- Department of Life Science (BK21 Program), Chung-Ang University, Seoul, South Korea
Abscisic acid (ABA) is a major phytohormone that plays important roles in stress responses, including regulation of gene expression and stomatal closure. Regulation of gene expression by transcription factors is a key cellular process for initiating defense responses to biotic and abiotic stresses. Here, using pepper (Capsicum annuum) leaves, we identified the MYB transcription factor CaDIM1 (Capsicum annuum Drought Induced MYB 1), which was highly induced by ABA and drought stress. CaDIM1 has an MYB domain in the N-terminal region and an acidic domain in the C-terminal region, which are responsible for recognition and transactivation of the target gene, respectively. Compared to control plants, CaDIM1-silenced pepper plants displayed ABA-insensitive and drought-sensitive phenotypes with reduced expression of stress-responsive genes. On the other hand, overexpression of CaDIM1 in Arabidopsis exhibited the opposite phenotypes of CaDIM1-silenced pepper plants, accompanied by enhanced ABA sensitivity and drought tolerance. Taken together, we demonstrate that CaDIM1 functions as a positive regulator of the drought-stress response via modulating ABA-mediated gene expression.
Introduction
Since plants are sessile organisms, they have developed mechanisms that facilitate adaptation to environmental stresses, such as high salinity, extreme temperatures and drought. Drought is a major environmental stress that causes osmotic stress to cells, damages plant tissues, and limits plant development and growth, ultimately reducing agricultural crop productivity (Zhu, 2002). Plants improve their drought tolerance by adjusting physiological and molecular processes, such as stomatal aperture, stress-related gene expression, and abscisic acid (ABA) biosynthesis (Lee and Luan, 2012). ABA is a key phytohormone that is involved in responding to abiotic stress, especially drought stress. Previous studies have elucidated ABA signaling core components and their interactors, which play a role upstream or downstream of ABA signaling core components (Umezawa et al., 2010; Ng et al., 2014; Yang et al., 2017; Kumar et al., 2019). Under drought stress, endogenous ABA is synthesized and accumulated in various plant tissues (Raghavendra et al., 2010). In the cell, ABA is perceived by ABA receptors, which transfer ABA signaling to downstream proteins by inhibiting protein phosphatases, leading to the activation of protein kinases (Lim et al., 2015). Activated protein kinases promote ion channel activation and gene expression via phosphorylation of the SLAC1 anion channel and transcription factors, respectively (Geiger et al., 2009; Lee et al., 2009; Geiger et al., 2010).
In the drought-stress condition, various transcription factors modulate stress-related genes via ABA-dependent or -independent pathways (Yoshida et al., 2014; Joshi et al., 2016). Transcription factors are classified into families based on their functional and structural features, especially their DNA-binding domains (Wingender et al., 2015). In response to drought stress, the bZIP, HD-ZIP, bHLH, NAC, AP2/ERF, and MYB families are activated or inactivated, and become involved in modulating the expression of stress-related genes (Yang et al., 2010). MYB transcription factors have highly conserved N-terminal MYB DNA-binding domain repeats, named R1, R2, and R3. The major MYB transcription factors belong to the R2R3-MYB subfamily, which participates in plant development, metabolic mechanisms, and stress responses (Dubos et al., 2010; Millard et al., 2019). Arabidopsis MYB transcription factors are involved in responding to drought stress via modulating stomatal opening and closure (Cominelli et al., 2008; Jung et al., 2008; Oh et al., 2011). For example, MYB60 expressed in Arabidopsis guard cells negatively modulated drought stress by regulating stomatal movement. The myb60 mutant enhanced drought tolerance by repressing stomatal opening (Cominelli et al., 2008), whereas MYB60-overexpressing plants showed hypersensitivity to drought stress (Oh et al., 2011). Moreover, overexpression of MYB44 increased ABA sensitivity and drought tolerance via enhancing stomatal closure (Jung et al., 2008). In addition to modulating stomatal movement, many MYB transcription factors facilitate drought tolerance by participating in ABA signaling. For example, MYB15, MYB37, and MYB96 modulate seed germination and root growth in response to ABA (Ding et al., 2009; Seo et al., 2009; Yu et al., 2016). However, in pepper plants, the biological function of MYB transcription factors in the drought-stress response has not yet been revealed.
In this study, we used RNA-seq analysis to isolate CaDIM1 (Capsicum annuum Drought-Induced Myb transcription factor 1) from pepper leaves that were subjected to drought stress. CaDIM1 expression was induced in response to drought and ABA treatments, and CaDIM1 localized in the nucleus. Moreover, we elucidated that the CaDIM1 C-terminal acidic domain contributes to transactivational activity. CaDIM1 knockdown in pepper and CaDIM1-OX Arabidopsis plants showed altered phenotypes under ABA and drought stress treatments. These results indicate that CaDIM1 modulates drought tolerance via enhancing ABA signaling.
Results
Molecular characterization of CaDIM1
To identify drought-induced transcription factors in pepper, we performed RNA-seq analysis with drought-treated pepper leaves, and then isolated the pepper CaDIM1 (Capsicum annuum Drought Induced Myb 1) gene corresponding to CA10g05760 and Capana10g000613 in the genomes of Capsicum annuum cv. CM334 (Kim et al., 2014) and cv. Zunla-1 (Qin et al., 2014), respectively. The CaDIM1 cDNA contains an open reading frame of a 966-bp nucleic acid and encodes 321 amino acid residues. The encoded protein has an isoelectric point of 6.85 and a molecular weight of 36.49 kD. Multiple sequence alignment analysis showed a high amino acid sequence identity (53–82.6%) and similarity (64.3–88.5%) between CaDIM1 and other plant species’ Myb proteins (Supplementary Figure S1). The phylogenetic tree shows that CaDIM1 and its homologous protein sequences are phylogenetically distant (Supplementary Figure S2). In particular, we found that the deduced amino acid sequences of CaDIM1 and its pepper paralog CaMYB102A share 61.0% identity and 69.4% similarity (Supplementary Figure S3A). CaDIM1 was identified in the drought-treated pepper leaves; therefore, we evaluated whether CaDIM1 is induced by abiotic stress treatment. To determine the expression pattern of CaDIM1 under various abiotic stress conditions, we performed qRT–PCR analysis using first and second pepper leaves after drought and ABA treatments (Figure 1A). Drought and ABA treatments induced CaDIM1 transcription in pepper leaves. Drought treatment induced CaDIM1 transcription after 2 h, with transcription reaching the maximum level at 6 h but decreasing at 12 h. On the other hand, ABA treatment induced CaDIM1 transcription after 2 h, with transcription reaching the maximum level at 12 h. As a paralog, CaMYB102A also showed similar expression patterns, but this gene reached a peak at 2 h after ABA treatment (Supplementary Figure S3B). These results suggest that CaDIM1 might be involved in abiotic stress signaling. To investigate the subcellular localization of the CaDIM1 protein, we fused the GFP reporter gene to the C-terminal of CaDIM1 under the 35S promoter (pro35S:CaDIM1-GFP), and transiently expressed GFP-fused proteins in the epidermal cells of N. benthamiana. CaDIM1-GFP protein localized in the nucleus and generated GFP signals that overlapped with DAPI staining signals (Figure 1B).
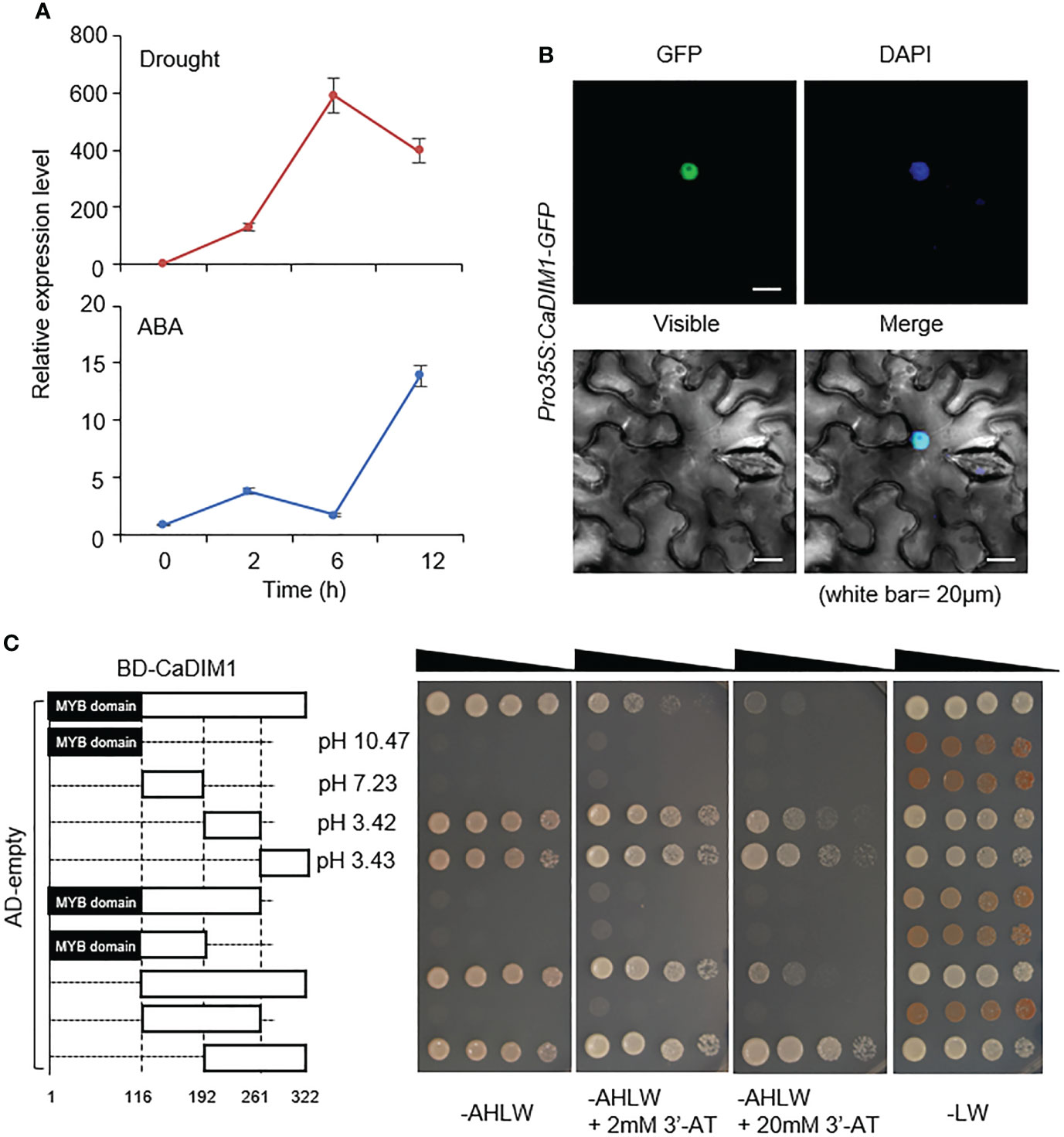
Figure 1 Molecular characterization of CaDIM1. (A) Expression levels of CaDIM1 in the pepper leaves after treatment with abscisic acid (ABA) (100 μM), or drought. The pepper Actin1 (CaACT1) was used as an internal control. (B) Subcellular localization of green fluorescent protein (GFP)-tagged CaDIM1 proteins. Expression of GFP in Nicotiana benthamiana leaves was detected under a confocal microscope. 4′,6-Diamidino-2-phenylindole (DAPI) was used as a nuclear marker. The white scale bars represent 20 μm. (C) Transactivation of the GAL4-responsive promoter by full-length and truncated forms of CaDIM1 fused to the GAL4 DNA-binding domain in the yeast strain AH109. Yeast harboring each construct were grown on selection media (SC-adenine-histidine-leucine-tryptophan [AHLW] SC-leucine-tryptophan [LW]). Representative images were taken at 5 days after incubation. BD, DNA-binding domain; AD, activation domain.
CaDIM1 has an MYB domain in the N-terminal region and an acidic domain in the C-terminal region. To test whether CaDIM1 has transactivational activity and functions as a transcriptional activator, we conducted a transactivation assay in yeast using GAL4-responsive reporter system. The full-length CaDIM1 and nine truncated fragments were cloned into the pGBKT7, carrying the Gal4 DNA-binding domain, and then transformed into yeast strain AH109 with the pGADT7 (Figure 1C). We determined the ability of each fragment to activate transcription from UAS (upstream activation sequence), based on yeast growth in selection medium lacking Ade, Trp, His, and Leu. The full length of CaDIM1-transformed yeast grew on a selection medium, suggesting that CaDIM1 presumably functions as a transcription factor. However, truncated CaDIM1 containing the MYB domain (amino acids 1-192) had no auto activation activity. On the other hand, CaDIM1 containing the acidic domain (amino acids 193-322) showed auto activation activity in the selection medium, demonstrating that the acidic domain has control of transcriptional activity.
Reduced tolerance of CaDIM1-silenced pepper plants to drought stress
To investigate the biological role of CaDIM1 in drought-stress conditions, we performed a virus-induced gene silencing (VIGS) analysis (Figure 2). We generated two VIGS constructs: CaDIM1-1 (378–677) and CaDIM1-2 (667–966). To confirm the efficiency of the gene silencing, we performed an RT–PCR analysis, which revealed that the expression levels of CaDIM1 were lower in CaDIM1-silenced pepper plants (TRV2:CaDIM1-1, TRV2:CaDIM1-2) than in control plants (TRV2:00) (Figure 2A). To investigate whether the reduced expression of CaDIM1 alters drought responses, control and CaDIM1-silenced pepper plants were subjected to drought stress (Figure 2). There were no phenotypic differences between CaDIM1-silenced and control pepper plants under normal conditions (Figure 2B; upper panel). However, after drought treatment for 14 days followed by rewatering for 2 days, CaDIM1-silenced pepper plants showed more wilted phenotypes than control plants (Figure 2B; middle and lower panels). The survival rate of control plants was higher (66.67%) than that of CaDIM1-silenced pepper plants (27.78% and 38.89%, Figure 2C). To confirm whether the drought-sensitive phenotype of CaDIM1-silenced pepper plants was due to differences in water-retention capacity, transpirational water loss was evaluated using detached leaves from control and CaDIM1-silenced pepper plants (Figure 2D). The transpirational water loss was higher in CaDIM1-silenced pepper leaves than in control leaves. Previous studies have revealed that altered survival rates under drought stress are associated with the expression levels of stress-related genes (Joo et al., 2019). Therefore, we investigated whether reduced expression of CaDIM1 have an effect on the induction of stress-related genes (Figure 2E). To do so, we performed qRT–PCR analysis of stress-related genes in pepper leaves from plants treated with drought stress. The expression levels of these genes, including CaOSR1, CaRAB18, and CaNCED3, were lower in CaDIM1-silenced plants than in control pepper plants.
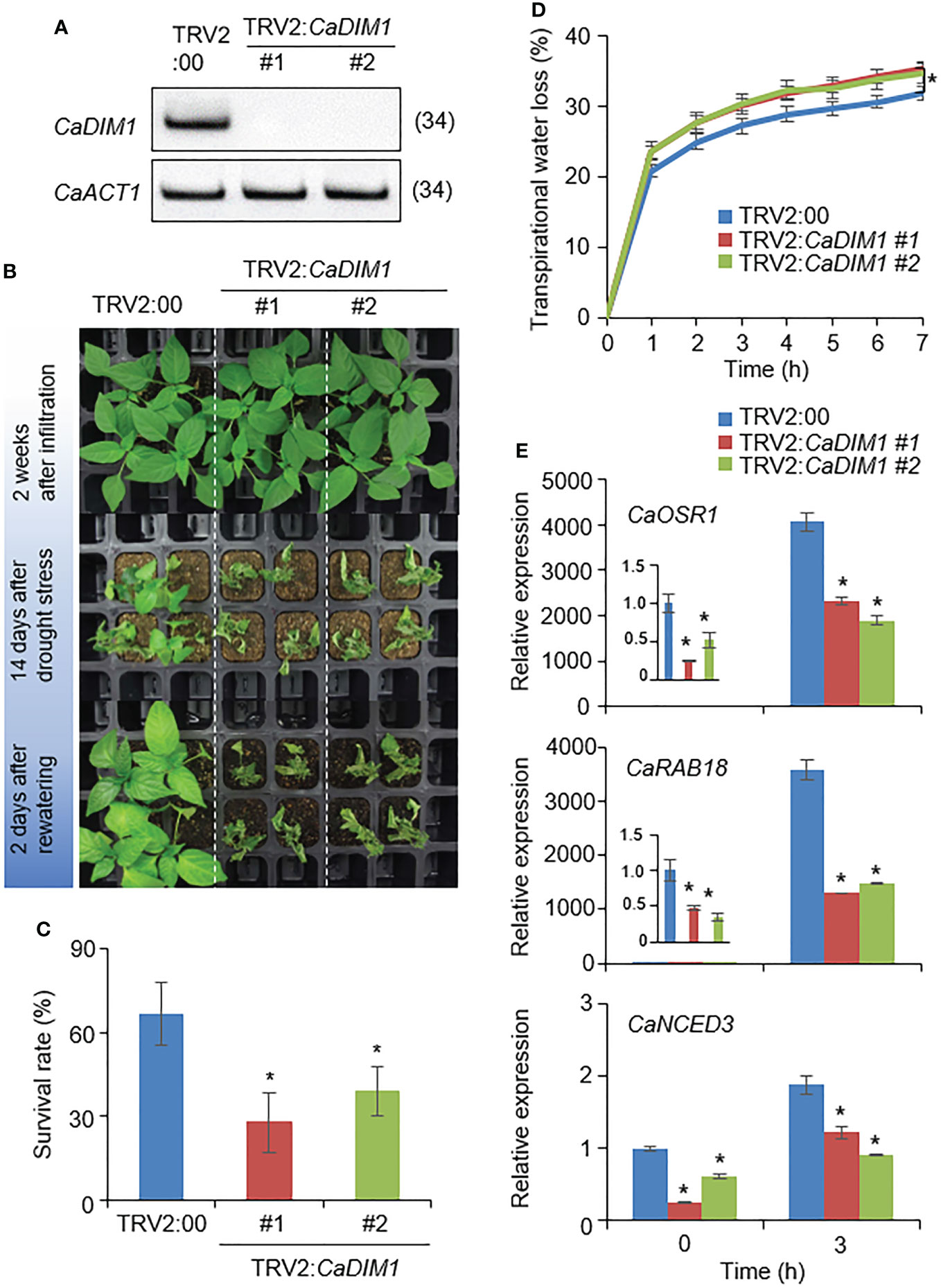
Figure 2 Reduced tolerance of CaDIM1-silenced pepper plants to drought stress. (A) RT-PCR assay of CaDIM1 expression in CaDIM1-silenced pepper plants and control plants (TRV2:00). The pepper Actin1 (CaACT1) gene was used as an internal control. (B) Drought sensitivity of control (TRV2:00) and CaDIM1-silenced pepper (TRV2:CaDIM1) plants. Four-week-old plants of each line were re-watered for 2 days after drought stress was imposed for 14 days by withholding watering. Representative images were taken before (top) and after (middle) drought, as well as after 2 days of re-watering (bottom). (C) Survival rates of TRV2:00 and TRV2:CaDIM1 pepper plants after 2 days of re-watering. Data represent the mean ± standard error of three independent experiments; in each of these experiments, 20 plants were evaluated. (D) Transpirational water loss from the leaves of TRV2:00 and TRV2:CaDIM1 plants. Leaves from plants of each line were detached, and the fresh weights of leaves were measured every hour for 7 h. Data represent the mean ± standard error of three independent experiments; in each of these experiments, 10 plants were evaluated. (E) Quantitative real-time polymerase chain reaction analysis of stress-responsive genes in the leaves of CaDIM1-silenced pepper plants (TRV2:CaDIM1) and control plants (TRV2:00). Control and CaDIM1-silenced pepper plants were subjected to dehydration for 3 h after detachment. The relative expression levels (ΔΔCT) of each gene were normalized to the geometric mean of the pepper Actin1 (CaACT1) gene, which was used as an internal control. Asterisks indicate significant differences between TRV2:00 and TRV2:CaDIM1 plants (Student’s t-test; *P < 0.05).
Reduced ABA sensitivity of CaDIM1-silenced pepper plants
Previous studies have suggested that differences in drought stress and transpiration, which ultimately affect survival rates, are caused by differences in ABA sensitivity (Lim et al., 2017). To determine ABA sensitivity, we measured leaf temperature and stomatal aperture with or without ABA treatment (Figure 3). There were no significant differences in leaf temperature or stomatal aperture between the CaDIM1-silenced pepper and control plants in the absence of ABA. ABA treated leaves exhibited higher temperatures and reduced stomatal aperture in both CaDIM1-silenced pepper and control plants than without ABA treatment. However, CaDIM1-silenced pepper plants exhibited low leaf temperatures (Figures 3A, B) and large stomatal aperture (Figures 3C, D) in comparison to control plants. These results reveal that CaDIM1 plays a positive role in drought-stress conditions via modulating ABA sensitivity.
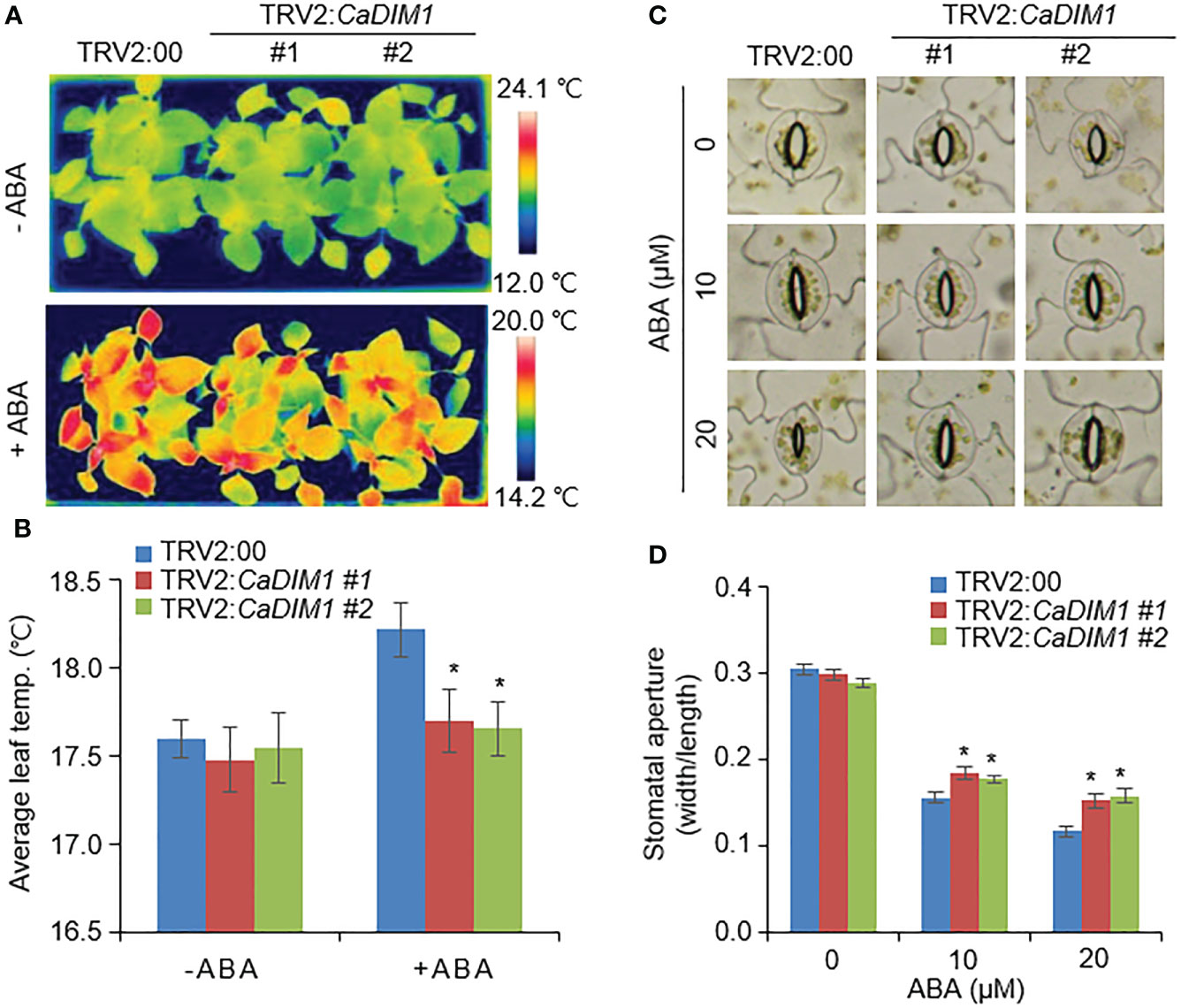
Figure 3 Reduction of ABA-mediated stomatal closure in the leaves of CaDIM1-silenced pepper plants. (A, B) Leaf temperatures of TRV2:00 and TRV2:CaDIM1 plants before and after exposure to ABA. Leaves from the plants of each line were sprayed with 0 and 100 μM ABA. At 3 h after treatment, representative thermographic images were taken (A) and leaf temperature was measured (B). (C, D) ABA-induced stomatal closure in TRV2:00 and TRV2:CaDIM1 plants. Leaf peels were incubated with 0, 10, and 20 μM ABA. Representative images were taken (C) and the stomatal aperture of each line was measured 3 h after treatment (D). All data represent the mean ± standard error of three independent experiments. Asterisks indicate significant differences between TRV2:00 and TRV2:CaDIM1 plants (Student’s t-test; *P < 0.05).
Enhanced tolerance of CaDIM1-OX plants to drought stress
To investigate additional biological functions of CaDIM1, we generated Arabidopsis transgenic plants that overexpressed CaDIM1 and obtained four independent T3 homozygous transgenic lines (CaDIM1-OX #1, #2, #3, #4) that showed high expression of CaDIM1 (Figure 4A). To verify whether the enhanced expression of CaDIM1 alters drought responses, both wild-type and CaDIM1-OX plants were subjected to drought stress (Figure 2B). All plants showed similar phenotypes under normal growth conditions (Figure 4B, upper panel). However, after 12 days of drought stress followed by 1 day of rewatering, CaDIM1-OX plants displayed less wilted phenotypes than wild-type plants (Figure 4B, middle and lower panels). Only 37.5% of wild-type plants resumed growth, while approximately 66–91% of CaDIM1-OX plants survived (Figure 4C). Moreover, CaDIM1-OX plants showed lower transpirational water loss than wild-type plants (Figure 4D), indicating that the enhanced drought tolerance might be attributed to altered water-retention capacity.
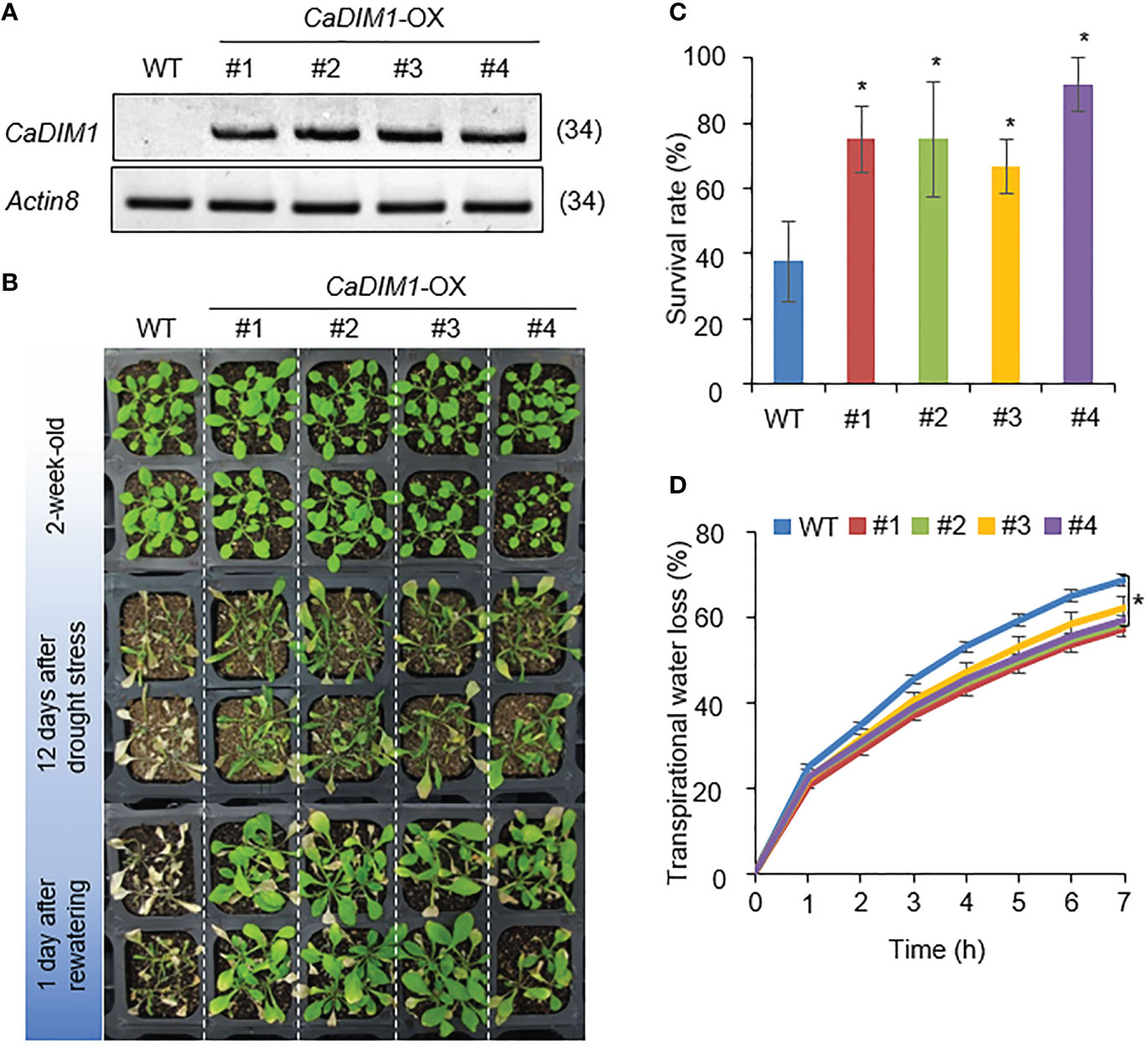
Figure 4 Enhanced tolerance of CaDIM1-overexpressing (OX) transgenic Arabidopsis plants to drought stress. (A) RT-PCR analysis of wild-type (WT) and CaDIM1-overexpressing (OX) transgenic Arabidopsis plants. The Arabidopsis Actin8 gene was used as an internal control. (B) Drought-tolerant phenotype of CaDIM1-OX transgenic plants. Two-week-old wild-type (WT) and transgenic plants were subjected to drought stress by withholding watering for 12 days and subsequently re-watering for 1 day. The survival rate of each line was measured after re-watering. Data represent the mean ± standard error of three independent experiments; in each of these experiments, 20 plants were evaluated. (C) Survival rates of wild-type and transgenic plants after 1 day of re-watering. Data represent the mean ± standard error of three independent experiments; in each of these experiments, 20 plants were evaluated. (D) Transpirational water loss from the leaves of wild-type and transgenic plants at different time points after leaf detachment. Asterisks indicate significant differences between wild-type and CaDIM1-OX plants (Student’s t-test; *P < 0.05).
Enhanced ABA sensitivity of CaDIM1-OX plants
In general, drought tolerance in plants is associated with ABA sensitivity; therefore, we measured the leaf temperature and stomatal aperture in wild-type and CaDIM1-OX plants with or without ABA treatment (Figures 5A–D). Under normal conditions, leaf temperature and stomatal aperture were similar in both plants. However, under ABA treatment, CaDIM1-OX plants showed high leaf temperatures and reduced stomatal aperture relative to wild-type plants (Figures 5A–D). These results suggest that CaDIM1 positively modulates drought stress responses by enhancing ABA sensitivity.
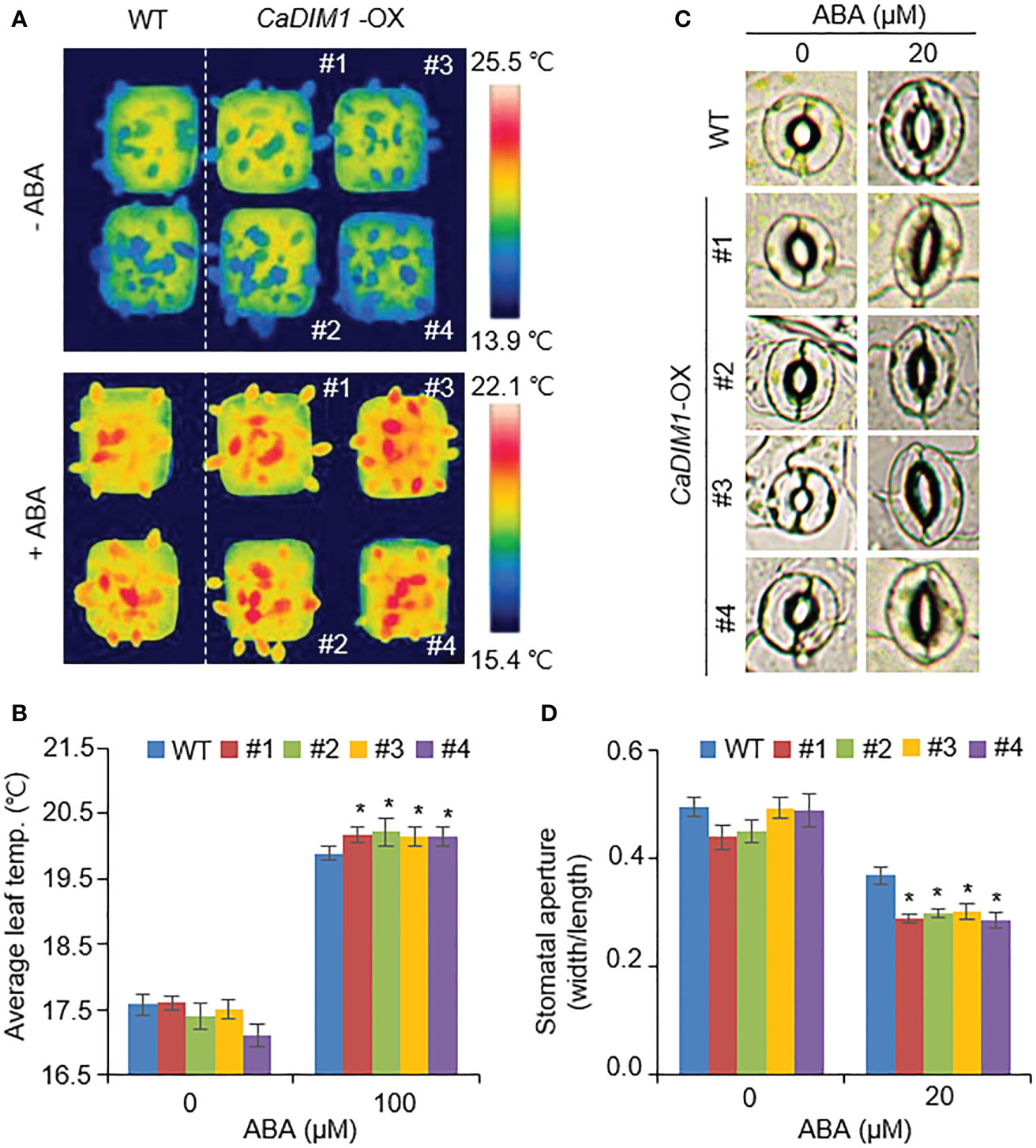
Figure 5 Enhanced ABA sensitivity of CaDIM1-overexpressing (OX) transgenic Arabidopsis plants. (A, B) Leaf temperatures of wild-type and CaDIM1-OX transgenic plants after exposure to 100 μM abscisic acid (ABA). At 6 h after treatment, representative thermographic images were taken (A) and leaf temperature was measured (B). (C, D) Stomatal aperture in wild-type and CaDIM1-OX plants treated with ABA. Leaf peels were harvested from 3-week-old plants of each line and incubated in stomatal opening solution containing the indicated concentrations of ABA. Representative images were taken under a microscope, (C) and the stomatal aperture was measured (D). All data represent the mean ± standard error of three independent experiments. Asterisks indicate significant differences between wild-type and CaDIM1-OX plants (Student’s t-test; *P < 0.05).
ABA regulates seed germination and post-germinative growth. Therefore, we measured seed germination rates, primary root growth, and green cotyledon rate in wild-type and CaDIM1-OX plants (Figure 6). Germination rates of wild-type and CaDIM1-OX seeds showed no significant differences without ABA. However, when grown on an ABA-treated medium, CaDIM1-OX seed germination rates were lower than those of wild-type seeds (Figure 6A). In addition, we estimated primary root growth and green cotyledon rates in response to ABA (Figures 6B–E). At 10 days after plating on an ABA-treated medium, primary root growth of CaDIM1-OX plants was severely reduced compared with that of wild-type plants (Figures 6B, C). Consistent with primary root growth, cotyledon greening rate was lower in CaDIM1-OX plants than in wild-type plants (Figures 6D, E). These results suggest that enhanced CaDIM1 expression contributed to ABA hypersensitivity in germination and post-germination growth.
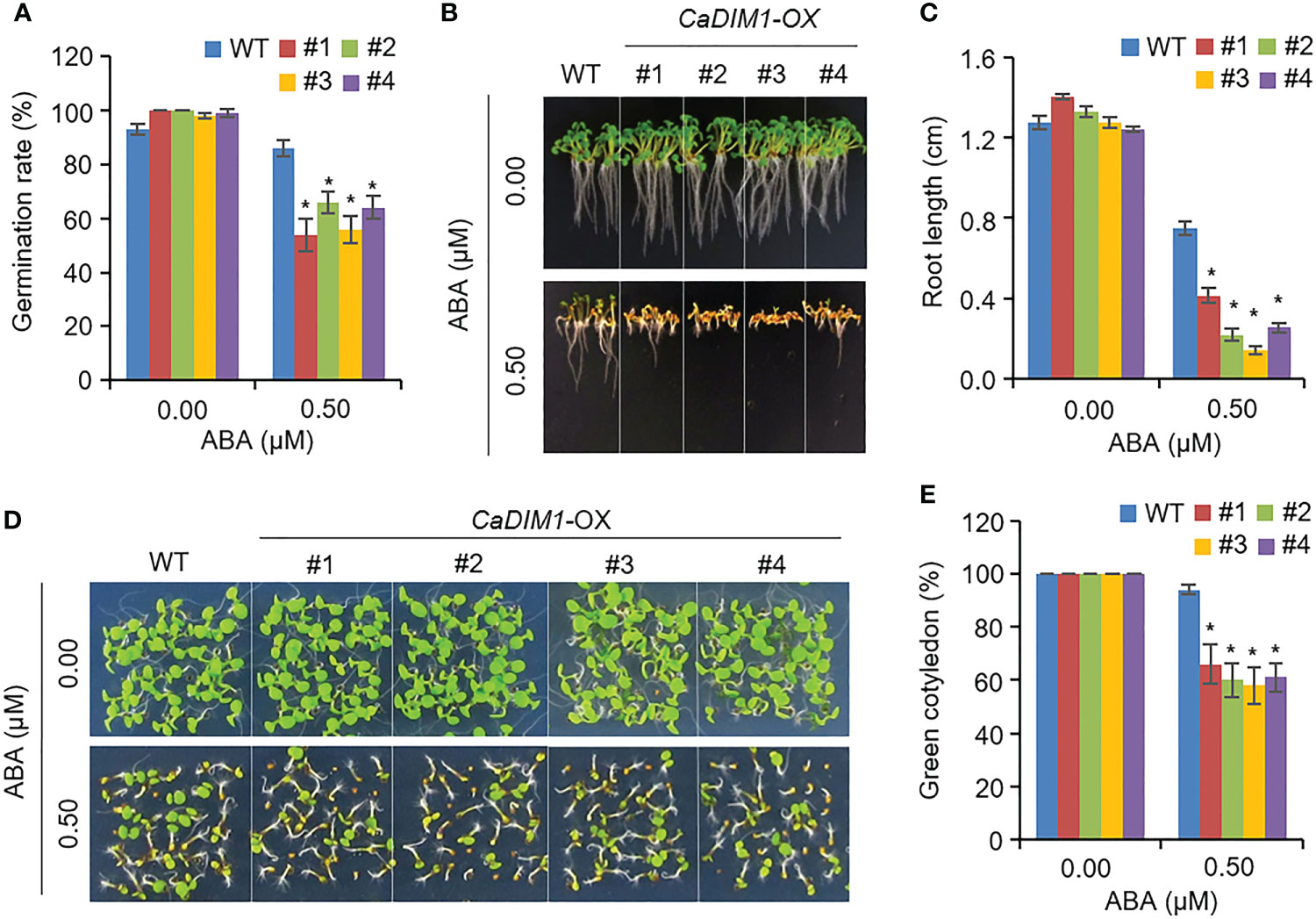
Figure 6 Increased sensitivity of CaDIM1-overexpressing (OX) transgenic Arabidopsis plants to ABA during germination and seedling growth. (A) Seed germination of wild-type (WT) and transgenic lines in response to ABA. Seeds were germinated on 0.5x Murashige and Skoog (MS) agar plates containing 0.0 μM and 0.5 μM of ABA. (B, C) Root elongation of wild-type and transgenic plants in response to 0.0 μM and 0.75 μM ABA. The representative images were taken (B) and the root length of each plant was measured 6 days after plating (C). Data represent the mean ± standard error of three independent experiments. (D, E) Growth of wild-type and transgenic line seedlings on 0.5x MS agar plates containing 0.0 μM and 0.5 μM ABA. Representative images were taken (D) and quantification of green cotyledons was conducted 7 days after plating (E). Data represent the mean ± standard error of four independent experiments; in each of these experiments, 36 seeds were evaluated. Asterisks indicate significant differences between wild-type and CaDIM1-OX plants (Student’s t-test; *P < 0.05).
Discussion
Plants inevitably endure various environmental stresses. Drought is major stress that causes osmotic stress to plant cells, ultimately limiting growth and development. In order to survive under stressful conditions, plants have evolved defense mechanisms, controlling physiological and biochemical responses to the type of stress. Regulation of gene expression is one of the most well-known drought-stress defense mechanisms, and is modulated by transcription factors (Xiong and Zhu, 2002). The present study showed that CaDIM1 is one component of the pepper plant drought-stress response. Many regulatory proteins, including protein kinases, phosphatases, and transcription factors, have been characterized as key factors of drought stress signaling, and the involvement of these proteins in the drought-stress response is well established (Singh and Laxmi, 2015; Sah et al., 2016; Zhu, 2016). Many studies have demonstrated that transcription factors activate or repress target genes, whose expression or repression is key in response to drought stress (Dong and Liu, 2010; Yoshida et al., 2014; Xie et al., 2019). For example, the Arabidopsis ABI3 transcription factor activates ABI5 expression, leading to drought-stress tolerance (Lopez-Molina et al., 2002). In general, DNA-binding domains of transcription factors have specific sequences and adhere to the promoter of target genes (Mitchell and Tjian, 1989; Latchman, 1990). Moreover, transcription factors contain a regulatory domain, which is rich in specific amino acids, including the acidic amino acids glutamine or proline (Licausi et al., 2013). MYB transcription factors contain two distinct domains in the N-terminal and C-terminal regions. The N-terminal domain is a conserved MYB domain for DNA binding, while the C-terminal domain is a diverse regulatory domain for activation or repression of target genes (Ambawat et al., 2013). CaDIM1 has an MYB domain and an acidic domain, which play roles in DNA binding and transactivation, respectively. However, a repression motif was not found in CaDIM1. These results indicate that CaDIM1 plays a role as a transcription activator in pepper plants.
The multiple sequence alignment with other plants showed that CaDIM1 has a conserved region in diverse plant species, and phylogenetic tree analysis showed that CaDIM1 belongs to the group 11 MYB transcription factors (Supplementary Figures S1, S2). These results suggest that CaDIM1 might have a similar function to that of other MYB transcription factors. In plants, MYB transcription factors play various roles, including UV-B tolerance and signaling, drought stress responses, ABA signaling, salt tolerance, and extreme temperature tolerance (Oh et al., 2001; Abe et al., 2003; Cominelli et al., 2005; Agarwal et al., 2006; Reyes and Chua, 2007; Jenkins, 2009; Sun et al., 2020; Yang et al., 2020). CaDIM1 expression levels were induced in the drought-stress and ABA treatments. Generally, under drought-stress conditions, plants initiate defense processes such as biosynthesis of ABA and induction of defense-related genes (Sato et al., 2018; Takahashi et al., 2020). Due to the very low efficiency of transformation in pepper, an overexpression analysis in Arabidopsis and a VIGS assay in pepper were used for genetic analysis of CaDIM1. After the drought-stress treatment, CaDIM1-silenced pepper plants showed drought-sensitive phenotypes, which were affected by low water-retention capacity. Conversely, CaDIM1-OX plants showed a drought-tolerant phenotype with ABA hypersensitivity, which led to altered stomatal aperture. These genetic analyses indicate that altered expression of CaDIM1 affects stomatal pore size, leading to regulation of water consumption and unique drought responses.
Drought-stress tolerance is related to expression levels of ABA- or stress-responsive genes (Fujita et al., 2011; Lu et al., 2019; Joo et al., 2020). ABA biosynthesis is also a key factor in determining tolerance or sensitivity (Iuchi et al., 2001). Moreover, accumulation of ABA in guard cells triggers stomatal closure, which inhibits transpirational water loss (Schroeder et al., 2001; Lim et al., 2015). In this study, the downstream target genes of CaDIM1 were not identified; however, the expression levels stress- or ABA-responsive genes, such as CaOSR1, CaRAB18, and CaNCED3 were significantly lower in CaDIM1-silenced pepper plants than in the control pepper plants. This result demonstrates that CaDIM1 is presumably involved in expression of these genes, directly or indirectly.
In conclusion, CaDIM1 contains an acidic domain for transactivation and is localized in the nucleus, where it may play a role as a transcriptional modulator. Silencing CaDIM1 in pepper induced drought hypersensitivity, whereas overexpression of CaDIM1 in Arabidopsis led to drought tolerance with ABA hypersensitivity. Taken together, we demonstrated that CaDIM1 positively regulates drought-stress responses via modulating ABA signaling. However, we were unable to identify direct target genes and interacting partners that modulate CaDIM1 activity. Thus, further study should focus on identifying CaDIM1 direct target genes and their interacting partners, and clarifying the signaling pathway through which CaDIM1 regulates drought tolerance.
Materials and methods
Plant materials
Pepper (Capsicum annuum L., cv. Nockwang), Arabidopsis (Arabidopsis thaliana ecotype Col-0), and tobacco (Nicotiana benthamiana) seeds were sown in a mix of loam soil, sand, and compost soil (1:1:1, volume). Plants were placed in a growth chamber at 24 ± 1°C with a 16 h/8 h (light/dark) cycle. Arabidopsis seeds were germinated on MS salt with microagar (Duchefa Biochemie, Haarlem, Netherlands) and 1% sucrose.
ABA and drought treatments
To analyze expression patterns of CaDIM1 in pepper plants, six-leaf-stage pepper plants were treated with ABA (100 μM) or drought, as described previously (Lim et al., 2017). Pepper leaves were harvested at various time points after each treatment and were subjected to qRT–PCR assay.
To analyze drought tolerance, 4-week-old pepper and 2-week-old Arabidopsis plants were randomly organized and subjected to drought treatment by withholding watering for 14 days and 12 days, respectively. After plants were rewatered for 2 days and 1 day, respectively, the survival rate was measured. To determine drought tolerance in a quantitative manner, transpirational water loss was measured. Leaves were detached from pepper and Arabidopsis plants, and the fresh weight was measured at the various time points. The experiments were repeated three times.
Subcellular localization analysis
To determine the subcellular location of CaDIM1, the coding region of CaDIM1 without the stop codon was inserted to the p326GFP vector (green fluorescent protein (GFP)-fused binary vector). Green fluorescent protein (GFP)-tagged CaDIM1 were expressed in leaves of N. benthamiana via agroinfiltration. A GV3101 strain of A. tumefaciens carrying the GFP-tagged CaDIM1 construct with p19 was infiltrated to N. benthamiana leaves. The GFP and DAPI signals were detected via a confocal microscope.
Transactivation assay
A transactivation assay was performed as described previously (Lim et al., 2017). The cDNA fragments of CaDIM1 were subcloned into the pGBKT7 vector, and co-transformed into yeast strain AH109 using the lithium acetate–mediated transformation method (Ito et al., 1983). Transformant candidates were selected on SC-Leu-Trp media. Each yeast was spotted onto SC-Leu-Trp media for quantitative control or SC-Ade-His-Leu-Trp media for selection. At 5 days after incubation, the images of colony were taken.
Virus-induced gene silencing
Virus-induced gene silencing (VIGS) was conducted to knock-down CaDIM1 in pepper plants as described previously (Lim et al., 2017). Using the VIGS tool (http://vigs.solgenomics.net), two 300-bp fragments of the CaDIM1 coding sequence, CaDIM1-1 (378–677) and CaDIM1-2 (667–966) with a target region score of above 99%, were designed to avoid off-target of silencing; each region was subsequently amplified by PCR. The CaDIM1 gene fragments were inserted into the pTRV2 vector and introduced into A. tumefaciens strain GV3101 via electroporation. A GV3101 containing pTRV1, pTRV2:00, pTRV2:CaDIM1-1, and pTRV2:CaDIM1-2 was infiltrated to pepper cotyledons.
Generation of transgenic Arabidopsis plants
To generate CaDIM1 transgenic plants in Arabidopsis, the p326GFP vector containing the coding region for CaDIM1 without the stop codon was used. The GV3101 strain of A. tumefaciens containing the 35S:CaDIM1-GFP construct was inoculated into Arabidopsis using the floral dip method (Clough and Bent, 1998). All transgenic plants were generated on the Col-0 background. To select CaDIM1-OX plants, seeds were harvested from the transgenic plants and sown on MS media containing 25 μg·mL-1 phosphinothricin.
Stomatal aperture bioassay and thermal imaging
Stomatal pore size measurement was performed as previously described (Lim et al., 2017). Briefly, leaf peels were placed in stomatal opening solution (SOS: 10 mM MES-KOH, 50 mM KCl, and 10 mM CaCl2) under the light conditions for 3 h. After incubation for 3 h, stomata closing was induced by 10 μM and 20 μM of ABA. After an additional 3 h, stomatal pore sizes were measured by microscope.
To estimate leaf temperature, first and second leaves of pepper and 3-week-old Arabidopsis plants were used. Absence or presence of 100 μM ABA, images were taken by an infrared camera (T420; FLIR systems, Wilsonville, OR, USA).
Data availability statement
The original contributions presented in this study are included in the article/Supplementary Material and the data presented in this study are deposited in the NCBI repository, accession number OP382712. Further inquiries can be directed to the corresponding author.
Author contributions
JL, CW, SL: conceptualization. JL and CW: methodology. JL and CW: data analysis. CW and SL: writing. All authors contributed to the article and approved the submitted version.
Funding
This work was supported by a grant from the Agriculture & Technology Development (Project No. PJ01652101), and the National Research Foundation of Korea (NRF) grant funded by the Korea Government (MSIT) (2021R1A2C2006338), Rural Development Administration, Republic of Korea.
Conflict of interest
The authors declare that the research was conducted in the absence of any commercial or financial relationships that could be construed as a potential conflict of interest.
Publisher’s note
All claims expressed in this article are solely those of the authors and do not necessarily represent those of their affiliated organizations, or those of the publisher, the editors and the reviewers. Any product that may be evaluated in this article, or claim that may be made by its manufacturer, is not guaranteed or endorsed by the publisher.
Supplementary material
The Supplementary Material for this article can be found online at: https://www.frontiersin.org/articles/10.3389/fpls.2022.1028392/full#supplementary-material
Supplementary Figure 1 | Alignment of the deduced CaDIM1 amino acid sequence with those of Solanum lycopersicum (accession no. XP_004248359.1), Solanum tuberosum (accession no. XP_006352596.1), Nicotiana tabacum (accession no. XP_016436111.1), and Arabidopsis thaliana (accession no. NP_567626.1) proteins. Identical amino acid residues are highlighted in black. Multiple alignment of the CaDIM1 protein sequence and its homologous proteins was performed using ClustalW2.
Supplementary Figure 2 | Phylogenetic tree analysis of the CaDIM1protein. A BLAST search was performed by using the deduced CaDIM1 and CaMYB102A amino acid sequences with highest similarity gathered from Arabidopsis. Multiple sequence alignment was performed using Clustal Omega (http://www.ebi.ac.uk/Tools/msa/clustalo/) with the default settings. The phylogenetic tree was drawn using the neighbor-joining method with MEGA software (version 7.0). Bootstrap values were calculated from 1,000 bootstrap replications and are indicated at each branch point. The scale bar indicates genetic distance.
Supplementary Figure 3 | Characterization of CaMYB102A, a paralog of CaDIM1. (A) Pairwise sequence alignment of CaMYB102A and CaDIM1. Sequence alignment was performed using EMBOSS Needle (https://www.ebi.ac.uk/Tools/psa/emboss_needle/index.html) with the default settings. (B) Expression levels of CaMYB102A gene in pepper leaves after treatment with abscisic acid (ABA) (100 μM) or drought. The pepper Actin1 (CaACT1) was used as an internal control. (C) Expression levels of CaDIM1 and CaMYB102A gene in the leaves of CaDIM1-silenced pepper plants treated with drought stress for 6 h. After quantitative real time polymerase chain reaction analysis RT-PCR analysis, the relative expression levels (ΔΔCT) of each gene were normalized using the pepper Actin1 (CaACT1) gene as an internal control and the expression value of each gene in TRV2:00 was set as 1.0. Asterisks indicate significant differences between TRV2:00 and TRV2:CaDIM1 plants (Student’s t-test; *P < 0.05).
Supplementary Table 1 | Sequences of primers used in this study.
References
Abe, H., Urao, T., Ito, T., Seki, M., Shinozaki, K., Yamaguchi-Shinozaki, K. (2003). Arabidopsis AtMYC2 (bHLH) and AtMYB2 (MYB) function as transcriptional activators in abscisic acid signaling. Plant Cell 15, 63–78. doi: 10.1105/tpc.006130
Agarwal, M., Hao, Y., Kapoor, A., Dong, C. H., Fujii, H., Zheng, X., et al. (2006). A R2R3 type MYB transcription factor is involved in the cold regulation of CBF genes and in acquired freezing tolerance. J. Biol. Chem. 281, 37636–37645. doi: 10.1074/jbc.M605895200
Ambawat, S., Sharma, P., Yadav, N. R., Yadav, R. C. (2013). MYB transcription factor genes as regulators for plant responses: an overview. Physiol. Mol. Biol. Plants. 19, 307–321. doi: 10.1007/s12298-013-0179-1
Clough, S. J., Bent, A. F. (1998). Floral dip: a simplified method for Agrobacterium-mediated transformation of Arabidopsis thaliana. Plant J. 16, 735–743. doi: 10.1046/j.1365-313x.1998.00343.x
Cominelli, E., Galbiati, M., Vavasseur, A., Conti, L., Sala, T., Vuylsteke, M., et al. (2005). A guard-cell-specific MYB transcription factor regulates stomatal movements and plant drought tolerance. Curr. Biol. 15, 1196–1200. doi: 10.1016/j.cub.2005.05.048
Cominelli, E., Sala, T., Calvi, D., Gusmaroli, G., Tonelli, C.. (2008). Over-expression of the Arabidopsis AtMYB41 gene alters cell expansion and leaf surface permeability. Plant J. 53, 53–64.
Ding, Z., Li, S., An, X., Liu, X., Qin, H., Wang, D. (2009). Transgenic expression of MYB15 confers enhanced sensitivity to abscisic acid and improved drought tolerance in Arabidopsis thaliana. J. Genet. Genom. 36, 17–29. doi: 10.1016/S1673-8527(09)60003-5
Dong, C. J., Liu, J. Y. (2010). The arabidopsis EAR-motif-containing protein RAP2.1 functions as an active transcriptional repressor to keep stress responses under tight control. BMC Plant Biol. 16, 10–47. doi: 10.1186/1471-2229-10-47
Dubos, C., Stracke, R., Grotewold, E., Weisshaar, B., Martin, C., Lepiniec, L. (2010). MYB transcription factors in Arabidopsis. Trends Plant Sci. 15, 573–881. doi: 10.1016/j.tplants.2010.06.005
Fujita, Y., Fujita, M., Shinozaki, K., Yamaguchi-Shinozaki, K. (2011). ABA-mediated transcriptional regulation in response to osmotic stress in plants. J. Plant Res. 124, 509–525. doi: 10.1007/s10265-011-0412-3
Geiger, D., Scherzer, S., Mumm, P., Marten, I., Ache, P., Matschi, S. (2010). Guard cell anion channel SLAC1 is regulated by CDPK protein kinases with distinct Ca2+ affinities. Proc. Natl. Acad. Sci. U.S.A. 107, 8023–8028. doi: 10.1073/pnas.0912030107
Geiger, D., Scherzer, S., Mumm, P., Stange, A., Marten, I., Bauer, H. (2009). Activity of guard cell anion channel SLAC1 is controlled by drought-stress signaling kinase-phosphatase pair. Proc. Natl. Acad. Sci. U.S.A. 106, 21425–21430. doi: 10.1073/pnas.0912021106
Ito, H., Fukuda, Y., Murata, K., Kimura, A. (1983). Transformation of intact yeast cells treated with alkali cations. J. Bacteriol. 153, 163–168. doi: 10.1128/jb.153.1.163-168.1983
Iuchi, S., Kobayashi, M., Taji, T., Naramoto, M., Seki, M., Kato, T. (2001). Regulation of drought tolerance by gene manipulation of 9-cis-epoxycarotenoid dioxygenase, a key enzyme in abscisic acid biosynthesis in Arabidopsis. Plant J. 27, 325–333. doi: 10.1046/j.1365-313x.2001.01096.x
Jenkins, G. I. (2009). Signal transduction in responses to UV-b radiation. Annu. Rev. Plant Biol. 60, 407–431. doi: 10.1146/annurev.arplant.59.032607.092953
Joo, H., Lim, C. W., Lee, S. C. (2019). A pepper RING-type E3 ligase, CaASRF1, plays a positive role in drought tolerance via modulation of CaAIBZ1 stability. Plant J. 98, 5–18. doi: 10.1111/tpj.14191
Joo, H., Lim, C. W., Lee, S. C. (2020). The pepper RING-type E3 ligase, CaATIR1, positively regulates abscisic acid signalling and drought response by modulating the stability of CaATBZ1. Plant Cell Environ. 43, 1911–1924. doi: 10.1111/pce.13789
Joshi, R., Wani, S. H., Singh, B., Bohra, A., Dar, Z. A., Lone, A. A., et al. (2016). Transcription factors and plants response to drought stress: current understanding and future directions. Front. Plant Sci. 7, 1029. doi: 10.3389/fpls.2016.01029
Jung, C., Seo, J. S., Han, S. W., Koo, Y. J., Kim, C. H., Song, S. I., et al. (2008). Overexpression of AtMYB44 enhances stomatal closure to confer abiotic stress tolerance in transgenic arabidopsis. Plant Physiol. 146, 623–635. doi: 10.1104/pp.107.110981
Kim, S., Park, M., Yeom, S. I., Kim, Y. M., Lee, J. M., Lee, H. A., et al. (2014). Genome sequence of the hot pepper provides insights into the evolution of pungency in capsicum species. Nat. Genet. 46, 270–278. doi: 10.1038/ng.2877
Kumar, M., Kesawat, M. S., Ali, A., Lee, S. C., Gill, S. S., Kim, A. H. U. (2019). Integration of abscisic acid signaling with other signaling pathways in plant stress responses and development. Plants. 8, 592. doi: 10.3390/plants8120592
Latchman, D. S. (1990). Eukaryotic transcription factors. Biochem. J. 270, 281–289. doi: 10.1042/bj2700281
Lee, S. C., Lan, W., Buchanan, B. B., Luan, S. (2009). A protein kinase-phosphatase pair interacts with an ion channel to regulate ABA signaling in plant guard cells. Proc. Natl. Acad. Sci. U.S.A. 106, 21419–21424. doi: 10.1073/pnas.0910601106
Lee, S. C., Luan, S. (2012). ABA signal transduction at the crossroad of biotic and abiotic stress responses. Plant Cell Environ. 35, 53–60. doi: 10.1111/j.1365-3040.2011.02426.x
Licausi, F., Ohme-Takagi, M., Perata, P. (2013). APETALA2/Ethylene responsive factor (AP2/ERF) transcription factors: mediators of stress responses and developmental programs. New Phytol. 199, 639–649. doi: 10.1111/nph.12291
Lim, C. W., Baek, W., Jung, J., Kim, J. H., Lee, S. C. (2015). Function of ABA in stomatal defense against biotic and drought stresses. Int. J. Mol. Sci. 16, 15251–15270. doi: 10.3390/ijms160715251
Lim, C. W., Baek, W., Lee, S. C. (2017). The pepper RING-type E3 ligase CaAIRF1 regulates ABA and drought signaling via CaADIP1 protein phosphatase degradation. Plant Physiol. 173, 2323–2339. doi: 10.1104/pp.16.01817
Lopez-Molina, L., Mongrand, S., McLachlin, D. T., Chait, B. T., Chua, N. H. (2002). ABI5 acts downstream of ABI3 to execute an ABA-dependent growth arrest during germination. Plant J. 32, 317–328. doi: 10.1046/j.1365-313X.2002.01430.x
Lu, S., Fadlalla, T., Tang, S., Li, L., Ali, U., Li, Q., et al. (2019). Genome-wide analysis of phospholipase d gene family and profiling of phospholipids under abiotic stresses in Brassica napus. Plant Cell Physiol. 60, 1556–1566. doi: 10.1093/pcp/pcz071
Millard, P. S., Kragelund, B. B., Burow, M. (2019). R2R3 MYB transcription factors–functions outside the DNA-binding domain. Trends Plant Sci. 24, 934–946. doi: 10.1016/j.tplants.2019.07.003
Mitchell, P. J., Tjian, R. (1989). Transcriptional regulation in mammalian cells by sequence-specific DNA binding proteins. Science. 245, 371–378. doi: 10.1126/science.2667136
Ng, L. M., Melcher, K., The, B. T., Xu, H. E. (2014). Abscisic acid perception and signaling: structural mechanisms and applications. Acta Pharmacol. Sin. 35, 567–584. doi: 10.1038/aps.2014.5
Oh, J. E., Kwon, Y., Kim, J. H., Noh, H., Hong, S. W., Lee, H. (2011). A dual role for MYB60 in stomatal regulation and root growth of Arabidopsis thaliana under drought stress. Plant Mol. Biol. 77, 91–103. doi: 10.1007/s11103-011-9796-7
Qin, C., Yu, C., Shen, Y., Fang, X., Chen, L., Min, J., et al. (2014). Whole genome sequencing of cultivated and wild peppers provides insights into capsicum domestication and specialization. Proc. Natl. Acad. Sci. U.S.A. 111, 5135–5140. doi: 10.1073/pnas.1400975111
Raghavendra, A. S., Gonugunta, V. K., Christmann, A., Grill, E. (2010). ABA perception and signalling. Trends Plant Sci. 15, 395–401. doi: 10.1016/j.tplants.2010.04.006
Reyes, J., Chua, N. (2007). ABA induction of mir159 controls transcript levels of two myb factors during arabidopsis seed germination. Plant J. 49, 592–606. doi: 10.1111/j.1365-313X.2006.02980.x
Sah, S. K., Reddy, K. R., Li, J. (2016). Abscisic acid and abiotic stress tolerance in crop plants front. Plant Front. 7, 571. doi: 10.3389/fpls.2016.00571
Sato, H., Takasaki, H., Takahashi, F., Suzuki, T., Iuchi, S., Mitsuda, N. (2018). Arabidopsis thaliana NGATHA1 transcription factor induces ABA biosynthesis by activating NCED3 gene during dehydration stress. Proc. Natl. Acad. Sci. U.S.A. 115, 11178–11187. doi: 10.1073/pnas.1811491115
Schroeder, J. I., Kwak, J. M., Allen, G. J. (2001). Guard cell abscisic acid signalling and engineering drought hardiness in plants. Nature. 410, 327–330. doi: 10.1038/35066500
Seo, P. J., Xiang, F., Qiao, M., Park, J. Y., Lee, Y. N., Kim, S. G., et al. (2009). The MYB96 transcription factor mediates abscisic acid signaling during drought stress response in arabidopsis. Plant Physiol. 151, 275–289. doi: 10.1104/pp.109.144220
Singh, D., Laxmi, A. (2015). Transcriptional regulation of drought response: a tortuous network of transcriptional factors. Front. Plant Sci. 6, 895. doi: 10.3389/fpls.2015.00895
Sun, Y., Zhao, J., Li, X., Li, Y. (2020). E2 conjugases UBC1 and UBC2 regulate MYB42-mediated SOS pathway in response to salt stress in Arabidopsis. New Phytol. 227, 455–472. doi: 10.1111/nph.16538
Takahashi, F., Kuromori, T., Urano, K., Yamaguchi-Shinozaki, K., Shinozaki, K. (2020). Drought stress responses and resistance in plants: from cellular responses to long-distance intercellular communication. Front. Plant Sci. 11, 556972. doi: 10.3389/fpls.2020.556972
Umezawa, T., Nakashima, K., Miyakawa, T., Kuromori, T., Tanokura, M., Shinozaki, K., et al. (2010). Molecular basis of the core regulatory network in ABA responses: sensing, signaling and transport. Plant Cell Physiol. 51, 1821–1839. doi: 10.1093/pcp/pcq156
Wingender, E., Schoeps, T., Haubrock, M., Dönitz, J. (2015). TFClass: a classification of human transcription factors and their rodent orthologs. Nucleic Acids Res. 43, 97–102. doi: 10.1093/nar/gku1064
Xie, Z., Nolan, T., Jiang, H., Tang, B., Zhang, M., Li, Z., et al. (2019). The AP2/ERF transcription factor TINY modulates brassinosteroid-regulated plant growth and drought responses in arabidopsis. Plant Cell. 31, 1788–1806. doi: 10.1105/tpc.18.00918
Xiong, L., Zhu, J. K. (2002). Molecular and genetic aspects of plant responses to osmotic stress. Plant Cell Environ. 25, 131–139. doi: 10.1046/j.1365-3040.2002.00782.x
Yang, S. J., Vanderbeld, B., Wan, J. X., Huang, Y. F. (2010). Narrowing down the targets: towards successful genetic engineering of drought-tolerant crops. Mol. Plant 3, 469–490. doi: 10.1093/mp/ssq016
Yang, Y., Zhang, L., Chen, P., Liang, T., Li, X., Liu, H. (2020). UV-B photoreceptor UVR8 interacts with MYB73/MYB77 to regulate auxin responses and lateral root development. EMBO J. 39, e101928. doi: 10.15252/embj.2019101928
Yang, W., Zhang, W., Wang, X. (2017). Post-translational control of ABA signalling: the roles of protein phosphorylation and ubiquitination. Plant Biotechnol. J. 15, 4–14. doi: 10.1111/pbi.12652
Yoshida, T., Mogami, J., Yamaguchi-Shinozaki, K. (2014). ABA-dependent and ABA-independent signaling in response to osmotic stress in plants. Curr. Opin. Plant Biol. 21, 133–139. doi: 10.1016/j.pbi.2014.07.009
Yu, Y.-T., Wu, Z., Lu, K., Bi, C., Liang, S., Wang, X.-F., et al. (2016). Overexpression of the MYB37 transcription factor enhances abscisic acid sensitivity and improves both drought tolerance and seed productivity in Arabidopsis thaliana. Plant Mol. Biol. 90, 267–279. doi: 10.1007/s11103-015-0411-1
Zhu, J. K. (2002). Salt and drought stress signal transduction in plants. Annu. Rev. Plant Biol. 53, 247–273. doi: 10.1146/annurev.arplant.53.091401.143329
Keywords: ABA, drought, MYB, pepper, transcription factor
Citation: Lim J, Lim CW and Lee SC (2022) Role of pepper MYB transcription factor CaDIM1 in regulation of the drought response. Front. Plant Sci. 13:1028392. doi: 10.3389/fpls.2022.1028392
Received: 26 August 2022; Accepted: 14 September 2022;
Published: 11 October 2022.
Edited by:
Dev Mani Pandey, Birla Institute of Technology, Mesra, IndiaReviewed by:
Manu Kumar, Dongguk University Seoul, South KoreaFrederik Börnke, Leibniz Institute of Vegetable and Ornamental Crops, Germany
Copyright © 2022 Lim, Lim and Lee. This is an open-access article distributed under the terms of the Creative Commons Attribution License (CC BY). The use, distribution or reproduction in other forums is permitted, provided the original author(s) and the copyright owner(s) are credited and that the original publication in this journal is cited, in accordance with accepted academic practice. No use, distribution or reproduction is permitted which does not comply with these terms.
*Correspondence: Sung Chul Lee, c2NsZWUxOTcyQGNhdS5hYy5rcg==
†These authors have contributed equally to this work