- 1MSU-DOE Plant Research Laboratory, Michigan State University, East Lansing, MI, United States
- 2Great Lakes Bioenergy Research Center, Michigan State University, East Lansing, MI, United States
- 3W. K. Kellogg Biological Station, Michigan State University, Hickory Corners, MI, United States
- 4Center for Global Change and Earth Observations, Michigan State University, East Lansing, MI, United States
- 5Department of Geography, Environment, and Spatial Sciences, Michigan State University, East Lansing, MI, United States
- 6Department of Integrative Biology, Michigan State University, East Lansing, MI, United States
- 7Department of Plant, Soil, and Microbial Sciences, Michigan State University, East Lansing, MI, United States
- 8Department of Plant Biology, Michigan State University, East Lansing, MI, United States
Leaf photosynthesis of perennial grasses usually decreases markedly from early to late summer, even when the canopy remains green and environmental conditions are favorable for photosynthesis. Understanding the physiological basis of this photosynthetic decline reveals the potential for yield improvement. We tested the association of seasonal photosynthetic decline in switchgrass (Panicum virgatum L.) with water availability by comparing plants experiencing ambient rainfall with plants in a rainfall exclusion experiment in Michigan, USA. For switchgrass exposed to ambient rainfall, daily net CO2 assimilation ( ) declined from 0.9 mol CO2 m-2 day-1 in early summer to 0.43 mol CO2 m-2 day-1 in late summer (53% reduction; P<0.0001). Under rainfall exclusion shelters, soil water content was 73% lower and was 12% and 26% lower in July and September, respectively, compared to those of the rainfed plants. Despite these differences, the seasonal photosynthetic decline was similar in the season-long rainfall exclusion compared to the rainfed plants; in switchgrass under the shelters declined from 0.85 mol CO2 m-2 day-1 in early summer to 0.39 mol CO2 m-2 day-1 (54% reduction; P<0.0001) in late summer. These results suggest that while water deficit limited late in the season, abundant late-season rainfalls were not enough to restore in the rainfed plants to early-summer values suggesting water deficit was not the sole driver of the decline. Alongside change in photosynthesis, starch in the rhizomes increased 4-fold (P<0.0001) and stabilized when leaf photosynthesis reached constant low values. Additionally, water limitation under shelters had no negative effects on the timing of rhizome starch accumulation, and rhizome starch content increased ~ 6-fold. These results showed that rhizomes also affect leaf photosynthesis during the growing season. Towards the end of the growing season, when vegetative growth is completed and rhizome reserves are filled, diminishing rhizome sink activity likely explained the observed photosynthetic declines in plants under both ambient and reduced water availability.
Introduction
Leaf photosynthesis of perennial grasses follows a clear seasonal dynamic – peaking with vegetative growth in summer and declining towards late summer several weeks before the end of the growing season while leaves are still green. This dynamic occurs in many genera of grasses including the bioenergy crops Panicum, Miscanthus, and Saccharum spp., and even in some evergreen and deciduous trees (Eggemeyer et al., 2006; Kosugi and Matsuo, 2006; Inman-Bamber et al., 2011; De Souza et al., 2013; Boersma et al., 2015; Gao et al., 2015; Yan et al., 2015; De Souza et al., 2018; Endres et al., 2019; Rusinowski et al., 2019; Stavridou et al., 2020). This seasonal decline in photosynthesis could be partially adaptative as it is associated with the remobilization of nutrients from leaves to belowground perennating organs, which allows the plant to recycle nutrients that otherwise would be lost to leaf drop (Yang et al., 2009; Yang et al., 2016; Yang and Udvardi, 2018; Massey et al., 2020). Beyond this putative adaptive role in nutrient retranslocation, additional physiological and environmental drivers of this decrease in photosynthesis are unknown but could have large effects on end-of-season biomass and long-term yield dynamics.
Perennial grasses notably increase in size during the growing season, with larger plants being progressively more susceptible to water limitation as a larger transpiring leaf area increases total water demand and increasingly depletes available soil water (Liu et al., 2016; Mocko and Jones, 2021; Wang et al., 2021). Therefore, the observed photosynthetic decline could be a consequence of water limitation as plants become larger and soil water becomes less available later in the growing season. Alternatively, the larger size could also lead to a nitrogen (N) limitation (Leroy et al., 2022), however, this decline was not affected by N availability across a wide range of N fertilization rates (Tejera et al., 2022).
Among perennial grasses, switchgrass (Panicum virgatum L.) is a leading candidate for cellulosic bioenergy feedstock in the United States (Parrish and Fike, 2005). Evidence increasingly supports switchgrass as a drought tolerant species, based on its leaf photosynthesis and plant growth resiliency in the face of water limitation (Barney et al., 2009; Lovell et al., 2016; Taylor et al., 2016; Hawkes and Kiniry, 2018; Chen et al., 2020). This suggests that water limitation may not drive the photosynthesis decline observed late in the growing season. However, previous water limitation studies mainly focused on the initial photosynthetic response to drought (Lovell et al., 2016; Taylor et al., 2016; Chen et al., 2020), and the response through the entire growing season is not as well resolved. Measuring drought effects on photosynthesis over the growing season is costly as it requires large rainfall-exclusion shelters in field experiments. Additionally, these experiments usually rely on natural precipitation events as control treatments, reducing the power of the experiment to detect water-deficit effects during dry periods. Despite these drawbacks, rainfall-exclusion experiments can be more realistic than controlled irrigation studies performed in greenhouses because field experiments include other environmental factors (Lovell et al., 2016).
Switchgrass leaf photosynthesis response to water limitation is mainly studied at peak light availability (Lovell et al., 2016; Taylor et al., 2016; Chen et al., 2020). While measurements at peak solar irradiance are useful to characterize photosynthesis when heat stress is maximal, they do not capture diurnal changes in light and water availability. These point measurements thus fail to incorporate the midday or early afternoon periods of photosynthesis depression observed in other perennials (Gao et al., 2015; Bucci et al., 2019), and overlook changes in the source-sink dynamic during the day.
The late-season photosynthesis decline could also be driven by co-occurring physiological changes. Specifically, physiological signals like carbohydrate buildup and sink strength are known to limit development and leaf photosynthesis in certain perennial grasses (McCormick et al., 2009; Van Heerden et al., 2010; De Souza et al., 2018; Ruiz-Vera et al., 2021; Tejera et al., 2021; Tejera et al., 2022) . For example, when the photoassimilate source-sink balance was perturbed by shading some leaves of sugarcane plants, photosynthesis in the unshaded leaves increased by 32%, indicating source-sink coordination at the level of the entire plant to match supply (source) with demand (sinks) (McCormick et al., 2006). Similarly, when sink strength was reduced by cold-girdling leaves or by placing leaves in sucrose solution, photosynthesis decreased by 30 – 45%, probably inhibited by foliar sucrose and hexose concentrations that increased 2 – 3 fold (McCormick et al., 2008). These studies reveal the short-term effects of source/sink relationships on photosynthetic rates in perennial systems but fail to explain seasonal dynamics and environmental limitations. The late-season decrease in photosynthesis could be caused by sink limitations in carbohydrate storage organs (Van Heerden et al., 2010; De Souza et al., 2018). As the season progresses, major sink organs and processes (i.e., growth, storage refill, reproduction) cease activity and limit their carbohydrate consumption, which would then lead to a lower demand for photosynthate and ultimately reducing leaf photosynthesis (Tejera et al., 2022).
Water limitation also imposes restrictions on carbon sink organs and processes that may interact with carbohydrate buildup and photosynthesis limitations (Lemoine et al., 2013; Rodrigues et al., 2019). On the one hand, water limitation causes a decrease in photosynthesis which in turn may lead to depletion of carbohydrate in belowground storage organs (Barney et al., 2009; Lovell et al., 2016; Taylor et al., 2016; Hawkes and Kiniry, 2018; Ivanov et al., 2019; Chen et al., 2020). After water stress is ameliorated, rhizomes would then resume carbohydrate accumulation, allowing photosynthesis to persist for longer in the season. On the other hand, water limitation also reduces above- and below-ground growth (Barney et al., 2009; Mann et al., 2013; Hui et al., 2018), which could cause additional sink limitations. In this case, the carbohydrate buildup in the rhizomes would diminish earlier in the season, with corresponding declines in switchgrass photosynthesis.
To resolve these interacting and potentially conflicting effects of source-sink relations and water deficit on the seasonal changes of photosynthesis, we studied the seasonal dynamics of photosynthesis in switchgrass, representing a fast-growing perennial grass, under rainfed and experimentally induced water limitation treatments. Specifically, we asked: 1) Does switchgrass photosynthesis correlate with rhizome sink strength on a seasonal basis under conditions of ambient rainfall and soil water availability? and 2) Does experimentally imposed season-long water limitation affect seasonal patterns of photosynthesis and sink strength, and particularly does it accelerate the onset of the observed late-season photosynthesis decline? To address these questions, we measured switchgrass source activity (i.e., diurnal course of photosynthesis) and sink strengths of carbohydrates in source (leaves) and sink (rhizome) organs in mature switchgrass stands across an entire growing season. The expected seasonal decrease in leaf photosynthesis, found in both plot and field-scale switchgrass stands, co-occurred with peak carbohydrate concentrations in both leaves and rhizomes. Water limitation reduced switchgrass leaf photosynthesis in July and September but, abundant late-season rainfalls were not enough to restore in the rainfed plants to early-summer values. Additionally, water limitations had no effects on rhizome starch dynamics and starch in the rhizomes increased ~ 6-fold. These results suggest that while water deficit limited in the late season, late-season precipitations did not restore to early-summer values suggesting that other limitations were also in place. Towards the end of the growing season, when vegetative growth is completed and rhizome reserves are filled, the insufficient sink activity presents a strong limitation, leading to the observed photosynthetic decline.
Materials and methods
Experimental design
Water limitation was imposed by placing rainfall exclusion shelters over switchgrass plots that are part of the Biofuel Cropping System Experiment (BCSE) of the Great Lakes Bioenergy Research Center (GLBRC), located at the Kellogg Biological Station (KBS) Long-term Ecological Research site in Hickory Corners, Michigan, USA (42.394290 N, -85.374126 W). For our experiment, we used four replicate switchgrass plots. Switchgrass (cv. Cave-in-rock) was planted in June 2008 at a seeding rate of 7.71 kg ha-1 and row spacing of 0.2 m and received 56 kg N ha-1 yr-1 after its initial establishment year (Sanford et al., 2016). The switchgrass was re-seeded in 2009 due to intense storms in mid-summer 2008 that redistributed un-germinated seeds. Each plot was 30 x 40 m and had a rainfall exclusion shelter that measured 4.2 x 5.5 m and 2.6 m tall located at least 1 m inside the plot. Corrugated roofing panels (Greca Lexan; Amerilux, De Pere, WI, USA) allowed ~90% light transmittance (385-700 nm). In 2020, the year of sampling, rainfall exclusion shelters were in place from May 27 – September 10. In 2019 rainfall exclusion shelters had been in place over the same footprints from May 24 – June 22 and September 9 – October 11, and in 2018 from June 13 – October 24. Soil volumetric water content (VWC) sensors (CS655; Campbell Scientific Inc. CSI, Logan, UT, USA) were installed in two plots horizontally at 0.10 m and 0.25 m depth under the rainfall exclusion shelter, and in the open field within 4m radius of the shelter.
Data and sample collection
We sampled the experiment five times during the 2020 growing season (19 June, 1 July, 28 July 6 August, and 3 September) to cover the entire switchgrass growth cycle. For the first two sampling dates switchgrass stands were in vegetative stages, for the third and fourth dates the stands were in reproductive stages, and for the last date the stands were >75% senesced. On each sampling date, we sampled plants outside the rainfall exclusion shelter (Rainfed treatment) and under the shelters (Rainfall exclusion shelter treatment) from each plot. Rainfall exclusion shelter samples were taken from a sampling area of 1 m2. Rainfed samples were collected from two sampling areas separated 4 m from each other. A 1-m2 end-of-season biomass sample was collected on November 3, 2020, from the center portion of each shelter, as well as from the rainfed sampling locations. Samples were dried at 60°C until constant moisture and weighed to estimate the dry biomass.
On each sampling date, we conducted a diurnal sampling of photosynthetic rate, leaf water potential (LWP), and carbohydrate content in source (leaves) and sink (rhizome) organs. The first and last timepoints of the diurnal samplings were collected before sunrise and after sunset. Three timepoints were collected during the day: at mid-morning (9:00 – 10:00 h), noon (13:00 – 14:00 h) and mid-afternoon (17:00 – 18:00 h). We sampled rhizomes before sunrise, around solar noon, and after sunset. Leaf net CO2 assimilation rate ( Anet ) and stomatal conductance to water (gsw) were measured in the middle portion of the youngest fully expanded leaf using an open gas exchange system (Li-6800; LI-COR Biosciences, Lincoln, NE, USA) equipped with an integrated modulated chlorophyll fluorometer and a light source. Air temperature, photosynthetic photon flux density (PPDF), and relative humidity (RH) inside the leaf chamber were set to mimic ambient conditions at each sampling time The Li-6800 controls the leaf environment using internal LED lighting, Peltier heating/cooling units and controlling incoming CO2 and H2O content using a series of chemical scrubbing columns. The CO2 concentration was maintained at 400 μmol mol–1. The diurnal sum of Anet ( ) was calculated using the area under the curve (AUC), with sunrise and sunset as the limits of integration. Non-photochemical quenching and dark-adapted Photosystem II (PSII) maximum quantum yield (Fv/Fm) values were measured using chlorophyll fluorescence from dark-adapted leaves at the pre-dawn sampling.
PSII quantum efficiency ( ϕPSII ) was calculated as:
where Fs is the steady-state fluorescence, and is the maximum fluorescence after a saturating light flash.
At each sampling in each plot, we evaluated two leaf samples for a total of 16 samples per sampling, 80 per sampling date, and 400 for the entire experiment. The same leaf was first measured with the Li-6800, then placed in a pressure chamber (model 1505D; PMS Instruments, Albany, OR, USA) equipped with a grass compression gland to measure its leaf water potential (LWP), and then flash-frozen in liquid nitrogen (N) within 5 min of harvest. A small rhizome sample (~3 g) was harvested from the same stem that bore the leaf and immediately frozen in liquid N. In all samplings, rhizomes were clearly distinguished from stems and newly formed tillers (Figure S1). All samples were kept in liquid N until the next day and then stored at -80°C until further processing.
Sample processing
Leaf samples were ground to a fine powder with a mortar and pestle. Rhizome samples were ground with a spice mixer (Cuisinart; SG-10). All samples stayed frozen during grinding and were then freeze-dried for at least 48 hours in a lyophilizer. Diurnal concentrations of starch, sucrose and free glucose were measured in leaves ( [starch]leaf, [suc]leaf , [glu]leaf , respectively) and rhizomes ( [starch]rhi, [suc]rhi , [glu]rhi ) for the five sampling dates. Diurnal accumulation rates of these carbohydrates were estimated as the slope of the linear regression of predawn and daylight values over time. All assays were performed at the Biomass Analytics Facility at Michigan State University following procedures described in Santoro et al. (2010) and Sekhon et al. (2016). In brief, glucose content was assayed using the glucose oxidase/peroxidase (GOPOD) method (K-GLUC, Megazyme, Ireland). To determine sucrose and starch concentrations, samples were first treated with a combination of alkaline buffer and high heat to degrade all pre-existing free glucose. Then samples were treated with invertase (Sigma-Aldrich, St. Louis, MO), or amyloglucosidase (K-TSTA, Megazyme, Ireland) and 5 μL α-amylase (K-TSTA, Megazyme) for sucrose and starch extraction, respectively. The rest of the processing was identical to that used for glucose.
Canopy-level gross CO2 assimilation
Gross primary production (GPP), defined here as canopy-level gross CO2 assimilation (canopy Agross ), was estimated from eddy covariance tower net ecosystem CO2 exchange (NEE) observations in an 18-ha switchgrass stand of the same age with similar soil type and management at the Lux Arbor reserve (42.476100N, -85.446945W), 11 km from the experimental site (Abraha et al., 2018). NEE was measured at 10 Hz using an LI-7500 open-path infrared gas analyzer (LI-COR Biosciences) and a CSAT3 three-dimensional sonic anemometer (Campbell Scientific Inc.). The raw data were processed to compute 30-min NEEs. The 30-min NEEs were gap-filled and partitioned into ecosystem respiration (Reco) and GPP using the nighttime partitioning method in REddyProc package (Reichstein et al., 2005; Wutzler et al., 2018). The method assumes NEE is equal to Reco, and GPP is zero during the night. Reco is then estimated – for both day and nighttime – from air temperature and nighttime Reco relationship, and GPP is computed as a residual of the estimated Reco and NEE (Abraha et al., 2018). Air temperature, incident solar radiation and vapor pressure deficit were also measured at the site.
Data analysis
We used R software (R Core Team, 2017) for all analyses and plots. All models had the same random structure. We used lmer() in the lme4 package (Bates et al., 2015) to fit the mixed models, Anova() in the car package (Fox and Weisberg, 2011) for analysis of deviance using type II Wald chi-square test, and emmeans() in the emmeans package (Lenth et al., 2018) for mean and slope comparisons.
Results
Leaf photosynthesis decreased by ~50% in the latter half of the growing season.
Leaf photosynthesis decreased by ~50% in the latter half of the growing season. Under ambient rainfall, switchgrass photosynthesis during the growing season markedly decreased by late July, prior to visible canopy senescence. Specifically, decreased from 0.84 – 0.96 mol CO2 m-2 day-1 in early summer (19 June and 1 July) to 0.30 – 0.50 mol CO2 m-2 day-1 in late summer (28 July, 6 August and 3 September; Figures 1, 2). This ~53% reduction in from early to late summer (P< 0.001) was contributed from the consistently low Anet values throughout the day (Figure 2; P< 0.001). Changes in Anet or were not explained by increases in leaf respiration because nocturnal respiration rates changed little during the season, with no significant differences found between early and late summer (Figure 2A; P = 0.90). Corresponding with changes in Anet , ϕPSII and the electron transport rate (ETR) had similar seasonal pattern, with mid-morning, noon and mid-afternoon ϕPSII at 29 – 45% lower in late-summer (Figure 3; P< 0.001).
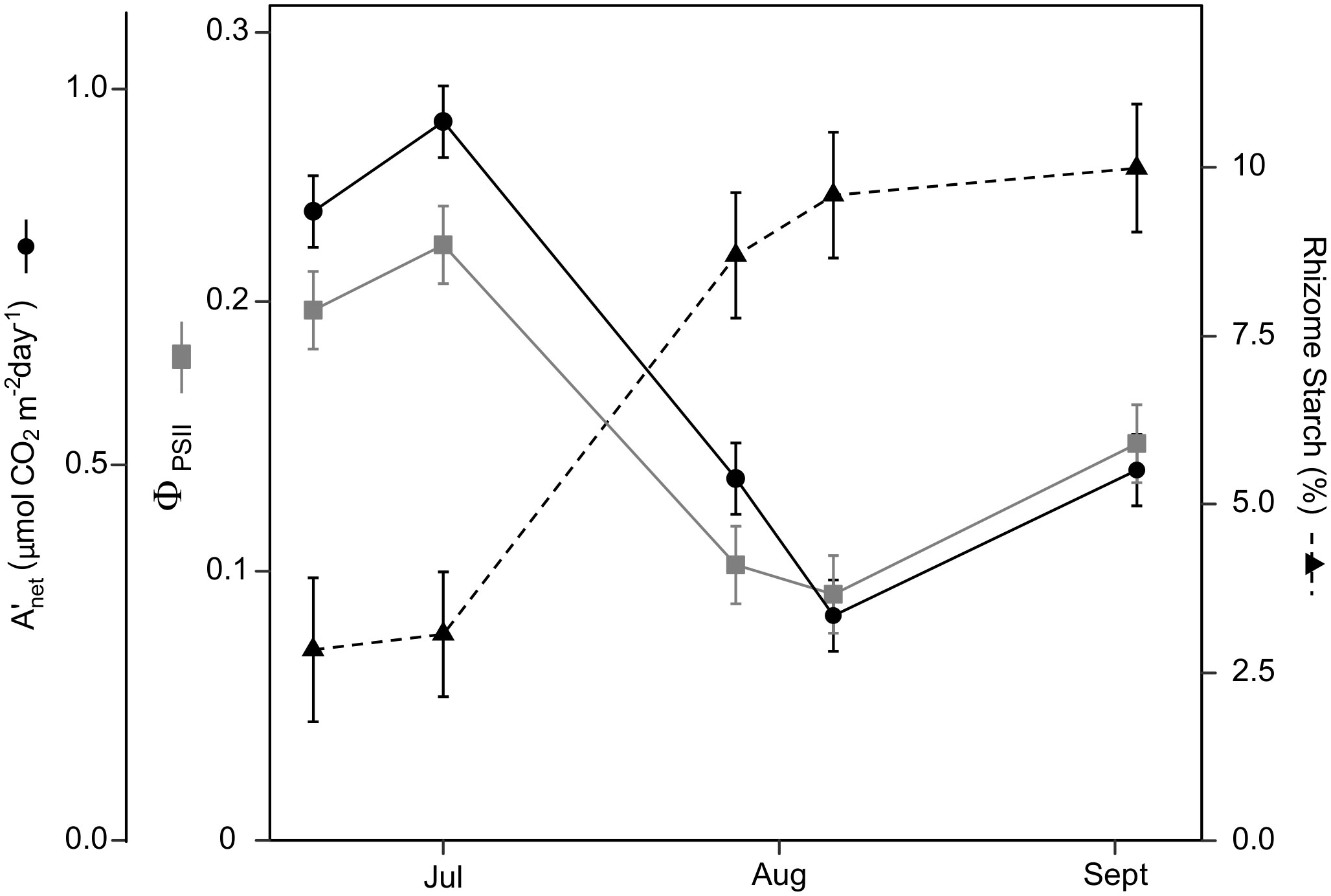
Figure 1 Switchgrass daily net CO2 assimilation ( ), efficiency of photosystem II ( PSII ), and rhizome starch content during 2020. As the season progressed and PSII decreased by ~50%, while rhizome starch increased 4-fold. During the first two sampling dates, switchgrass was in vegetative stages, the next two was in reproductive stages and in the last measurement switchgrass was 70% senesced. Error bars represent plus/minus one standard error of the mean (n = 4).
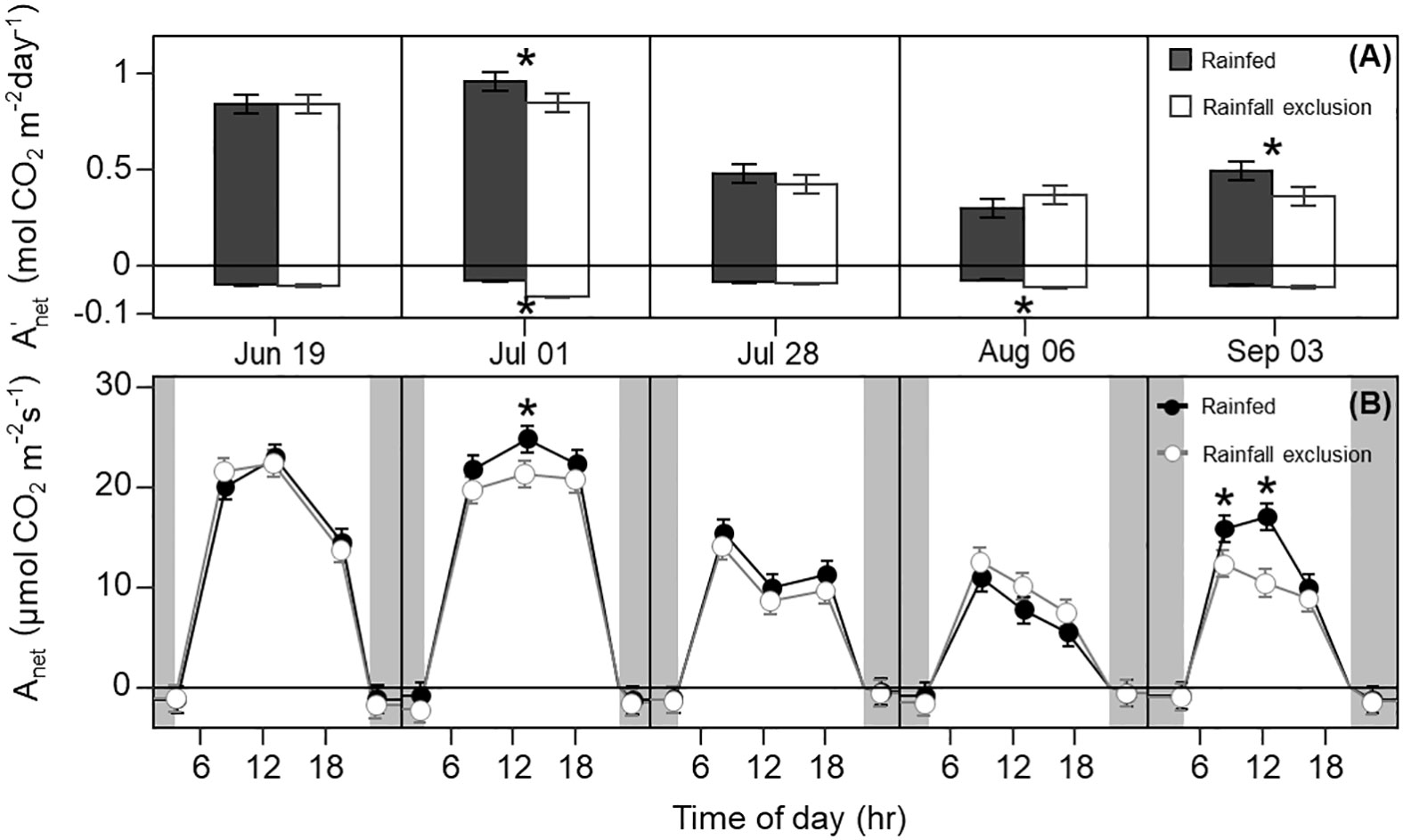
Figure 2 Switchgrass daily net CO2 assimilation ( ; A) integrated over each sampling date, and net CO2 assimilation rate ( ; B) at each timepoint during the day, for plants grown under (white fill, grey line) and outside (black fill, black line) rainfall exclusion shelters. Negative values indicate dark respiration during the night period (A), and dark respiration at predawn and post-dusk timepoints (B). Asterisks indicate a significant difference between treatments (P< 0.05). Data are mean ± S.E. (n = 4).
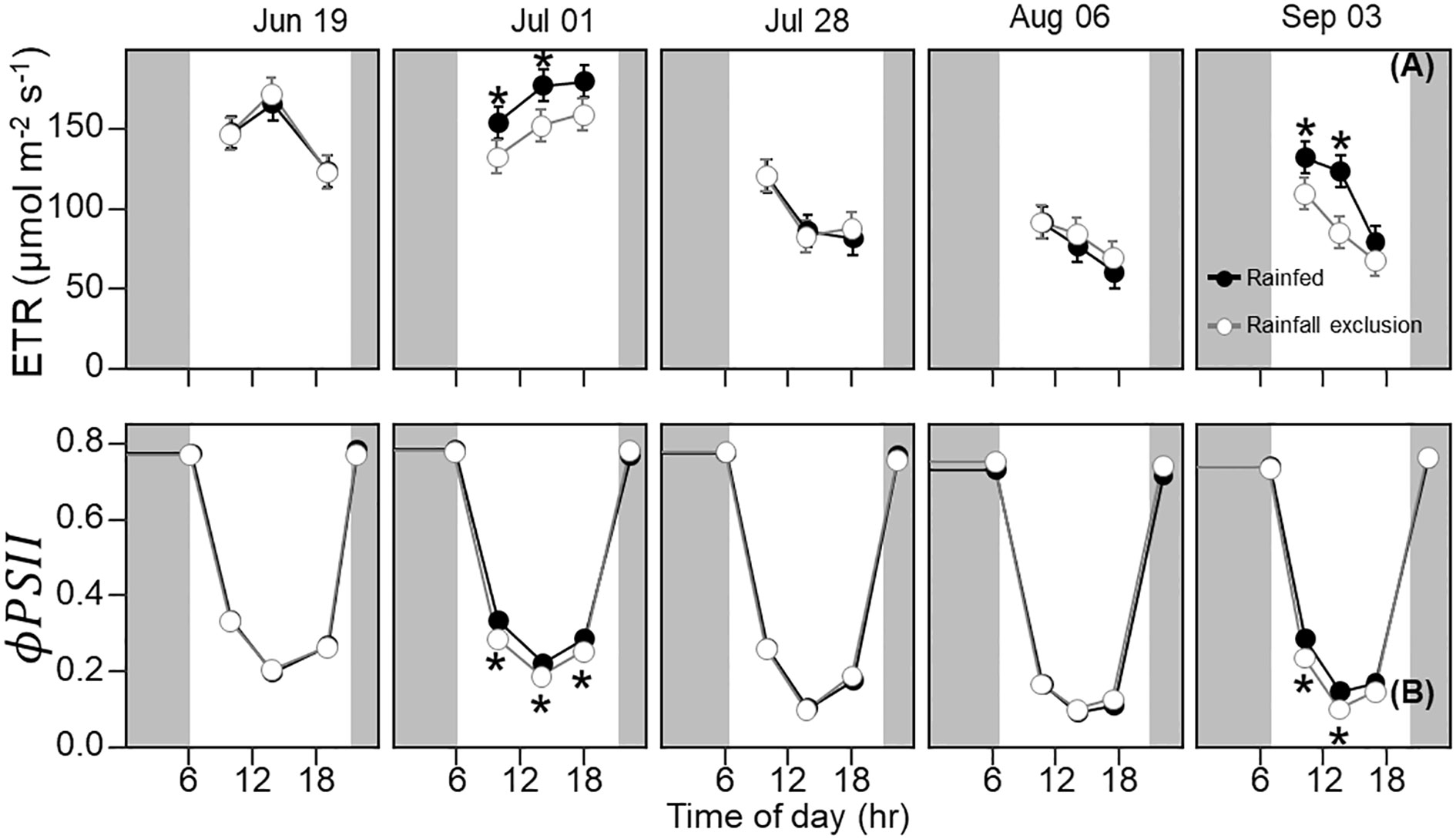
Figure 3 Switchgrass electron transport rate ( ETR ; (A) and photosystem II quantum efficiency ( ϕPSII ; (B) at each timepoint during the day, over the course of the growing season, for plants grown under (white fill, grey line) and outside (black fill, black line) rainfall exclusion shelters. Asterisks indicate a significant difference between treatments (P< 0.05). Data are mean ± S.E. (n = 4).
Canopy-level CO2 gross assimilation decreased by ~34% from June to September
Canopy photosynthesis under ambient rainfall as measured by eddy covariance at the Lux Arbor switchgrass stand peaked in July and steadily decreased during the second half of the growing season (Figure 4). Specifically, Canopy Agross decreased by ~34%, from ~1.24 mol CO2 m-2 day-1 in early-summer (June 19 – July 1) to ~0.82 mol CO2 m-2 day-1 in late-summer (July 28 – September 3; Figure S2). Canopy Agross and leaf Anet were highly correlated both as individual measurements and daily accumulation (P<0.0001; Figure S3), indicating that the observed decline at the leaf level in the upper canopy was not compensated by a larger canopy leaf area in late summer. Comparison of canopy Agross with climatic variables shows that the beginning of the canopy Agross decline occurred almost six weeks sooner than the seasonal declines in air temperature or incident radiation (Figure S4).
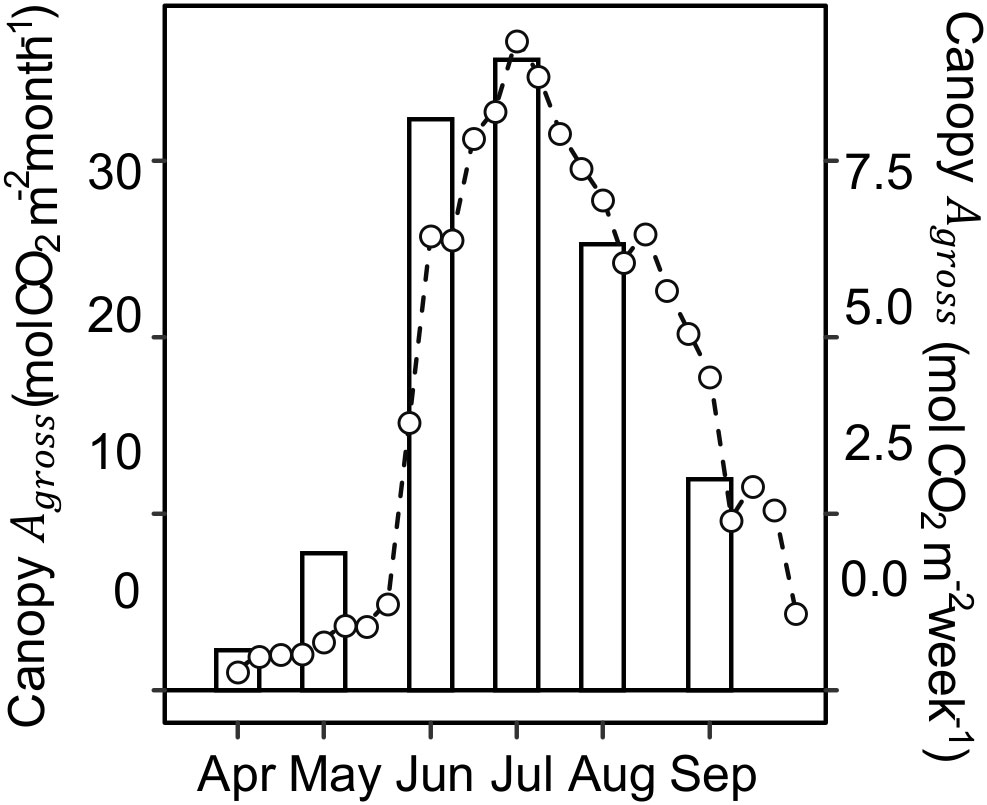
Figure 4 Monthly (bars) and weekly (lines and open circles) canopy gross CO2 assimilation (Canopy Agross ) during the 2020 growing season. Canopy Agross was computed from net ecosystem CO2 exchange (NEE) observations using eddy covariance method conducted at a switchgrass field of the same age as the experimental site but located 11 km away.
Leaf carbohydrates had strong diurnal dynamics, while rhizome carbohydrate followed strong seasonal dynamics
Under ambient rainfall, leaf carbohydrates had no clear seasonal dynamics but did show clear diurnal dynamics (Figure S5). Leaf glucose and sucrose followed the diurnal pattern of photosynthesis, being lower in morning and afternoon samplings, and higher at noon. Leaf starch, in contrast, tended to accumulate during the day; its accumulation rate was ~7-fold higher than the other carbohydrates when expressed on a glucose-equivalent basis (Figure S5; Table S1). September 4 showed the largest fluctuation in rhizome starch in the ambient treatment. It is unclear if this midday decrease in rhizome starch is of physiological meaning or a product of sample variability. Overall, rhizome carbohydrate concentrations remained constant during the day and changed over the course of the season (Figure 5). Glucose and sucrose concentrations decreased from late July onward, while the starch concentration showed a corresponding increase (Figure 5 and Table 1S). Rhizome glucose decreased from 3.1% in early summer to 1.5% in late summer (52% decrease; P< 0.0001; Figure 5), while rhizome sucrose decreased from 2.2% to 1.6% (28% decrease; P = 0.022; Figure 5). Starch increased from 2.6% in early summer to 9.9% in late summer (3.94-fold increase; P< 0.0001; Figure 5).
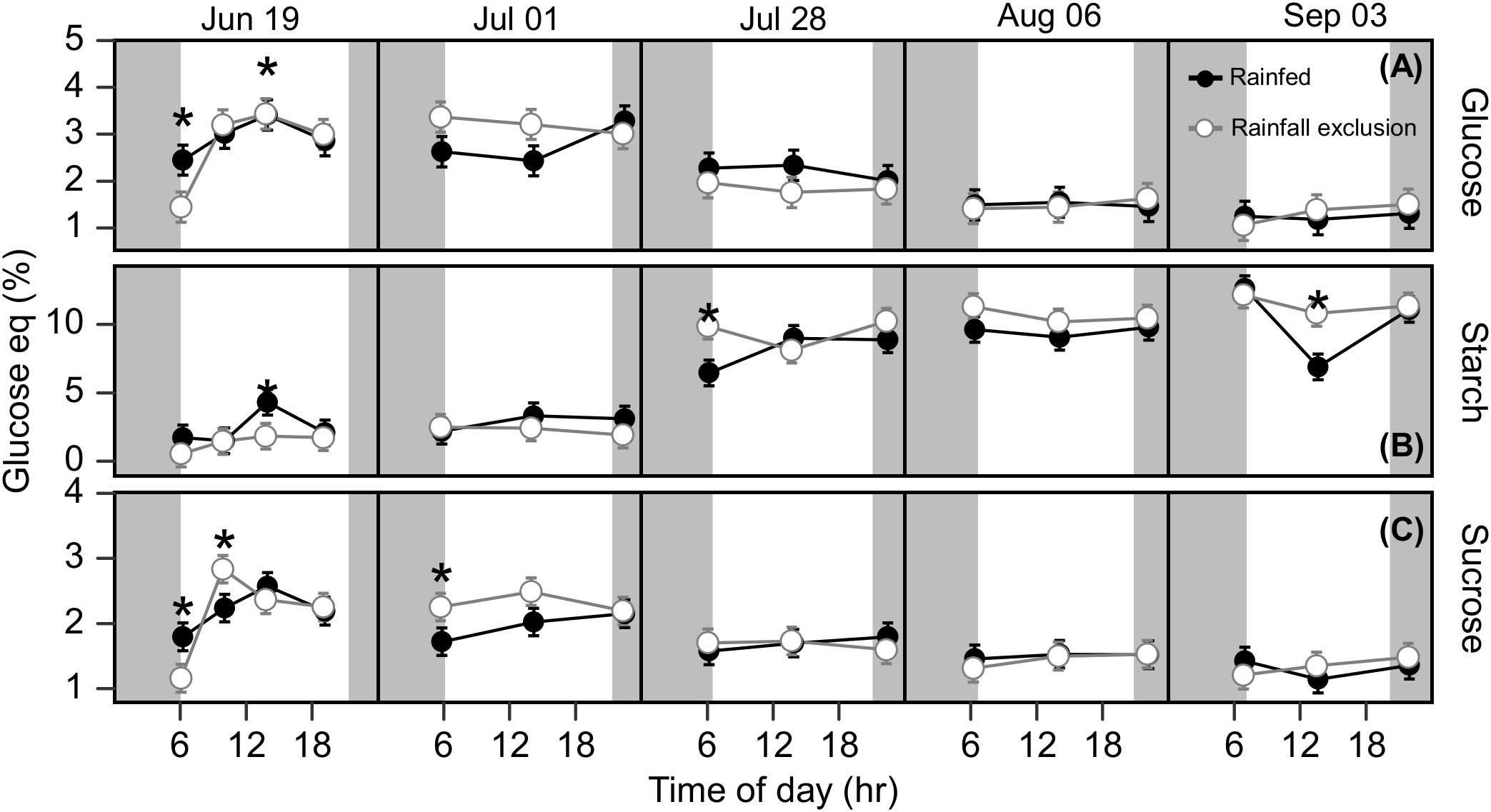
Figure 5 Rhizome free glucose (A), starch (B) and sucrose (C) at each timepoint during the day, over the course of the growing season, for plants grown inside (white fill, grey line) and outside (black fill, black line) rainfall exclusion shelters. Asterisks indicate a significant difference between treatments (P< 0.05). Data are mean ± S.E. (n = 4).
End-of-season biomass was 33% lower, and soil water content was ~27% lower under the rainfall exclusion shelters
The 2020 growing season witnessed several large rain events (Table 1; Figure S6A). Rainfall exclusion shelters successfully blocked rainfall and imposed water limitation for the switchgrass plots. Soil VWC was consistently lower under the rainfall exclusion shelters at the 10-cm and 25-cm depths (Table 1; Figure S6B). After each rain event, soil VWC under the shelters was at least 2-fold, and on average 3.7-fold, lower than outside the rainfall exclusion shelters. End-of-season aboveground biomass of the switchgrass was 4.67 ± 0.52 kg m-2 outside the rainfall exclusion shelters and 2.95 ± 0.52 kg m-2 under the rainfall exclusion shelters (~37% decrease; P = 0.059).
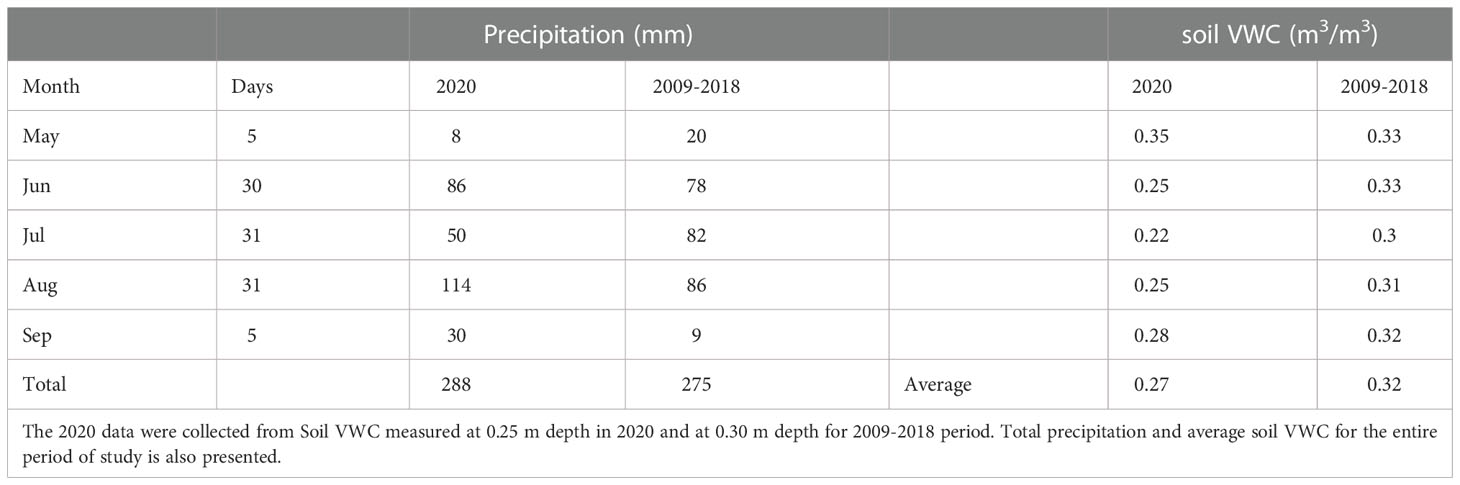
Table 1 Total monthly precipitation (mm) and soil volumetric water content (VWC; m3/m3) in 2020 at the rainfall exclusion shelters Biofuel Cropping System Experiment (42.394290, -85.374126) and historic record (2009-2018) between shelter deployment (May 27th) and last sampling date (Sept 3rd).
Imposed water-deficit conditions decreased leaf photosynthesis, ϕPSII , and leaf water potential
The imposed season-long water deficit reduced leaf photosynthesis during the growing season. Plants under the shelters showed 12% and 26% lower on July 1 (P = 0.059) and September 3, respectively, compared to outside of the shelters (P = 0.030; Figure 2). These differences were mainly driven by reductions in Anet at noon, which decreased by 14% and 38% on July 1 (P = 0.023) and September 3, respectively (P< 0.0001; Figure 2). In addition, Anet under the shelters was 22% lower in the mid-morning on September 3 (P = 0.023). Similarly, plants under the shelters had reduced ϕPSII on the same dates and timepoints of the day; ϕPSII was on average ~5% lower under the shelters on July 1 (P = 0.013) and September 3 (P< 0.0049; Figure 3).
Water-deficit effects on LWP were consistent over the season, and we found significant effects for at least one sampling timepoint in 4 out of the 5 sampling dates (Figure 6A). Plants under the rainfall exclusion shelters had 12 – 37% lower LWP than rainfed plants. These differences only corresponded with significant effects on Anet and on September 3 after a large precipitation event. No water-deficit effects were found on stomatal conductance ( gsw) during the day or across the growing season (P > 0.1; Figure 6B).
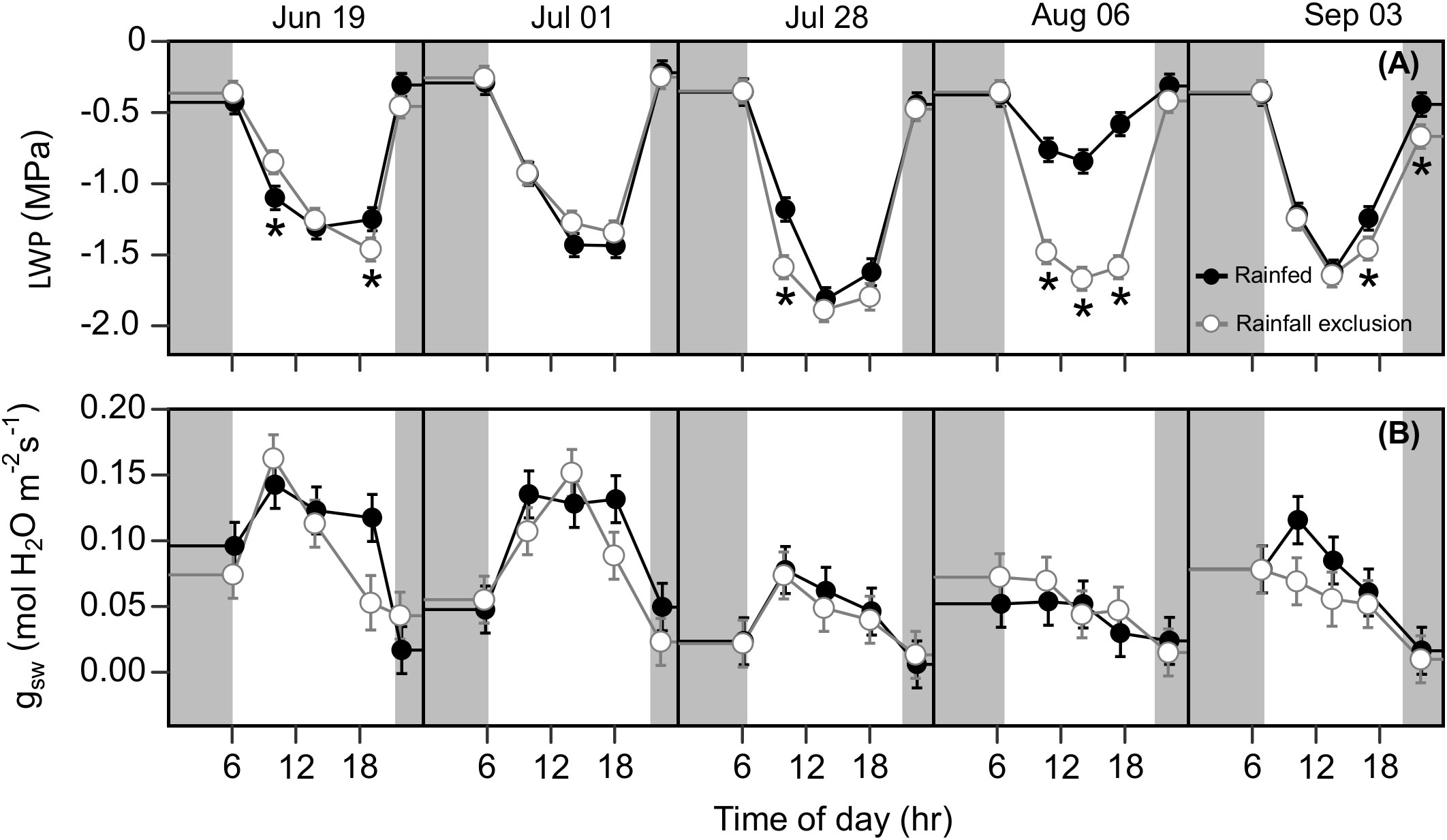
Figure 6 Switchgrass leaf water potential ( LWP ; (A) and stomatal conductance to water ( gsw ; (B) at each timepoint during the day, over the course of the growing season, for plants grown under (white fill, grey line) and outside (black fill, black line) rainfall exclusion shelters. Asterisks indicate a significant difference between treatments (P< 0.05). Data are mean ± S.E. (n = 4).
Imposed water-deficit conditions had only marginal effects on leaf glucose accumulation, and no effects on rhizome carbohydrates
Rhizome carbohydrates were not affected significantly by the imposed water-deficit conditions in most comparisons (Figure 5). Leaves had on average 12 – 22% higher mean sucrose and starch concentrations outside the shelters, but the differences were not significant (Pglucose = 0.13; Psucrose = 0.17; Pstarch = 0.015; Figure S5). In late summer, plants outside the shelters had higher leaf glucose and starch accumulation rates than under the shelters (Table S1). Sucrose accumulation rates in the leaf were positive in plants outside the shelters until July 28 and then became almost null until the end of the experiment (Table S1). In turn, sucrose accumulation in plants under the shelters followed the opposite pattern, i.e., positive only after July 28 (Table S1).
Discussion
Switchgrass carbon source-sink balances markedly changed during the growing season. In early summer under ambient rainfall, source indicators (i.e., leaf photosynthesis: Anet , , ϕPSII , ETR; Figures 1, 2, 4) were at their seasonal maximum, and sink indicators (i.e., leaf and rhizome carbohydrate concentrations) were at their seasonal minimum (Figures 1, 5). As the season progressed, switchgrass plants transitioned to lower photosynthetic activity ( Anet and decreased by ~ 50%) and higher sink activities (4-fold increase in rhizome starch; Figure 5). This seasonal decline in photosynthetic rates was also observed at the canopy level based on eddy covariance estimates of canopy Agross (Figure 4) at a companion site. Exclusion of rainfall by shelters across the entire growing season showed that water limitation did not alter these dynamics; the photosynthetic decline and carbohydrate accumulation showed similar onsets and magnitudes to the rainfed plants (Figures 2, 3, 5; Figure S4). While late-season rainfalls increased switchgrass photosynthesis, they did not restore to early-summer values, which supports the hypothesis that decreasing rhizome sink demand drives the seasonal photosynthetic decrease more than does decreasing water availability.
Sink limitations
The late summer photosynthetic decline may be driven by sink limitations triggered by rhizome carbohydrate buildup. As perennial grass biomass allocation to flowers and seeds is minimal (Boe, 2007; Giannoulis et al., 2016), mature stands may lack major sinks for photosynthates once vegetative growth has ceased (Tejera et al., 2021; Tejera et al., 2022) and rhizome carbohydrate accumulation is complete. Rhizome carbohydrate concentrations and leaf photosynthesis were inversely correlated, and as rhizome starch reached maximum accumulation in mid-summer, leaf photosynthesis declined to low rates (Figures 1, 5). Similar seasonal patterns have been reported in other perennial grasses. In sugarcane (Saccharum sp.), sucrose (the carbohydrate storage molecule for that species) increased 5-fold in the main sink organ during summer while Anet decreased by >50% (De Souza et al., 2018), and in Miscanthus × giganteus starch content in the leaf increased by 8-fold by the end of the growing season while Anet decreased ~50% (De Souza et al., 2013). The findings of our study on switchgrass support the sink limitation hypothesis and resolve the interacting and potentially conflicting effects of source-sink relations and water deficit on seasonal changes of photosynthesis. Our results showed that water deficit conditions later in the season had insignificant effects on the photosynthetic decline.
The decrease in leaf photosynthesis was accompanied by a decrease in ϕPSII (Figures 1, 3), suggesting that autumnal senescence was ongoing. Autumnal senescence is characterized by a decrease in chlorophyll content and an up-regulation of genes associated with protein degradation (Palmer et al., 2015; Palmer et al., 2019). During this process, light-harvesting pigments of the photosynthetic system are degraded (Moy et al., 2015), leading to N retranslocation from aboveground to belowground organs (Yang et al., 2009; Yang et al., 2016; Yang and Udvardi, 2018; Massey et al., 2020), and a concomitant decrease in photosynthetic capacity (Tang et al., 2005; Galvagno et al., 2013; Donnelly et al., 2020). Therefore, the decline in leaf photosynthesis may not be driven solely by sink limitations but also a decrease in photochemical efficiency of photosynthesis resulting from senescence. Note that sink limitations and senescence are not mutually exclusive mechanisms but rather could be a coherent response triggered by the environment or carbohydrate buildup and sink limitations. Sugar and/or starch accumulation is known to trigger leaf senescence in maize, trees, and other species, but this has not been tested in perennial grasses (Noodén et al., 1997; Wingler and Roitsch, 2008; Holland et al., 2016).
Water-deficit
Experimentally imposed water stress affected switchgrass photosynthesis both in early and late summer. If water availability had been the sole driver of the late-season photosynthesis decline, switchgrass plants outside the shelters, where soil water was replenished after precipitation events, would have shown different seasonal dynamics (e.g., later onset of the decline or no decline). In our experiment, we found that late-season rainfall increased switchgrass photosynthesis, but the magnitude of the effect was not enough to compensate for the seasonal decline. In comparison, the imposed water limitation had marginal effects on rhizome carbohydrate accumulation; concentrations from plants under and outside the rainfall exclusion shelters were almost identical (Figure 5). Leaf carbohydrates were less resilient to water deficit; during the growing season switchgrass leaves under the shelters had 12 – 22% lower sucrose and starch content (Figure S5). Carbohydrate accumulation rate, as a direct proxy for sink activity, was also higher under the shelters than outside the shelter in early-summer (Table S1). These differences in leaf starch corresponded with some differences in . Our interpretation is that plants under the shelter with lower Anet had less carbon available for sucrose and starch synthesis, leading to lower sucrose and starch in the leaf (Figure S5). With lower carbohydrate concentrations in the leaf, switchgrass growing under the shelters would need to adjust carbohydrate mobilization and degradation through the night to avoid carbon starvation at the end of the night (Smith and Stitt, 2007). This reduction in carbohydrate mobilization and degradation at night may drive the lower nocturnal respiration rates observed on July 1 and August 6.
Water deficit effects on switchgrass are mainly studied using leaf photosynthesis and physiological responses, while effects on sink activities are not as well understood. Few studies reporting changes in leaf carbohydrate under drought conditions in switchgrass suggest that the response may be specific to the particular storage carbohydrate. Key carbohydrates such as trehalose, fructose (Liu et al., 2015), and proline (Kim et al., 2016; Hoover et al., 2018) seem to be more readily affected, while other soluble sugars such as glucose or sucrose as well as starch remain relatively constant (Hoover et al., 2018). Our work is distinct from earlier water deficit studies because we present diurnal and seasonal leaf carbohydrate measurements. We found that the small effects of water deficit on diurnal dynamics of leaf carbohydrates did not show a clear relationship with the seasonal course. Our results suggest that starch, in addition to accumulating in the rhizomes during the growing season, acts as a transitory carbohydrate storage molecule in switchgrass leaves, accumulating during the day and presumably being consumed overnight.
Switchgrass resilience to drought
Switchgrass photosynthesis was resistant to water limitation. Even when soil VWC dropped by ~4-fold under the shelters, and Anet decreased by up to ~26%. Other studies have found a stronger negative leaf photosynthesis response to water deficit (Barney et al., 2009; Lovell et al., 2016; Taylor et al., 2016; Hawkes and Kiniry, 2018; Chen et al., 2020), including across 49 switchgrass genotypes in which decreased by 40 – 80% under drought stress (Liu et al., 2015). This discrepancy may be because the control treatment in our field experiment (i.e., plants outside the shelter) experienced ambient environmental conditions, while other studies used irrigated plants as the control. While the latter is useful to characterize the response, our approach is more consistent with the natural environment and allows a better characterization of the seasonal dynamics.
In light of our results, it may seem counterintuitive that, at the whole-plant level, end-of-season aboveground biomass was reduced by 33% by the shelters. The switchgrass strategy to cope with water deficit stress may rely on whole-plant responses, such as modifying the number of tillers and/or leaves per tiller, producing smaller and thinner stands under the shelter while maintaining leaves that had similar photosynthetic performance to leaves outside the shelters. Alternatively, differences in end-of-season biomass could be driven by switchgrass stands with similar tiller density and leaf area, but small (and often insignificant) differences in leaf photosynthesis accumulate over the growing season yielding larger differences in end-of-season biomass.
The presence of late-season decline in photosynthesis as an improvement strategy for switchgrass and other perennials
The switchgrass photosynthetic decline over the growing season, which we observed in both plot and field-scale switchgrass stands, reveals a potential for yield improvement. Using simple linear interpolation between sampling dates, the upper canopy of switchgrass assimilated ~45 mol CO2 m-2 during the growing season. If leaf photosynthesis had remained constant at early summer levels during the 6 weeks when the environment was still favorable, switchgrass would have assimilated an additional 52% carbon. Scaling leaf-level photosynthesis to end-of-season biomass is beyond the scope of this study, but assuming end-of-season biomass is proportional to the accumulated CO2 during the study period, this forgone 52% of CO2 assimilation could potentially lead to an extra ~1.2 Mg ha-1 of end-of-season aboveground biomass. While these calculations oversimplify the relationship between gross carbon fixation and biomass production, the physiological understanding of this decline could elucidate ways to maintain higher photosynthetic rates for longer in the season and thereby increase biomass yields. This late-season decline in photosynthesis is commonly observed in many perennial grasses, and therefore improvement strategies could have a broader impact on cropping systems that include perennial grasses and possibly trees (Kosugi and Matsuo, 2006; Boersma et al., 2015; De Souza et al., 2018; Kar et al., 2020; Stavridou et al., 2020).
Conclusions
Switchgrass leaf photosynthesis decreased by ~50% during the latter half of the growing season. This seasonal photosynthetic decline appears to be common across several perennial grasses, but the underlying mechanisms and the potential implications for management are not well understood. Our results suggest that this decline is not entirely driven by water limitation since leaf photosynthesis outside the rainfall exclusion shelters remained at low values even after heavy rain events in late-summer and early spring. Eddy covariance data shows that the decline also occurred at the whole-field scale and represents a physiological response that occurs even when environmental conditions remain favorable for photosynthesis. We suggest sink limitation as an important driving mechanism of the photosynthetic decline, as rhizome starch reached peak concentrations around the same time that leaf photosynthesis fell to lower rates. If sink limitation were the leading cause of the seasonal photosynthesis decline, then strategies to alleviate sink limitation could be included in switchgrass breeding programs with the goal of increasing yields.
Data availability statement
The raw data supporting the conclusions of this article will be made available by the authors, without undue reservation.
Author contributions
All authors contributed ideas; MT-N collected and analyzed field data and led the writing of the manuscript. MA and JC maintained eddy covariance towers, and collected and curated the data. SH and GR contributed to conception and design of the experiment. BW oversaw and provided substantial contributions to the project. All authors contributed to the article and approved the submitted version.
Funding
This work was supported by the Great Lakes Bioenergy Research Center, U.S. Department of Energy, Office of Science, Office of Biological and Environmental Research under Award Number DE-SC0018409, by the National Science Foundation Long-term Ecological Research Program (DEB 1832042) at the Kellogg Biological Station, and by Michigan State University AgBioResearch.
Acknowledgments
We thank Kevin Kahmark for constructing and maintaining the rainfall exclusion shelters, and Joe Simmons and Stacey VanderWulp for site management.
Conflict of interest
The authors declare that the research was conducted in the absence of any commercial or financial relationships that could be construed as a potential conflict of interest.
Publisher’s note
All claims expressed in this article are solely those of the authors and do not necessarily represent those of their affiliated organizations, or those of the publisher, the editors and the reviewers. Any product that may be evaluated in this article, or claim that may be made by its manufacturer, is not guaranteed or endorsed by the publisher.
Supplementary material
The Supplementary Material for this article can be found online at: https://www.frontiersin.org/articles/10.3389/fpls.2022.1023571/full#supplementary-material
References
Abraha, M., Hamilton, S. K., Chen, J., Robertson, G. P. (2018). Ecosystem carbon exchange on conversion of conservation reserve program grasslands to annual and perennial cropping systems. Agric. For. Meteorology. 253–254, 151–160. doi: 10.1016/J.AGRFORMET.2018.02.016
Barney, J. N., Mann, J. J., Kyser, G. B., Blumwald, E., Van Deynze, A., DiTomaso, J. M. (2009). Tolerance of switchgrass to extreme soil moisture stress: Ecological implications. Plant Sci. 177 (6), 724–732. doi: 10.1016/j.plantsci.2009.09.003
Bates, D., Mächler, M., Bolker, B., Walker, S. (2015). Fitting linear mixed-effects models using lme4. J. Stat. Softw. 67 (1), 1–48. doi: 10.18637/jss.v067.i01
Boe, A. (2007). Variation between two switchgrass cultivars for components of vegetative and seed biomass. Crop Science. 47 (2), 636–640. doi: 10.2135/CROPSCI2006.04.0260
Boersma, N. N., Dohleman, F. G., Miguez, F. E., Heaton, E. A. (2015). Autumnal leaf senescence in Miscanthus × giganteus and leaf [N] differ by stand age. J Experimental Botany 66, 14, 4395–4401. doi: 10.1093/jxb/erv129
Bucci, S. J., Carbonell Silletta, L. M., Garré, A., Cavallaro, A., Efron, S. T., Arias, N. S., et al. (2019). Functional relationships between hydraulic traits and the timing of diurnal depression of photosynthesis. Plant Cell Environment. John Wiley Sons Ltd 42 (5), 1603–1614. doi: 10.1111/pce.13512
Chen, P., Chen, J., Sun, M., Yan, H., Feng, G., Wu, B., et al. (2020). Comparative transcriptome study of switchgrass (Panicum virgatum l.) homologous autopolyploid and its parental amphidiploid responding to consistent drought stress. Biotechnol. Biofuels. BioMed. Cent. 13 (1), 1–18. doi: 10.1186/s13068-020-01810-z
Core Team, R. (2017) (Vienna). Available at: https://www.r-project.org/.
De Souza, A. P., Arundale, R. A., Dohleman, F. G., Long, S. P., Buckeridge, M. S. (2013). Will the exceptional productivity of Miscanthus x giganteus increase further under rising atmospheric CO2? Agric. For. Meteorology. 171–172, 82–92. doi: 10.1016/j.agrformet.2012.11.006
De Souza, A. P., Grandis, A., Arenque-Musa, B. C., Buckeridge, M. S.. (2018). Diurnal variation in gas exchange and nonstructural carbohydrates throughout sugarcane development. Funct. Plant Biol. 45 (8), 865–876. doi: 10.1071/FP17268
Donnelly, A., Yu, R., Rehberg, C., Meyer, G., Young, E. B. (2020). Leaf chlorophyll estimates of temperate deciduous shrubs during autumn senescence using a SPAD-502 meter and calibration with extracted chlorophyll. Ann. For. Sci. 77 (2), 30. doi: 10.1007/s13595-020-00940-6
Eggemeyer, K. D., Awada, T., Wedin, D. A., Harvey, F. E., Zhou, X. (2006). Ecophysiology of two native invasive woody species and two dominant warm-season grasses in the semiarid grasslands of the Nebraska sandhills. Int. J. Plant Sci. 167 (5), 991–999. doi: 10.1086/505797
Endres, L., dos Santos, C. M., Silva, J. V., Barbosa de, G. V. S., Silva, A. L. J., Froehlich, A., et al. (2019). Inter-relationship between photosynthetic efficiency, Δ13C, antioxidant activity and sugarcane yield under drought stress in field conditions. J. Agron. Crop Sci. 205 (5), 433–446. doi: 10.1111/jac.12336
Fox, J., Weisberg, S. (2011). Available at: http://socserv.socsci.mcmaster.ca/jfox/Books/Companion.
Galvagno, M., Rossini, M., Migliavacca, M., Cremonese, E., Colombo, R., Morra di Cella, U. (2013). Seasonal course of photosynthetic efficiency in Larix decidua mill. in response to temperature and change in pigment composition during senescence. Int. J. Biometeorology 57 (6), 871–880. doi: 10.1007/s00484-012-0614-y
Gao, Z. J., Xu, B. C., Wang, J., Huo, L. J., Li, S.. (2015). Diurnal and seasonal variations in photosynthetic characteristics of switchgrass in semiarid region on the loess plateau of China. Photosynthetica 53 (4), 489–498. doi: 10.1007/s11099-015-0108-3
Giannoulis, K. D., Karyotis, T., Sakellariou-Makrantonaki, M., Bastiaans, L., Struik, P. C., Danalatos, N. G. (2016). Switchgrass biomass partitioning and growth characteristics under different management practices. NJAS - Wageningen J. Life Sci. Taylor Francis 78, 61–67. doi: 10.1016/j.njas.2016.03.011
Hawkes, C., Kiniry, J. (2018). Legacies in switchgrass resistance to and recovery from drought suggest that good years can sustain plants through bad years. Bioenergy Res. Bioenergy Res. 11 (1), 86–94. doi: 10.1007/s12155-017-9879-7
Holland, V., Koller, S., Lukas, S., Brüggemann, W. (2016). Drought- and frost-induced accumulation of soluble carbohydrates during accelerated senescence in quercus pubescens. Trees 30 (1), 215–226. doi: 10.1007/s00468-015-1290-4
Hoover, A., Emerson, R., Ray, A., Stevens, D., Morgan, S., Cortez, M., et al. (2018). Impact of drought on chemical composition and sugar yields from dilute-acid pretreatment and enzymatic hydrolysis of miscanthus, a tall fescue mixture, and switchgrass. Front. Energy Res. 6, 54. doi: 10.3389/fenrg.2018.00054
Hui, D., et al. (2018). Effects of precipitation changes on switchgrass photosynthesis, growth, and biomass: A mesocosm experiment. PloS One 13 (2), 1–18. doi: 10.1371/journal.pone.0192555
Inman-Bamber, N. G., Jackson, P. A., Hewitt, M. (2011). Sucrose accumulation in sugarcane stalks does not limit photosynthesis and biomass production. Crop Pasture Sci. 62 (10), 848–858. doi: 10.1071/CP11128
Ivanov, Y. V., Kartashov, A. V., Zlobin, I. E., Sarvin, B., Stavrianidi, A. N., Kuznetsov, V. V., et al. (2019). Water deficit-dependent changes in non-structural carbohydrate profiles, growth and mortality of pine and spruce seedlings in hydroculture. Environ. Exp. Bot. 157, 151–160. doi: 10.1016/j.envexpbot.2018.10.016
Kar, S., Weng, T. Y., Nakashima, T., Villanueva-Morales, A., Stewart, J. R., Sacks, E. J., et al. (2020). Field performance of Saccharum × Miscanthus intergeneric hybrids (Miscanes) under cool climatic conditions of northern Japan. Bioenergy Res. 13 (1), 132–146. doi: 10.1007/s12155-019-10066-x
Kim, J., Liu, Y., Zhang, X., Zhao, B., Childs, K. L. (2016). Analysis of salt-induced physiological and proline changes in 46 switchgrass (Panicum virgatum) lines indicates multiple response modes. Plant Physiol. Biochem. 105, 203–212. doi: 10.1016/j.plaphy.2016.04.020
Kosugi, Y., Matsuo, N. (2006). Seasonal fluctuations and temperature dependence of leaf gas exchange parameters of co-occurring evergreen and deciduous trees in a temperate broad-leaved forest. Tree Physiol. 26 (9), 1173–1184. doi: 10.1093/treephys/26.9.1173
Lemoine, R., La Camera, S., Atanassova, R., Dédaldéchamp, F., Allario, T., Pourtau, N., et al. (2013). Source-to-sink transport of sugar and regulation by environmental factors. Front. Plant Sci. 4. doi: 10.3389/fpls.2013.00272
Lenth, R., et al. (2018). Available at: https://cran.r-project.org/package=emmeans.
Leroy, J., Ferchaud, F., Giauffret, C., Mary, B., Fingar, L., Mignot, E., et al. (2022). Miscanthus sinensis is as efficient as Miscanthus × giganteus for nitrogen recycling in spite of smaller nitrogen fluxes. Bioenergy Res. 15 (2), 686–702. doi: 10.1007/s12155-022-10408-2
Liu, Y., Zhang, X., Tran, H., Shan, L., Kim, J., Childs, K., et al. (2015). Assessment of drought tolerance of 49 switchgrass (Panicum virgatum) genotypes using physiological and morphological parameters. Biotechnol. Biofuels. BioMed. Cent. 8 (1), 1–18. doi: 10.1186/s13068-015-0342-8
Liu, J. C., Temme, A. A., Cornwell, W. K., van Logtestijn, R. S. P., Aerts, R., Cornelissen, J. H. C. (2016). Does plant size affect growth responses to water availability at glacial, modern and future CO2 concentrations? Ecol. Res. 31 (2), 213–227. doi: 10.1007/s11284-015-1330-y
Lovell, J. T., Shakirov, E. V., Schwartz, S., Lowry, D. B., Aspinwall, M. J., Taylor, S. H., et al. (2016). Promises and challenges of eco-physiological genomics in the field: Tests of drought responses in switchgrass. Plant Physiol. 172 (2), 734–748. doi: 10.1104/pp.16.00545
Mann, J. J., Barney, J. N., Kyser, G. B., DiTomaso, J. M. (2013). Root system dynamics of Miscanthus × giganteus and Panicum virgatum in response to rainfed and irrigated conditions in California. Bioenergy Res. 6 (2), 678–687. doi: 10.1007/s12155-012-9287-y
Massey, J., Antonangelo, J., Zhang, H. (2020). Nutrient dynamics in switchgrass as a function of time. Agronomy 10 (7), 940. doi: 10.3390/AGRONOMY10070940
McCormick, A. J., Cramer, M. D., Watt, D. A. (2006). Sink strength regulates photosynthesis in sugarcane. New Phytol. 171 (4), 759–770. doi: 10.1111/j.1469-8137.2006.01785.x
McCormick, A. J., Cramer, M. D., Watt, D. A. (2008). Regulation of photosynthesis by sugars in sugarcane leaves. J. Plant Physiol. Urban Fischer 165 (17), 1817–1829. doi: 10.1016/J.JPLPH.2008.01.008
McCormick, A. J., Watt, D. A., Cramer, M. D. (2009). Supply and demand: Sink regulation of sugar accumulation in sugarcane. J. Exp. Bot. 60 (2), 357–364. doi: 10.1093/jxb/ern310
Mocko, K., Jones, C. S. (2021). ) do seedlings of larger geophytic species outperform smaller ones when challenged by drought? Am. J. Bot. 108 (2), 320–333. doi: 10.1002/ajb2.1612
Moy, A., Le, S., Verhoeven, A. (2015). Different strategies for photoprotection during autumn senescence in maple and oak. Physiologia Plantarum 155 (2), 205–216. doi: 10.1111/ppl.12331
Noodén, L. D., Guiamét, J. J., John, I. (1997). Senescence mechanisms. Physiologia Plantarum. 101 (4), 746–753. doi: 10.1111/J.1399-3054.1997.TB01059.X
Palmer, N. A., Donze-Reiner, T., Horvath, D., Heng-Moss, T., Waters, B., Tobias, C., et al. (2015). Switchgrass (Panicum virgatum l) flag leaf transcriptomes reveal molecular signatures of leaf development, senescence, and mineral dynamics. Funct. Integr. Genomics 15 (1), 1–16. doi: 10.1007/s10142-014-0393-0
Palmer, N. A., Chowda-Reddy, R. V., Muhle, A. A., Tatineni, S., Yuen, G., Edmé, S. J., et al. (2019). Transcriptome divergence during leaf development in two contrasting switchgrass (Panicum virgatum l.) cultivars. PloS One 14 (9):e0218352. doi: 10.1371/journal.pone.0222080
Parrish, D. J., Fike, J. H. (2005). The biology and agronomy of switchgrass for biofuels. Crit. Rev. Plant Sci. 24, 423–459. doi: 10.1080/07352680500316433
Reichstein, M., Falge, E., Baldocchi, D., Papale, D., Aubinet, M., Berbigier, P., et al. (2005). On the separation of net ecosystem exchange into assimilation and ecosystem respiration: review and improved algorithm. Global Change Biol. John Wiley Sons Ltd 11 (9), 1424–1439. doi: 10.1111/J.1365-2486.2005.001002.X
Rodrigues, J., Inzé, D., Nelissen, H., Saibo, N. J. M. (2019). Source–sink regulation in crops under water deficit. Trends Plant Sci. 24 (7), 652–663. doi: 10.1016/j.tplants.2019.04.005
Ruiz-Vera, U. M., De Souza, A. P., Ament, M. R., Gleadow, R. M., Ort, D. R.. (2021). High sink strength prevents photosynthetic down-regulation in cassava grown at elevated CO2 concentration. J. Exp. Bot. 72 (2), 542–560. doi: 10.1093/jxb/eraa459
Rusinowski, S., Krzyżak, J., Clifton-Brown, J., Jensen, E., Mos, M., Webster, R., et al. (2019). New miscanthus hybrids cultivated at a polish metal-contaminated site demonstrate high stomatal regulation and reduced shoot Pb and cd concentrations. Environ. pollut. 252, 1377–1387. doi: 10.1016/j.envpol.2019.06.062
Sanford, G. R., Oates, L. G., Jasrotia, P., Thelen, K. D., Robertson, G. P., Jackson, R. D., et al. (2016). Comparative productivity of alternative cellulosic bioenergy cropping systems in the north central USA. Agriculture Ecosyst. Environment. Elsevier 216, 344–355. doi: 10.1016/j.agee.2015.10.018
Santoro, N., Cantu, S. L., Tornqvist, C.-E., Falbel, T. G., Bolivar, J. L., Patterson, S. E., et al. (2010). A high-throughput platform for screening milligram quantities of plant biomass for lignocellulose digestibility. Bioenergy Res. 3, 93–102. doi: 10.1007/S12155-009-9074-6
Sekhon, R. S., Breitzman, M. W., Silva, R. R., Santoro, N., Rooney, W. L., De Leon, N., et al. (2016). Stover composition in maize and sorghum reveals remarkable genetic variation and plasticity for carbohydrate accumulation. Front. Plant Sci. 7, 822. doi: 10.3389/FPLS.2016.00822
Smith, A. M., Stitt, M. (2007). Coordination of carbon supply and plant growth. Plant Cell Environ. 30 (9), 1126–1149. doi: 10.1111/j.1365-3040.2007.01708.x
Stavridou, E., Webster, R. J., Robson, P. R. H. (2020). The effects of moderate and severe salinity on composition and physiology in the biomass crop Miscanthus × giganteus. Plants 9 (10), 1–27. doi: 10.3390/plants9101266
Tang, Y., Wen, X., Lu, C. (2005). Differential changes in degradation of chlorophyll-protein complexes of photosystem I and photosystem II during flag leaf senescence of ric. Plant Physiol. Biochem. 43 (2), 193–201. doi: 10.1016/j.plaphy.2004.12.009
Taylor, S. H., Lowry, D. B., Aspinwall, M. J., Bonnette, J. E., Fay, P. A., Juenger, T. E., et al. (2016). QTL and drought effects on leaf physiology in lowland Panicum virgatum. Bioenergy Res. 9 (4), 1241–1259. doi: 10.1007/s12155-016-9768-5
Tejera, M., Boersma, N. N., Archontoulis, S. V, Miguez, F. E., VanLoocke, A., Heaton, E. A. (2022). Photosynthetic decline in aging perennial grass is not fully explained by leaf nitroge. J. Exp. Bot 73 (22), 7582–7595. doi: 10.1093/jxb/erac382
Tejera, M. D., Miguez, F. E., Heaton, E. A. (2021). The older plant gets the sun: Age-related changes in Miscanthus × giganteus phenology. GCB Bioenergy 13 (1), 4–20. doi: 10.1111/gcbb.12745
Van Heerden, P. D. R., Donaldson, R. A., Watt, D. A., Singels, A. (2010). Biomass accumulation in sugarcane: Unravelling the factors underpinning reduced growth phenomena. J. Exp. Bot. 61 (11), 2877–2887. doi: 10.1093/jxb/erq144
Wang, N., Li, Q., Liu, X., Yi, S., Zhao, M., Sun, X., et al. (2021). Plant size plays an important role in plant responses to low water availability and defoliation in two woody leguminosae species. Front. Plant Sci. 12, 1–14. doi: 10.3389/fpls.2021.643143
Wingler, A., Roitsch, T. (2008). Metabolic regulation of leaf senescence: interactions of sugar signalling with biotic and abiotic stress responses. Plant Biol. 10, 50–62. doi: 10.1111/j.1438-8677.2008.00086.x
Wutzler, T., Lucas-Moffat, A., Migliavacca, M., Knauer, J., Sickel, K., Šigut, L., et al. (2018). Basic and extensible post-processing of eddy covariance flux data with REddyProc. Biogeosciences. 15 (16), 5015–5030. doi: 10.5194/BG-15-5015-2018
Yan, J., Zhu, C., Liu, W., Luo, F., Mi, J., Ren, Y., et al. (2015). High photosynthetic rate and water use efficiency of Miscanthus lutarioriparius characterize an energy crop in the semiarid temperate region. GCB Bioenergy 7 (2), 207–218. doi: 10.1111/gcbb.12118
Yang, J., Worley, E., Wang, M., Lahner, B., Salt, D. E., Saha, M.. (2009). Natural variation for nutrient use and remobilization efficiencies in switchgrass. Bioenergy Res. 2, 4. doi: 10.1007/S12155-009-9055-9
Yang, J., Preiser, A. L., Li, Z., Weise, S. E., Sharkey, T. D, et al. (2016). Triose phosphate use limitation of photosynthesis: short-term and long-term effects. Planta 243, 687–698. doi: 10.1007/s00425-015-2436-8
Keywords: photosyhthesis, storage carbohydrates, sink limitation, perennial grass, drought, circadian, source- sink- relationships, C4 photosynthesis
Citation: Tejera-Nieves M, Abraha M, Chen J, Hamilton SK, Robertson GP and Walker BJ (2023) Seasonal decline in leaf photosynthesis in perennial switchgrass explained by sink limitations and water deficit. Front. Plant Sci. 13:1023571. doi: 10.3389/fpls.2022.1023571
Received: 19 August 2022; Accepted: 07 December 2022;
Published: 04 January 2023.
Edited by:
Jiban Shrestha, Nepal Agricultural Research Council, NepalCopyright © 2023 Tejera-Nieves, Abraha, Chen, Hamilton, Robertson and Walker. This is an open-access article distributed under the terms of the Creative Commons Attribution License (CC BY). The use, distribution or reproduction in other forums is permitted, provided the original author(s) and the copyright owner(s) are credited and that the original publication in this journal is cited, in accordance with accepted academic practice. No use, distribution or reproduction is permitted which does not comply with these terms.
*Correspondence: Berkley James Walker, YmVya2xleUBtc3UuZWR1