFLS1s, the 2-ODD member ANS might be able to contribute to flavonol production, as AthANS exhibit FLS and F3H side activities in vitro (Turnbull et al., 2004). In planta, FLS is the major enzyme in flavonol production as AthANS was not able to fully substitute AthFLS1 in vivo which is visible in the flavonol deficient fls1-2 mutant (Owens et al., 2008; Stracke et al., 2009).
BnaFLS2-1 is most likely a pseudogene. Although BnaFLS2-1 is still marginally expressed as shown by RNA-Seq data, it carries amino acid exchanges within 3/5 substrate binding residues in addition to a truncated N-terminus, which render the protein non-functional. In addition, an alternative transcript of BnaFLS2-1 was discovered (Supplementary Figure 5) that leads to a frameshift and thus likely encodes a non-functional protein as well. In A. thaliana, a heterologous expressed mutated FLS carrying one of the identified amino acid exchanges, namely K202R (K173R in BnaFLS2-1) is described to possess only 12% of the wild type FLS activity (Chua et al., 2008). In accordance, AthFLS2 encodes a most likely non-functional protein, which also harbors a truncated N-terminus (Owens et al., 2008). We assume that BnaFLS2-1 might be derived from a gene duplication event, losing its original function over time due to a pseudogenization process similar to that proposed for the AthFLS gene family members (Preuss et al., 2009; Stracke et al., 2009). The rather low expression of BnaFLS2-1 across various organs supports this hypothesis.
BnaFLS1s Are Major Players in Flavonol Biosynthesis in Brassica napus Seeds
The spatio-temporal patterns of flavonol accumulation in B. napus are characterized by the activity of multiple BnaFLS genes. Both BnaFLS3s are expressed in early seed development while BnaFLS1s are expressed during late seed development (Table 2). The similar expression patterns of both BnaFLS1s are expected because they are homeologs. Thus, their expression patterns in the parental species B. rapa and B. oleraceae were likely to be very similar as they fulfill similar functions. In line with these results, BnaFLS1-1 and BnaFLS1-2 share co-expressed genes of the flavonoid and phenylpropanoid pathway. Both BnaFLS1s are co-expressed with MYB111, a regulator of flavonol biosynthesis (Stracke et al., 2007) and contain SG7 MRE in their putative promoter regions. Additionally, genes important for flavonoid transport into the vacuole and anthocyanidin/flavonol glycosylation like GSTF12 (TT19) and UGT84A2 (Kitamura et al., 2004; Yonekura-Sakakibara et al., 2012) were identified to be co-expressed with BnaFLS1s. These results further support the role of BnaFLS1-1 and BnaFLS1-2 as major players of flavonol biosynthesis in B. napus seeds. Moreover, transcriptomic and functional analysis of BnaFLS1s indicate gene redundancy.
Both BnaFLS1s were mainly expressed in reproductive organs as observed for AthFLS1 (Owens et al., 2008). BnaFLS3-4 was identified to be co-expressed with the well-known transcription factors MYB61, MYB123, and MYB5 which play a role in flavonoid biosynthesis and seed coat development in A. thaliana (Penfield et al., 2001; Li et al., 2009; Xu et al., 2014). This indicates a likely conserved transcriptional regulation between these two closely related species and supports the importance of flavonols during reproductive processes, e.g., pollen tube growth (Muhlemann et al., 2018).
In line with metabolomic studies showing that phenolic and flavonoid seed content maximized 35 days after flowering (DAF) (Wang et al., 2018), the expression of BnaFLS1s was higher at 35 DAF compared to 23 DAF. In accordance, most kaempferol and quercetin derivates reach their abundance peak at 35 DAF (Wang et al., 2018). Thus the expression pattern of BnaFLS1s fit well with the flavonol accumulation pattern of developing seeds, where flavonols contribute to seed quality (Wang et al., 2018).
Finally, the expression of BnaFLSs family members is not restricted to seeds. Some BnaFLSs were identified to be expressed in roots including BnaFLS3-3 and BnaFLS3-4 indicating a role of those BnaFLS family members in flavonoid biosynthesis in roots.
Future Perspectives in Engineering Flavonol Content in Brassica napus
Engineering and breeding of flavonol content is of agronomical, economical, and ornamental importance (Takahashi et al., 2007; Cook et al., 2013; Yin et al., 2019). Flavonol content in petals influences pollinator attraction and drives microevolution of pollinators (Sheehan et al., 2015; Grotewold, 2016). Moreover, flavonols possess ROS scavenging activities and provide protection against UV-B radiation (Harborne and Williams, 2000). Besides the potential of engineering flavonol biosynthesis, anthocyanin and proanthocyanindin production can be engineered as FLS and DFR compete for substrates (Figure 8), thereby influencing important agronomical traits e.g., seed color (Luo et al., 2016). This study identified two bifunctional BnaFLS1s which are highly expressed in seeds and can thus be harnessed to engineer the metabolic flux of seed flavonol biosynthesis in the future (Figure 8). For example, the main bitter off-taste component in rapeseed protein isolates is kaempferol 3-O-(2″′-O-Sinapoyl-β-sophoroside) (Hald et al., 2019). Thus, the results of this study provide the basis for breeding low-phenolics lines with focus on the reduction of e.g., kaempferols in seeds, thereby supporting the use of rapeseed protein in human consumption.
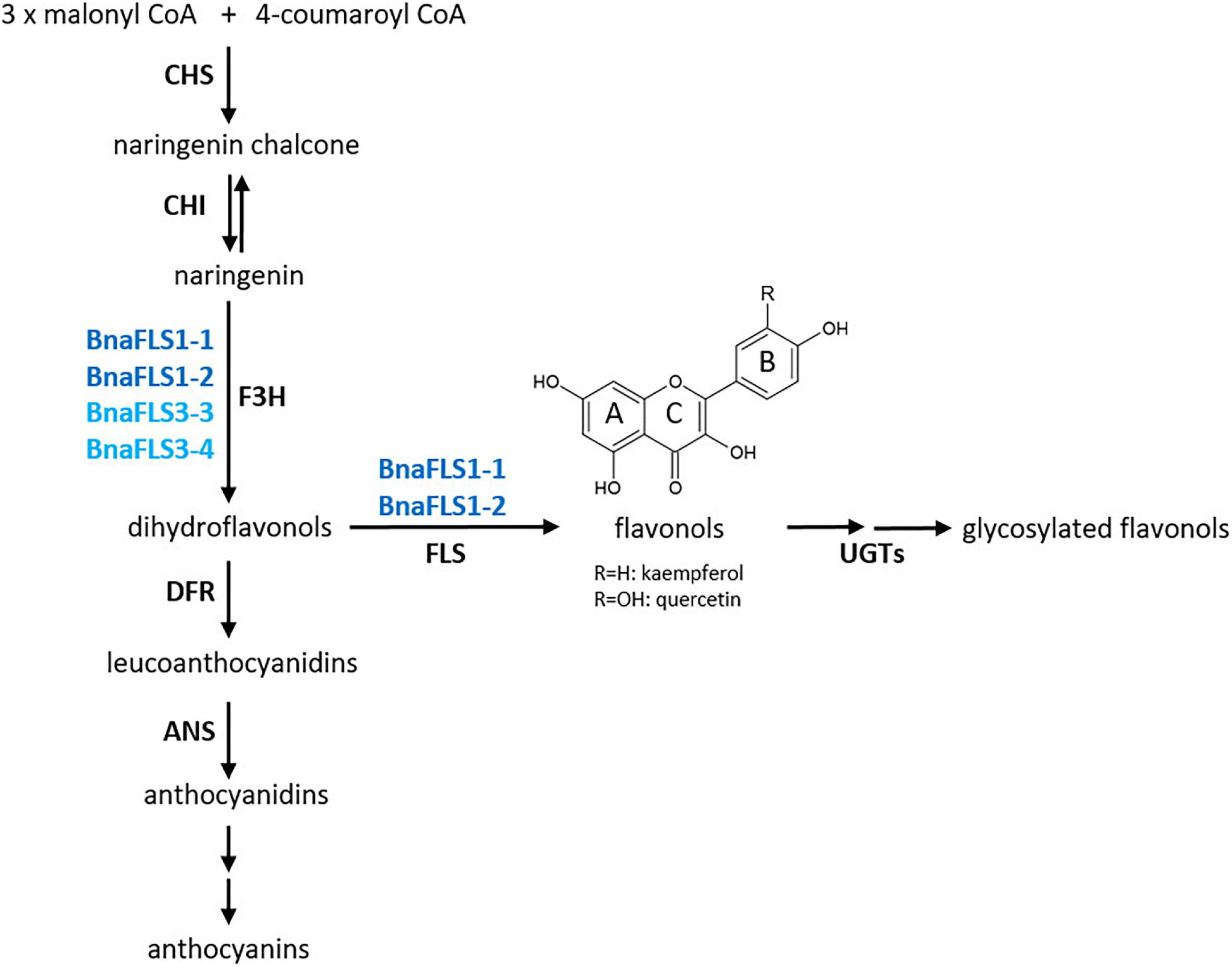
Figure 8. Functional activities of the B. napus flavonol synthase family. BnaFLS1-1 and BnaFLS1-2 marked in dark blue, are bifunctional enzyme exhibiting F3H and FLS activity. BnaFLS3-3 and BnaFLS3-4 labeled in light blue possess F3H activity.
Data Availability Statement
The original contributions presented in the study are publicly available. The RNA-Seq data sets generated for this study can be found in the ENA/NCBI BioProject PRJEB45399.
Author Contributions
HS, DH, and BW conceived and designed research. HS, MS, TB, MB, and PV investigated and conducted experiments. HS performed bioinformatic analyses and data curation, and wrote the initial draft manuscript. HS, BW, DH, MB, and TB revised the manuscript. All authors contributed to the article and approved the submitted version.
Funding
This research was funded by the BMBF project RaPEQ, Grant Numbers “FKZ 031B0198A” and “FKZ 031B0888A.” We acknowledge support for the publication costs (APC) by the Open Access Publication Fund of Bielefeld University.
Conflict of Interest
The authors declare that the research was conducted in the absence of any commercial or financial relationships that could be construed as a potential conflict of interest.
Publisher’s Note
All claims expressed in this article are solely those of the authors and do not necessarily represent those of their affiliated organizations, or those of the publisher, the editors and the reviewers. Any product that may be evaluated in this article, or claim that may be made by its manufacturer, is not guaranteed or endorsed by the publisher.
Acknowledgments
We are extremely grateful to all researchers who submitted their B. napus RNA-Seq data sets to the appropriate databases and published their experimental findings. We thank Ralf Stracke for critical proof-reading and discussion. Moreover, we are grateful to Andrea Voigt for excellent technical assistance. In addition, we thank Nele Tiemann for her help in constructing BnaFLS1-2 plasmids. We thank Rod Snowdon and Huey Tyng Lee for their support and early excess to the B. napus Express 617 reference genome sequence. We thank Christian Möllers for supporting us with viable seeds of Express 617. We thank the Sequencing Core Facility for doing an excellent job in determining DNA sequences. We thank the Center for Biotechnology (CeBiTec) at Bielefeld University for providing an environment to perform the computational analyses.
Supplementary Material
The Supplementary Material for this article can be found online at: https://www.frontiersin.org/articles/10.3389/fpls.2021.733762/full#supplementary-material
Supplementary File 1 | List of plant 2-ODDs amino acid sequences used in phylogenetic analysis.
Supplementary File 2 | 3D secondary structure models used in this work.
Supplementary File 3 | Corrected structural annotation of BnaFLS genes.
Supplementary File 4 | CDS of BnaFLSs from this work.
Footnotes
- ^ http://tree.bio.ed.ac.uk/software/figtree/
- ^ https://github.com/hschilbert/BnaFLS
- ^ https://www.ncbi.nlm.nih.gov/sra
- ^ https://github.com/ncbi/sra-tools
References
Agati, G., Stefano, G., Biricolti, S., and Tattini, M. (2009). Mesophyll distribution of ’antioxidant’ flavonoid glycosides in Ligustrum vulgare leaves under contrasting sunlight irradiance. Ann. Bot. 104, 853–861. doi: 10.1093/aob/mcp177
Almagro Armenteros, J. J., Tsirigos, K. D., Sonderby, C. K., Petersen, T. N., Winther, O., Brunak, S., et al. (2019b). SignalP 5.0 improves signal peptide predictions using deep neural networks. Nat. Biotechnol. 37, 420–423. doi: 10.1038/s41587-019-0036-z
Almagro Armenteros, J. J., Salvatore, M., Emanuelsson, O., Winther, O., von Heijne, G., Elofsson, A., et al. (2019a). Detecting sequence signals in targeting peptides using deep learning. Life Sci. Alliance 2:e201900429. doi: 10.26508/lsa.201900429
Andrews, S. (2018). FastQC, a quality control tool for high throughput sequence data. Available online at: http://www.bioinformatics.babraham.ac.uk/projects/fastqc/ (accessed October 6, 2018)
Appelhagen, I., Thiedig, K., Nordholt, N., Schmidt, N., Huep, G., Sagasser, M., et al. (2014). Update on transparent testa mutants from Arabidopsis thaliana: characterisation of new alleles from an isogenic collection. Planta 240, 955–970. doi: 10.1007/s00425-014-2088-0
Auger, B., Marnet, N., Gautier, V., Maia-Grondard, A., Leprince, F., Renard, M., et al. (2010). A detailed survey of seed coat flavonoids in developing seeds of Brassica napus L. J. Agricult. Food Chem. 58, 6246–6256. doi: 10.1021/jf903619v
Battat, M., Eitan, A., Rogachev, I., Hanhineva, K., Fernie, A., Tohge, T., et al. (2019). A MYB Triad Controls Primary and Phenylpropanoid Metabolites for Pollen Coat Patterning. Plant Physiol. 180, 87–108. doi: 10.1104/pp.19.00009
Bray, N. L., Pimentel, H., Melsted, P., and Pachter, L. (2016). Near-optimal probabilistic RNA-seq quantification. Nat. Biotechnol. 34, 525–527. doi: 10.1038/nbt.3519
Britsch, L., Dedio, J., Saedler, H., and Forkmann, G. (1993). Molecular characterization of flavanone 3 beta-hydroxylases. Consensus sequence, comparison with related enzymes and the role of conserved histidine residues. Eur. J. Biochem. 217, 745–754. doi: 10.1111/j.1432-1033.1993.tb18301.x
Britsch, L., Heller, W., and Grisebach, H. (1981). Conversion of flavanone to flavone, dihydroflavonol to flavonol with enzyme systems from cell cultures of parsley. Zeitschrift fur Naturforschung. C J. Biosci. 36, 742–750. doi: 10.1515/znc-1981-9-1009
Busche, M., Acatay, C., Martens, S., Weisshaar, B., and Stracke, R. (2021). Functional characterisation of banana (Musa spp.) 2-oxoglutarate- dependent dioxygenases involved in flavonoid biosynthesis. Front. Plant Sci. 12:701780. doi: 10.3389/fpls.2021.701780
Chalhoub, B., Denoeud, F., Liu, S., Parkin, I. A., Tang, H., Wang, X., et al. (2014). Early allopolyploid evolution in the post-neolithic Brassica napus oilseed genome. Science 345, 950–953. doi: 10.1126/science.1253435
Cheng, A. X., Han, X. J., Wu, Y. F., and Lou, H. X. (2014). The function and catalysis of 2-oxoglutarate-dependent oxygenases involved in plant flavonoid biosynthesis. Int. J. Mol. Sci. 15, 1080–1095. doi: 10.3390/ijms15011080
Cheng, C. Y., Krishnakumar, V., Chan, A., Thibaud-Nissen, F., Schobel, S., and Town, C. D. (2017). Araport11: a complete reannotation of the Arabidopsis thaliana reference genome. Plant J. 89, 789–804. doi: 10.1111/tpj.13415
Chou, K. C., and Shen, H. B. (2010). Plant-mPLoc: a top-down strategy to augment the power for predicting plant protein subcellular localization. PLoS One 5:e11335. doi: 10.1371/journal.pone.0011335
Chua, C. S., Biermann, D., Goo, K. S., and Sim, T. S. (2008). Elucidation of active site residues of Arabidopsis thaliana flavonol synthase provides a molecular platform for engineering flavonols. Phytochemistry 69, 66–75. doi: 10.1016/j.phytochem.2007.07.006
Clough, S. J., and Bent, A. F. (1998). Floral dip: a simplified method for Agrobacterium-mediated transformation of Arabidopsis thaliana. Plant J. 16, 735–743. doi: 10.1046/j.1365-313x.1998.00343.x
Cook, S. M., Skellern, M. P., Döring, T. F., and Pickett, J. A. (2013). Red oilseed rape? The potential for manipulation of petal colour in control strategies for the pollen beetle (Meligethes aeneus). Arthropod Plant Interact. 7, 249–258. doi: 10.1007/s11829-013-9252-5
Dobin, A., Davis, C. A., Schlesinger, F., Drenkow, J., Zaleski, C., Jha, S., et al. (2013). STAR: ultrafast universal RNA-seq aligner. Bioinformatics 29, 15–21. doi: 10.1093/bioinformatics/bts635
Downey, M. O., Harvey, J. S., and Robinson, S. P. (2003). Synthesis of flavonols and expression of flavonol synthase genes in the developing grape berries of Shiraz and Chardonnay (Vitis vinifera L.). Austral. J. Grape Wine Res. 9, 110–121. doi: 10.1111/j.1755-0238.2003.tb00261.x
Emms, D. M., and Kelly, S. (2019). OrthoFinder: phylogenetic orthology inference for comparative genomics. Genome Biol. 20:238. doi: 10.1186/s13059-019-1832-y
Falcone Ferreyra, M. L., Casas, M. I., Questa, J. I., Herrera, A. L., Deblasio, S., Wang, J., et al. (2012). Evolution and expression of tandem duplicated maize flavonol synthase genes. Front. Plant Sci. 3:101. doi: 10.3389/fpls.2012.00101
Forkmann, G., De Vlaming, P., Spribille, R., Wiering, H., and Schram, A. W. (1986). Genetic and biochemical studies on the conversion of dihydroflavonols to flavonols in flowers of Petunia hybrida. Zeitschrift fur Naturforschung C J. Biosci. 41, 179–186. doi: 10.1515/znc-1986-1-227
Fujita, A., Goto-Yamamoto, N., Aramaki, I., and Hashizume, K. (2006). Organ-specific transcription of putative flavonol synthase genes of grapevine and effects of plant hormones and shading on flavonol biosynthesis in grape berry skins. Biosci. Biotechnol. Biochem. 70, 632–638. doi: 10.1271/bbb.70.632
Gasteiger, E., Hoogland, C., Gattiker, A., Duvaud, S. E., Wilkins, M. R., Appel, R. D., et al. (2005). Protein Identification and Analysis Tools on the ExPASy Server. Proteom. Protoc. Handb. 2005, 571–607. doi: 10.1385/1-59259-890-0:571
Gebhardt, Y. H., Witte, S., Steuber, H., Matern, U., and Martens, S. (2007). Evolution of flavone synthase I from parsley flavanone 3beta-hydroxylase by site-directed mutagenesis. Plant Physiol. 144, 1442–1454. doi: 10.1104/pp.107.098392
Grotewold, E. (2016). Flavonols drive plant microevolution. Nat. Genet. 48, 112–113. doi: 10.1038/ng.3490
Guo, N., Cheng, F., Wu, J., Liu, B., Zheng, S., Liang, J., et al. (2014). Anthocyanin biosynthetic genes in Brassica rapa. BMC Genomics 15:426. doi: 10.1186/1471-2164-15-426
Hahlbrock, K., and Scheel, D. (1989). Physiology and molecular biology of phenylpropanoid metabolism. Annu. Rev. Plant Physiol. Plant Mol. Biol. 40, 347–369. doi: 10.1146/annurev.pp.40.060189.002023
Hald, C., Dawid, C., Tressel, R., and Hofmann, T. (2019). Kaempferol 3-O-(2”’-O-sinapoyl-β-sophoroside) Causes the Undesired Bitter Taste of Canola/Rapeseed Protein Isolates. J. Agricult. Food Chem. 67, 372–378. doi: 10.1021/acs.jafc.8b06260
Harborne, J. B., and Williams, C. A. (2000). Advances in flavonoid research since 1992. Phytochemistry 55, 481–504. doi: 10.1016/s0031-9422(00)00235-1
Hartmann, U., Sagasser, M., Mehrtens, F., Stracke, R., and Weisshaar, B. (2005). Differential combinatorial interactions of cis-acting elements recognized by R2R3-MYB, BZIP, and BHLH factors control light-responsive and tissue-specific activation of phenylpropanoid biosynthesis genes. Plant Mol. Biol. 57, 155–171. doi: 10.1007/s11103-004-6910-0
Holton, T. A., Brugliera, F., and Tanaka, Y. (1993). Cloning and expression of flavonol synthase from Petunia hybrida. Plant J. 4, 1003–1010. doi: 10.1046/j.1365-313x.1993.04061003.x
Hu, B., Jin, J., Guo, A. Y., Zhang, H., Luo, J., and Gao, G. (2015). GSDS 2.0: an upgraded gene feature visualization server. Bioinformatics 31, 1296–1297. doi: 10.1093/bioinformatics/btu817
Jakoby, M., Wang, H. Y., Reidt, W., Weisshaar, B., and Bauer, P. (2004). FRU (BHLH029) is required for induction of iron mobilization genes in Arabidopsis thaliana. FEBS Lett. 577, 528–534. doi: 10.1016/j.febslet.2004.10.062
Jiang, X., Shi, Y., Fu, Z., Li, W. W., Lai, S., Wu, Y., et al. (2020). Functional characterization of three flavonol synthase genes from Camellia sinensis: Roles in flavonol accumulation. Plant Sci. 300:110632.
Katoh, K., and Standley, D. M. (2013). MAFFT multiple sequence alignment software version 7: improvements in performance and usability. Mol. Biol. Evolut. 30, 772–780. doi: 10.1093/molbev/mst010
Kawai, Y., Ono, E., and Mizutani, M. (2014). Evolution and diversity of the 2-oxoglutarate-dependent dioxygenase superfamily in plants. Plant J. 78, 328–343. doi: 10.1111/tpj.12479
Kitamura, S., Shikazono, N., and Tanaka, A. (2004). TRANSPARENT TESTA 19 is involved in the accumulation of both anthocyanins and proanthocyanidins in Arabidopsis. Plant J. 37, 104–114. doi: 10.1046/j.1365-313x.2003.01943.x
Koncz, C., and Schell, J. (1986). The promoter of TL-DNA gene 5 controls the tissue-specific expression of chimaeric genes carried by a novel type of Agrobacterium binary vector. Mol. Genet. Genom. 204, 383–396. doi: 10.1007/bf00331014
Krogh, A., Larsson, B., von Heijne, G., and Sonnhammer, E. L. L. (2001). Predicting transmembrane protein topology with a hidden markov model: application to complete genomes. J. Mol. Biol. 305, 567–580. doi: 10.1006/jmbi.2000.4315
Lee, H., Chawla, H. S., Obermeier, C., Dreyer, F., Abbadi, A., and Snowdon, R. (2020). Chromosome-Scale Assembly of Winter Oilseed Rape Brassica napus. Front. Plant Sci. 11:496. doi: 10.3389/fpls.2020.00496
Li, S. F., Milliken, O. N., Pham, H., Seyit, R., Napoli, R., Preston, J., et al. (2009). The Arabidopsis MYB5 transcription factor regulates mucilage synthesis, seed coat development, and trichome morphogenesis. Plant Cell 21, 72–89. doi: 10.1105/tpc.108.063503
Lukacin, R., Wellmann, F., Britsch, L., Martens, S., and Matern, U. (2003). Flavonol synthase from Citrus unshiu is a bifunctional dioxygenase. Phytochemistry 62, 287–292. doi: 10.1016/S0031-9422(02)00567-8
Luo, P., Ning, G., Wang, Z., Shen, Y., Jin, H., Li, P., et al. (2016). Disequilibrium of Flavonol Synthase and Dihydroflavonol-4-Reductase Expression Associated Tightly to White vs. Red Color Flower Formation in Plants. Front. Plant Sci. 6:1257. doi: 10.3389/fpls.2015.01257
Mehrtens, F., Kranz, H., Bednarek, P., and Weisshaar, B. (2005). The Arabidopsis transcription factor MYB12 is a flavonol-specific regulator of phenylpropanoid biosynthesis. Plant Physiol. 138, 1083–1096. doi: 10.1104/pp.104.058032
Muhlemann, J. K., Younts, T. L. B., and Muday, G. K. (2018). Flavonols control pollen tube growth and integrity by regulating ROS homeostasis during high-temperature stress. PNAS 115, E11188–E11197. doi: 10.1073/pnas.1811492115
Nesi, N., Delourme, R., Bregeon, M., Falentin, C., and Renard, M. (2008). Genetic and molecular approaches to improve nutritional value of Brassica napus L. seed. Comptes Rendus Biol. 331, 763–771. doi: 10.1016/j.crvi.2008.07.018
Olsen, K. M., Slimestad, R., Lea, U. S., Brede, C., Lovdal, T., Ruoff, P., et al. (2009). Temperature and nitrogen effects on regulators and products of the flavonoid pathway: experimental and kinetic model studies. Plant Cell Environ. 32, 286–299. doi: 10.1111/j.1365-3040.2008.01920.x
Owens, D. K., Alerding, A. B., Crosby, K. C., Bandara, A. B., Westwood, J. H., and Winkel, B. S. (2008). Functional analysis of a predicted flavonol synthase gene family in Arabidopsis. Plant Physiol. 147, 1046–1061. doi: 10.1104/pp.108.117457
Park, S., Kim, D. H., Lee, J. Y., Ha, S. H., and Lim, S. H. (2017). Comparative Analysis of Two Flavonol Synthases from Different-Colored Onions Provides Insight into Flavonoid Biosynthesis. J. Agricult. Food Chem. 65, 5287–5298. doi: 10.1021/acs.jafc.7b01036
Park, S., Kim, D. H., Park, B. R., Lee, J. Y., and Lim, S. H. (2019). Molecular and Functional Characterization of Oryza sativa Flavonol Synthase (OsFLS), a Bifunctional Dioxygenase. J. Agricult. Food Chem. 67, 7399–7409. doi: 10.1021/acs.jafc.9b02142
Parkin, I. A., Koh, C., Tang, H., Robinson, S. J., Kagale, S., Clarke, W. E., et al. (2014). Transcriptome and methylome profiling reveals relics of genome dominance in the mesopolyploid Brassica oleracea. Genome Biol. 15:R77.
Peer, W. A., and Murphy, A. S. (2007). Flavonoids and auxin transport: modulators or regulators? Trends Plant Sci. 12, 556–563. doi: 10.1016/j.tplants.2007.10.003
Pelletier, M. K., Burbulis, I. E., and Shirley, B. W. (1999). Disruption of specific flavonoid genes enhances the accumulation of flavonoid enzymes and endproducts in Arabidopsis seedlings. Plant Mol. Biol. 40, 45–54.
Pelletier, M. K., Murrell, J. R., and Shirley, B. W. (1997). Characterization of flavonol synthase and leucoanthocyanidin dioxygenase genes in arabidopsis. Plant Physiol. 113, 1437–1445. doi: 10.1104/pp.113.4.1437
Penfield, S., Meissner, R. C., Shoue, D. A., Carpita, N. C., and Bevan, M. W. (2001). MYB61 Is Required for Mucilage Deposition and Extrusion in the Arabidopsis Seed Coat. Plant Cell 13, 2777–2791. doi: 10.1105/tpc.13.12.2777
Pettersen, E. F., Goddard, T. D., Huang, C. C., Couch, G. S., Greenblatt, D. M., Meng, E. C., et al. (2004). UCSF Chimera–a visualization system for exploratory research and analysis. J. Computat. Chem. 25, 1605–1612. doi: 10.1002/jcc.20084
Prescott, A. G., and John, P. (1996). DIOXYGENASES: Molecular Structure and Role in Plant Metabolism. Annu. Rev. Plant Physiol. Plant Mol. Biol. 47, 245–271. doi: 10.1146/annurev.arplant.47.1.245
Prescott, A. G., Stamford, N. P. J., Wheeler, G., and Firmin, J. L. (2002). In vitro properties of a recombinant flavonol synthase from Arabidopsis thaliana. Phytochemistry 60, 589–593. doi: 10.1016/s0031-9422(02)00155-3
Preuss, A., Stracke, R., Weisshaar, B., Hillebrecht, A., Matern, U., and Martens, S. (2009). Arabidopsis thaliana expresses a second functional flavonol synthase. FEBS Lett. 583, 1981–1986. doi: 10.1016/j.febslet.2009.05.006
Price, M. N., Dehal, P. S., and Arkin, A. P. (2009). FastTree: computing large minimum evolution trees with profiles instead of a distance matrix. Mol. Biol. Evolut. 26, 1641–1650. doi: 10.1093/molbev/msp077
Pucker, B., Holtgräwe, D., and Weisshaar, B. (2017). Consideration of non-canonical splice sites improves gene prediction on the Arabidopsis thaliana Niederzenz-1 genome sequence. BMC Res. Notes 10:667. doi: 10.1186/s13104-017-2985-y
Pucker, B., Holtgräwe, D., Rosleff Sörensen, T., Stracke, R., Viehöver, P., and Weisshaar, B. (2016). A De Novo Genome Sequence Assembly of the Arabidopsis thaliana Accession Niederzenz-1 Displays Presence/Absence Variation and Strong Synteny. PLoS One 11:e0164321. doi: 10.1371/journal.pone.0164321
Pucker, B., Reiher, F., and Schilbert, H. M. (2020). Automatic Identification of Players in the Flavonoid Biosynthesis with Application on the Biomedicinal Plant Croton tiglium. Plants 9:1103. doi: 10.3390/plants9091103
Qu, C., Zhao, H., Fu, F., Wang, Z., Zhang, K., Zhou, Y., et al. (2016). Genome-Wide Survey of Flavonoid Biosynthesis Genes and Gene Expression Analysis between Black- and Yellow-Seeded Brassica napus. Front. Plant Sci. 7:1755. doi: 10.3389/fpls.2016.01755
Robert, X., and Gouet, P. (2014). Deciphering key features in protein structures with the new ENDscript server. Nucleic Acids Res. 42, W320–W324. doi: 10.1093/nar/gku316
Roy, A., Kucukural, A., and Zhang, Y. (2010). I-TASSER: a unified platform for automated protein structure and function prediction. Nat. Protoc. 5, 725–738. doi: 10.1038/nprot.2010.5
Seitz, C., Ameres, S., and Forkmann, G. (2007). Identification of the molecular basis for the functional difference between flavonoid 3′-hydroxylase and flavonoid 3′,5′-hydroxylase. FEBS Lett. 581, 3429–3434. doi: 10.1016/j.febslet.2007.06.045
Shan, X., Li, Y., Yang, S., Yang, Z., Qiu, M., Gao, R., et al. (2020). The spatio-temporal biosynthesis of floral flavonols is controlled by differential phylogenetic MYB regulators in Freesia hybrida. N. Phytol. 228, 1864–1879. doi: 10.1111/nph.16818
Sheehan, H., Moser, M., Klahre, U., Esfeld, K., Dell’Olivo, A., Mandel, T., et al. (2015). MYB-FL controls gain and loss of floral UV absorbance, a key trait affecting pollinator preference and reproductive isolation. Nat. Genet. 48, 159–166. doi: 10.1038/ng.3462
Song, J. M., Guan, Z., Hu, J., Guo, C., Yang, Z., Wang, S., et al. (2020). Eight high-quality genomes reveal pan-genome architecture and ecotype differentiation of Brassica napus. Nat. Plants 6, 34–45. doi: 10.1038/s41477-019-0577-7
Stracke, R., De Vos, R. C. H., Bartelniewoehner, L., Ishihara, H., Sagasser, M., Martens, S., et al. (2009). Metabolomic and genetic analyses of flavonol synthesis in Arabidopsis thaliana support the in vivo involvement of leucoanthocyanidin dioxygenase. Planta 229, 427–445. doi: 10.1007/s00425-008-0841-y
Stracke, R., Ishihara, H., Huep, G., Barsch, A., Mehrtens, F., Niehaus, K., et al. (2007). Differential regulation of closely related R2R3-MYB transcription factors controls flavonol accumulation in different parts of the Arabidopsis thaliana seedling. Plant J. 50, 660–677. doi: 10.1111/j.1365-313X.2007.03078.x
Stracke, R., Jahns, O., Keck, M., Tohge, T., Niehaus, K., Fernie, A. R., et al. (2010). Analysis of PRODUCTION OF FLAVONOL GLYCOSIDES-dependent flavonol glycoside accumulation in Arabidopsis thaliana plants reveals MYB11-, MYB12- and MYB111-independent flavonol glycoside accumulation. N. Phytol. 188, 985–1000. doi: 10.1111/j.1469-8137.2010.03421.x
Takahashi, R., Githiri, S. M., Hatayama, K., Dubouzet, E. G., Shimada, N., Aoki, T., et al. (2007). A single-base deletion in soybean flavonol synthase gene is associated with magenta flower color. Plant Mol. Biol. 63, 125–135. doi: 10.1007/s11103-006-9077-z
Turnbull, J. J., Nakajima, J., Welford, R. W., Yamazaki, M., Saito, K., and Schofield, C. J. (2004). Mechanistic studies on three 2-oxoglutarate-dependent oxygenases of flavonoid biosynthesis: anthocyanidin synthase, flavonol synthase, and flavanone 3-beta-hydroxylase. J. Biol. Chem. 279, 1206–1216. doi: 10.1074/jbc.M309228200
Usadel, B., Obayashi, T., Mutwil, M., Giorgi, F. M., Bassel, G. W., Tanimoto, M., et al. (2009). Co-expression tools for plant biology: opportunities for hypothesis generation and caveats. Plant Cell Environ. 32, 1633–1651. doi: 10.1111/j.1365-3040.2009.02040.x
Vu, T. T., Jeong, C. Y., Nguyen, H. N., Lee, D., Lee, S. A., Kim, J. H., et al. (2015). Characterization of Brassica napus Flavonol Synthase Involved in Flavonol Biosynthesis in Brassica napus L. J. Agricult. Food Chem. 63, 7819–7829. doi: 10.1021/acs.jafc.5b02994
Wanasundara, J. P. D., McIntosh, T. C., Perera, S. P., Withana-Gamage, T. S., and Mitra, P. (2016). Canola/rapeseed protein-functionality and nutrition. Oilseeds Fats Crops Lipids 23:2016028. doi: 10.1051/ocl/2016028
Wang, X., Wang, H., Wang, J., Sun, R., Wu, J., Liu, S., et al. (2011). The genome of the mesopolyploid crop species Brassica rapa. Nat. Genet. 43, 1035–1039. doi: 10.1038/ng.919
Wang, Y., Meng, G., Chen, S., Chen, Y., Jiang, J., and Wang, Y. P. (2018). Correlation Analysis of Phenolic Contents and Antioxidation in Yellow- and Black-Seeded Brassica napus. Molecules 23:1815. doi: 10.3390/molecules23071815
Weisshaar, B., and Jenkins, G. I. (1998). Phenylpropanoid biosynthesis and its regulation. Curr. Opin. Plant Biol. 1, 251–257. doi: 10.1016/S1369-5266(98)80113-1
Welford, R. W. D., Turnbull, J. J., Claridge, T. D. W., Prescott, A. G., and Schofield, C. J. (2001). Evidence for oxidation at C-3 of the flavonoid C-ring during anthocyanin biosynthesis. Chem. Commun. 1828–1829. doi: 10.1039/b105576n
Wellmann, F., Lukacin, R., Moriguchi, T., Britsch, L., Schiltz, E., and Matern, U. (2002). Functional expression and mutational analysis of flavonol synthase from Citrus unshiu. Eur. J. Biochem. 269, 4134–4142. doi: 10.1046/j.1432-1033.2002.03108.x
Williams, C. A., and Grayer, R. J. (2004). Anthocyanins and other flavonoids. Nat. Prod. Rep. 21, 539–573. doi: 10.1039/b311404j
Winkel-Shirley, B. (2001). Flavonoid biosynthesis. A colorful model for genetics, biochemistry, cell biology, and biotechnology. Plant Physiol. 126, 485–493. doi: 10.1104/pp.126.2.485
Winkel-Shirley, B. (2002). Biosynthesis of flavonoids and effects of stress. Curr. Opin. Plant Biol. 5, 218–223. doi: 10.1016/S1369-5266(02)00256-X
Wisman, E., Hartmann, U., Sagasser, M., Baumann, E., Palme, K., Hahlbrock, K., et al. (1998). Knock-out mutants from an En-1 mutagenized Arabidopsis thaliana population generate new phenylpropanoid biosynthesis phenotypes. PNAS 95, 12432–12437. doi: 10.1073/pnas.95.21.12432
Xu, F., Li, L., Zhang, W., Cheng, H., Sun, N., Cheng, S., et al. (2012). Isolation, characterization, and function analysis of a flavonol synthase gene from Ginkgo biloba. Mol. Biol. Rep. 39, 2285–2296. doi: 10.1007/s11033-011-0978-9
Xu, W., Lepiniec, L., and Dubos, C. (2014). New insights toward the transcriptional engineering of proanthocyanidin biosynthesis. Plant Signal. Behav. 9:28736. doi: 10.4161/psb.28736
Yin, N. W., Wang, S. X., Jia, L. D., Zhu, M. C., Yang, J., Zhou, B. J., et al. (2019). Identification and Characterization of Major Constituents in Different-Colored Rapeseed Petals by UPLC-HESI-MS/MS. J. Agricult. Food Chem. 67, 11053–11065. doi: 10.1021/acs.jafc.9b05046
Yonekura-Sakakibara, K., Fukushima, A., Nakabayashi, R., Hanada, K., Matsuda, F., Sugawara, S., et al. (2012). Two glycosyltransferases involved in anthocyanin modification delineated by transcriptome independent component analysis in Arabidopsis thaliana. Plant J. 69, 154–167. doi: 10.1111/j.1365-313X.2011.04779.x
Keywords: flavonoid biosynthesis, specialized metabolism, rapeseed, 2-oxoglutarate-dependent dioxygenases, flavanone 3-hydroxylase, bifunctionality, gene family
Citation: Schilbert HM, Schöne M, Baier T, Busche M, Viehöver P, Weisshaar B and Holtgräwe D (2021) Characterization of the Brassica napus Flavonol Synthase Gene Family Reveals Bifunctional Flavonol Synthases. Front. Plant Sci. 12:733762. doi: 10.3389/fpls.2021.733762
Received: 30 June 2021; Accepted: 21 September 2021;
Published: 13 October 2021.
Edited by:
Ryo Fujimoto, Kobe University, JapanReviewed by:
Junxing Lu, Chongqing Normal University, ChinaBenbo Xu, Yangtze University, China
Fuyou Fu, Agriculture and Agri-Food Canada (AAFC), Canada
Copyright © 2021 Schilbert, Schöne, Baier, Busche, Viehöver, Weisshaar and Holtgräwe. This is an open-access article distributed under the terms of the Creative Commons Attribution License (CC BY). The use, distribution or reproduction in other forums is permitted, provided the original author(s) and the copyright owner(s) are credited and that the original publication in this journal is cited, in accordance with accepted academic practice. No use, distribution or reproduction is permitted which does not comply with these terms.
*Correspondence: Daniela Holtgräwe, dholtgra@cebitec.uni-bielefeld.de