- 1Institute of Molecular and Cellular Biology, National Tsing Hua University, Hsinchu, Taiwan
- 2Taiwan Semiconductor Manufacturing Company, Hsinchu, Taiwan
- 3Depto. de Botânica, Universidade Federal do Paraná, Curitiba, Brazil
- 4Institut de Systématique, Evolution, Biodiversité (ISYEB), Muséum National d’Histoire Naturelle, EPHE, UA, CNRS, Sorbonne Université, Paris, France
- 5Department of Biology, University of Florida, Gainesville, FL, United States
- 6Department of Life Science, Biodiversity Program, Taiwan International Graduate Program, Biodiversity Research Center, Academia Sinica and National Taiwan Normal University, Taipei, Taiwan
- 7Museum of New Zealand Te Papa Tongarewa, Wellington, New Zealand
- 8Royal Botanic Gardens, South Yarra, VIC, Australia
- 9Wildland Consultants, Rotorua, New Zealand
- 10The Lewis B. and Dorothy Cullman Program for Molecular Systematics, New York Botanical Garden, Bronx, NY, United States
- 11Boyce Thompson Institute, Ithaca, NY, United States
- 12Plant Biology Section, Cornell University, Ithaca, NY, United States
While the family Schizaeaceae (Schizaeales) represents only about 0.4% of the extant fern species diversity, it differs from other ferns greatly in gross morphologies, niche preferences, and life histories. One of the most notable features in this family is its mycoheterotrophic life style in the gametophytic stage, which appears to be associated with extensive losses of plastid genes. However, the limited number of sequenced plastomes, and the lack of a well-resolved phylogenetic framework of Schizaeaceae, makes it difficult to gain any further insight. Here, with a comprehensive sampling of ~77% of the species diversity of this family, we first inferred a plastid phylogeny of Schizaeaceae using three DNA regions. To resolve the deep relationships within this family, we then reconstructed a plastome-based phylogeny focusing on a selection of representatives that covered all the major clades. From this phylogenomic backbone, we traced the evolutionary histories of plastid genes and examined whether gene losses were associated with the evolution of gametophytic mycoheterotrophy. Our results reveal that extant Schizaeaceae is comprised of four major clades—Microschizaea, Actinostachys, Schizaea, and Schizaea pusilla. The loss of all plastid NADH-like dehydrogenase (ndh) genes was confirmed to have occurred in the ancestor of extant Schizaeaceae, which coincides with the evolution of mycoheterotrophy in this family. For chlorophyll biosynthesis genes (chl), the losses were interpreted as convergent in Schizaeaceae, and found not only in Actinostachys, a clade producing achlorophyllous gametophytes, but also in S. pusilla with chlorophyllous gametophytes. In addition, we discovered a previously undescribed but phylogenetically distinct species hidden in the Schizaea dichotoma complex and provided a taxonomic treatment and morphological diagnostics for this new species—Schizaea medusa. Finally, our phylogenetic results suggest that the current PPG I circumscription of Schizaea is non-monophyletic, and we therefore proposed a three-genus classification moving a subset of Schizaea species sensu PPG I to a third genus—Microschizaea.
Introduction
Among the ~ 11,000 extant fern species, fewer than 40 belong to the family Schizaeaceae (Schizaeales; PPG I, 2016). Schizaeaceae differ from other ferns in their gross morphologies, niche preferences, life forms, and plastome structures (Reed, 1947; Bierhorst, 1971b; Kramer, 1990; Labiak and Karol, 2017). Despite the simplified morphology of these ferns, some foliar features can be used to separate them into different groups or genera—Microschizaea, Actinostachys, and Schizaea (Reed, 1947). These genera also differ in the unusual growth forms and habits of their gametophytes (Table 1). In Microschizaea, gametophytes are chlorophyllous, filamentous, and live on the soil surface (Table 1), and likely rely on fungal symbionts to survive in nutrition-poor habitats, such as bogs (Swatzell et al., 1996). By contrast, the genera Actinostachys and Schizaea have achlorophyllous, subterranean, endomycorrhizal gametophytes, with Actinostachys having tuberous and Schizaea thin-cylindrical growth forms (Table 1). The non-green and endomycorrhizal habit implies obligate mycoheterotrophy throughout their gametophyte generation, and might be an adaptation to deeply shaded habitats such as forest interiors, as well as sandy, nutrient poor soils (Graham et al., 2017). In comparison, the other members in the order Schizaeales—Lygodiaceae and Anemiaceae—have green planar and winged gametophytes typical of most ferns.
The current generic classification within Schizaeaceae has been largely based on morphological features (Reed, 1947; Tryon and Tryon, 1982), but whether these groupings reflect the phylogenetic relationships is unclear. The only molecular phylogenetics to date on Schizaeaceae was done by Wikström et al. (2002). In this study, while the plastid phylogeny was largely congruent with the morphology, their sampling included only one Microschizaea, the New World species M. pusilla (= Schizaea pusilla), which grouped with Schizaea. Microschizaea from the Old World (Table 1), including the type of the genus (M. fistulosa), have not yet been included in any molecular phylogenetic studies. The current phylogenetic consensus, in which Microschizaea is thought to be sister to Schizaea, was the basis for the acceptance of two genera in Schizaeaceae by Smith et al. (2006) and PPG I (2016) that include Actinostachys and Schizaea, under which Microschizaea is synonymized.
Recent studies on Schizaeaceae plastomes identified extensive gene losses in Schizaea and Actinostachys, which is unprecedented in ferns (Labiak and Karol, 2017; reviewed in Kuo et al., 2018a). For instance, all NADH-like dehydrogenase (ndh) genes were missing in all plastomes. These genes encode subunits for the NADH-like dehydrogenase complex that mediates photosynthetic electron flow of the photosystem I (Yamori and Shikanai, 2016), and is believed to be able to alleviate photo-oxidative stresses when plants are exposed to excessive light (Graham et al., 2017). In addition, all chlorophyll biosynthesis (chl) genes were missing in the Actinostachys plastome. The chl genes encode light-independent protochlorophyllide oxidoreductase (DPOR), and regulate one of the most important pathways of chlorophyll synthesis (Suzuki et al., 1998). Although similar losses have been documented in flowering plants that are predominately heterotrophs (Graham et al., 2017), no fern other than Schizaeaceae is known to have such extensive gene losses. It is possible that the plastid gene losses are associated with the mycoheterotrophic gametophytes in some Schizaeaceae members. To further test this relationship, it is necessary to look into the plastome of Microschizaea, which produces chlorophyllous gametophytes instead. In addition, the losses of some tRNA genes and structural changes in Schizaeaceae plastomes warrant further investigations with a more comprehensive species sampling.
In this study, we first inferred a Schizaeaceae phylogeny using a three-plastid-region dataset. Our sampling is the most comprehensive to date at the species level, and included all previously recognized genera and species groups (Table 1). Based on this phylogeny, representatives from every clade were then selected for a phyloplastomic reconstruction together with species from the other two Schizaeales families. This plastome-based approach resulted in better resolved inter-generic relationships within Schizaeaceae, allowing us to trace the evolutionary changes of plastome structures. To check whether ndh and chl genes have been transferred to the nuclear genome, we also examined their presence in transcriptomes. Finally, by mapping the gene loss events onto the phylogeny, we discussed the potential links to the specialization of life form in Schizaeaceae, in particular gametophytic mycoheterotrophy.
Materials and Methods
Sampling and Sequencing for Phylogenetic Analyses
A total of 47 Schizaeaceae specimens from 27 species (~ 77% of the species diversity of the family; PPG I, 2016) were sampled, including seven Microschizaea spp., seven Actinostachys spp., and 13 Schizaea spp. (Supplementary Table 1). This sampling covered all bioregions in each genus/clade (Table 1). For outgroups, we sampled the other two Schizaeales families: Anemia phylliditis from Anemiaceae and Lygodium japonicum from Lygodiaceae (PPG I, 2016). A modified CTAB protocol was used for DNA extractions (Kuo, 2015). The rpoC2, rbcL, and trnL-L-F (trnL gene + trnL-F intergenic spacer) were sequenced for our three-plastid-region dataset. PCRs were performed in 15 μl reactions each with 20 ng of genomic DNA, 0.5 μM of each primer, and 1 × SuperRed PCR Master Mix RED (TOOLS, New Taipei City, Taiwan). The resulting PCR products were purified and sequenced using the standard Sanger method with Applied Biosystems 3730XL (Thermo Fisher Scientific, Waltham, MA, United States of America) at Genomics Corp. (New Taipei City, Taiwan).
For the plastome phylogeny, we selected both Schizaeales outgroups and a total of 11 Schizaeaceae representatives. These included species from every genus and major clade within Schizaeaceae (two Microschizaea spp., two Actinostachys spp., and seven Schizaea spp.; Supplementary Table 1). Among these, published plastome sequences were already available for L. japonicum and three of the 11 species of Schizaeaceae (Gao et al., 2013; Labiak and Karol, 2017), and these were used for all downstream analyses. To assemble the remaining plastomes, we used the Illumina reads generated by either the GoFlag project (Breinholt et al., 2021) or the genome skimming of this study. When the sequencing depth of a GoFlag sample was insufficient to yield a circular plastome using NOVOplasty (see below for details), we designed PCR primers and closed the gaps between the contigs. The PCR recipe was the same as described earlier. For the genome skimming, we first sheared the genomic DNA into 400 ~ 500 bp fragments using a Covaris S2 ultrasonicator (Covaris, Woburn, MA, United States of America), which were then input into the NEBNext Ultra II DNA Library Prep Kit (New England Biolabs, Ipswich, MA, United States of America). Sequencing was done by HiSeq X Ten (Illumina, San Diego, CA, United States of America) with 150 bp PE and ~ 3Gb per sample. Fastp (Chen et al., 2018) was used to trim the reads using the default settings. NOVOplasty (Dierckxsens et al., 2017) was used to assemble the plastomes with the setting of “Kmer = 39,” and conspecific rbcL sequences were used as the input seeds. These plastome assemblies were annotated using Geneious (Kearse et al., 2012) with the published Schizaeaceae plastomes (GenBank accessions: KU764518, KX258660-61) as references. We manually inspected every protein-coding gene annotation, and adjusted the coordinates if necessary.
Details about the PCR primers are provided in Supplementary Table 2. Details about the voucher information, GenBank accessions, and NCBI Sequence Read Archive accessions can be found in Supplementary Table 1.
Phylogenetic Analyses
For the three-plastid-region dataset (i.e., “rpoC2 + rbcL + trnL-L-F”), the nucleotide sequences were first aligned with MUSCLE (Edgar, 2004), as implemented in AliView (Larsson, 2014), and then concatenated into a single matrix. This three-plastid-region matrix was partitioned by gene, by intergenic spacer (IGS), and by codon position in order to find the best partition scheme and substitution models using ModelFinder (Kalyaanamoorthy et al., 2017) with the Bayesian information criterion (BIC; Schwarz, 1978). Based on the inferred partition scheme and the substitution models, IQtree 1.6.8 (Nguyen et al., 2015) was used to construct the maximum likelihood (ML) phylogeny with 1,000 ultrafast bootstrap replicates (UFBS). The Bayesian phylogeny was inferred using MrBayes 3.2.7 (Ronquist et al., 2012). Two simultaneous runs were carried out with four chains (10 million generations each). Each chain was sampled every 1,000 generations. Log likelihoods of MCMC runs were inspected in Tracer 1.6 (Rambaut and Drummond, 2013) and RWTY (Warren et al., 2017) to confirm their convergence. The first 25% of the sample was discarded as burn-in, and the remaining was used to calculate the maximum clade credibility consensus tree with TreeAnnotator (Rambaut and Drummond, 2013).
For the phyloplastomic datasets, we only included coding sequences, introns, and intergenic spacers (IGS) that were consistently located in the large single copy (LSC) region of the Schizaeaceae plastomes. The restriction to LSC genes aimed to reduce phylogenetic artifacts resulting from substitution rate heterotachy, because the movement of genes between the inverted repeat (IR) and single copy (SC) regions would lead to changes in their substitution rates (Li et al., 2016). For alignment of every individual DNA region, we used MAFFT v7.450 (Katoh and Standley, 2013) and MACSE v2.03 (Ranwez et al., 2011). In total, we compiled five different matrices that consist of either the coding genes, noncoding regions (i.e., “IGS/INTRON”), or both (i.e., “67CDS + IGS/INTRON”). In the three coding gene matrices, 67 loci from the LSC were included with sequences of either (1) the first two codon positions (i.e., “codon1 + 2”), (2) the third codon position (i.e., “codon3”), and (3) all three codon positions (i.e., “67CDS”). For each of the five matrices, we performed four analyses with different models and partitions, as detailed in Table 2. With all the matrix/partition/model combinations, we conducted a total of 20 (= 5 × 4; Table 2) ML phylogenetic analyses in IQtree 1.6.8 each with 1,000 UFBS replicates and a series of tree topology tests (such as KH, SH, ELW, and AU; detailed in Minh et al., 2020) with a RELL replicate number of 10,000.
To examine the potential effects of homoplasy driven by rapidly evolving sites in CDS, particularly the third codon positions, we also analyzed two degenerate-coded matrices: one “67CDS” with “Degen”-coding (Zwick et al., 2012) and one “codon3” with RY-coding of third codon positions, and compared these results with those generated from original non-degenerated ones. For these two matrices, the same region partitions and model selection were used as those inferred by the original non-degenerated matrices, and the ML phylogenetic analyses was then performed with 1,000 UFBS replicates in IQtree 1.6.8. In addition, to examine the evolution of Schizaeales chl genes in more detail, we combined their sequences with those used in Kuo et al. (2018a), which has a complete order-level sampling. These sequences were aligned with MAFFT, and used to infer an ML phylogeny in IQtree 1.6.8 with the model GTR + FO*H4 and 1,000 UFBS replicates.
Morphological Comparisons Within the Schizaea dichotoma Complex
We examined herbarium collections from MO, P, TAIF, UC and WELT to conduct morphological comparisons between the S. dichotoma complex from Africa/Malagasy and Asia/Oceania. The Asian/Oceanian S. dichotoma complex contains several species—S. asperula, S. bifida, S. biroi, S. dichotoma and S. forsteri—whereas the African/Malagasy complex was represented by a single species—S. medusa sp. nov. We also measured several quantitative traits from these specimens, such as rhizome thickness, frond sizes, and stipe sizes (Supplementary Table 3).
Confirming the Existence of ndh and Missing Plastid Genes in Transcriptomes and Genomes
To confirm whether the nuclear-encoded ndh complex related genes was present or not in the Schizaeales, we downloaded their homologue sequences from the well-annotated case of Apostasia odorata (detailed in the Supplementary Material S2 of Lin et al., 2017), and blast-searched them against six Schizaeales transcriptomes (Qi et al., 2018; Shen et al., 2018; One Thousand Plant Transcriptomes (OTPT) Initiative, 2019) and two fern whole genomes (Li et al., 2018). For PnsB1, the homologue sequence from Marchantia polymorpha (Ueda et al., 2012) was used instead, because the annotated sequence from A. odorata (GenBank accession: KX156894) appeared to be misidentified. We also included the ndhV from Zostera marina (Ma et al., 2022), because the sequence of A. odorata appeared to be unusually diverged from other plants. The transcriptomes were obtained from previous studies which used foliar, photosynthetic tissues for RNAseq, and included two Lygodiaceae (Lygodium japonicum and Lygodium flexuosum), two Anemiaceae (Anemia tomentosa and Anemia phyllitidis), and two Schizaeaceae (Actinostachys digitata and Schizaea dichotoma). We first used the sequences from A. odorata, Z. marina, and M. polymorpha as the query for tBLASTx searches against the Azolla filiculoides and Salvinia cucullata genomes via FernBase (https://www.fernbase.org/). Based on the criterion of a percentage of identity, we obtained the best-matched sequences from the fern genomes. To confirm whether these sequences are homologues or not, we conducted tBLASTx searches against the nucleotide collections of NCBI, and inferred their gene trees based on the blast results. After obtaining the fern homologous sequences from these genomes, we then added these sequences into the tBLASTx searches against the transcriptomic assemblies using blast-2.10.0 + (Camacho et al., 2009). Only the transcripts having > 60% sequence identity and consistent hits to all query homologues (i.e., those from the two ferns, A. odorata, Z. marina, and M. polymorpha) were kept.
To examine the possibility that the missing protein-coding genes in the plastome have been transferred to and are now transcribed in the nuclear genome, we also conducted local tBLASTx searches for these genes as mentioned above. The query sequences were derived from plastomes of the closest relatives that still retain those genes (e.g., using Anemiaceae’s rps16 sequence to blast against the Schizaeaceae’s transcriptomes).
Results
Plastome Features of Schizaeaceae
In total, nine plastomes were assembled in this study, including one Anemia and eight Schizaeaceae. They all assembled into a single circular contig, except for the plastome of Microschizaea tenella in which the gap between psbK and trnQ could not be closed by PCR and was thus separated into two contigs. All members of Schizaeaceae share several plastome features, including the expansions of the IR and losses of all ndh genes (ndhA–J), rps16, ycf94, and several tRNA genes (Figure 1). IR/SC boundaries of the Schizaeaceae plastomes are almost identical, and their small single copy region (SSC) are highly reduced (Figure 1). In the most extreme case, the SSC of S. pusilla is only 1,091 bp in length and contains only one gene, trnN. Actinostachys, on the other hand, appears to be an outlier where the SSC expanded to include ccsA, rps15, and ycf1. The chl genes (chlB, chlN, and chlL) are absent in the plastomes of two clades—S. pusilla and Actinostachys, but this is unlikely to have resulted from a single loss event (Figure 2; see below in “Discussion”). The loss of psaM gene was shared by Schizaeaceae and its sister family—Anemiaceae (Figure 2).
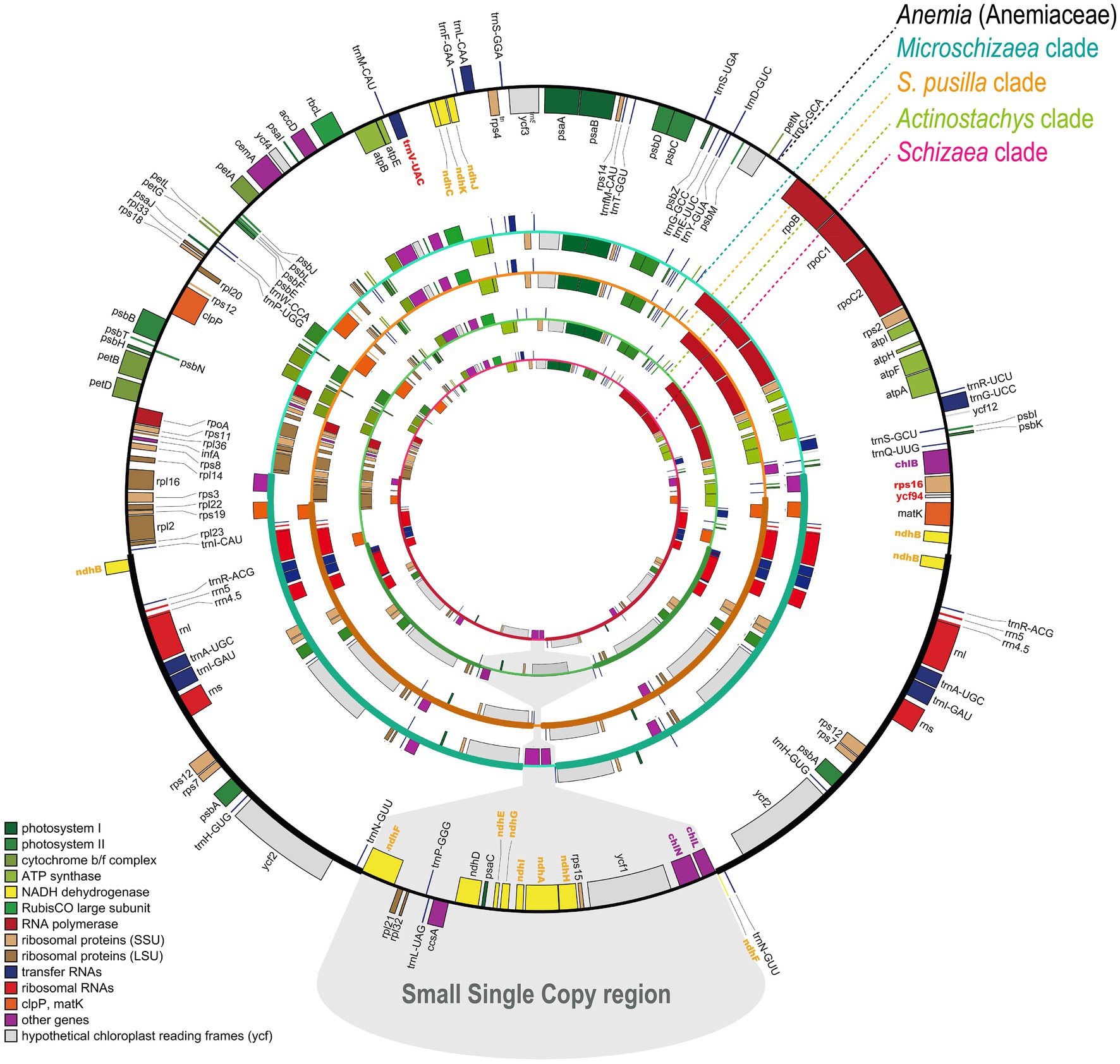
Figure 1. The plastome maps of Schizaeaceae and Anemiaceae. The genes missing in Schizaeaceae plastomes have bolded and colored names. The maps are derived from OrganellarGenomeDRAW (Greiner et al., 2019).
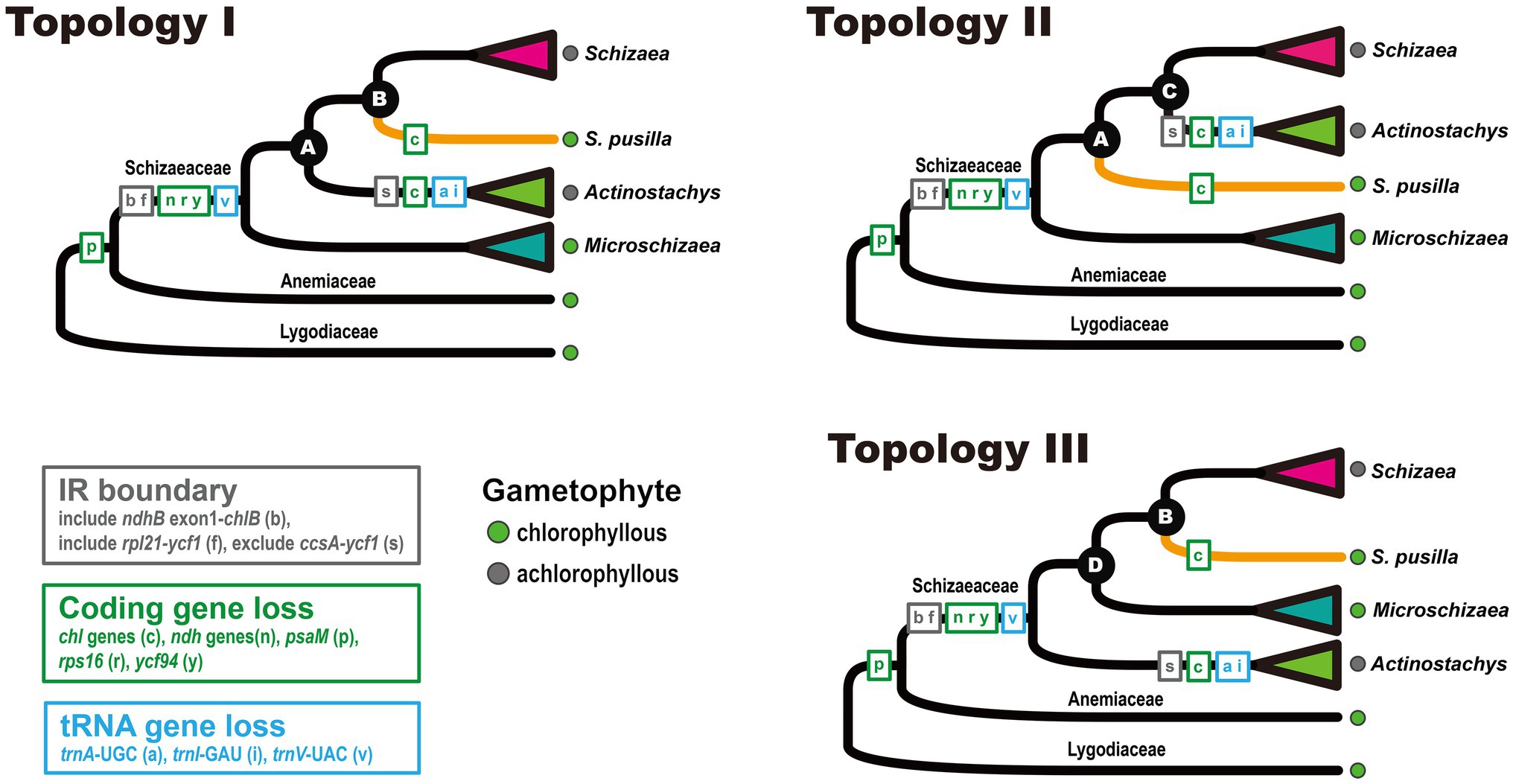
Figure 2. Plastome structural evolution in Schizaeaceae. The three topologies were derived from the phyloplastomic analyses that included different data matrices, models, and partitions.
Phylogeny of Schizaeaceae
From our three-plastid-region and plastome datasets (Figures 2, 3) four well-supported clades were recovered (UFBS = 100, Bayesian inference = 1.00) within Schizaeaceae—Schizaea, S. pusilla, Actinostachys, and Microschizaea (Figure 3). These clades closely matched their generic definition by Reed (1947), except for S. pusilla which formed a rather distinct clade from other Microschizaea spp. However, the inferred inter-clade relationships differed among our phylogenetic analyses (Table 2). Generally, topology I received the highest branch supports, and another two were rejected by most of our topological tests (Table 2). Only the “codon3” matrix with a gene-partitioned GTR + F + R10 model highly supported topology II (Table 2), but this support was declined or nearly unchanged when applying the degenerate-coded matrices with analyses (Supplementary Table 4).
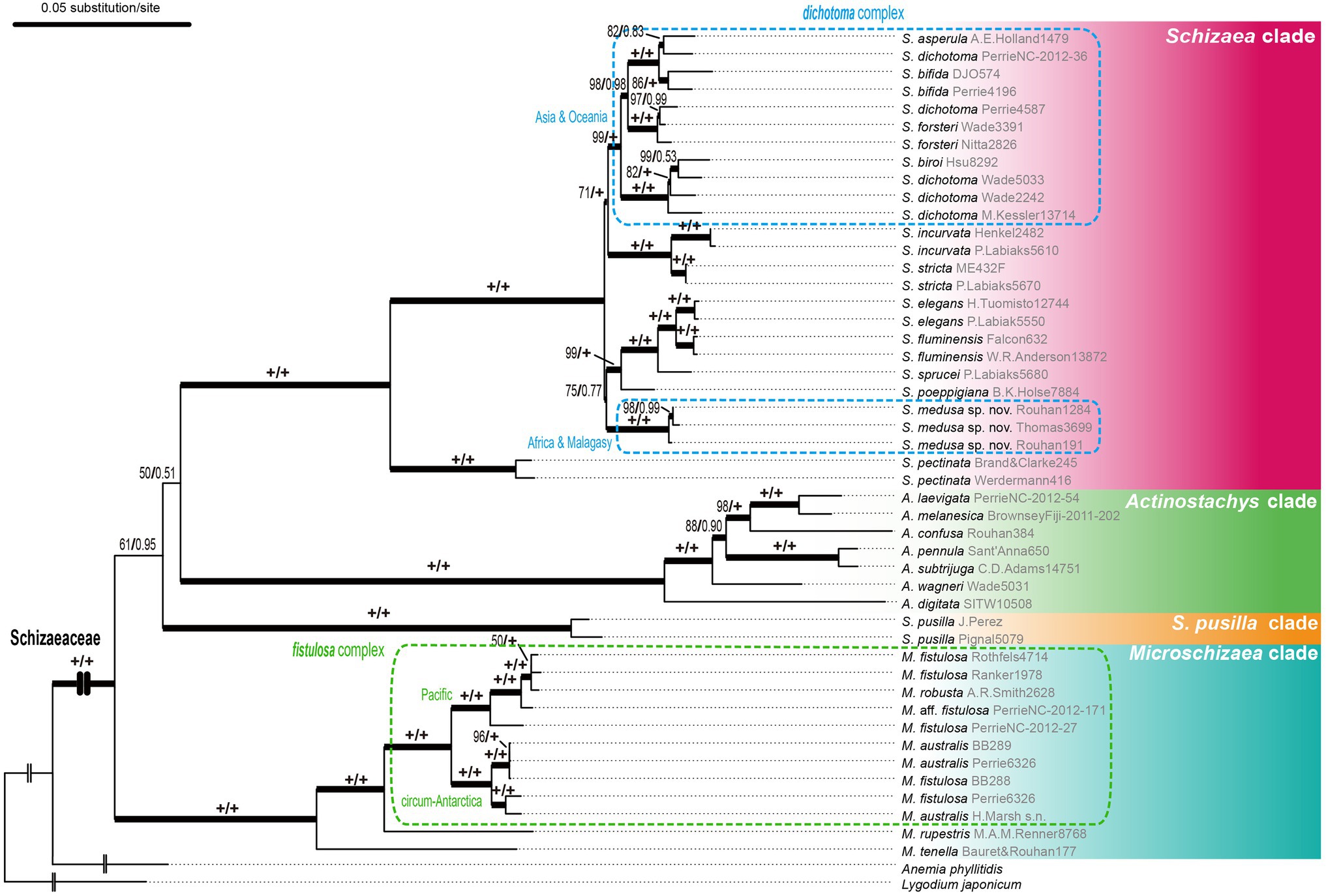
Figure 3. The plastid phylogeny of Schizaeaceae based on the rpoC2 + rbcL + trnL-L-F dataset. Maximum likelihood ultrafast bootstrap supports (UFBS) and Bayesian inference (BI) are indicated on each branch as UFBS/BI.
In the Schizaea clade, the S. dichotoma complex was non-monophyletic with two separate lineages, one from Africa/Madagascar, and the second from Asia/Oceania (Figure 3). In the Microschizaea clade, M. robusta and M. australis were nested within M. fistulosa, rendering M. fistulosa polyphyletic (Figure 3).
Presence of ndh and chl Genes in Transcriptomes and Genomes
We detected the nuclear-encoded ndh-related genes in the transcriptomes of other Schizaeales (Anemiaceae and Lygodiaceae) and the genomes of Salvinia and Azolla, except for pnsL1–4, psnB2, ndhV, CRR2–4, and CRR21 (Figure 4). For CRR41, we identified it in Salvinia but not in Azolla. This gene likely existed in other Schizaeales (Anemiaceae and Lygodiaceae), whose CRR41-matched transcripts showed consistent blast-hits to the homologous queries of CRR41 although relatively low in the identity ranging from 40–54%. In contrast, all plastid and most nuclear-encoded ndh-related genes were missing in the Schizaeaceae transcriptomes (Figure 4). We were also unable to detect any chl genes in the transcriptome of Actinostachys, whose plastomes also lack these genes. The plastid rps16 and ycf94 genes were also absent from the transcriptomes of Schizaeaceae, and psaM was absent from both Schizaeaceae and Anemiaceae.
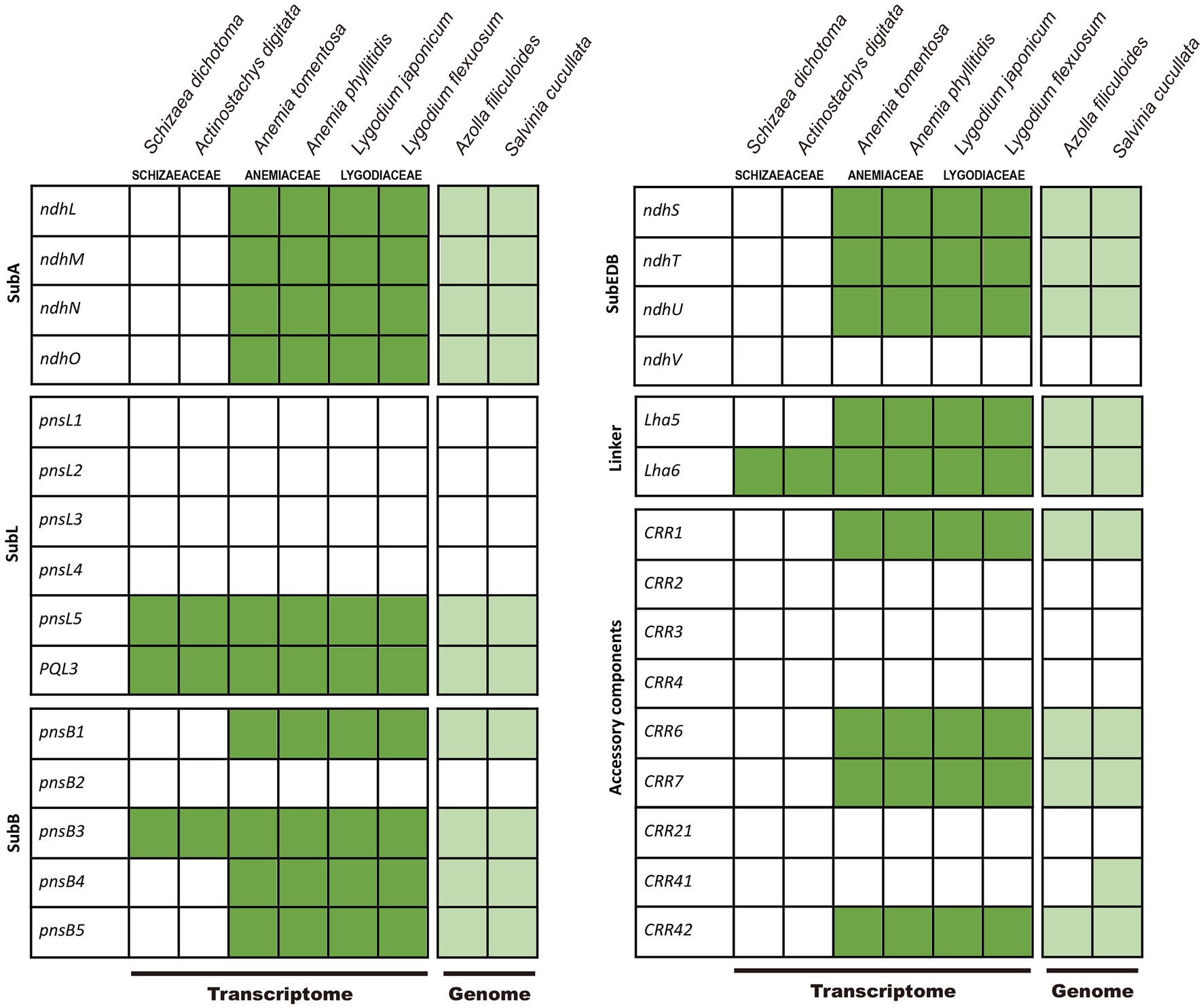
Figure 4. Presence of the nuclear-encoded genes of NADH-like dehydrogenase (ndh) complex in Schizaeales transcriptome and fern genomes. The subcomplexes (sub), linker, and accessory components follow the scheme by Shikanai (2016).
Taxonomic Treatment
Schizaea medusa L.Y.Kuo, B.F.Ke, F.W.Li, and Rouhan, sp. nov (Figure 5).
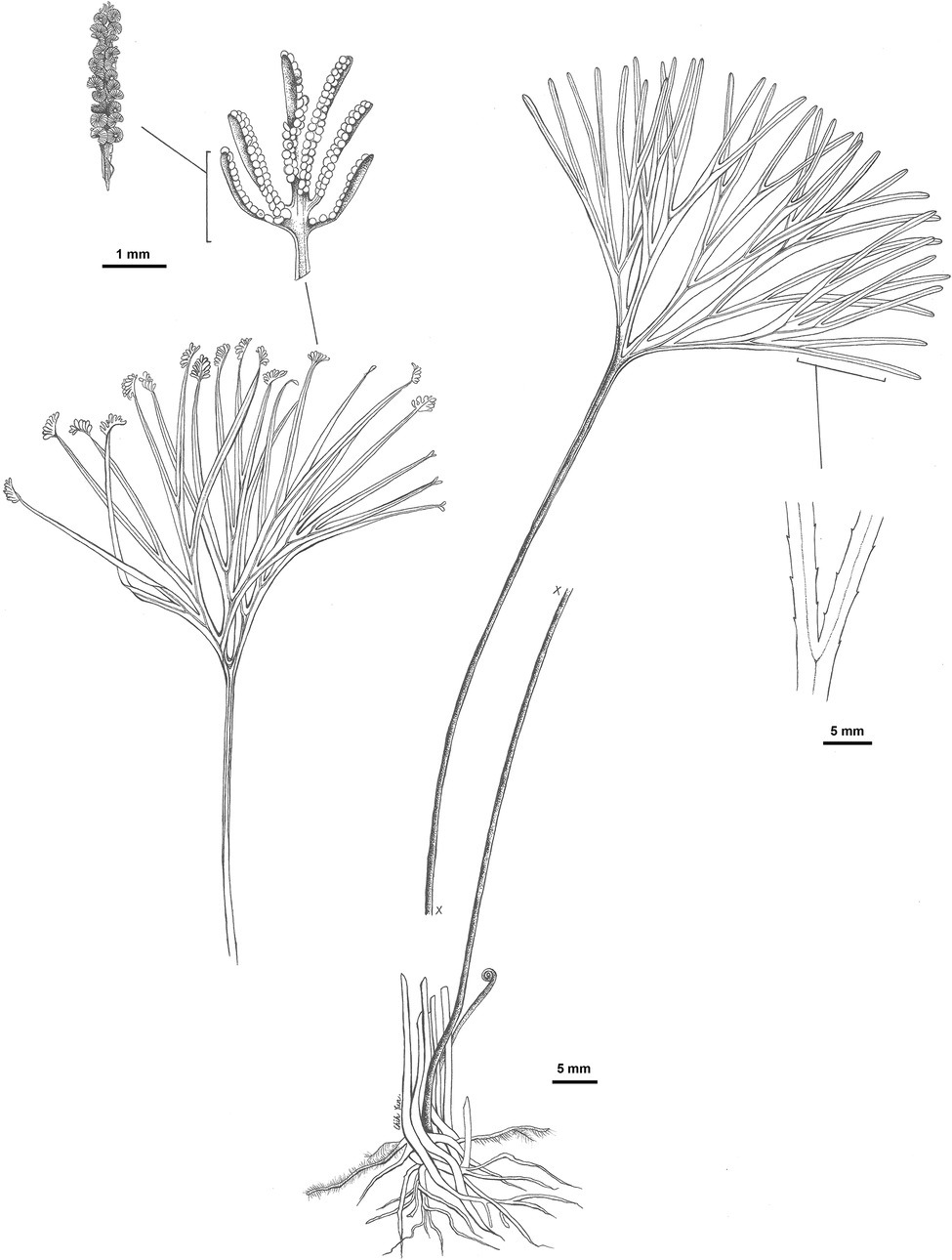
Figure 5. Illustration of Schizaea medusa L.-Y. Kuo, B.-F. Ke, F.-W. Li, and Rouhan, sp. nov., based on the holotype G. Rouhan 1284 (P02432844).
Type: MADAGASCAR, Andapa, Sava, 31 October 2011, G. Rouhan, M. Gaudeul & J. Ranaivo 1284 (holotype: P[P02432844]! isotypes: NY, TAN).
Diagnosis: This species is morphologically most similar to S. dichotoma and other members of this species complex, but, in comparison, has a thicker (usually 5–12 mm in diameter) and erect/ascending rhizome (vs. creeping rhizomes with 0.7–6 mm diameter in other members of the S. dichotoma complex). The blade-to-stipe ratio in this species is on average higher than that in other members of the S. dichotoma complex (Supplementary Figure 1).
Description: Plants terrestrial. Rhizomes erect or ascending, (3–) 5–12 mm in diameter, bearing chestnut-brown, septate hairs. Fronds 20.5–42.2 cm long; stipes 11.1–30.0 cm long; sterile portion of laminae 2–6 times dichotomously divided, 4.2–12.8 cm long, ±terete, furrowed on one side with stomata 81–114 μm long. Fertile portion of laminae pinnately divided, 2–11 mm long, 1–4 mm wide. Fertile segments in 4–15 pairs per spike, infolded, 1–5 mm long, with fimbriate margins. Sporangia borne in two rows, 24–26 per branch, intermixed with yellow-brown septate hairs. Spores with perines granular or smooth, polar lengths 22–30 μm, equatorial lengths 37–38 μm, ratios of polar to equatorial length 0.57–0.66.
Additional Specimens Examined: Madagascar. Tulear Province, 22 March 1992, R. A. Clement, P.B. Phillipson & G. Rafamantanantsoa 2088 (MO); 16 March 1985, L. J. Dorr 3979 (MO); Toamasina, 04 October 2003, R. Razakamalala et al. 773 (MO); 12 June 2004, R. Ranaivojaona et al. 750 (MO); 10 December 2006, J. Razanatsoa & T. Marcellin 268 (MO); 1 February 2001, F. Ratovoson et al. 450 (MO); 24 October 2001, J. -N. Labat 3409 (MO); 8 June 1987, P. Phillipson 1879 (MO); 31, May 2007, A. Razanatsima et al. 265 (MO); 21 October 2007, P. Antilahimena & T. Marcelin 5859 (MO); Antsiranana Province, 22 October 1989, J.S. Miller & A. Randrianasolo 4343 (MO); 2 December 2007, C. Rakotovao et al. 3878 (MO); 19 January 2004, L. Nusbaumer, LN 1044 (MO); 11 September 2007, L. Nusbaumer et al., LN 2423 (MO); 8–12 May 1993, S. Malcomber & C. Hemingway 2483 (MO); 26 November 2000, A. Rasolohery 100 (MO); 11 November 2006, C. Rakotovao et al. 3326 (MO); 30 October 2000, P. Antilahimenaet et al. 622 (MO); 24 September 2004, T. Janssen 2386 (P); Fianarantsoa Province, 26 August 2003, D. Rabehevitra, R. Razakamalala & I. Dely 562 (MO); 4 June 1992, R. Rakoto 58 (MO); 14 January 2006, A. Anderberg et al. 71 (MO); 10 October 1992, H. van der Werff et al. 12651 (MO); 30 September 1987, P. Phillipson 2197 (MO); 12 October 2015, G. Rouhan 1656 (P); Toliara, 25 May 2005, N. M. Andrianjafy et al. 1105 (MO); November 2005, Richard Razakamalala et al. 2523 (MO); 27 February 2009, R. Razakamalala et al. 4332 (MO); 20 November 2009, C. Rakotovao et al. 4647 (MO); 19 February 2009, C. Rakotovao et al. 4344 (MO); 13 March 1989, N. Dumetz 574 (MO); Tamatave Province, 2 November 1985, L. J. Dorr et al. 4320 (MO); 2–5 November 1984, L. J. Dorr & L. C. Barnett 3223 (MO); 29 August 1987, G. E. Schatz & W. D’Arcy 1489 (MO); 19 January 1986, L.C. Barnett & C. Rakotozafy 4588 (MO); Anosy region, 22 May 2006, F. Randriatafika et al. 675 (MO); 26 November 2004, G. Rouhan 481 (P); Majunga Province, 11 January 1985, A. Rakotozafy & R. Rajemisa 289 (MO); Mahajanga, 25 April 2007, D. Ravelonarivo 226 (MO); Antananarivo, 16 November 2003, P. P. Lowry II et al. 6274 (MO); August 1987, David K. Edelman 142 (MO); Lokobe Strict Reserve, 4 October 1991, C. Birkinshaw 35 (MO); Tanzania. Morogoro region, 22 September 1984, Thomas 3699 (MO, P); 2 December 1987, J. Lovett & D. W. Thomas 2621 (MO); Iringa, 2 August 1989, C. J. Kayombo 764 (MO). Réunion. Hauts de Saint Louis, 2006, J. Dupont 3997 (P); 6 July 1973, T. Cadet 4316 (P). Mauritius. Mare Longue Plateau, 1 April 2003, G. Rouhan 191 (P).
Distribution: Africa (Tanzania), Madagascar, Réunion, Comoros, and Mauritius.
Etymology: The fronds of this species produce numerous terminal branches that are somewhat interlaced each ending with a sporangiate spike. This foliar diagnostic is superficially similar to Medusa, a well-known winged human female in Greek mythology, whose head is crowned by entwining snakes.
Discussion
A New Generic Classification of Schizaeaceae
Our phylogenetic analyses based on different datasets, models, and partitions resulted in three general topologies (Figure 2). While data from plastome structure and gene content failed to provide additional evidence, we have more confidence in topology I (Table 2). This topology received, on average, higher branch supports (i.e., nodes A and B), and was not rejected in most of the topology tests (Table 2). On the other hand, although topology II also received high branch supports in some analyses, it was mostly derived from matrices comprised of the third codon positions. It is likely that the support for topology II is a result of high homoplasy at the third codon position (Supplementary Table 4), which is known to have higher substitution rates and therefore be more likely to reach saturation (e.g., Breinholt and Kawahara, 2013).
Notably, our study is the first to include Microschizaea species other than S. pusilla. All the inferred phylogenies suggested that Microschizaea sensu Reed (1947) is not monophyletic and fell into two distinct, non-sister clades—Microschizaea and S. pusilla (Figure 2). The Microschizaea clade comprises all Microschizaea species except S. pusilla (Figures 2, 3). The position of S. pusilla varied across the three inferred topologies, but was never found to be sister to Microschizaea. In the most highly supported topology (topology I), S. pusilla was placed sister to the Schizaea clade similar to what was reported by Wikström et al. (2002). The close relationship between the S. pusilla and the Schizaea clades is morphologically supported by the shared presence of multicellular hairs intermixing with sporangia, a trait that is absent in the Microschizaea clade (Table 1). Schizaea pusilla also differs from Microschizaea by its more ellipsoidal (i.e., bilateral) spores with a ratio of polar to equatorial length (P:E) about 0.66, compared to the subglobose or ovoid spores in the Microschizaea clade with P:E around 0.72–0.76 (Reed, 1947; Table 1). Finally, spore perines of S. pusilla are alveolate with shallow pits but those of the Microschizaea clade are granular or smooth (Table 1).
Despite bearing morphological and phylogenetic differences, Microschizaea was retained in synonymy of the genus Schizaea in the latest phylogenetic classification of PPG I (2016), and Actinostachys was there recognized as the second genus of Schizaeaceae. Here, we show Schizaea as circumscribed by PPG I is very likely paraphyletic. We therefore propose a three-genus framework for Schizaeaceae that recognizes Microschizaea, Actinostachys, and Schizaea. This classification is similar but different from Reed (1947) in that we placed S. pusilla in Schizaea rather than in Microschizaea. It can be argued that given its unresolved placement and distinct morphology, S. pusilla could be erected as a separate genus. We are however hesitant to do so based on the current data. Regarding the infra-generic system of Reed (1947), many of his subgenera and (sub)sections are non-monophyletic; further studies are needed to provide a clear picture for these infra-generic schemes.
Systematics of Species Complexes
The simple foliar structure in Schizaeaceae presents taxonomic challenges, particularly in the Microschizaea fistulosa and Schizaea dichotoma species complexes. The M. fistulosa complex contains the polyphyletic “M. fistulosa” and most of its congeneric members (Figure 3), and has a broad distribution across Southeastern Asia, Pacific Islands, Oceania, and South America (Lash, 1966; Brownsey and Perrie, 2014; Giacosa et al., 2015). Morphologically variable but continuous forms make taxonomy of this complex still unsettled. There are more than ten names within this complex but these names are always treated under a single name—M. fistulosa or with another—M. australis (Lash, 1966; Brownsey and Perrie, 2013, 2014). In addition, two cytotypes/ploidy levels have been discovered. The specimens in New Zealand can be separated into M. australis with a lower chromosome number (n = 94) and smaller overall plant sizes, and M. fistulosa with a higher chromosome number (n = c.150, 190) and larger individual sizes (Brownsey and Perrie, 2013). From our plastid tree, Pacific samples of this complex appear to form a group, while circum-Antarctic samples form another (Figure 3). However, because plastid sequences can track only the maternal lineage in ferns (reviewed in Kuo et al., 2018b), the current phylogeny is still insufficient to shed light into any polyploidization and reticulation history in the M. fistulosa complex. Future systematics studies need to incorporate cytological information, details of microcharacters, and analyses of nuclear markers.
The S. dichotoma complex likewise exhibits wide morphological and cytological variations (Brownsey and Perrie, 2014). Some forms have been formally named to reflect their distinct morphology, such as S. asperula showing sterile-fertile dimorphic fronds and S. bifida showing only twice-to-thrice bifurcated lamina. However, most members of this complex are poorly characterized, and have been collectively lumped into “S. dichotoma”, which is found to be polyphyletic in our phylogeny (Figure 3). We discovered that the African and Malagasy specimens form a clade that is phylogenetically distinct from the rest of the S. dichotoma members (Figure 3). Because the types of all the previously named species in this complex are based on Asian and Oceanian materials, a new species is warranted, which we named Schizaea medusa. By quantifying several key morphological characters, we showed that S. medusa can be distinguished from S. dichotoma (Supplementary Figure S1; also see in “Taxonomic Treatment”). There are likely additional cryptic species in the S. dichotoma complex and more studies are clearly needed.
Specialization of Gametophytic Lifestyle and Losses of Plastid Genes
Gametophytes of Schizaeaceae are peculiar among extant ferns because of their strongly mycotrophic lifestyle, whether they are achlorophyllous (like Schizaea and Actinostachys) or not (like S. pusilla and Microschizaea). Only one exceptional case, reporting a surface-living and chlorophyllous gametophyte in the Schizaea clade, is from the observation of S. bifida by Thomas (1902). However, this record needs to be confirmed because S. bifida usually co-occurs with Microschizaea (Brownsey and Perrie, 2014), and fern gametophytes in the field could be easily misidentified without genetic evidence (Nitta and Chambers, 2022; Wu et al., 2022). Despite being chlorophyllous, gametophytes of S. pusilla and Microschizaea still show several properties that are not found in other chlorophyllous fern gametophytes. Their gametophytes are filamentous and partially or sometimes completely underground, with their subterranean parts being achlorophyllous (Britton and Taylor, 1901; Lash, 1966; Bierhorst, 1968). In addition, previous studies failed to regenerate sporophytes from these gametophytes under axenic conditions (Lash, 1966; Swatzell et al., 1996), implying that microbial symbionts might be required for sexual reproduction (Britton and Taylor, 1901; Lash, 1966; Swatzell et al., 1996). Finally, at least in S. pusilla, the gametophytes unusually exhibit a negative phototropism during spore germination (Kiss, 1994). Taken together, Schizaeaceae are clearly heterotrophic or mixotrophic at the gametophyte stage, and have intimate associations with fungal symbionts.
Heterotrophic or mixotrophic plants often display certain diagnostic genomic signatures (e.g., Vogel et al., 2018; Su et al., 2019; Xu et al., 2021), such as plastomes with extensive gene losses (Graham et al., 2017; Hadariová et al., 2018; Wicke and Naumann, 2018). Here we confirmed that the loss of plastid ndh genes is a synapomorphy of extant Schizaeaceae (Figure 2), and we also could not detect expression of most nuclear ndh-related genes from Schizaeaceae transcriptomes. In other words, there might be a concerted loss of ndh-related genes in both genomic compartments (Figure 4), a feature that has been suggested to be the initial (but irreversible) step toward mycoheterotrophy in flowering plants (Graham et al., 2017; Lin et al., 2017). Interestingly, a separate fern lineage Stromatopteris (Gleicheniaceae), which also produces mycoheterotrophic gametophytes (Bierhorst, 1971b), has likewise experienced ndh gene losses in the plastome (Du et al., 2022).
Loss of the plastid chl genes is frequently found in plants with a heterotrophic and/or achlorophyllous nature for their gametophyte generation (e.g., all flowering plants), and is thus considered as one genomic indicator for heterotrophs (Ueda et al., 2014). These plastid chl genes encode all subunits for light-independent protochlorophyllide oxidoreductase (DPOR), which plays an important role for chlorophyll synthesis under dark, particularly during the gametophyte stage (Suzuki et al., 1998; Ueda et al., 2014). Complete lack of chl genes had been documented in two fern lineages, Psilotaceae and Actinostachys in Schizaeaceae (Grewe et al., 2013; Zhong et al., 2014; Labiak and Karol, 2017; Kuo et al., 2018a), whose gametophytes are also achlorophyllous (Bierhorst, 1971b). Here we found that in Schizaeaceae, there were actually two independent losses of chl genes (Figures 1, 2; Supplementary Figure 2). One of them, however, is found in S. pusilla, a species that produces chlorophyllous gametophytes (Figures 1, 2). The link between chl gene loss and the achlorophyllous nature of gametophytes might therefore seem not obligate. The gametophytes of S. pusilla likely rely on nuclear-encoded light-dependent NADPH-protochlorophyllide oxidoreductase (LPOR) instead, the alternative pathway for chlorophyll synthesis, but advanced genetic evidence is required to test such a hypothesis. On the other hand, several fern and lycophyte lineages producing achlorophyllous gametophytes retain functional chl genes in their plastomes, such as Lycopodiaceae, Ophioglossaceae, and the Schizaea clade in the present case (reviewed in Kuo et al., 2018a). These retentions imply that DPOR remains important for chlorophyll synthesis in the autotrophic (or mixotrophic) sporophytes of these ferns. Alternatively, DPOR could be involved in different physiological functions, resembling the situation in the (potentially) fully mycoheterotrophic bryophytes, which also have chl-retained plastomes (Bell et al., 2020).
In addition to ndh and chl genes, several other coding and tRNA genes have disappeared from Schizaeaceae plastomes (Figures 1, 2). From our blast results against the transcriptomes, the coding genes are unlikely to have been transferred to the nuclear genome. However, these genes do not seem to be associated with the specialization of gametophytic lifestyle in Schizaeaceae, and are mostly considered to have minor functions in the plastid. For instance, rps16 has been lost several times in ferns (Zhang et al., 2014; Kuo et al., 2018a; Du et al., 2022). Ycf94, which was recently identified in plastomes of seed-free plants and whose function is still unknown (Song et al., 2018), seems to be absent only in Schizaeaceae (Du et al., 2022).
Conclusion and Future Perspectives
Schizaeaceae is one of the most understudied families of ferns. With the most comprehensive sampling to date, our phylogenomic analyses resolved important relationships in this family, and provided the most robust infrafamiliar backbone for Schizaeaceae. We proposed a new phylogenetic classification modified from the Reed’s (1947) system, in which a portion of Schizaea sensu PPG I (2016) is moved to a third genus—Microschizaea. In addition, our species-level phylogeny illustrated species complexes within this family. One cryptic species hidden in the S. dichotoma complex was identified and described as a new species, Schizaea medusa. Using our new phylogenetic framework, we were able to trace the evolution of plastome features as well as the gametophytic lifestyle in Schizaeaceae. Specifically, we provided a better picture of the relationship between plastid gene loss and mycoheterotrophy in fern gametophytes.
Importantly, this phylogenetic study sets the stage for future work delving into the unique biological features of Schizaeaceae and in Schizaeales, such as Mesozoic biogeography (Skog, 2001), epiphytism on tree ferns (Amoroso et al., 2020), leaf simplification and lamina reformation (Vasco et al., 2013), biosynthesis of silica bodies (Ribeiro et al., 2007; Tzu-Tong Kao personal communications), and recruitment of symbiotic microbiome (e.g., Chen et al., 2022). Lastly, the pheromone-mediated (i.e., antheridiogen) mating system has been well-studied in the other two Schizaeales families (Yamane, 1991, 1998; Tanaka et al., 2014) but not yet for Schizaeaceae. It would be very interesting to explore whether gametophytes of Schizaeaceae rely on the similar pheromone system for their “underground” mating.
Collaborators of GoFlag Consortium
GoFlag is an NSF-funded project (DEB 1541506) based at the University of Florida, Field Museum, and University of Arizona. Project personnel include (at UF): J. Gordon Burleigh, Emily Sessa, Stuart McDaniel, Christine Davis, Pavlo Antonenko, Sarah Carey, Lorena Endara, Weston Testo; (at Field): Matt von Konrat, Eve Gaus; (at UA): Hong Cui.
Data Availability Statement
The datasets presented in the study are publicly available. The data can be found at Nucleotide Collections at GenBank: https://www.ncbi.nlm.nih.gov/nuccore/ with the accession numbers: ON120846, ON207049-ON207054, ON314247-ON314248, and ON368093-ON368187.
Author Contributions
L-YK and B-FK designed the experiments and drafted the manuscript. L-YK, B-FK, GoFlag Consortium, and F-WL carried out the experiments. L-YK, B-FK, and G-JW analyzed the data. PL, GR, C-WC, LS, DO, MR, and KK collected important samples and DNA sequences. L-YK, B-FK, GR, and F-WL collected materials for the taxonomic treatment. All authors contributed to the article and approved the submitted version.
Funding
The main funding was from Ministry of Science and Technology of Taiwan (MOST 109-2621-B-007-001-MY3), NSF GoLife program (DEB-1541506 to P Antonenko, JG Burleigh, EC Davis, SF McDaniel, and EB Sessa), and the Bioresource Conservation Research Center in College of Life Science from the Higher Education Sprout Project by MOE was granted.
Conflict of Interest
G-JW and MR were employed by Taiwan Semiconductor Manufacturing Company and Wildland Consultants.
The remaining authors declare that the research was conducted in the absence of any commercial or financial relationships that could be construed as a potential conflict of interest.
Publisher’s Note
All claims expressed in this article are solely those of the authors and do not necessarily represent those of their affiliated organizations, or those of the publisher, the editors and the reviewers. Any product that may be evaluated in this article, or claim that may be made by its manufacturer, is not guaranteed or endorsed by the publisher.
Supplementary Material
The Supplementary Material for this article can be found online at: https://www.frontiersin.org/articles/10.3389/fpls.2022.885501/full#supplementary-material
Acknowledgments
We thank Leon Perrie, Claudine Mynssen, Joel Nitta, Carl Rothfels, Tian-Chuan Hsu, Michael Kessler and Kathleen Pryer’s lab for providing their DNA collections of this study; Taiwan Pteridophyte Research Group (TPG) for maintaining DNA collections of this study; the staff in herbaria MEL, MO, NSW, P, PE, SEL, TAIF, UC, and WELT for making their collections available for this study; the two reviewers for their comments on the manuscript; Chih-Yun Sun for preparing the line drawing; Technology Common (College of Life Science, National Taiwan University, Taiwan) for the assistance with DNA library constructions; Genomics Corp. (New Taipei City, Taiwan) for Hiseq sequencing; and Mr. Shann-Jye Moore Memorial Scholarship for providing financial support for herbarium collection surveys.
References
Amoroso, V. B., Coritico, F. P., and Fritsch, P. W. (2020). Actinostachys minuta, a new species of grass fern from Mindanao, Philippines. PhytoKeys 151, 59–66. doi: 10.3897/phytokeys.151.53100
Bell, D., Lin, Q., Gerelle, W. K., Joya, S., Chang, Y., Taylor, Z. N., et al. (2020). Organellomic data sets confirm a cryptic consensus on (unrooted) land-plant relationships and provide new insights into bryophyte molecular evolution. Am. J. Bot. 107, 91–115. doi: 10.1002/ajb2.1397
Bierhorst, D. W. (1965). Older gametophytes and young sporophytes of Schizaea melanesica. Bull. Torrey. Bot. Club 92, 475–488. doi: 10.2307/2483825
Bierhorst, D. W. (1966). The fleshy, cylindrical, subterranean gametophyte of Schizaea melanesica. Am. J. Bot. 53, 123–133. doi: 10.1002/j.1537-2197.1966.tb07311.x
Bierhorst, D. W. (1967). The gametophyte of Schizaea dichotoma. Am. J. Bot. 54, 538–549. doi: 10.2307/2440657
Bierhorst, D. W. (1968). Observations on Schizaea and Actinostachys spp., including A. oligostachys, sp. nov. Am. J. Bot. 55, 87–108. doi: 10.2307/2440497
Bierhorst, D. W. (1971a). Morphology and anatomy of new species of Schizaea and Actinostachys. Am. J. Bot. 58, 634–648. doi: 10.2307/2441008
Bierhorst, D. W. (1975). Gametophytes and embryos of Actinostachys pennula, A. wagneri, and Schizaea elegans, with notes on other species. Am. J. Bot. 62, 319–335. doi: 10.2307/2442086
Breinholt, J. W., Carey, S. B., Tiley, G. P., Davis, E. C., Endara, L., McDaniel, S. F., et al. (2021). A target enrichment probe set for resolving the flagellate land plant tree of life. Appl. Plant Sci. 9:e11406. doi: 10.1002/aps3.11406
Breinholt, J. W., and Kawahara, A. Y. (2013). Phylotranscriptomics: saturated third codon positions radically influence the estimation of trees based on next-gen data. Genome Biol. Evol. 5, 2082–2092. doi: 10.1093/gbe/evt157
Britton, E. G., and Taylor, A. (1901). Life history of Schizaea pusilla. Bull. Torrey. Bot. Club 28, 1–19. doi: 10.2307/2478391
Brownsey, P. J., and Perrie, L. R. (2013). Taxonomic notes on the New Zealand flora: the status of Schizaea australis and S. fistulosa, and lectotypes in Lygodiaceae and Schizaeaceae. New Zeal. J. Bot. 51, 79–87. doi: 10.1080/0028825X.2011.650649
Brownsey, P. J., and Perrie, L. R. (2014). “Schizaeaceae,” in Flora of New Zealand - Ferns and Lycophytes. Fascicle 5. eds. I. Breitwieser, P. B. Heenan, and A. D. Wilton (Lincoln: Manaaki Whenua Press).
Camacho, C., Coulouris, G., Avagyan, V., Ma, N., Papadopoulos, J., Bealer, K., et al. (2009). BLAST+: architecture and applications. BMC Bioinform. 10:421. doi: 10.1186/1471-2105-10-421
Chen, K.-H., Xi, Q.-Y., Chang, C.-C., and Kuo, L.-Y. (2022). Mycobiome detection from a single subterranean gametophyte using metabarcoding techniques. Appl. Plant Sci. 10:e11461. doi: 10.1002/aps3.11461
Chen, S., Zhou, Y., Chen, Y., and Gu, J. (2018). Fastp: an ultra-fast all-in-one FASTQ preprocessor. Bioinformatics 34, i884–i890. doi: 10.1093/bioinformatics/bty560
Dierckxsens, N., Mardulyn, P., and Smits, G. (2017). NOVOPlasty: de novo assembly of organelle genomes from whole genome data. Nucleic Acids Res. 45:e18. doi: 10.1093/nar/gkw955
Du, X.-Y., Kuo, L.-Y., Zuo, Z.-Y., Li, D.-Z., and Lu, J.-M. (2022). Structural variation of plastomes provides key insight into the deep phylogeny of ferns. Front. Plant Sci. 13:862772. doi: 10.3389/fpls.2022.862772
Edgar, R. C. (2004). MUSCLE: multiple sequence alignment with high accuracy and high throughput. Nucleic Acids Res. 32, 1792–1797. doi: 10.1093/nar/gkh340
Gao, L., Wang, B., Wang, Z.-W., Zhou, Y., Su, Y.-J., and Wang, T. (2013). Plastome sequences of Lygodium japonicum and Marsilea crenata reveal the genome organization transformation from basal ferns to core leptosporangiates. Genome Biol. Evol. 5, 1403–1407. doi: 10.1093/gbe/evt099
Giacosa, J. P. R., and Barakat, M. C. (2018). Spore morphology and wall ultrastructure of Actinostachys pennula (Sw.) hook. And A. subtrijuga (Mart.) C. Presl. (Schizaeaceae). Palynology 42, 483–491. doi: 10.1080/01916122.2017.1413688
Giacosa, J. P. R., Morbelli, M. A., and Giudice, G. E. (2015). Morphology and ultrastructure of Schizaea fisulosa (Schizaeaceae) spores from Chile. Bull. Bot. Soc. Argentina 50, 17–22. doi: 10.31055/1851.2372.v50.n1.10846
Goebel, K. (1915). Organographie der Pflanzen, insbesondere der Archegoniaten und Samenpflanzen. Jena: G. Fischer.
Graham, S. W., Lam, V. K. Y., and Merckx, V. S. F. T. (2017). Plastomes on the edge: the evolutionary breakdown of mycoheterotroph plastid genomes. New Phytol. 214, 48–55. doi: 10.1111/nph.14398
Greiner, S., Lehwark, P., and Bock, R. (2019). OrganellarGenomeDRAW (OGDRAW) version 1.3.1: expanded toolkit for the graphical visualization of organellar genomes. Nucleic Acids Res. 47, W59–W64. doi: 10.1093/nar/gkz238
Grewe, F., Guo, W., Gubbels, E. A., Hansen, A. K., Mower, J. J. P., Wicke, S., et al. (2013). Complete plastid genomes from Ophioglossum californicum, Psilotum nudum, and Equisetum hyemale reveal an ancestral land plant genome structure and resolve the position of Equisetales among monilophytes. BMC Evol. Biol. 13:8. doi: 10.1186/1471-2148-13-8
Hadariová, L., Vesteg, M., Hampl, V., and Krajčovič, J. (2018). Reductive evolution of chloroplasts in non-photosynthetic plants, algae and protists. Curr. Genet. 64, 365–387. doi: 10.1007/s00294-017-0761-0
Kalyaanamoorthy, S., Minh, B. Q., Wong, T. K. F., Von Haeseler, A., and Jermiin, L. S. (2017). ModelFinder: fast model selection for accurate phylogenetic estimates. Nat. Methods 14, 587–589. doi: 10.1038/nmeth.4285
Katoh, K., and Standley, D. M. (2013). MAFFT multiple sequence alignment software version 7: improvements in performance and usability. Mol. Biol. Evol. 30, 772–780. doi: 10.1093/molbev/mst010
Kearse, M., Moir, R., Wilson, A., Stones-Havas, S., Cheung, M., Sturrock, S., et al. (2012). Geneious basic: an integrated and extendable desktop software platform for the organization and analysis of sequence data. Bioinformatics 28, 1647–1649. doi: 10.1093/bioinformatics/bts199
Kiss, J. Z. (1994). Negative phototropism in young gametophytes of the fern Schizaea pusilla. Plant Cell Environ. 17, 1339–1343. doi: 10.1111/j.1365-3040.1994.tb00536.x
Kramer, K. U. (1990). “Schizaeaceae,” in The Families and Genera of Vascular Plants. Vol. I. Pteridophytes and Gymnosperms. eds. K. U. Kramer and P. S. Green (Berlin Heidelber: Springer), 258–262.
Kuo, L.-Y. (2015). Polyploidy and biogeography in genus Deparia and phylogeography in Deparia lancea. in Ph.D. dissertation, Taipei: National Taiwan University.
Kuo, L.-Y., Qi, X., Ma, H., and Li, F.-W. (2018a). Order-level fern plastome phylogenomics: new insights from Hymenophyllales. Am. J. Bot. 105, 1545–1555. doi: 10.1002/ajb2.1152
Kuo, L.-Y., Tang, T.-Y., Su, H.-J., Li, F.-W., Chiou, W.-L., Huang, Y.-M., et al. (2018b). Organelle genome inheritance in Deparia ferns (Athyriaceae, Aspleniineae, Polypodiales). Front. Plant Sci. 9:486. doi: 10.3389/fpls.2018.00486
Labiak, P. H., and Karol, K. G. (2017). Plastome sequences of an ancient fern lineage reveal remarkable changes in gene content and architecture. Am. J. Bot. 104, 1008–1018. doi: 10.3732/ajb.1700135
Larsson, A. (2014). AliView: a fast and lightweight alignment viewer and editor for large data sets. Bioinformatics 30, 3276–3278. doi: 10.1093/bioinformatics/btu531
Lash, I. (1966). Studies in Schizaea fistulosa Labill. Master thesis. Christchurch: University of Canterbury.
Li, F.-W., Brouwer, P., Carretero-Paulet, L., Cheng, S., Vries, J. d., Delaux, P.-M., et al. (2018). Fern genomes elucidate land plant evolution and cyanobacterial symbioses. Nat. Plants 4, 460–472. doi: 10.1038/s41477-018-0188-8
Li, F.-W., Kuo, L.-Y., Pryer, K. M., and Rothfels, C. J. (2016). Genes translocated into the plastid inverted repeat show decelerated substitution rates and elevated GC content. Genome Biol. Evol. 8, 2452–2458. doi: 10.1093/gbe/evw167
Lin, C. S., Chen, J. J. W., Chiu, C. C., Hsiao, H. C. W., Yang, C. J., Jin, X. H., et al. (2017). Concomitant loss of NDH complex-related genes within chloroplast and nuclear genomes in some orchids. Plant J. 90, 994–1006. doi: 10.1111/tpj.13525
Ma, M., Zhong, M., Zhang, Q., Zhao, W., Wang, M., Luo, C., et al. (2022). A genome-wide analysis of the chloroplast NADH dehydrogenase-like genes in Zostera marina. J. Oceanol. Limnol. 40, 656–677. doi: 10.1007/s00343-021-0027-z
Minh, B. Q., Trifinopoulos, J., Schrempf, D., Schmidt, H. A., and Lanfear, R. (2020). IQ-TREE version 2.0: tutorials and manual Phylogenomic software by maximum likelihood. Available at: http://www.iqtree.org (Accessed April 06, 2021).
Nguyen, L. T., Schmidt, H. A., Von Haeseler, A., and Minh, B. Q. (2015). IQ-TREE: a fast and effective stochastic algorithm for estimating maximum-likelihood phylogenies. Mol. Biol. Evol. 32, 268–274. doi: 10.1093/molbev/msu300
Nitta, J. H., and Chambers, S. M. (2022). Identifying cryptic fern gametophytes using DNA barcoding: a review. Appl. Plant Sci. 10:e11465. doi: 10.1002/aps3.11465
One Thousand Plant Transcriptomes (OTPT) Initiative (2019). One thousand plant transcriptomes and the phylogenomics of green plants. Nature 574, 679–685. doi: 10.1038/s41586-019-1693-2
Parkinson, B. M. (1994). Morphological and ultrastructural variations in Schizaea pectinata (Schizaeaceae: Pteridophyta). Bothalia 24, 203–210. doi: 10.4102/abc.v24i2.772
PPG I (2016). A community-derived classification for extant lycophytes and ferns. J. Syst. Evol. 54, 563–603. doi: 10.1111/jse.12229
Qi, X., Kuo, L.-Y., Guo, C., Li, H., Li, Z., Qi, J., et al. (2018). A well-resolved fern nuclear phylogeny reveals the evolution history of numerous transcription factor families. Mol. Phylogenet. Evol. 127, 961–977. doi: 10.1016/J.YMPEV.2018.06.043
Rambaut, A., and Drummond, A. J. (2013). Trace v1.6. Available at: http://tree.bio.ed.ac.uk/software/tracer/ (Accessed December 11, 2013).
Ranwez, V., Harispe, S., Delsuc, F., and Douzery, E. J. P. (2011). MACSE: multiple alignment of coding sequences accounting for frameshifts and stop codons. PLoS One 6:e22594. doi: 10.1371/journal.pone.0022594
Reed, C. F. (1947). The phylogeny and ontogeny of the Pteropsida. I. Schizaeales. Bol. da Soc. Broteriana, ser. 2 21, 71–197.
Ribeiro, M. L. R. C., Santos, M. G., and Moraes, M. G. (2007). Leaf anatomy of two Anemia Sw. Species (Schizaeaceae-Pteridophyte) from a rocky outcrop in Niterói, Rio de Janeiro. Brazil. Rev. Bras. Bot. 30, 695–702. doi: 10.1590/S0100-84042007000400014
Ronquist, F., Teslenko, M., van derMark, P., Ayres, D. L., Darling, A., Höhna, S., et al. (2012). MrBayes 3.2: efficient Bayesian phylogenetic inference and model choice across a large model space. Syst. Biol. 61, 539–542. doi: 10.1093/sysbio/sys029
Schwarz, G. (1978). Estimating the dimension of a model. Ann. Stat. 6, 461–464. doi: 10.1214/aos/1176344136
Shen, H., Jin, D., Shu, J., Zhou, X., Lei, M., Wei, R., et al. (2018). Large scale phylogenomic analysis resolves a backbone phylogeny in ferns. Gigascience 7, 1–11. doi: 10.1093/gigascience/gix116/4656250
Shikanai, T. (2016). Chloroplast NDH: a different enzyme with a structure similar to that of respiratory NADH dehydrogenase. Biochim. Biophys. Acta Bioenerg. 1857, 1015–1022. doi: 10.1016/j.bbabio.2015.10.013
Skog, J. (2001). Biogeography of Mesozoic leptosporangiate ferns related to extant ferns. Brittonia 53, 236–269. doi: 10.1007/BF02812701
Smith, A. R., Pryer, K. M., Schuettpelz, E., Korall, P., Schneider, H., and Wolf, P. G. (2006). A classification for extant ferns. Taxon 55, 705–731. doi: 10.2307/25065646
Song, M., Kuo, L.-Y., Huiet, L., Pryer, K. M., Rothfels, C. J., and Li, F.-W. (2018). A novel chloroplast gene reported for flagellate plants. Am. J. Bot. 105, 117–121. doi: 10.1002/ajb2.1010
Su, H. J., Barkman, T. J., Hao, W., Jones, S. S., Naumann, J., Skippington, E., et al. (2019). Novel genetic code and record-setting AT-richness in the highly reduced plastid genome of the holoparasitic plant Balanophora. Proc. Natl. Acad. Sci. 116, 934–943. doi: 10.1073/pnas.1816822116
Suzuki, T., Takio, S., and Satoh, T. (1998). Light-dependent expression in liverwort cells of chlL/N and chlB identified as chloroplast genes involved in chlorophyll synthesis in the dark. J. Plant Physiol. 152, 31–37. doi: 10.1016/S0176-1617(98)80098-9
Swatzell, L. J., Powell, M. J., and Kiss, J. Z. (1996). The relationship of endophytic fungi to the gametophyte of the fern Schizaea pusilla. Int. J. Plant Sci. 157, 53–62. doi: 10.1086/297320
Tanaka, J., Yano, K., Aya, K., Hirano, K., Takehara, S., Koketsu, E., et al. (2014). Antheridiogen determines sex in ferns via a spatiotemporally split gibberellin synthesis pathway. Science 346, 469–473. doi: 10.1126/science.1259923
Thomas, A. P. W. (1902). An alga-like fern-prothallium. Ann. Bot. os-16, 165–170. doi: 10.1093/oxfordjournals.aob.a088864
Tryon, A. F., and Lugardon, B. (1990). Spores of the Pteridophyta. New York, NY: Springer-Verlag Press.
Ueda, M., Kuniyoshi, T., Yamamoto, H., Sugimoto, K., Ishizaki, K., Kohchi, T., et al. (2012). Composition and physiological function of the chloroplast NADH dehydrogenase-like complex in Marchantia polymorpha. Plant J. 72, 683–693. doi: 10.1111/j.1365-313X.2012.05115.x
Ueda, M., Tanaka, A., Sugimoto, K., Shikanai, T., and Nishimura, Y. (2014). ChlB requirement for chlorophyll biosynthesis under short photoperiod in Marchantia polymorpha L. Genome Biol. Evol. 6, 620–628. doi: 10.1093/gbe/evu045
Vasco, A., Moran, R. C., and Ambrose, B. A. (2013). The evolution, morphology, and development of fern leaves. Front. Plant Sci. 4:345. doi: 10.3389/fpls.2013.00345
Vogel, A., Schwacke, R., Denton, A. K., Usadel, B., Hollmann, J., Fischer, K., et al. (2018). Footprints of parasitism in the genome of the parasitic flowering plant Cuscuta campestris. Nat. Commun. 9:2515. doi: 10.1038/s41467-018-04344-z
Warren, D. L., Geneva, A. J., and Lanfear, R. (2017). RWTY (R we there yet): an R package for examining convergence of Bayesian phylogenetic analyses. Mol. Biol. Evol. 34, 1016–1020. doi: 10.1093/molbev/msw279
Wicke, S., and Naumann, J. (2018). Molecular evolution of plastid genomes in parasitic flowering plants. Adv. Bot. Res. (Elsevier Ltd.) 85, 315–347. doi: 10.1016/bs.abr.2017.11.014
Wikström, N., Kenrick, P., and Vogel, J. C. (2002). Schizaeaceae: a phylogenetic approach. Rev. Palaeobot. Palynol. 119, 35–50. doi: 10.1016/S0034-6667(01)00128-2
Wu, Y.-H., Ke, Y.-T., Chan, Y.-Y., Wang, G.-J., and Kuo, L.-Y. (2022). Integrating tissue-direct PCR into genetic identification: an upgraded molecular ecology way to survey fern field gametophytes. Appl. Plant Sci. 10:e11462. doi: 10.1002/aps3.11462
Xu, Y., Lei, Y., Su, Z., Zhao, M., Zhang, J., Shen, G., et al. (2021). A chromosome-scale Gastrodia elata genome and large-scale comparative genomic analysis indicate convergent evolution by gene loss in mycoheterotrophic and parasitic plants. Plant J. 108, 1609–1623. doi: 10.1111/tpj.15528
Yamane, H. (1991). “Antheridiogens of Schizaeaceous Ferns: Structures, Biological Activities, and Biosynthesis,” in Gibberellins. eds. N. Takahashi, B. O. P. Hinney, and J. MacMillan (New York, NY: Springer), 378–388.
Yamane, H. (1998). Fern antheridiogens. Int. Rev. Cyrol. 184, 1–32. doi: 10.1016/S0074-7696(08)62177-4
Yamori, W., and Shikanai, T. (2016). Physiological functions of cyclic electron transport around photosystem I in sustaining photosynthesis and plant growth. Annu. Rev. Plant Biol. 67, 81–106. doi: 10.1146/annurev-arplant-043015-112002
Zhang, W.-Y., Kuo, L.-Y., Li, F.-W., Wang, C.-N., and Chiou, W.-L. (2014). The hybrid origin of Adiantum meishanianum (Pteridaceae): a rare and endemic species in Taiwan. Syst. Bot. 39, 1034–1041. doi: 10.1600/036364414X682616
Zhong, B., Fong, R., Collins, L. J., McLenachan, P. A., and Penny, D. (2014). Two new fern chloroplasts and decelerated evolution linked to the long generation time in tree ferns. Genome Biol. Evol. 6, 1166–1173. doi: 10.1093/gbe/evu087
Keywords: chl, Microschizaea, mycoheterotrophy, ndh, phyloplastome, Schizaeaceae
Citation: Ke B-F, Wang G-J, Labiak PH, Rouhan G, on behalf of the GoFlag Consortium, Chen C-W, Shepherd LD, Ohlsen DJ, Renner MAM, Karol KG, Li F-W and Kuo L-Y (2022) Systematics and Plastome Evolution in Schizaeaceae. Front. Plant Sci. 13:885501. doi: 10.3389/fpls.2022.885501
Edited by:
Kathleen Pryer, Duke University, United StatesReviewed by:
Domingos Cardoso, Federal University of Bahia, BrazilKathryn T. Picard, Smithsonian National Museum of Natural History (SI), United States
Copyright © 2022 Ke, Wang, Labiak, Rouhan, Chen, Shepherd, Ohlsen, Renner, Karol, Li and Kuo. This is an open-access article distributed under the terms of the Creative Commons Attribution License (CC BY). The use, distribution or reproduction in other forums is permitted, provided the original author(s) and the copyright owner(s) are credited and that the original publication in this journal is cited, in accordance with accepted academic practice. No use, distribution or reproduction is permitted which does not comply with these terms.
*Correspondence: Li-Yaung Kuo, lykuo@life.nthu.edu.tw