- 1Laboratory of Plant Physiology, InBioS-PhytoSYSTEMS, Department of Life Sciences, University of Liège, Liège, Belgium
- 2Plant Sciences Department, University of California, Davis, Davis, CA, United States
- 3Laboratory of Genetics, University of Wisconsin, Madison, WI, United States
- 4Department of Biochemistry, University of Wisconsin, Madison, WI, United States
- 5United States Department of Energy Great Lakes Bioenergy Research Center, University of Wisconsin, Madison, WI, United States
- 6Howard Hughes Medical Institute, Chevy Chase, MD, United States
The proper timing of flowering, which is key to maximize reproductive success and yield, relies in many plant species on the coordination between environmental cues and endogenous developmental programs. The perception of changes in day length is one of the most reliable cues of seasonal change, and this involves the interplay between the sensing of light signals and the circadian clock. Here, we describe a Brachypodium distachyon mutant allele of the evening complex protein EARLY FLOWERING 3 (ELF3). We show that the elf3 mutant flowers more rapidly than wild type plants in short days as well as under longer photoperiods but, in very long (20 h) days, flowering is equally rapid in elf3 and wild type. Furthermore, flowering in the elf3 mutant is still sensitive to vernalization, but not to ambient temperature changes. Molecular analyses revealed that the expression of a short-day marker gene is suppressed in elf3 grown in short days, and the expression patterns of clock genes and flowering time regulators are altered. We also explored the mechanisms of photoperiodic perception in temperate grasses by exposing B. distachyon plants grown under a 12 h photoperiod to a daily night break consisting of a mixture of red and far-red light. We showed that 2 h breaks are sufficient to accelerate flowering in B. distachyon under non-inductive photoperiods and that this acceleration of flowering is mediated by red light. Finally, we discuss advances and perspectives for research on the perception of photoperiod in temperate grasses.
Introduction
In many flowering plant species, photoperiod sensing is key to the synchronization of reproduction with seasonal changes in order to maximize reproductive success. Sensitivity to photoperiod has long been a major agricultural trait selected by breeders to improve yields or adapt crop varieties to different latitudes (e.g., Turner et al., 2005; Lundqvist, 2009; Wilhelm et al., 2009; Casao et al., 2011; Faure et al., 2012). In Pooideae, a monophyletic group of temperate grasses that includes the model grass Brachypodium distachyon (B. distachyon) as well as important cereal crops such as wheat, oat, and barley, the lengthening of photoperiod in the spring is a signal that stimulates flowering, so that seeds are produced and ripen under favorable conditions (e.g., Ream et al., 2012). Although the transduction mechanisms and pathways through which the perception of day length regulates developmental processes remain relatively poorly understood in temperate grasses, this has been the focus of extensive research in other groups of plants, especially in the model Brassicaceae Arabidopsis thaliana (Arabidopsis; Song et al., 2015).
Light signals, which are perceived by photoreceptors, are integrated into circadian clock-regulated processes to be translated into developmental responses (Paik and Huq, 2019). The perception of photoperiod and light quality are achieved through complementary photoreceptors: phytochromes are responsible for the perception of red/far-red wavelengths while cryptochromes, phototropins, and ZEITLUPEs (ZTLs) mediate responses to blue light (Quail, 2002; Möglich et al., 2010). The phytochromes are photolabile photoreceptors existing in two reversible states: the active Pfr form, which is formed under red light, and the inactive Pr form, which accumulates under far-red light or through the temperature-mediated reversion of the Pfr form (Cheng et al., 2021). In Arabidopsis, five phytochromes (PhyA-E) contribute to the modulation of important developmental processes, such as photomorphogenesis, gravitropism, circadian clock entrainment, and flowering time regulation (Legris et al., 2019). Phytochromes form dimers which, upon activation by red light, can be translocated toward the nucleus where they modulate gene expression through their interaction with protein partners such as PHYTOCHROME INTERACTING PROTEINS (PIFs) (Leivar and Quail, 2011; Cheng et al., 2021). PIFs are basic helix-loop-helix transcription factors that typically act as regulators of light responses by direct binding to the promoter of target genes. PIFs are degraded upon interaction with light-activated phytochromes, leading both to broad transcriptional reprogramming (Lucas and Prat, 2014) and to modifications of the chromatin landscape (Willige et al., 2021). Although five phytochromes were identified in Arabidopsis, only PHYA, PHYB, and PHYC are conserved in temperate grasses (Mathews, 2010), among which PHYB and PHYC were shown to be key to control flowering time under long days (LD; Chen et al., 2014; Woods et al., 2014; Pearce et al., 2016; Kippes et al., 2020).
Photoreceptor signaling provides input into the biological clock that controls circadian rhythms, which enables organisms to anticipate daily changes in the environment and thus avoid possible stresses (Johansson and Staiger, 2014). There is an interdependent regulatory loop between photoreceptors and the clock, photoreceptors reporting the changes in the length of days that enable the clock to adapt to seasonal changes (Oakenfull and Davis, 2017). Indeed, in Arabidopsis, while the clock is reset every morning by light, the induction of PHYA expression at night leads to the accumulation of phyA protein in the morning, so that the pool of activated phytochrome at dawn is sufficient to trigger morning genes (Seaton et al., 2018). The daily oscillation of the clock is controlled through a complex array of interactions, which is often summarized as three interlocked feedback loops (Creux and Harmer, 2019). The morning-expressed CIRCADIAN CLOCK ASSOCIATED1 (CCA1) and LATE ELONGATED HYPOCOTYL (LHY) genes encode inhibitors of the PSEUDO-RESPONSE REGULATORs (PRRs) PRR7 and PRR9, which themselves repress CCA1 and LHY, thus forming the morning loop. The central oscillator is formed by mutual repression between CCA1/LHY and TIMING OF CAB EXPRESSION1 (TOC1). TOC1 also inhibits the expression of GIGANTEA (GI) and the components of the evening complex (EC), LUX ARRHYTHMO (LUX), EARLY FLOWERING3 (ELF3), and ELF4, whose expression peaks at dusk (Huang and Nusinow, 2016). The loss of any of these components impairs the function of the EC (Hicks et al., 1996; Covington, 2001; Doyle et al., 2002; Hazen et al., 2005) and thus causes circadian clock malfunction by preventing the EC-mediated repression of PRR7/9, GI, TOC1, and LUX (Huang and Nusinow, 2016). These intricate interactions produce daily rhythms that are synchronized with changes in the photoperiod and/or temperature to control the expression of thousands of genes (Covington et al., 2008).
The role of circadian rhythms in the photoperiodic induction of flowering has been most extensively studied in Arabidopsis. For example, the circadian clock entrains the expression of CONSTANS (CO), whose transcripts accumulate at dusk but whose protein is only stable in the light (Suárez-López et al., 2001; Valverde et al., 2004). Night-break experiments demonstrated that providing a short period of light at a specific time of the night was sufficient to induce flowering (Goto et al., 1991) and suggested that a process known as external coincidence (Bunning, 1937) is operating in Arabidopsis. It was later demonstrated that the coincidence between light and sufficient CO protein levels, which typically occurs in nature under the extended photoperiod of the spring, leads to the stabilization of CO that activates the expression of FLOWERING LOCUS T (FT) in leaves (An et al., 2004). FT encodes a protein annotated as a phosphatidylethanolamine-binding protein and now referred to as florigen, which is transported from leaves to the shoot apical meristem to induce the floral transition (Corbesier et al., 2007; Tamaki et al., 2007).
In long-day flowering temperate grasses, the photoperiod-mediated floral transition starts with the perception of light signals by phyB and phyC, which can form heterodimers (Nishida et al., 2013; Chen et al., 2014; Woods et al., 2014; Kippes et al., 2020). Active alleles of these two phytochromes are required for the induction of the pseudo-response regulator PHOTOPERIOD1 (PPD1) under LD (Chen et al., 2014; Pearce et al., 2016). Once induced by LD, PPD1 stimulates the expression of the florigen FT1, but whether or not this induction is direct is not known. FT1 then interacts with the transcription factor FD and, together, they trigger the expression of the MADS-box protein encoding gene VERNALIZATION1 (VRN1) in the leaves (Li and Dubcovsky, 2008). VRN1 in turn upregulates the expression of FT1 in a positive feedback loop that eventually overcomes the repressive effect mediated by the zinc-finger-CCT domain transcription factor VRN2 on FT1 expression (Distelfeld and Dubcovsky, 2010; Ream et al., 2014). The FT1 protein is then thought to migrate to the shoot apical meristem, as shown in Arabidopsis, to induce the expression of VRN1, thus promoting flowering under favorable photoperiods (Woods and Amasino, 2015).
The absolute requirement for inductive photoperiods for flowering in B. distachyon suggests that this process is tightly controlled by circadian clock mechanisms (Woods and Amasino, 2015). The EC component ELF3 is a key regulator at the intersection of photoperiod-induced flowering and the circadian clock, and, not surprisingly, this gene has been an important breeding target for crop improvement (Bendix et al., 2015). Indeed, in LD flowering plants, natural variation in ELF3 allowed growing seed crops in new environments, whether at latitudes where shorter photoperiods would have otherwise prevented flowering, or under stressful conditions in which early flowering represents an advantage (Bendix et al., 2015). For instance, the wild relatives of cultivated peas from temperate regions are obligate LD plants whose domestication as spring crops was associated with natural variation at two photoperiod-sensitivity loci, HIGH RESPONSE and PHOTOPERIOD, which correspond to two distinct orthologs of ELF3 (Weller et al., 2012; Rubenach et al., 2017). Natural variation in ELF3 also allowed adaptation of short-day flowering crops to new cultivation conditions. For instance, in soybean, which is mostly cultivated in temperate climates, natural variation at ELF3 delayed flowering under the shorter photoperiod of tropical regions, thus enabling an extended flowering phase and increased yields (Lu et al., 2017; Bu et al., 2021). In Arabidopsis, independently of its role as a component of the EC, ELF3 is also able to interact with PIF4 in order to prevent the activation of its transcriptional targets (Nieto et al., 2015). In addition to its role in mediating the photoperiodic response, ELF3 acts as a thermosensor mediating the interplay between the circadian clock, flowering, and ambient temperature (Bullrich et al., 2002; Strasser et al., 2009; Thines and Harmon, 2010; Jung et al., 2020). The Arabidopsis ELF3 protein contains a prion-like domain that, at higher temperatures, undergoes conformational changes that reversibly inactivate ELF3. However, the extent to which this mechanism is conserved across land plants remains to be established as, for instance, the prion-like domain conferring the ambient temperature sensitivity is absent from the B. distachyon ELF3 protein (Jung et al., 2020).
Comparative genomics led to the identification of orthologs of circadian clock genes, including ELF3, among Arabidopsis, rice, and B. distachyon (Higgins et al., 2010), so it is not surprising that ELF3 is also a key photoperiod response regulator in monocots. In rice, studies on the natural variation of flowering time between Japanese cultivars identified a single-nucleotide polymorphism at the ELF3 locus as a likely candidate (Matsubara et al., 2012). Indeed, a polymorphism that delayed flowering under short-day inductive photoperiods was caused by a change in OsELF3 that impedes its ability to control phytochrome-mediated signaling pathways (Saito et al., 2012; Zhao et al., 2012; Itoh et al., 2018). Likewise, certain rapidly flowering barley mutants that were adapted to shorter growing seasons (Gustafsson et al., 1960) were shown to be mutated at the ELF3 locus (Faure et al., 2012; Zakhrabekova et al., 2012; Boden et al., 2014; Wang et al., 2016). The early flowering phenotype of the barley elf3 mutant is suppressed by the inhibition of gibberellin biosynthesis, suggesting that the contribution of this hormone is key to the early flowering phenotype caused by the disruption of the clock rhythmicity (Boden et al., 2014). In wheat, the thermosensitive earliness per se locus Eps-Am1 (Bullrich et al., 2002) was shown to be linked to mutations in ELF3 (Alvarez et al., 2016). The conserved role of ELF3 across the monocot/eudicot divide is indicated by the ability of the B. distachyon ELF3 gene to rescue the hypocotyl elongation, clock arrhythmicity, and flowering phenotypes of the Arabidopsis elf3 mutant (Huang et al., 2017). Here, we describe a new mutant allele of elf3 that was identified in a mutagenized population grown under short days (SD), highlighting that the role of ELF3 in circadian clock function and mediating the photoperiodic induction of flowering genes is conserved.
Materials and Methods
Plant Material, Growth Conditions, and Phenotyping
Experiments were conducted using the Bd21-3 accession of B. distachyon. An EMS-mutagenized M2 population used for screening was generated as described in Woods et al. (2014). All experiments were carried out using the elf3 mutant that had been back-crossed twice. For phenotyping and RT-qPCR experiments, plants were grown in 0.5 l pots containing a 4:1 mixture of soil (Brill, Germany) and perlite supplemented with 8 g l–1 of Osmocote Exact Standard 5–6 M (ICL Specialty Fertilizers, Israel). Seeds were stratified for 2 days in the dark at 4°C before sowing, and plants were grown under 8, 12, 16, or 20 h photoperiods provided by fluorescent tubes (Philips Master TL-D Super 80 58W 4100K) at an intensity of 150 μmol.m–2.s–1 (PAR), 70% humidity, 20°C day/night. For the mean internode lengths, we dissected 10–16 plants per genotype at a developmental stage 1–2 leaves before the estimated stage when the elf3 mutant would transition to flowering based on preliminary experiments (i.e., dissection was performed 43 days after germination under 8 h, 30 days under 12 h, 21 days under 16 h, and 20 days under 20 h), and measured the distance between each node on the main stem. Estimates of chlorophyll contents were performed on the third emerged leaf at the fourth leaf stage using a MC-100 probe (Apogee Instruments, United States).
Temperature and Night Break Experiments
For vernalization treatments, seeds were stratified for 2 days at 4°C, then placed in soil and cold treated for 3 weeks at 4°C in the dark before transfer to standard growth conditions (150 μmol.m–2.s–1 light, 70% humidity, 20°C day/night, 8 or 16 h photoperiod). For ambient temperature experiments, Bd21-3 seeds were stratified for 2 days at 4°C and planted in a 16 h photoperiod, 20°C day/night conditions. After 2 weeks, seedlings were transferred to growth chambers at 15, 20, or 25°C day/night. For night-break (NB) experiments performed using fluorescent white light (Philips Master TL-D Super 80 58W 4100K), plants were germinated for 1 week under 10 h SD before being transferred to either 10 h SD, 10 h SD supplemented with a 2 h NB, or 8 h SD supplemented with a 2 h NB. All NBs started at Zeitgeber time (ZT) 16 h, since this time was reported to be the most efficient in other temperate grasses (Pearce et al., 2017). For NB experiments using a red:far-red mixture, plants were grown in 3 l pots under a 12 h photoperiod for 8 weeks. They were subsequently transferred to a 12 h photoperiod supplemented with a 2 h NB given in phytotronic cabinets equipped with Lumiatec PHS::16 (300 W) modular LED luminaries (GDTech, Belgium). NBs were provided at ZT16h using a red to far-red gradient and low light intensities (20 –25 μmol.m–2.s–1). The spectral distributions of lights (white, red, far-red) provided by LED luminaries are in Supplementary Figure 1A. Controls were either kept under 12 h SD without NB or exposed to a 12 h photoperiod supplemented with a 2 h NB at ZT16h provided by fluorescent tubes (150 μmol.m–2.s–1). For end-of-day far-red (FR) treatments, plants were grown for 1 week under white light with a 12-h photoperiod before transfer to either 18 h LD or 18 h LD followed by 1-h of FR (Supplementary Figure 1B). All experiments were stopped 100 days after transfer.
Mapping the elf3 Mutation and RT-qPCR Analyses
To map the mutation, an M3 homozygous mutant line was crossed with the Bd3-1 accession. The mapping was performed using PCR-amplified InDel markers (Woods et al., 2014) on 40 plants segregating for early flowering under 8 h SD. The candidate genes in this interval were identified using Phytozome (Goodstein et al., 2012), and the coding region of the most likely candidate, ELF3, was analyzed by Sanger sequencing. For RT-qPCR, the third leaf at the three-leaf stage of WT and elf3 plants were harvested every 2 h and pooled separately (n = 6–8). RNA was extracted using the NucleoSpin RNA plant kit (Macherey-Nigel, Germany) and reverse transcription was carried out on 1.5 μg of RNA using MMLV RT (Promega, United States), following the manufacturer’s instructions. The RT-qPCR was performed with Takyon Low Rox MasterMix (Eurogentec, Belgium) using 40 cycles of amplification: 10″ at 95°C for denaturation, 20″ at 57°C for hybridization, and 30″ at 72°C for elongation. The geometric mean of ACT3 and UBC18 was used to normalize data (Vandesompele et al., 2002). Primers are listed in Supplementary Table 1.
Generation of UBI: EARLY FLOWERING 3 Transgenic elf3 Plants
The ELF3 coding region cloned into the pENTR/D-TOPO vector, originally published in Huang et al. (2017), was obtained from Dmitri Nusinow. Cloning of ELF3 into pANIC10a was done as described in Ream et al. (2014). Clones were verified by sequencing and then transformed into chemically competent Agrobacterium tumefaciens strain Agl-1. Plant callus transformation of elf3 with the pANIC10a vector containing the wild type ELF3 gene was performed as described in Vogel and Hill (2008) by the Great Lakes Bioenergy Research Center for Brachypodium transformation facility. Independent transgenic lines were genotyped for the transgene using a gene-specific ELF3 forward primer and a pANIC10a specific reverse primer (Supplementary Table 1).
Results
Identification of the elf3 Mutation
B. distachyon is an obligate LD species, requiring photoperiods of 14 h or more to flower rapidly (Ream et al., 2014; Woods et al., 2017). In order to further understand the mechanisms controlling this requirement, we screened M2 EMS-mutagenized lines for flowering phenotypes under 8 h SD and identified one rapidly flowering mutant (Figure 1A). To test whether the rapid flowering phenotype was specific to SD, we grew the homozygous mutant lines under a 16 h photoperiod and found that the mutant was also rapid flowering in LD compared to WT plants (Figure 1B). We crossed M3 homozygous mutant lines with the Bd3-1 accession in order to obtain a mapping population. The 1:3 segregation of the rapid flowering phenotype in the F2 population indicated a recessive causative mutation. We used InDel markers (Woods et al., 2014) to locate the mutation within a 2 Mb region on chromosome 2 (Figure 1C). Analysis of candidate genes in the Bd21-3 genome revealed that this genomic region contained a homolog of EARLY FLOWERING 3 (Bradi2g14290). Because mutations in this gene were known to cause rapid flowering in other species (Hicks et al., 1996, 2001; Zagotta et al., 1996; Faure et al., 2012; Yang et al., 2013; Boden et al., 2014; Alvarez et al., 2016; Rubenach et al., 2017), we sequenced the coding region of ELF3 and found a single base pair mutation in the fourth exon of the gene that resulted in a premature STOP codon (Figure 1D) and is predicted to result in a truncated protein that lacks the fourth conserved domain of ELF3 (Figure 1E). The genotyping of a segregating population originating from a cross of the elf3 mutant using dCAPS primers showed that the segregation of the phenotype was 100% linked with the presence of the elf3 mutation (Supplementary Figure 2). To further confirm that the elf3 mutation is causative, we were able to rescue the rapid flowering mutant phenotype by overexpressing the ELF3 cDNA using the maize ubiquitin promoter (UBI:ELF3) in the elf3 mutant background (Supplementary Figure 3). The mutant was back-crossed twice with Bd21-3 before further characterization.
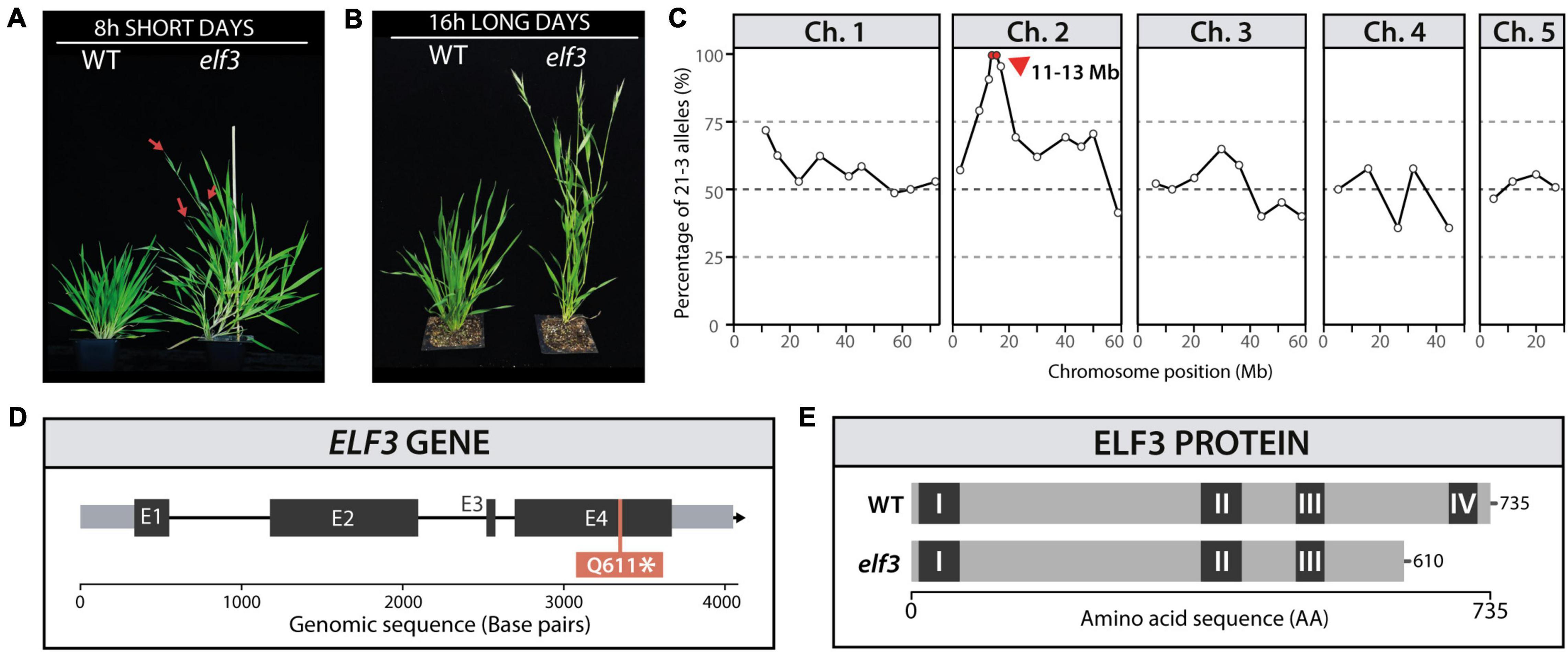
Figure 1. Identification of the elf3 mutant. Phenotype of the elf3 mutant and wild-type (WT) Brachypodium distachyon plants under (A) 8 h (80 days) and (B) 16 h photoperiods (50 days). The red arrows indicate early emerging spikes. (C) InDel mapping of the segregation of the early flowering phenotype in an M2 mapping population (Bd21-3 and Bd3-1). (D,E) Schematic representation of the ELF3 gene (D; E1 to E4 indicate exons) and the ELF3 protein (E; regions I to IV indicate conserved protein domains).
The elf3 Phenotypes Mimic Long-Day Grown Plants
We further characterized the elf3 mutant by growing it under 8, 12, 16, and 20 h photoperiods. The mutant flowered earlier than WT plants under all photoperiods except 20 h, under which both genotypes flowered very rapidly (Figure 2A). We also observed increased internode lengths (Figure 2B) and a lower estimated chlorophyll content in the elf3 mutant (Figure 2C). The difference in mean internode lengths was dependent on the photoperiod as the length of internodes increased with longer photoperiods in WT plants and decreased in elf3 plants, such that no difference was measured under 20 h photoperiod. The estimated chlorophyll content, on the contrary, was significatively lower in the mutant under all photoperiods, which is in accordance with the color of the leaves that were visibly paler in the mutant. Because increased RNA level of FT-Like9 (FTL9) was shown to be a marker of SD in B. distachyon (Qin et al., 2019; Woods et al., 2019), we tested whether its expression was altered in the mutant under a 12 h photoperiod. We observed that FTL9 transcripts were undetectable at all-time points in the elf3 mutant under conditions in which the gene was highly expressed in Bd21-3 (Figure 2D).
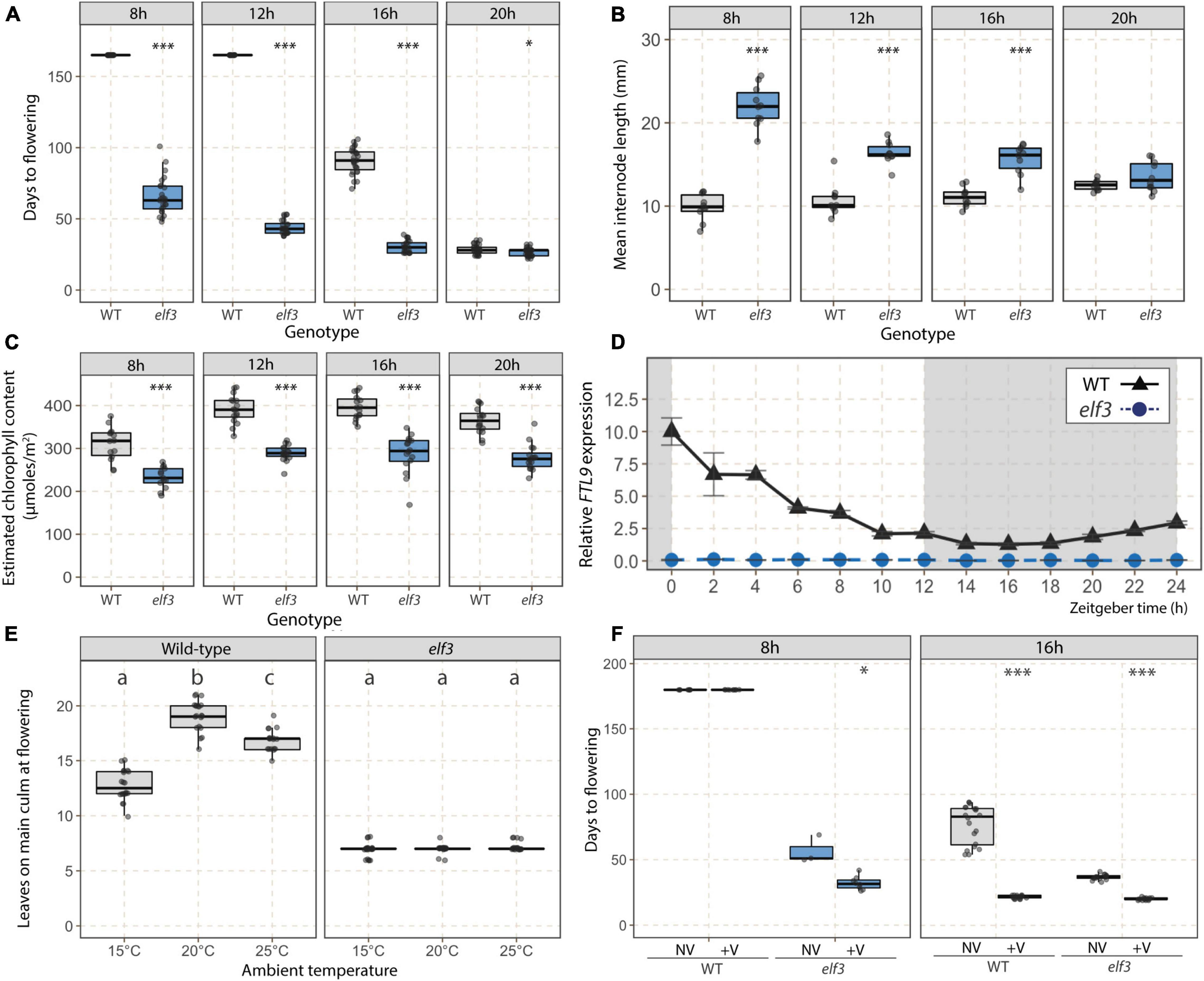
Figure 2. Phenotypic characterization of the elf3 mutant. Flowering time (A; days to flowering), mean internode lengths (B), and estimated chlorophyll contents (C; μmoles.m–2) for the elf3 mutant wild-type (WT) plants under 8, 12, 16, and 20 h photoperiods (n = 10–25). For (B,C), data were collected 1 week prior to estimated floral transition according to a preliminary experiment. (D) Relative FTL9 expression kinetics in WT (black triangles) and elf3 (blue circles) plants under a 12 h photoperiod. Data were normalized using the geometric mean of ACT3 and UBC18 reference genes. Error bars represent the standard error of the mean. Time is expressed as the number of hours following the start of the light period (Zeitgeber time, ZT). (E) Flowering time (number of leaves on the main culm at flowering) of elf3 mutant and WT plants grown at distinct temperatures. Letters (a,b,c) indicate statistical differences (p < 0.05) according to a Tukey’s HSD test used to perform multiple comparisons. (F) Flowering time (days to flowering) of elf3 mutant and WT plants exposed (V) or not (NV) to a 3-week vernalizing treatment and subsequently grown under 8 or 16 h photoperiods. Student t-tests were used for pairwise comparisons in (A–C,F) (***p < 0.001; *p < 0.05).
We next tested the effect of temperature on the flowering time of the elf3 mutant. Both higher (25°C) and lower (15°C) ambient temperatures accelerated flowering of WT plants grown under a 16 h photoperiod compared to the 20°C standard in terms of leaf number on the primary culm (Figure 2E). In contrast, the number of leaves at flowering was not altered by temperature changes in the elf3 mutant (Figure 2E). We then tested the effect of vernalization on the elf3 mutant by exposing seeds to 3 weeks of cold (4°C; a saturating vernalization treatment for Bd21-3, as shown in Ream et al., 2014) before growing them in either 8 or 16 h photoperiods (Figure 2F). The early flowering of elf3 was further accelerated by vernalization under both photoperiods, suggesting that the mutation of ELF3 does not affect the vernalization response. Because vernalization provides the competence to flower through the up-regulation of VRN1 in B. distachyon (Ream et al., 2014), we tested whether elevated VRN1 mRNA levels affected the flowering time of the elf3 mutant without vernalization. We found that homozygous lines overexpressing VRN1 (UBI:VRN1 lines originally developed and characterized in Ream et al., 2014) in the elf3 mutant background displayed an even more rapid flowering phenotype than the elf3 mutant (Supplementary Figure 4).
Role of EARLY FLOWERING 3 in Controlling Circadian Clock and Flowering Time Genes
The ELF3 protein is a component of the EC of the circadian clock, which controls the expression of other clock genes, as well as flowering genes (Huang and Nusinow, 2016). We thus analyzed the expression patterns of a set of those targets in the leaves of the elf3 mutant under different photoperiods (Figure 3). In WT plants, the peak of CCA1 expression occurs in the morning in all photoperiods, except under 20 h LD, in which it occurs at midday (Figure 3A). In the elf3 mutant, these peaks were strongly damped under all photoperiods, indicating that ELF3 is required for proper induction of CCA1 expression. Alterations were also visible in the expression kinetics of GI, another clock gene that participates in Arabidopsis flowering induction (Figure 3B). The expression peak of GI was advanced by about 4 h in the elf3 mutant under all photoperiods. Interestingly, whereas GI expression was undetectable during the nights under photoperiods shorter than 20 h in WT plants, it could be detected at most time points in the elf3 mutant independently of the photoperiod. The expression of PPD1, a clock-regulated flowering time regulator in temperate grasses (Turner et al., 2005), was also altered: it was much stronger at all-time points in the elf3 mutant except at the end of the day when the expression level was similar to that in WT (Figure 3C). On the contrary, the expression of CO1, another output of the circadian clock (Shaw et al., 2020), seemed to be downregulated at night in the mutant under photoperiods shorter than 20 h LD (Supplementary Figure 5). Overall, these results show the strong impact that mutation of ELF3 has on circadian clock-regulated gene expression in B. distachyon.
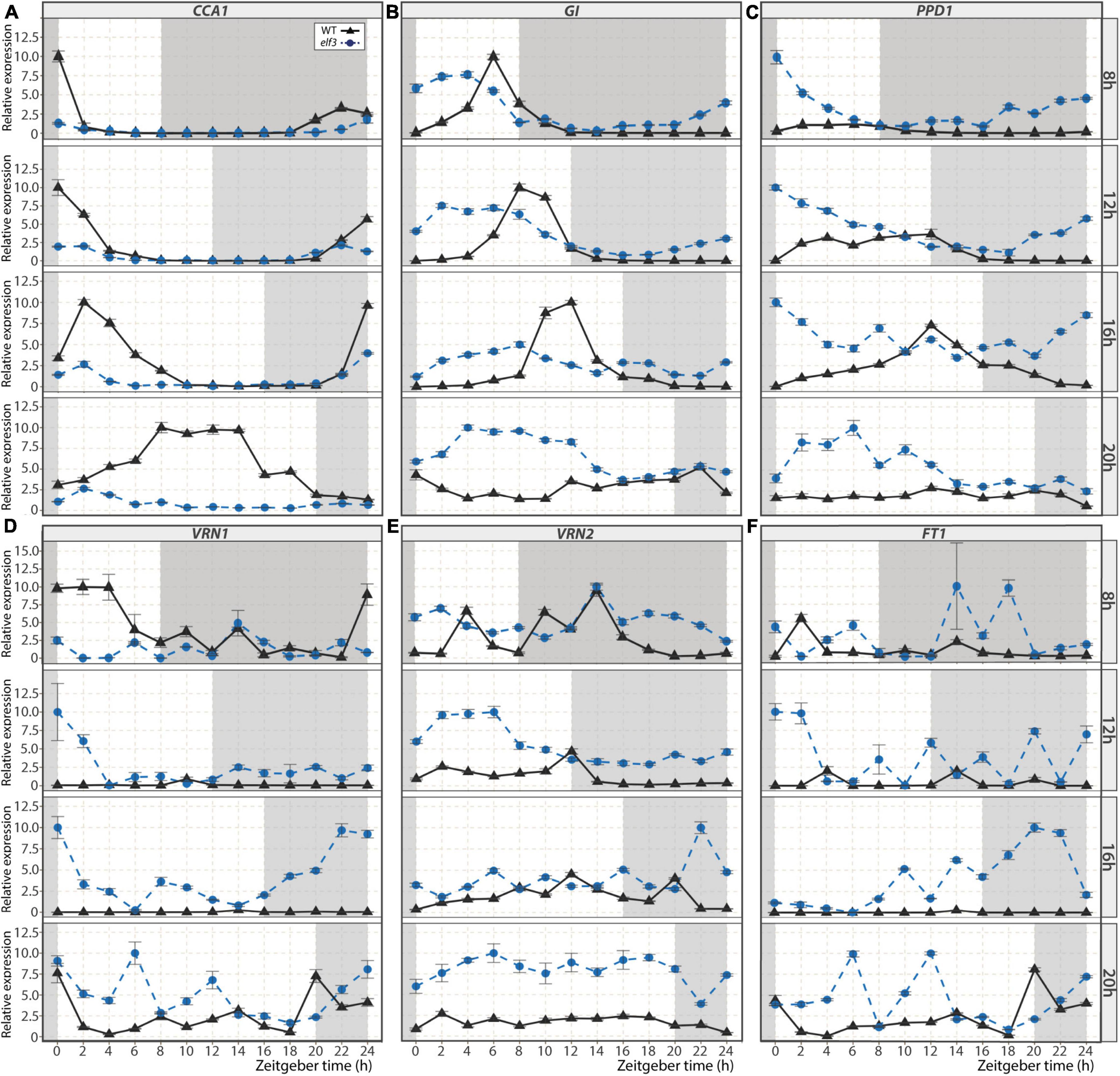
Figure 3. Expression kinetics of selected genes in the elf3 mutant. Relative expression of circadian clock-regulated genes CCA1 (A), GI (B), PPD1 (C), and the flowering genes VRN1 (D), VRN2 (E), and FT1 (F) during a 24 h time course for WT plants (black triangles) and elf3 mutants (blue circle) cultivated under 8, 12, 16, and 20 h photoperiods. Error bars represent the standard error of the mean. Data were normalized using the geometric mean of ACT3 and UBC18. The white background indicates the light period, and the gray background shows the dark period. Time is expressed as the number of hours following the start of the light period (Zeitgeber time, ZT).
We then analyzed the expression patterns of the flowering time genes VRN1 and VRN2. Although the expression of the floral inducer VRN1 seemed slightly down-regulated in the elf3 mutant under 8 h SD during daytime, we observed an increase in its expression in all other photoperiods (Figure 3D). Interestingly, the expression of the floral repressor VRN2 was also stimulated at most time points in the mutant (Figure 3E). Because VRN1 and VRN2 play antagonistic roles in controlling the expression of the florigen FT1 (Woods et al., 2016) and were both up-regulated in elf3, we examined FT1 expression. Consistent with the rapid flowering elf3 phenotype, FT1 expression was higher in the mutant than in WT plants (Figure 3F).
Links Between EARLY FLOWERING 3, Photoreceptors, and Night Breaks
To test whether night breaks (NBs) could accelerate flowering in the elf3 mutant as in WT plants, we exposed plants grown in 8 or 10 h SD to a 2 h NB from ZT16h to ZT18h (Figure 4A). While most of the control plants had not flowered after 150 days, the exposure to NBs accelerated flowering of WT plants both in 8 h SD, in which plants flowered 100–125 days after germination, and even more in 10 h SD, in which they flowered after around 70 days (Figure 4B). In the elf3 mutant, we also observed a very slight acceleration of flowering upon NB exposure—about 4 days when NBs were provided in 8 h SD and 7 days under 10 h SD—indicating that the elf3 mutation attenuates the flowering response to NB.
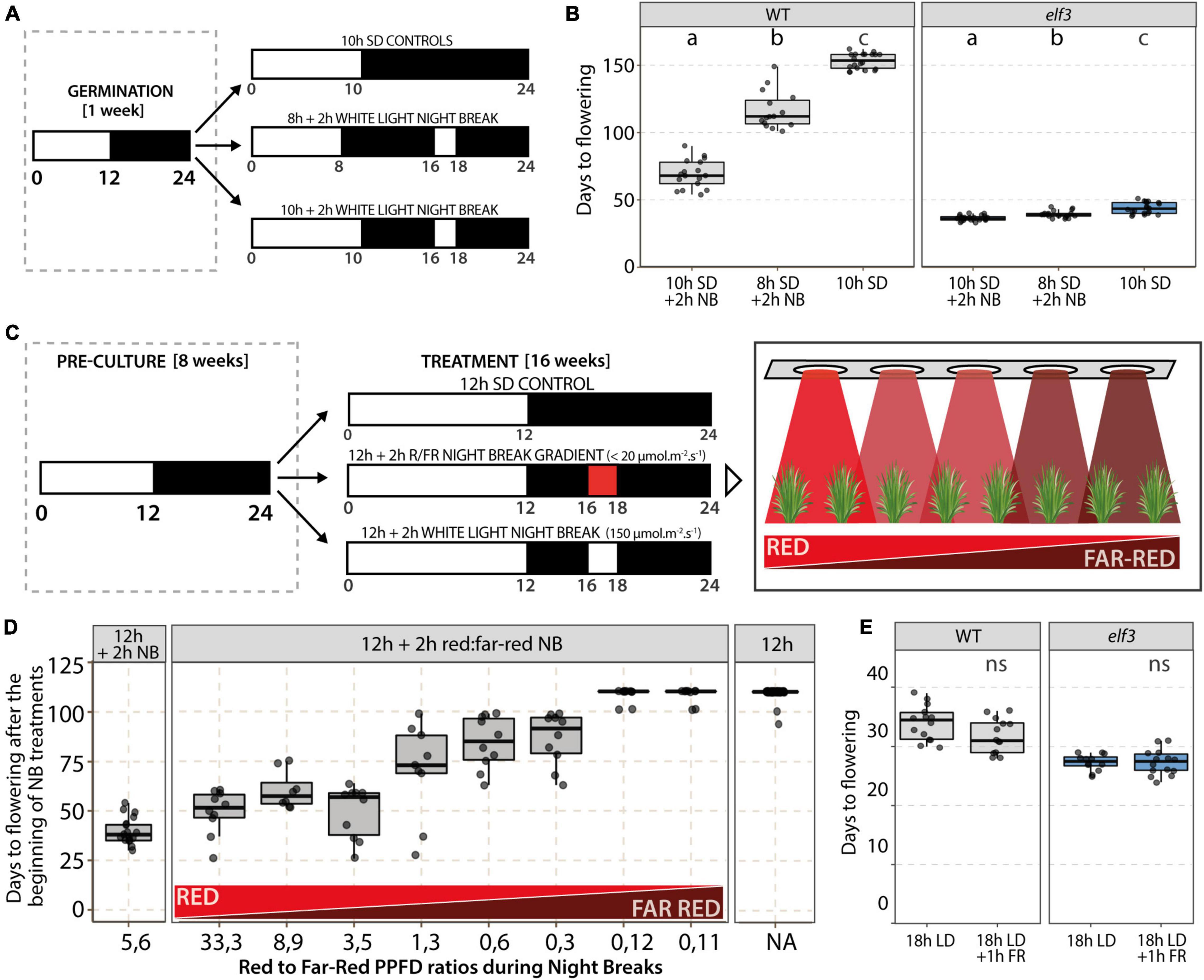
Figure 4. Effect of night breaks and light quality on flowering time. (A) Experimental design and (B) results of night break (NB) experiments performed by exposing wild-type (WT) and elf3 plants growing in either 8 or 10 h photoperiods to a 2 h NB (NB; 150 μmol.m–2.s–1) from Zeitgeber time (ZT) 16 h to ZT18h. Controls were maintained in 10 h SD (n = 15–20). Note that most of the control plants had not flowered after 150 days in 10 h SD. Letters indicate statistical difference (p < 0.05) using Tukey’s HSD tests. (C) Experimental design used to test the effect of the red (R) to far-red (FR) ratio during NBs on flowering. (D) Flowering time of plants exposed to a NB under a red to far-red gradient. WT plants were grown in a 12 h photoperiod for 8 weeks before being transferred to a 12 h photoperiod supplemented with a 2 h NB (from ZT16h to ZT18h) under a red to far-red gradient (n = 8–15 per condition). Control plants were either maintained under a 12 h photoperiod (Right panel) or exposed to a 2 h white light NB (Left panel). (E) Effect of far-red (FR) light on flowering time. At the beginning of the night, WT and elf3 plants grown under an 18 h photoperiod were exposed to 1 h of FR light (25 μmol.m–2.s–1). Control plants were exposed to an uninterrupted night (n = 15–20); t-test revealed no statistically significant differences (ns).
We then decided to test the effect of varying red to far-red ratios on the NB efficiency. Accordingly, wild-type plants grown under 12 h SD for 8 weeks were exposed daily to a 2 h NB from ZT16h to ZT18h, provided as a mixture of red and far-red light (Figure 4C). Different red:far-red ratios were provided using an LED light gradient during the NB, with low light intensities to limit photosynthetic effects. Control plants were either maintained under SD without NB or exposed to 2 h NBs of white light. We observed a strong correlation between flowering induction and higher red:far-red ratios. Indeed, red:far-red ratios over 3 led to the strongest acceleration of flowering, whereas ratios between 0.3 and 1.3 provided only a slight acceleration of flowering, and lower ratios did not induce flowering (Figure 4D). Finally, we performed end-of-day far-red treatments to see whether switching phytochromes to their inactive Pr form before night-time would affect flowering. We therefore provided a 1-h far-red treatment to plants grown under 18 h LD and observed that the far-red treatment at the end of the day did not affect flowering time (Figure 4E).
Discussion and Perspectives
Interplay Between EARLY FLOWERING 3, the Photoperiod, and the Circadian Clock
Although much is known about the many genes whose mutations affect flowering in Arabidopsis (Bouché et al., 2016), substantially fewer flowering control genes have been identified to date in temperate grasses, and many of these genes do not have homologs involved in flowering in Arabidopsis (Higgins et al., 2010; Ream et al., 2012). However, ELF3 has been described as a key hub between photoperiodic signals and the circadian clock in both eudicots and monocots (Huang and Nusinow, 2016) and the results presented in this paper strongly support this interpretation and extend the characterization of the role of ELF3 to B. distachyon. Indeed, a mutation in ELF3 severely reduces the requirement for LD exposure to induce flowering in the Bd21-3 accession, similar to the phenotype described in a preprint from Gao et al. (2019).
That flowering in the elf3 mutant occurs rapidly in all photoperiods including 8 h SD indicates that elf3 mutants perceive all photoperiods as LD. In some plants, including B. distachyon and other temperate grasses, the exposure to the shorter photoperiods of winter can substitute for the exposure to cold temperature as a winter cue providing floral competence, a process known as SD vernalization (Purvis and Gregory, 1937). Recently, FTL9 was shown to be key in establishing the SD-vernalization ability of B. distachyon, and FTL9 transcript levels exhibit a diurnal oscillation with a high peak in SD but are always low in LD (Woods et al., 2019). We observed that the expression of FTL9 was undetectable in the elf3 mutant in SD throughout a diurnal cycle. Interestingly, this expression pattern is opposite to that observed in the late flowering phyC mutant, in which flowering is insensitive to LD (Woods et al., 2014, 2019). Therefore, the disruption of the EC complex caused by the absence of functional ELF3 mimics constitutive exposure to LD like the absence of phyC mimics constitutive exposure to SD. Furthermore, these mutants show opposite transcriptomic profiles for several gene clusters (Gao et al., 2019). The specific pathways through which ELF3 and phyC exert their opposite roles remain to be determined. ELF3 might also act through FT1-independent pathways; for example, in Arabidopsis, the elf3;co double mutant is early flowering but does not display any increase in FT expression (Kim et al., 2005; Song et al., 2018). In B. distachyon, it was shown that the phyC mutant does not display any difference in ELF3 expression (Woods et al., 2014), but a large part of the regulation of ELF3 function occurs at the protein level (Huang and Nusinow, 2016). The physical interaction between ELF3 and PHYC, which has been reported (Gao et al., 2019), could thus be critical in the regulatory process.
Interactions Between the elf3 Mutation and Other Flowering Pathways
The elf3 mutant was found to be insensitive to SD but still responded to vernalization by cold. Indeed, a 3-week exposure to 4°C further accelerated the flowering of the mutant in all tested photoperiods. At the molecular level, we found that the expression of the positive regulator of flowering VRN1 was low under 8 h SD in the elf3 mutant, which seems in contrast with its rapid flowering phenotype. However, the rapid flowering but low VRN1 RNA levels could be caused by the increase in the expression of PPD1 under SD that we observed in the mutant. Indeed, in wheat, the ppd1 mutation causes increased VRN1 mRNA levels specifically under short photoperiods, indicating that PPD1 is a negative regulator of VRN1 under SD (Shaw et al., 2020); thus, the increased PPD1 expression levels in the elf3 mutant in SD could be responsible for the observed repression of VRN1. The VRN1-independent acceleration of flowering in elf3 could also be due to the increase in PPD1 which is itself a flowering promoter (Shaw et al., 2013, 2020). It is noteworthy that the elf3 mutant is responsive to VRN1 because the overexpression of VRN1 in the elf3 background results in a very rapid flowering under SD, highlighting the additive roles played by the vernalization and the photoperiodic pathways. In 16 h LD, we found higher VRN1 expression levels in the elf3 background in the absence of cold. However, the vernalization treatment also accelerated flowering in the elf3 mutant under 16 h LD, suggesting that cold exposure accelerates flowering either by further activation of VRN1 or by the regulation of other targets. Further experiments are required to test these possibilities.
Ambient temperature also plays a role in flowering time control in many species (Capovilla et al., 2015). In B. distachyon, earlier reports showed that increasing the ambient temperature cannot substitute for LD to induce flowering (Boden et al., 2013), and that different accessions have distinct optimal temperatures for floral induction (Li et al., 2019). In our conditions, Bd21-3 flowered much more rapidly at 15°C than at 20 or 25°C. A similar observation was made in winter wheat cultivars, in which bolting occurred earlier at 11°C than at 25°C (Dixon et al., 2019). However, we did not see any effect of ambient temperature on the flowering time of the elf3 mutant. This lack of temperature-response might either be due to the rapid flowering phenotype of the mutant, which would mask the temperature effect, or could indicate that ELF3 plays a role in the temperature-dependent flowering response, as suggested earlier in barley (Ford et al., 2016). In Arabidopsis, the ELF3 protein acts as a thermosensor: at high temperature it is sequestered in liquid droplets and is prevented from exerting its transcriptional repressor role, resulting in accelerated flowering (Jung et al., 2020). However, the prion-like domain required for this behavior is absent in the B. distachyon ELF3 protein (Jung et al., 2020); moreover, the acceleration of flowering in the Bd21-3 accession is observed at lower rather than higher temperatures as in Arabidopsis. Because phyC and ELF3 proteins were shown to interact physically (Gao et al., 2019), one hypothesis would be that changes in ambient temperatures affect their interaction to modulate the repressing effect of ELF3 on the phyC-mediated induction of flowering. It would be interesting to test whether natural variation in ELF3, phyC, and PIFs among B. distachyon accessions affects temperature sensitivity for flowering induction.
Perception of the Photoperiodic Pathway
The pathways through which photoperiodic signals are perceived and implemented into developmental responses in temperate grasses are not fully understood, and NB experiments can shed light on underlying mechanisms. Consistent with a previous study (Gao et al., 2019), we observed that the exposure of Bd21-3 plants to NBs was sufficient to accelerate flowering in SD. In wheat, the induction of flowering can also be triggered by NBs provided at different time points to plants grown in SD, and this acceleration of flowering was shown to require a functional PPD1 allele (Pearce et al., 2017). When applying daily NBs to the elf3 mutant, we observed only a very slight acceleration of flowering, suggesting that NBs act mainly through ELF3-mediated processes, although parallel pathways might also play a minor role, possibly through GI, CO, or yet unknown pathways. Further molecular work is required to establish the pathway that is triggered under such conditions.
Phytochromes can switch between the inactive Pr form, which accumulates under darkness or far-red light, and the active Pfr form, which is stimulated by red light (Quail, 2002). In Arabidopsis, lower red:far-red ratios, which indicate the presence of proximate plants that compete for light exposure, results in the acceleration of flowering (Casal, 2013). On the contrary, in wheat, lower red:far-red ratios were shown to reduce yields through delayed spike development and reduced floret numbers (Ugarte et al., 2010). Here we provided NBs using a varying mixture of low intensity red and far-red lights to B. distachyon plants grown under 12 h non-inductive conditions, and we observed a positive correlation between the induction of flowering and higher red:far-red ratios. These results suggest that phytochromes in their Pfr form stimulate floral induction although they do not preclude the participation of other molecular pathways in the induction of flowering.
The promotion of flowering by NBs supports the external coincidence model of the photoperiodic control of flowering in which the inductive pathways are activated when light is perceived at the appropriate circadian time. Flowering of LD plants can indeed be induced without increasing the length of the photoperiod but by displacing SD at the appropriate circadian time. These “displaced SD” can be reduced in length and still induce flowering, as shown for example in Lolium temulentum (Périlleux et al., 1994). An alternative explanation was proposed in which the photoperiod-mediated induction of flowering in temperate grasses relies on the hourglass model (Borthwick and Hendricks, 1960). In this model, the effect of LD is due to the shorter nights that do not allow a full reversion of the pool of active Pfr into the inactive Pr form, so that flowering is eventually triggered by the accumulation of the active Pfr form. However, a previous report in wheat indicated that far-red light, which induces full reversion of Pfr into Pr, diminishes the effect of 1 h NBs when given during the NB but not after, indicating that 1 h NBs are sufficient to irreversibly activate flowering (Pearce et al., 2017). Here, in B. distachyon, we showed that when exposing the WT and the elf3 mutant to a 1 h far-red treatment at the end of each 18 h photoperiod, flowering was not delayed. Collectively, these results suggest that neither the day-to-day accumulation of active Pfr nor its role during night-time are key to floral induction, and rather indicate that Pfr plays its inductive role before the end of the light period in LD. Complementary experiments using transgenics constitutively expressing active phytochromes or experimental designs in which far-red light is provided during the daytime to reduce the accumulation of the active Pfr would help to further elucidate the underlying mechanisms. Recently, the introduction of a constitutively active, light-insensitive, allele of the rice phyB into B. distachyon led to a mild acceleration (4 days) of flowering in 16 h LD grown plants (Hu et al., 2020). The lack of a strong rapid flowering phenotype in these transgenics might be the indirect consequence of phyB mode of action, which could require the formation of an heterodimer with limiting levels of active phyC, or due to the heterologous rice phytochrome being used instead of the B. distachyon phytochrome. However, testing whether flowering is also accelerated under non-inductive photoperiods and whether these transgenics remain sensitive to NB would provide valuable insights. In any case, the current knowledge acquired through both physiological and transgenic experiments indicates that the external coincidence model does play a role in the photoperiodic induction of flowering in temperate grasses.
Perspectives on the Photoperiodic Research in Brachypodium distachyon
The current model of the photoperiodic induction of flowering in B. distachyon involves the phyC-mediated activation of PPD1 expression, possibly in part through ELF3 (Figure 5). In turn, PPD1 induces the expression of FT1 in leaves, which forms a positive regulatory loop with VRN1 before FT1 protein moves toward the shoot apical meristem to induce flowering (Woods and Amasino, 2015). The elucidation of the exact pathway—or pathways—triggering flowering, however, will require more genetic work, including the creation of multiple mutants and transgenic lines, and the new mutant allele of ELF3 described here provides an additional tool toward this goal. For example, phyC;elf3 or phyB;elf3 double mutants would be informative to evaluate if indeed most of the light signal from the phytochromes is mediated through ELF3. Furthermore, ELF3 plays a repressive role on PPD1 but is PPD1 the main target impacting photoperiodic flowering or is ELF3 involved in repressing other important components of the photoperiod pathway? The elf3;ppd1 double mutants would be well suited to address this important question. Also, coupling these lines with mutants and overexpressors for GI, CO1, and CO2 will help to test the epistatic interactions between these genes as well as their involvement in the photoperiodic pathway of floral induction. Indeed, exploring the impact these genes have on flowering has already led to some insights. For example, knock-down of CO1 in B. distachyon via RNAi results in lower VRN2 mRNA levels yet plants are delayed in flowering, whereas overexpression of CO1 results in higher VRN2 mRNA levels but interestingly more rapid flowering (Qin et al., 2019). This is consistent with studies from barley where the overexpression of HvCO1/CO2 results in more rapid flowering even though HvVRN2 is elevated (Mulki and von Korff, 2015). However, in barley, when comparing UBI:HvCO2 lines in a segregating population with and without HvVRN2, plants with a functional HvVRN2 allele are more delayed in flowering than those where HvVRN2 is deleted, despite the presence of UBI:HvCO2 in both segregating plants (Mulki and von Korff, 2015). The studies above highlight the importance of comparative genetic studies which, together with the development of new tools for B. distachyon (e.g., tissue-specific promoters, interactome maps, etc.), will help us to decipher the spatio-temporal regulation of flowering time in temperate grasses.
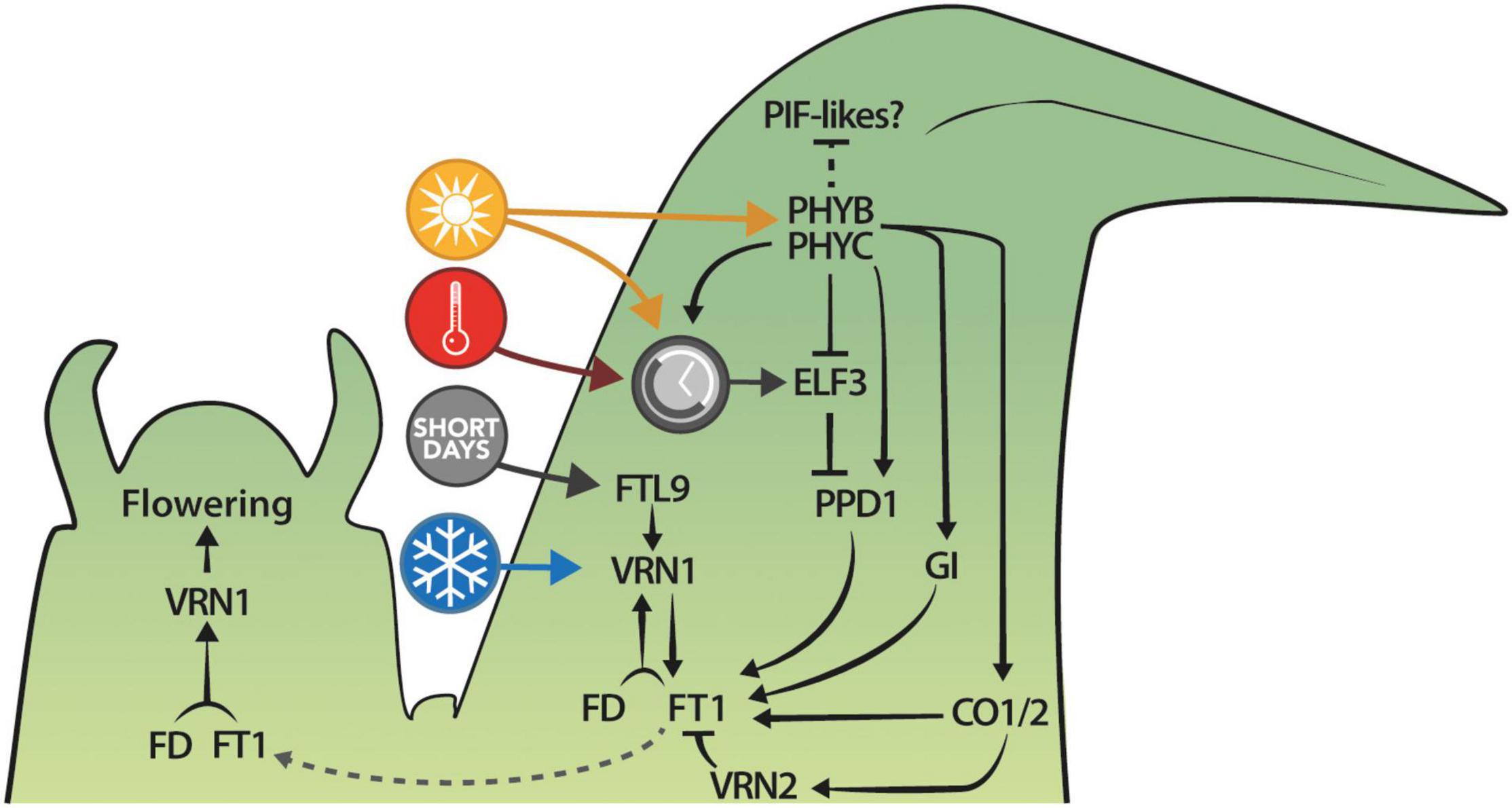
Figure 5. Summary of the current understanding of the photoperiodic regulation of flowering in B. distachyon. Colored circles represent the ambient temperature (red), the photoperiod (yellow), the circadian clock (gray), and the vernalization (blue) pathways.
Finally, the recent improvements in LED technology will help to better understand the genetic regulations occurring in natural environments. Often, the lighting and temperature conditions used to grow plants in culture chambers do not reflect actual outdoor conditions. In A. thaliana, the expression of florigen/FT shows a different pattern in the field— with a peak in the morning—than previously described in the literature (Song et al., 2018). Exposing plants to daily temperature rhythms as well as changing red:far-red ratios in growth chambers was sufficient to mimic its natural expression pattern (Song et al., 2018). In B. distachyon, most of the diurnal gene regulation is caused by changes in the ambient temperature rather than light (Matos et al., 2014; MacKinnon et al., 2020), and phytochromes are known to act as thermosensors as well as photoreceptors (Franklin et al., 2014). Custom LED lighting systems associated with phytotronic cabinets now provide the possibility to better reproduce daily and seasonal cycles of temperature and daylight spectrum in any region of the planet (Wilson et al., 2015), thus opening new areas of exploration regarding the genetic mechanisms governing the adaptation to local environments, an evolutionary process to which ELF3 could be key.
Data Availability Statement
The raw data supporting the conclusions of this article will be made available by the authors, without undue reservation.
Author Contributions
FB, DW, RA, and CP contributed to conception and design of the study. DW, FB, and RA performed the mutagenesis experiment, the screening, and the mapping of elf3 mutant. FB and JL performed the expression analyses and the night break experiments. WL and KM produced plant material for genetic analyses. FB wrote the first draft of the manuscript. DW, WL, RA, and CP contributed to the submitted version of the manuscript. All authors read and approved the submitted version.
Funding
This work was funded in part by the US National Science Foundation (Award IOS-1755224) and by the Great Lakes Bioenergy Research Center, US Department of Energy, Office of Science, Office of Biological and Environmental Research (Award No. DE-SC0018409). The work conducted by the U.S Department of Energy Joint Genome Institute, a DOE Office of Science User Facility, was supported by the Office of Science of the US Department of Energy under Contract No. DE-AC02-05CH11231. FB is grateful to the F.R.S.-FNRS for the award of postdoc fellowship (FC87200) and JL for the award of a Ph.D. fellowship (FC33993). DW is a Howard Hughes Medical Institute Fellow of the Life Sciences Research Foundation and is grateful for the support of Jorge Dubcovsky in his flowering time pursuits.
Conflict of Interest
The authors declare that the research was conducted in the absence of any commercial or financial relationships that could be construed as a potential conflict of interest.
Publisher’s Note
All claims expressed in this article are solely those of the authors and do not necessarily represent those of their affiliated organizations, or those of the publisher, the editors and the reviewers. Any product that may be evaluated in this article, or claim that may be made by its manufacturer, is not guaranteed or endorsed by the publisher.
Acknowledgments
The authors are very grateful to Jorge Dubcovsky for his critical reading and useful comments on the manuscript as well as to undergraduate student Margaux Claes for her assistance in the realization of transcript quantifications.
Supplementary Material
The Supplementary Material for this article can be found online at: https://www.frontiersin.org/articles/10.3389/fpls.2021.769194/full#supplementary-material
Supplementary Figure 1 | Spectra and light conditions records. (A) Spectral distribution of white, red, and far-red lights used during night breaks and end-of-day far-red treatments. (B) Diurnal monitoring of far-red, total light, and photosynthetically active radiations (PAR) intensities for plants exposed to 18 h long day (LD) followed by 1 h end-of-day far-red (FR) treatment (left) or not (right).
Supplementary Figure 2 | Example of a Derived Cleaved Amplified Polymorphic Sequence for elf3 genotyping. The PCR was performed using the dCAPS primer pair shown in Supplementary Table 1. The PCR product was digested using the hpy166ii restriction enzyme (NEB, United States), which cuts only into the WT (+) sequences, creating shorter fragments than in mutated ELF3 sequences (–). The elf3 mutant phenotypes segregated perfectly with the homozygous elf3 mutation.
Supplementary Figure 3 | Complementation of elf3 mutant with the UBI:ELF3 construct. Six independent transgenic lines exhibited rescue of the rapid flowering phenotype in the T0 generation under 16-h LD. Controls were sown 3 weeks before transplantation of T0 lines to soil. The picture was taken 45 days after transplantation to soil.
Supplementary Figure 4 | Flowering phenotype of elf3 mutants overexpressing VRN1. Individuals were segregated from the elf3;UBI:VRN1 F2 population. The picture was taken 110 days after germination under 8 h SD. elf3 mutant flowered after 86.5 ± 13.7 days (n = 15), elf3;UBI:VRN1 flowered after 36.3 ± 2.9 days (n = 27). WT and UBI:VRN1 plants had not flowered after 180 days.
Supplementary Figure 5 | Expression kinetics of CO1 in the elf3 mutant. Relative expression of CO1 during a 24 h time course for WT plants (black triangles) and elf3 mutants (blue circle) cultivated under 8, 12, 16, and 20 h photoperiods. Error bars represent the standard error of the mean. Data were normalized using the geometric mean of ACT3 and UBC18. The white background indicates the light period, and the gray background shows the dark period. Time is expressed as the number of hours following the start of the light period (Zeitgeber time, ZT).
References
Alvarez, M. A., Tranquilli, G., Lewis, S., Kippes, N., and Dubcovsky, J. (2016). Genetic and physical mapping of the earliness per se locus Eps-Am1 in Triticum monococcum identifies EARLY FLOWERING 3 (ELF3) as a candidate gene. Funct. Integr. Genomics 16, 365–382. doi: 10.1007/s10142-016-0490-3
An, H., Roussot, C., Suárez-López, P., Corbesier, L., Vincent, C., Piñeiro, M., et al. (2004). CONSTANS acts in the phloem to regulate a systemic signal that induces photoperiodic flowering of Arabidopsis. Development 131, 3615–3626. doi: 10.1242/dev.01231
Bendix, C., Marshall, C. M., and Harmon, F. G. (2015). Circadian clock genes universally control key agricultural traits. Mol. Plant 8, 1135–1152. doi: 10.1016/j.molp.2015.03.003
Boden, S. A., Kavanová, M., Finnegan, E. J., and Wigge, P. A. (2013). Thermal stress effects on grain yield in Brachypodium distachyon occur via H2A.Z-nucleosomes. Genome Biol. 14:R65. doi: 10.1186/gb-2013-14-6-r65
Boden, S. A., Weiss, D., Ross, J. J., Davies, N. W., Trevaskis, B., Chandler, P. M., et al. (2014). EARLY FLOWERING 3 regulates flowering in spring barley by mediating gibberellin production and flowering locus T expression. Plant Cell 26, 1557–1569. doi: 10.1105/tpc.114.123794
Borthwick, H. A., and Hendricks, S. B. (1960). Photoperiodism in plants. Science 132, 1223–1228. doi: 10.1126/science.132.3435.1223
Bouché, F., Lobet, G., Tocquin, P., and Périlleux, C. (2016). FLOR-ID: an interactive database of flowering-time gene networks in Arabidopsis thaliana. Nucleic Acids Res. 44, D1167–D1171. doi: 10.1093/nar/gkv1054
Bu, T., Lu, S., Wang, K., Dong, L., Li, S., Xie, Q., et al. (2021). A critical role of the soybean evening complex in the control of photoperiod sensitivity and adaptation. Proc. Natl. Acad. Sci. U.S.A. 118:e2010241118. doi: 10.1073/pnas.2010241118
Bullrich, L., Appendino, M., Tranquilli, G., Lewis, S., and Dubcovsky, J. (2002). Mapping of a thermo-sensitive earliness per se gene on Triticum monococcum chromosome 1Am. Theor. Appl. Genet. 105, 585–593. doi: 10.1007/s00122-002-0982-5
Bunning, E. (1937). Die endonome tagesrhythmik als grundlage der photoperiodischen reaktion. Ber. Deut. Bot. Ges. 54, 590–607.
Capovilla, G., Schmid, M., and Posé, D. (2015). Control of flowering by ambient temperature. J. Exp. Bot. 66, 59–69. doi: 10.1093/jxb/eru416
Casal, J. J. (2013). Photoreceptor signaling networks in plant responses to shade. Annu. Rev. Plant Biol. 64, 403–427. doi: 10.1146/annurev-arplant-050312-120221
Casao, M. C., Karsai, I., Igartua, E., Gracia, M. P., Veisz, O., and Casas, A. M. (2011). Adaptation of barley to mild winters: a role for PPDH2. BMC Plant Biol. 11:164. doi: 10.1186/1471-2229-11-164
Chen, A., Li, C., Hu, W., Lau, M. Y., Lin, H., Rockwell, N. C., et al. (2014). Phytochrome C plays a major role in the acceleration of wheat flowering under long-day photoperiod. Proc. Natl. Acad. Sci. U.S.A. 111, 10037–10044. doi: 10.1073/pnas.1409795111
Cheng, M.-C., Kathare, P. K., Paik, I., and Huq, E. (2021). Phytochrome signaling networks. Annu. Rev. Plant Biol. 72, 217–244. doi: 10.1146/annurev-arplant-080620-024221
Corbesier, L., Vincent, C., Jang, S., Fornara, F., Fan, Q., Searle, I., et al. (2007). FT protein movement contributes to long-distance signaling in floral induction of Arabidopsis. Science 316, 1030–1033. doi: 10.1126/science.1141752
Covington, M. F. (2001). ELF3 modulates resetting of the circadian clock in Arabidopsis. Plant Cell Online 13, 1305–1316. doi: 10.1105/tpc.13.6.1305
Covington, M. F., Maloof, J. N., Straume, M., Kay, S. A., and Harmer, S. L. (2008). Global transcriptome analysis reveals circadian regulation of key pathways in plant growth and development. Genome Biol. 9:R130. doi: 10.1186/gb-2008-9-8-r130
Creux, N., and Harmer, S. (2019). Circadian rhythms in plants. Cold Spring Harb. Perspect. Biol. 11:a034611. doi: 10.1101/cshperspect.a034611
Distelfeld, A., and Dubcovsky, J. (2010). Characterization of the maintained vegetative phase deletions from diploid wheat and their effect on VRN2 and FT transcript levels. Mol. Genet. Genom. 283, 223–232. doi: 10.1007/s00438-009-0510-2
Dixon, L. E., Karsai, I., Kiss, T., Adamski, N. M., Liu, Z., Ding, Y., et al. (2019). Vernalization1 controls developmental responses of winter wheat under high ambient temperatures. Development 146:dev172684. doi: 10.1242/dev.172684
Doyle, M. R., Davis, S. J., Bastow, R. M., McWatters, H. G., Kozma-Bognár, L., Nagy, F., et al. (2002). The ELF4 gene controls circadian rhythms and flowering time in Arabidopsis thaliana. Nature 419, 74–77. doi: 10.1038/nature00954
Faure, S., Turner, A. S., Gruszka, D., Christodoulou, V., Davis, S. J., von Korff, M., et al. (2012). Mutation at the circadian clock gene early maturity 8 adapts domesticated barley (Hordeum vulgare) to short growing seasons. Proc. Natl. Acad. Sci. U.S.A. 109, 8328–8333. doi: 10.1073/pnas.1120496109
Ford, B., Deng, W., Clausen, J., Oliver, S., Boden, S., Hemming, M., et al. (2016). Barley (Hordeum vulgare) circadian clock genes can respond rapidly to temperature in an EARLY FLOWERING 3 -dependent manner. J. Exp. Bot. 67, 5517–5528. doi: 10.1093/jxb/erw317
Franklin, K. A., Toledo-Ortiz, G., Pyott, D. E., and Halliday, K. J. (2014). Interaction of light and temperature signalling. J. Exp. Bot. 65, 2859–2871. doi: 10.1093/jxb/eru059
Gao, M., Geng, F., Klose, C., Staudt, A.-M., Huang, H., Nguyen, D., et al. (2019). Phytochromes measure photoperiod in Brachypodium. bioRxiv [Preprint]. doi: 10.1101/697169
Goodstein, D. M., Shu, S., Howson, R., Neupane, R., Hayes, R. D., Fazo, J., et al. (2012). Phytozome: a comparative platform for green plant genomics. Nucleic Acids Res. 40, D1178–D1186. doi: 10.1093/nar/gkr944
Goto, N., Kumagai, T., and Koornneef, M. (1991). Flowering responses to light-breaks in photomorphogenic mutants of Arabidopsis thaliana, a long-day plant. Physiol. Plant. 83, 209–215. doi: 10.1111/j.1399-3054.1991.tb02144.x
Gustafsson, Å, Hagberg, A., and Lundqvist, U. (1960). The induction of early mutants in bonus barley. Hereditas 46, 675–699. doi: 10.1111/j.1601-5223.1960.tb03109.x
Hazen, S. P., Schultz, T. F., Pruneda-Paz, J. L., Borevitz, J. O., Ecker, J. R., and Kay, S. A. (2005). LUX ARRYTHMO encodes a MYB domain protein essential for circadian rhythms. Proc. Natl. Acad. Sci. U.S.A. 102, 10387–10392. doi: 10.1073/pnas.0503029102
Hicks, K. A., Albertson, T. M., and Wagner, D. R. (2001). EARLY FLOWERING 3 encodes a novel protein that regulates circadian clock function and flowering in Arabidopsis. Plant Cell 13, 1281–1292. doi: 10.1105/tpc.010070
Hicks, K. A., Millar, A. J., Carré, I. A., Somers, D. E., Straume, M., Meeks-Wagner, D. R., et al. (1996). Conditional circadian dysfunction of the arabidopsis early-flowering 3 mutant. Science 274, 790–792. doi: 10.1126/science.274.5288.790
Higgins, J. A., Bailey, P. C., and Laurie, D. A. (2010). Comparative genomics of flowering time pathways using Brachypodium distachyon as a model for the temperate grasses. PLoS One 5:e10065. doi: 10.1371/journal.pone.0010065
Hu, W., Figueroa-Balderas, R., Chi-Ham, C., and Lagarias, J. C. (2020). Regulation of monocot and dicot plant development with constitutively active alleles of phytochrome B. Plant Direct 4:e00210. doi: 10.1002/pld3.210
Huang, H., Gehan, M. A., Huss, S. E., Alvarez, S., Lizarraga, C., Gruebbling, E. L., et al. (2017). Cross-species complementation reveals conserved functions for EARLY FLOWERING 3 between monocots and dicots. Plant Direct 1: e00018. doi: 10.1002/pld3.18
Huang, H., and Nusinow, D. A. (2016). Into the evening: complex interactions in the Arabidopsis circadian clock. Trends Genet. 32, 674–686. doi: 10.1016/j.tig.2016.08.002
Itoh, H., Tanaka, Y., and Izawa, T. (2018). Genetic relationship between phytochromes and OsELF3–1 reveals the mode of regulation for the suppression of phytochrome signaling in rice. Plant Cell Physiol. 60, 549–561. doi: 10.1093/pcp/pcy225
Johansson, M., and Staiger, D. (2014). Time to flower: interplay between photoperiod and the circadian clock. J. Exp. Bot. 66, 719–730. doi: 10.1093/jxb/eru441
Jung, J.-H., Barbosa, A. D., Hutin, S., Kumita, J. R., Gao, M., Derwort, D., et al. (2020). A prion-like domain in ELF3 functions as a thermosensor in Arabidopsis. Nature 585, 256–260. doi: 10.1038/s41586-020-2644-7
Kim, W.-Y., Hicks, K. A., and Somers, D. E. (2005). Independent roles for EARLY FLOWERING 3 and ZEITLUPE in the control of circadian timing, hypocotyl length, and flowering time. Plant Physiol. 139, 1557–1569. doi: 10.1104/pp.105.067173
Kippes, N., VanGessel, C., Hamilton, J., Akpinar, A., Budak, H., Dubcovsky, J., et al. (2020). Effect of phyB and phyC loss-of-function mutations on the wheat transcriptome under short and long day photoperiods. BMC Plant Biol. 20:297. doi: 10.1186/s12870-020-02506-0
Legris, M., Ince, Y. Ç, and Fankhauser, C. (2019). Molecular mechanisms underlying phytochrome-controlled morphogenesis in plants. Nat. Commun. 10:5219. doi: 10.1038/s41467-019-13045-0
Leivar, P., and Quail, P. H. (2011). PIFs: pivotal components in a cellular signaling hub. Trends Plant Sci. 16, 19–28. doi: 10.1016/j.tplants.2010.08.003
Li, C., and Dubcovsky, J. (2008). Wheat FT protein regulates VRN1 transcription through interactions with FDL2. Plant J. 55, 543–554. doi: 10.1111/j.1365-313x.2008.03526.x
Li, M., Kennedy, A., Huybrechts, M., Dochy, N., and Geuten, K. (2019). The effect of ambient temperature on Brachypodium distachyon development. Front. Plant Sci. 10:1011. doi: 10.3389/fpls.2019.01011
Lu, S., Zhao, X., Hu, Y., Liu, S., Nan, H., Li, X., et al. (2017). Natural variation at the soybean J locus improves adaptation to the tropics and enhances yield. Nat. Genet. 49, 773–779. doi: 10.1038/ng.3819
Lucas, M., and Prat, S. (2014). PIFs get BRright: phytochrome interacting factors as integrators of light and hormonal signals. New Phytol. 202, 1126–1141. doi: 10.1111/nph.12725
Lundqvist, U. (2009). Eighty Years of Scandinavian Barley Mutation Genetics and Breeding. Rome: Food and Agriculture Organization of the United Nations.
MacKinnon, K. J., Cole, B. J., Yu, C., Coomey, J. H., Hartwick, N. T., Remigereau, M., et al. (2020). Changes in ambient temperature are the prevailing cue in determining Brachypodium distachyon diurnal gene regulation. New Phytol. 227, 1709–1724. doi: 10.1111/nph.16507
Mathews, S. (2010). Evolutionary studies illuminate the structural-functional model of plant phytochromes. Plant Cell 22, 4–16. doi: 10.1105/tpc.109.072280
Matos, D. A., Cole, B. J., Whitney, I. P., MacKinnon, K. J.-M., Kay, S. A., and Hazen, S. P. (2014). Daily changes in temperature, not the circadian clock, regulate growth rate in Brachypodium distachyon. PLoS One 9:e100072. doi: 10.1371/journal.pone.0100072
Matsubara, K., Ogiso-Tanaka, E., Hori, K., Ebana, K., Ando, T., and Yano, M. (2012). Natural variation in Hd17, a homolog of Arabidopsis ELF3 that is involved in rice photoperiodic flowering. Plant Cell Physiol. 53, 709–716. doi: 10.1093/pcp/pcs028
Möglich, A., Yang, X., Ayers, R. A., and Moffat, K. (2010). Structure and function of plant photoreceptors. Annu. Rev. Plant Biol. 61, 21–47. doi: 10.1146/annurev-arplant-042809-112259
Mulki, M. A., and von Korff, M. (2015). Constans controls floral repression by up-regulating vernalization2 (VRN-H2) in Barley. Plant Physiol. 170, 325–337. doi: 10.1104/pp.15.01350
Nieto, C., López-Salmerón, V., Davière, J.-M., and Prat, S. (2015). ELF3-PIF4 interaction regulates plant growth independently of the evening complex. Curr. Biol. 25, 187–193. doi: 10.1016/j.cub.2014.10.070
Nishida, H., Ishihara, D., Ishii, M., Kaneko, T., Kawahigashi, H., Akashi, Y., et al. (2013). Phytochrome C is a key factor controlling long-day flowering in Barley. Plant Physiol. 163, 804–814. doi: 10.1104/pp.113.222570
Oakenfull, R. J., and Davis, S. J. (2017). Shining a light on the Arabidopsis circadian clock. Plant Cell Environ. 40, 2571–2585. doi: 10.1111/pce.13033
Paik, I., and Huq, E. (2019). Plant photoreceptors: multi-functional sensory proteins and their signaling networks. Semin. Cell Dev. Biol. 92, 114–121. doi: 10.1016/j.semcdb.2019.03.007
Pearce, S., Kippes, N., Chen, A., Debernardi, J. M., and Dubcovsky, J. (2016). RNA-seq studies using wheat PHYTOCHROME B and PHYTOCHROME C mutants reveal shared and specific functions in the regulation of flowering and shade-avoidance pathways. BMC Plant Biol. 16:141. doi: 10.1186/s12870-016-0831-3
Pearce, S., Shaw, L. M., Lin, H., Cotter, J. D., Li, C., and Dubcovsky, J. (2017). Night-break experiments shed light on the photoperiod1-mediated flowering. Plant Physiol. 174, 1139–1150. doi: 10.1104/pp.17.00361
Périlleux, C., Bernier, G., and Kinet, J.-M. (1994). Circadian rhythms and the induction of flowering in the long-day grass Lolium temulentum L. Plant Cell Environ. 17, 755–761. doi: 10.1111/j.1365-3040.1994.tb00168.x
Purvis, O. N., and Gregory, F. G. (1937). Studies in vernalisation of cereals: i. a comparative study of vernalisation of winter rye by low temperature and by short days. Ann. Bot 1, 569–591. doi: 10.2307/42906574
Qin, Z., Bai, Y., Muhammad, S., Wu, X., Deng, P., Wu, J., et al. (2019). Divergent roles of FT-like 9 in flowering transition under different day lengths in Brachypodium distachyon. Nat. Commun. 10:812. doi: 10.1038/s41467-019-08785-y
Quail, P. H. (2002). Phytochrome photosensory signalling networks. Nat. Rev. Mol. Cell Bio. 3, 85–93. doi: 10.1038/nrm728
Ream, T. S., Woods, D. P., and Amasino, R. M. (2012). The molecular basis of vernalization in different plant groups. Cold Spring Harb. Symp. Quant Biol. 77, 105–115. doi: 10.1101/sqb.2013.77.014449
Ream, T. S., Woods, D. P., Schwartz, C. J., Sanabria, C. P., Mahoy, J. A., Walters, E. M., et al. (2014). Interaction of photoperiod and vernalization determines flowering time of Brachypodium distachyon. Plant Physiol. 164, 694–709. doi: 10.1104/pp.113.232678
Rubenach, A. J. S., Hecht, V., Schoor, J. K. V., Liew, L. C., Aubert, G., Burstin, J., et al. (2017). EARLY FLOWERING 3 redundancy fine-tunes photoperiod sensitivity. Plant Physiol. 173, 2253–2264. doi: 10.1104/pp.16.01738
Saito, H., Ogiso-Tanaka, E., Okumoto, Y., Yoshitake, Y., Izumi, H., Yokoo, T., et al. (2012). Ef7 encodes an ELF3-like protein and promotes rice flowering by negatively regulating the floral repressor gene GHD7 under both short- and long-day conditions. Plant Cell Physiol. 53, 717–728. doi: 10.1093/pcp/pcs029
Seaton, D. D., Toledo-Ortiz, G., Ganpudi, A., Kubota, A., Imaizumi, T., and Halliday, K. J. (2018). Dawn and photoperiod sensing by phytochrome A. Proc. Natl. Acad. Sci. U.S.A. 115:201803398. doi: 10.1073/pnas.1803398115
Shaw, L. M., Li, C., Woods, D. P., Alvarez, M. A., Lin, H., Lau, M. Y., et al. (2020). Epistatic interactions between PHOTOPERIOD1, CONSTANS1 and CONSTANS2 modulate the photoperiodic response in wheat. PLoS Genet. 16:e1008812. doi: 10.1371/journal.pgen.1008812
Shaw, L. M., Turner, A. S., Herry, L., Griffiths, S., and Laurie, D. A. (2013). Mutant Alleles of Photoperiod-1 in Wheat (Triticum aestivum L.) that confer a late flowering phenotype in long days. PLoS One 8:e79459. doi: 10.1371/journal.pone.0079459
Song, Y. H., Kubota, A., Kwon, M. S., Covington, M. F., Lee, N., Taagen, E. R., et al. (2018). Molecular basis of flowering under natural long-day conditions in Arabidopsis. Nat. Plants 4, 824–835. doi: 10.1038/s41477-018-0253-3
Song, Y. H., Shim, J. S., Kinmonth-Schultz, H. A., and Imaizumi, T. (2015). Photoperiodic flowering: time measurement mechanisms in leaves. Annu. Rev. Plant Biol. 66, 441–464. doi: 10.1146/annurev-arplant-043014-115555
Strasser, B., Alvarez, M. J., Califano, A., and Cerdán, P. D. (2009). A complementary role for ELF3 and TFL1 in the regulation of flowering time by ambient temperature. Plant J. 58, 629–640. doi: 10.1111/j.1365-313x.2009.03811.x
Suárez-López, P., Wheatley, K., Robson, F., Onouchi, H., Valverde, F., and Coupland, G. (2001). CONSTANS mediates between the circadian clock and the control of flowering in Arabidopsis. Nature 410, 1116–1120. doi: 10.1038/35074138
Tamaki, S., Matsuo, S., Wong, H. L., Yokoi, S., and Shimamoto, K. (2007). Hd3a protein is a mobile flowering signal in rice. Science 316, 1033–1036. doi: 10.1126/science.1141753
Thines, B., and Harmon, F. G. (2010). Ambient temperature response establishes ELF3 as a required component of the core Arabidopsis circadian clock. Proc. Natl. Acad. Sci. U.S.A. 107, 3257–3262. doi: 10.1073/pnas.0911006107
Turner, A., Beales, J., Faure, S., Dunford, R. P., and Laurie, D. A. (2005). The pseudo-response regulator Ppd-H1 provides adaptation to photoperiod in Barley. Science 310, 1031–1034. doi: 10.1126/science.1117619
Ugarte, C. C., Trupkin, S. A., Ghiglione, H., Slafer, G., and Casal, J. J. (2010). Low red/far-red ratios delay spike and stem growth in wheat. J. Exp. Bot. 61, 3151–3162. doi: 10.1093/jxb/erq140
Valverde, F., Mouradov, A., Soppe, W., Ravenscroft, D., Samach, A., and Coupland, G. (2004). Photoreceptor regulation of constans protein in photoperiodic flowering. Science 303, 1003–1006. doi: 10.1126/science.1091761
Vandesompele, J., Preter, K. D., Pattyn, F., Poppe, B., Roy, N. V., Paepe, A. D., et al. (2002). Accurate normalization of real-time quantitative RT-PCR data by geometric averaging of multiple internal control genes. Genome Biol. 3:research0034. doi: 10.1186/gb-2002-3-7-research0034
Vogel, J., and Hill, T. (2008). High-efficiency agrobacterium-mediated transformation of Brachypodium distachyon inbred line Bd21-3. Plant Cell Rep. 27, 471–478. doi: 10.1007/s00299-007-0472-y
Wang, J., Wen, W., Hanif, M., Xia, X., Wang, H., Liu, S., et al. (2016). TaELF3-1DL, a homolog of ELF3, is associated with heading date in bread wheat. Mol. Breed. 36:161. doi: 10.1007/s11032-016-0585-5
Weller, J. L., Liew, L. C., Hecht, V. F. G., Rajandran, V., Laurie, R. E., Ridge, S., et al. (2012). A conserved molecular basis for photoperiod adaptation in two temperate legumes. Proc. Natl. Acad. Sci. U.S.A. 109, 21158–21163. doi: 10.1073/pnas.1207943110
Wilhelm, E. P., Turner, A. S., and Laurie, D. A. (2009). Photoperiod insensitive Ppd-A1a mutations in tetraploid wheat (Triticum durum Desf.). Theor. Appl. Genet. 118, 285–294. doi: 10.1007/s00122-008-0898-9
Willige, B. C., Zander, M., Yoo, C. Y., Phan, A., Garza, R. M., Trigg, S. A., et al. (2021). Phytochrome-interacting factors trigger environmentally responsive chromatin dynamics in plants. Nat. Genet. 53, 955–961. doi: 10.1038/s41588-021-00882-3
Wilson, P., Streich, J., and Borevitz, J. (2015). “Genetics and genomics of Brachypodium,” in Plant Genetics and Genomics: Crops and Models, ed. J. P. Vogel (New York, NY: Springer International Publishing), 107–127. doi: 10.1007/7397_2015_18
Woods, D., Dong, Y., Bouche, F., Bednarek, R., Rowe, M., Ream, T., et al. (2019). A florigen paralog is required for short-day vernalization in a pooid grass. Elife 8:e42153. doi: 10.7554/elife.42153
Woods, D. P., and Amasino, R. M. (2015). “Genetics and genomics of Brachypodium,” in Plant Genetics and Genomics: Crops and Models, ed. J. P. Vogel (New York, NY: Springer International Publishing), 259–273. doi: 10.1007/7397_2015_10
Woods, D. P., Bednarek, R., Bouché, F., Gordon, S. P., Vogel, J. P., Garvin, D. F., et al. (2017). Genetic architecture of flowering-time variation in Brachypodium distachyon. Plant Physiol. 173, 269–279. doi: 10.1104/pp.16.01178
Woods, D. P., McKeown, M. A., Dong, Y., Preston, J. C., and Amasino, R. M. (2016). Evolution of VRN2/Ghd7-like genes in vernalization-mediated repression of grass flowering. Plant Physiol. 170, 2124–2135. doi: 10.1104/pp.15.01279
Woods, D. P., Ream, T. S., Minevich, G., Hobert, O., and Amasino, R. M. (2014). Phytochrome C is an essential light receptor for photoperiodic flowering in the temperate grass, Brachypodium distachyon. Genetics 198, 397–408. doi: 10.1534/genetics.114.166785
Yang, Y., Peng, Q., Chen, G.-X., Li, X.-H., and Wu, C.-Y. (2013). OsELF3 is involved in circadian clock regulation for promoting flowering under long-day conditions in rice. Mol. Plant 6, 202–215. doi: 10.1093/mp/sss062
Zagotta, M. T., Hicks, K. A., Jacobs, C. I., Young, J. C., Hangarter, R. P., and Meeks-Wagner, D. R. (1996). The Arabidopsis ELF3 gene regulates vegetative photomorphogenesis and the photoperiodic induction of flowering. Plant J. 10, 691–702. doi: 10.1046/j.1365-313x.1996.10040691.x
Zakhrabekova, S., Gough, S. P., Braumann, I., Müller, A. H., Lundqvist, J., Ahmann, K., et al. (2012). Induced mutations in circadian clock regulator Mat-a facilitated short-season adaptation and range extension in cultivated barley. Proc. Natl. Acad. Sci. U.S.A. 109, 4326–4331. doi: 10.1073/pnas.1113009109
Keywords: Brachypodium, flowering, circadian clock, photoperiod, ELF3, Pooideae, temperate grasses
Citation: Bouché F, Woods DP, Linden J, Li W, Mayer KS, Amasino RM and Périlleux C (2022) EARLY FLOWERING 3 and Photoperiod Sensing in Brachypodium distachyon. Front. Plant Sci. 12:769194. doi: 10.3389/fpls.2021.769194
Received: 01 September 2021; Accepted: 13 December 2021;
Published: 06 January 2022.
Edited by:
Chris Helliwell, Commonwealth Scientific and Industrial Research Organisation (CSIRO), AustraliaReviewed by:
Richard Macknight, University of Otago, New ZealandNorihito Nakamichi, Nagoya University, Japan
Copyright © 2022 Bouché, Woods, Linden, Li, Mayer, Amasino and Périlleux. This is an open-access article distributed under the terms of the Creative Commons Attribution License (CC BY). The use, distribution or reproduction in other forums is permitted, provided the original author(s) and the copyright owner(s) are credited and that the original publication in this journal is cited, in accordance with accepted academic practice. No use, distribution or reproduction is permitted which does not comply with these terms.
*Correspondence: Claire Périlleux, cperilleux@uliege.be
†These authors have contributed equally to this work and share last authorship