- 1Department of Horticulture, University of Georgia, Griffin, GA, United States
- 2AgriLife Research Center, Department of Horticultural Sciences, Texas A&M University, Dallas, TX, United States
- 3Department of Horticulture and Architecture, Colorado State University, Fort Collins, CO, United States
Peat moss has desirable properties as a container substrate, however, harvesting it from peatland for greenhouse/nursery production use has disturbed peatland ecosystem and caused numerous environmental concerns. More recently, many nations have taken actions to reduce or ban peat moss production to reach the carbon neutral goal and address the environmental concerns. Also, the overuse of fertilizers and pesticides with peat moss in greenhouse/nursery production adds extra environmental and economic issues. Thus, it is urgent to find a peat moss replacement as a container substrate for greenhouse/nursery production. Biochar, a carbon-rich material with porous structure produced by the thermo-chemical decomposition of biomass in an oxygen-limited or oxygen-depleted atmosphere, has drawn researchers’ attention for the past two decades. Using biochar to replace peat moss as a container substrate for greenhouse/nursery production could provide environmental and economic benefits. Biochar could be derived from various feedstocks that are regenerated faster than peat moss, and biochar possesses price advantages over peat moss when local feedstock is available. Certain types of biochar can provide nutrients, accelerate nutrient adsorption, and suppress certain pathogens, which end up with reduced fertilizer and pesticide usage and leaching. However, among the 36,474 publications on biochar, 1,457 focused on using biochar as a container substrate, and only 68 were used to replace peat moss as a container substrate component. This study provides a review for the environmental and economic concerns associated with peat moss and discussed using biochar as a peat moss alternative to alleviate these concerns.
1 Introduction
Peatlands contribute vital ecological services such as storing organic carbon (C) and nitrogen (N), regulating water, influencing methane (CH4), and providing habitats (Leifeld and Menichetti, 2018; Humpenöder et al., 2020). Peatlands occupied around 4% of the terrestrial surface but stored 644 Gt of C or 21% of the global total soil organic C stock (Yu et al., 2010; Scharlemann et al., 2014; Dargie et al., 2017; Leifeld and Menichetti, 2018). Northern peatlands alone store 17 Gt N, and for well-grown sphagnum peatlands, one single sphagnum farming site takes up N at 35~56 kg ha-1 yr-1 (Temmink et al., 2017; Hugelius et al., 2020). By regulating water flows, peatlands help minimize the risk of flooding and drought and prevent seawater intrusion (Rizzuti et al., 2004). In the peatland system, up to 90% of biologically CH4 produced is consumed due to activities of methanogens and methanotrophs (Liebner et al., 2011). Peatlands also provide precious habitats for different wild animals (Alexander et al., 2008).
Harvesting peat moss, a commonly used container substrate in horticulture, has caused numerous environmental concerns. Large scale peatlands drainage caused carbon dioxide (CO2) and nitrous oxide (N2O) emissions more than 2 Gt CO2-eq yr-1. The CO2 emissions from the drained peatlands are estimated at 1.3 Gt CO2 annually, which is equivalent to 5.6% of the global anthropogenic CO2 emissions (Nature, 2017). In addition, the drainage of peatland, with other gas and fuels extractions, contributed 23% of the total CH4 budget of 500 to 600 tera gram per annum (Reumer et al., 2018), and increased the total CH4 emissions from 334 Tg yr-1 to 366 Tg yr-1 (Saunois et al., 2020). Peatland extraction reduced surface and groundwater quality, and increased land compaction (Temmink et al., 2017). Moreover, peat extraction has caused 15% of global peatland habitats lost for wild animals, including Bornean Orangutans (Barthelmes, 2016; Nature, 2017; Vaughn et al., 2018). If the peatland extraction trend continues, the cumulative of greenhouse gases (GHGs) CO2 equivalent emission would reach to 249 Gt by 2100 (Heck et al., 2021). Among the 17 United Nations Sustainable Development Goals, 8 goals are closely related to ecosystem interference and global warming (The United Nations Sustainable Development Goals, 2015). Therefore, reducing the use of peat moss and finding a peat moss replacement is necessary and urgent.
There are several potential organic materials that can be used as peat moss replacements, including coconut coir, rice hull, and wood bark. In addition to these materials, recently, biochar has received attention as a superb peat moss alternative with many advantages. Since it has been long discovered in the amazon rainforest as terra preta (black soil), biochar has been evaluated and studied from researchers in the past two decades (Denevan and Woods, 2004). Biochar is a carbon-rich material with porous structure produced by the thermo-chemical decomposition of biomass in an oxygen depleted or oxygen-limited atmosphere (Demirbas and Arin, 2002; Lehmann, 2007; Nartey and Zhao, 2014). Data from the literature were obtained from web of science database from 2010 to 2023 with searching terms such as “biochar”, “biochar container substrate”, “biochar environment”, “biochar peat moss” etc. The number of biochar-related publications increased from 76 to 3,6474 in the past two decades (Figure 1.), with its main applications being soil amendments (Lehmann et al., 2011), pollutant removal (Ahmad et al., 2014), beneficial bacterial carrier (Belonogova et al., 2018), and mitigate climate change (Woolf et al., 2010). Most of studies were either focused on increasing crop growth or reducing non-peat moss related environmental concerns such as carbon sequestration, contaminants remediation, greenhouse gas emission reduction (Das et al., 2020; Bolan et al., 2022a; Bolan et al., 2022b). There were decent number of studies concentrated on biochar production, characterization and engineering (Albert et al., 2021; Basak et al., 2022; Bolan et al., 2023). Among these 36,474 publications, 1457 focused on using biochar as a container substrate, and only 68 were used to replace peat moss as a container substrate component.
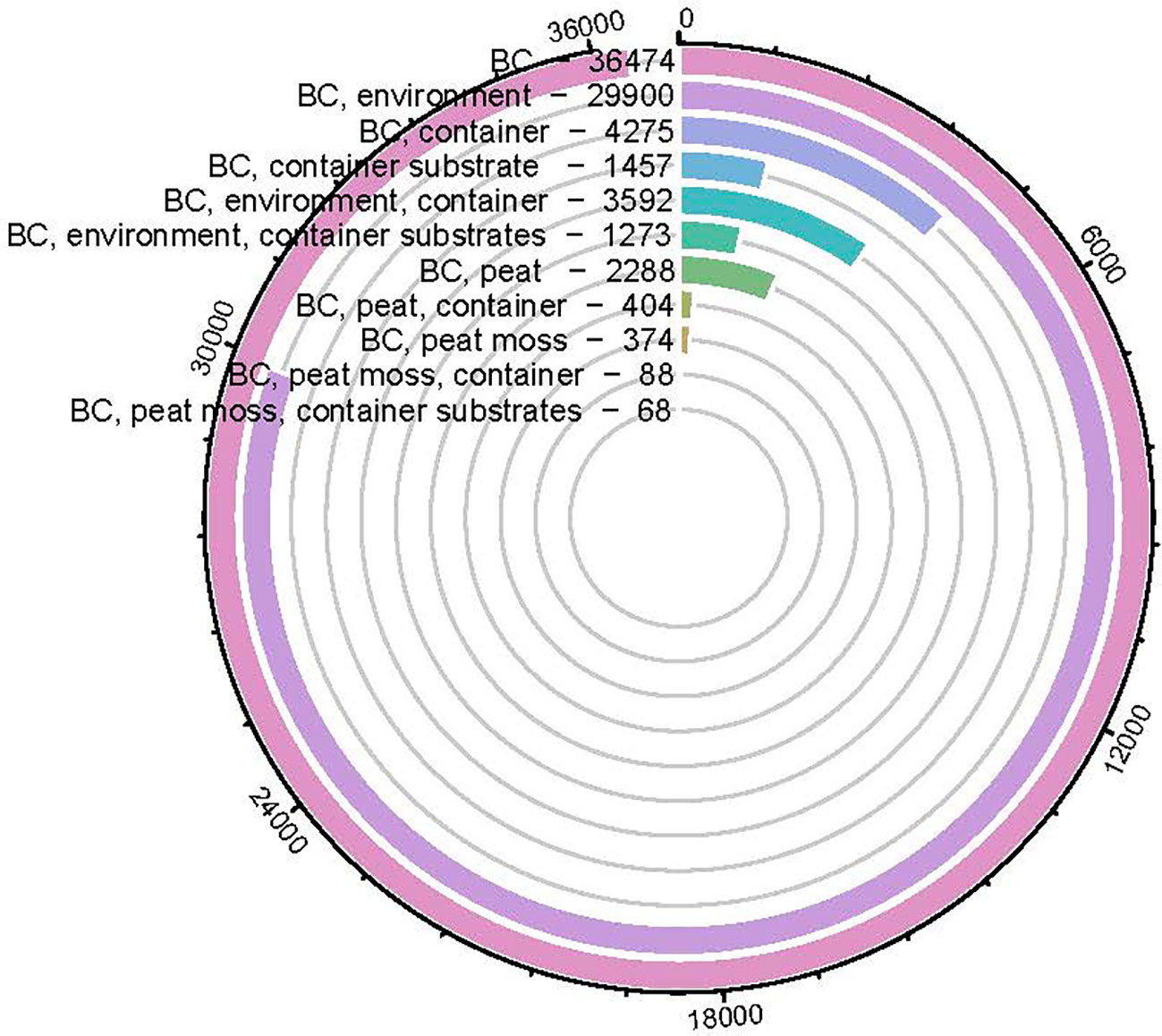
Figure 1 Circular bar-plot indicating the number of biochar (BC)-related articles published from 2010~2023 based on key words searching in Science Direct database.
Based on the existing information, using biochar as a container substrate holds immense potential to offer substantial environmental and economic benefits for various compelling reasons. Unlike peat moss, which needs a long time to regenerate, biochar is considered as a renewable material since it can be derived from various and fast generating feedstocks (Yan et al., 2020), ranging from plant-based material such as green waste (Tian et al., 2012), wood (Vaughn et al., 2015; Gascó et al., 2018; Fascella et al., 2020; Ferlito et al., 2020), straw (Spokas et al., 2009; Spokas et al., 2010; Vaughn et al., 2013; Hansen et al., 2015; Hansen et al., 2016), bark (Hina et al., 2010), rice hull (Locke et al., 2013), wheat straw (Vaughn et al., 2013; Xu et al., 2016; Xu et al., 2016) to other sources such as deinking sludge (Méndez et al., 2015; Méndez et al., 2017). For the same reason, biochar has price advantages over peat moss, especially when biochar is made from feedstocks from local industries and farms (Yan et al., 2020). Using biochar as a peat moss replacement protects peatland from further drainage for peat moss harvesting, thus protecting peatland ecosystems and reducing GHGs emissions (Hao et al., 2010; Ro et al., 2010; Cornelissen et al., 2013; Conversa et al., 2015). Moreover, producing straw biochar and adding it into agriculture production can directly reduce CO2 emission by 47% and 57% for rice and maize, respectively (18,479.35–37,457.66 kg) and reduce CH4 and N2O emission (Ji et al., 2018; Xu et al., 2018). Biochar could increase water and nutrient use efficiency, reduce fertilizer and pesticide runoff, render equivalent plant yield, thus providing both environmental and economic benefits (Guo et al., 2018a; Huang et al., 2019a; Yan et al., 2020; Yu et al., 2020a).
As such, this article discussed the use of biochar to replace peat moss as a container substrate to alleviate environmental issues by collecting exponentially increased number of publications and reviewing them to explain how the properties of biochar make it a viable alternative to peat moss, how biochar helps in reducing fertilizer pollution and the leaching of nutrients, how it addresses issues related to peatland disturbance, and how it provides potential economic benefits. This article also provides new insights into the research gap, state-of-the-art challenges of using biochar on a large scale and the possible solutions. The future research directions of using biochar as a peat moss alternative was also discussed. The structures and key points for this study are: 1) biochar has huge potential to replace peat moss as a container substrate component; 2) biochar can provide environmental and economic benefits; 3) more actions need to be taken to use biochar in horticulture area in a large scale.
2 Peat moss used as a container substrate
2.1 Properties of peat moss
Peat moss has long been widely used a container substrate due to its suitable properties, which allows it to support plants, hold nutrients, retain water, and change gases (Yeager et al., 2007; Nelson, 2012). Despite its suitable properties, peat moss could have rewetting and leaching issues (Gaudig et al., 2017; Kumar, 2017). The drying process during commercial peat moss production made it hydrophobic (Beardsell and Nichols, 1982; Gaudig et al., 2017; Kumar, 2017), and as an organic material, peat moss breaks down during greenhouse practices, which changes its hydrophobicity intensity and causes rewetting issues (Valat et al., 1991; Dekker and Ritsema, 2000). Especially after dried out, when the moisture content decreases below 20%, peat moss requires a longer time to rewet as it becomes more hydrophobic (Michel et al., 2001). Additionally, peat moss-based substrate leads to more nutrient leaching than bark substrate, which may be due to its higher content of macropores (>50 nm, 11%) comparing to bark substrate (7%) (Drzal et al., 1997).
2.2 Environmental concerns caused by peat moss
Harvesting peat moss for container substrate from peatland has interfered peatland’s ecological functions (Leifeld and Menichetti, 2018). Peat moss harvesting reduced peatland C capacity, thus hindered its climate change mitigation capacity (Alexander et al., 2008). Also, harvesting peat moss disturbed N and CH4 cycles (James et al., 2021). Additionally, peatland disturbance may bring challenges to the native animals, making it harder for them to find new habitats, thus reduce ecosystem biodiversity (Alexander et al., 2008).
Besides interfering with peatland’s ecological functions, peat moss, as a container substrate component, also creates environmental concerns due to nutrient runoff as well as pesticide runoff (Michel et al., 2001; Kumar, 2017). In a common nursery production, a 15% leaching fraction was recommended to prevent the buildup of soluble salts in the container substrate (Cahn and Phillips, 2019). However, extensive irrigation, fertilizers, and pesticides were more often applied to containers to reduce the risk of crop failure (Savvas et al., 2013). Plants can only use 50% of nitrogenous fertilizers applied even under ideal conditions (Sönmez et al., 2008; Savci, 2012). The excessive nitrogen (N), phosphorus (P), and potassium (K) were lost through runoff, causing environmental concerns such as eutrophication, dead zones, and algal blooms (Power and Schepers, 1989; Zhu et al., 2004; Savci, 2012). Because of the low irrigation efficacy (80% of water runoff) in container production, highly soluble pesticides such as acephate, glyphosate, and mefenoxam are likely to dissolve and move with runoff water to a containment water body (Poudyal and Cregg, 2019). A 10-year survey of major streams and groundwater found that 97% of stream water and 61% of shallow groundwater near agricultural areas had one or more pesticides present (Stone et al., 2014).
2.3 Challenges of peat moss
Peat moss encounters production challenges as its volume and area have been largely reduced. The total volume and area of global peatlands have been decreased at a rate of 0.05% annually and by 10%~20% since 1800 owing to harvesting and land development (Temmink et al., 2017; Heck et al., 2021). Peat production was estimated to have decreased in 2019 in some peatland-rich countries (Temmink et al., 2017). Peatland area in Estonia has declined from 22% coverage of the country to only 5.5% for the past decade (Orru and Orru, 2006; Karofeld et al., 2020). In Ireland, around 84% of ombrotrophic peatlands (bogs) have been affected by peat extraction (Renou-Wilson et al., 2019). In Germany and Netherlands, 98% and 95% domestic peatland area have degraded due to the extensive peat moss harvesting (Barthelmes, 2016).
Peat moss also faces legislation challenges due to the implementation of peatland restoration projects and carbon neutral plans (Peng et al., 2018). Several European countries including Belarus, Ireland, and Sweden, were planning or implementing peatland restoration projects, reducing peat production across Europe in the future (Carlile and Coules, 2011). In Canada, among the total of 27, 615 ha peat moss production areas, more than 31% has been or is currently restored or reclaimed, with another 3% converted to other land-use (Shotyk, 1988). Also, the UK and Europe have legislated laws in order to protect the peatland from being over harvested (Alexander et al., 2008; Carlile and Coules, 2011). In 2019, Ireland announced its plan to stop all peat harvesting by 2028 (Brioche, 2020). In the same year, Finland announced its goal to become carbon neutral by 2035 by phasing out peat production (Brioche, 2020).
3 Biochar replacing peat moss as a container substrate
3.1 Biochar has suitable properties
Although biochar properties vary widely, many types of biochar could fall into the recommendation range either by itself or by combining with other components (Huang and Gu, 2019). Detailed biochar properties have been reported by Lan et al. (Huang and Gu, 2019), we summarized in Table 1 to compare several differences between biochar and peat moss-based substrates used in containers. For the most commonly used container substrate components such as peat moss and perlite, their total porosity was high, 83% and 92%, respectively, indicating low total porosity components need to be included to reach the ideal range (50-85%). As far as pH concerned, peat moss and vermicompost had a low pH lower than 5, 4.3-5 and 4.8, respectively, indicating that other alkaline components such as mixed hardwood biochar (pH 10.8-11.8) need to be incorporated to reach the ideal pH range (5.4-6.5) for container substrate (Table 1). For vermicompost and chicken manure, since their electricity conductivity and bulk density were high, 6.7 and 32.9 mS cm-1, 0.38 and 0.62 g cm-3 respectively, their amount needs to be considered carefully when adding them into container substrates. Pinewood biochar, mixed hardwood biochar, and sugarcane bagasse biochar used in our previous studies had similar total porosity (74~85%), air space (3~34%), and bulk density (0.09~0.17 g cm-3) to peat moss (83%, 19%, and 0.08 g cm-3, respectively) and peat moss-based commercial substrate (71~78%, 3~20%, and 0.11 g cm-3, respectively) (Guo et al., 2018b; Webber et al., 2018; Huang et al., 2019a; Yan et al., 2020; Yu et al., 2020b).
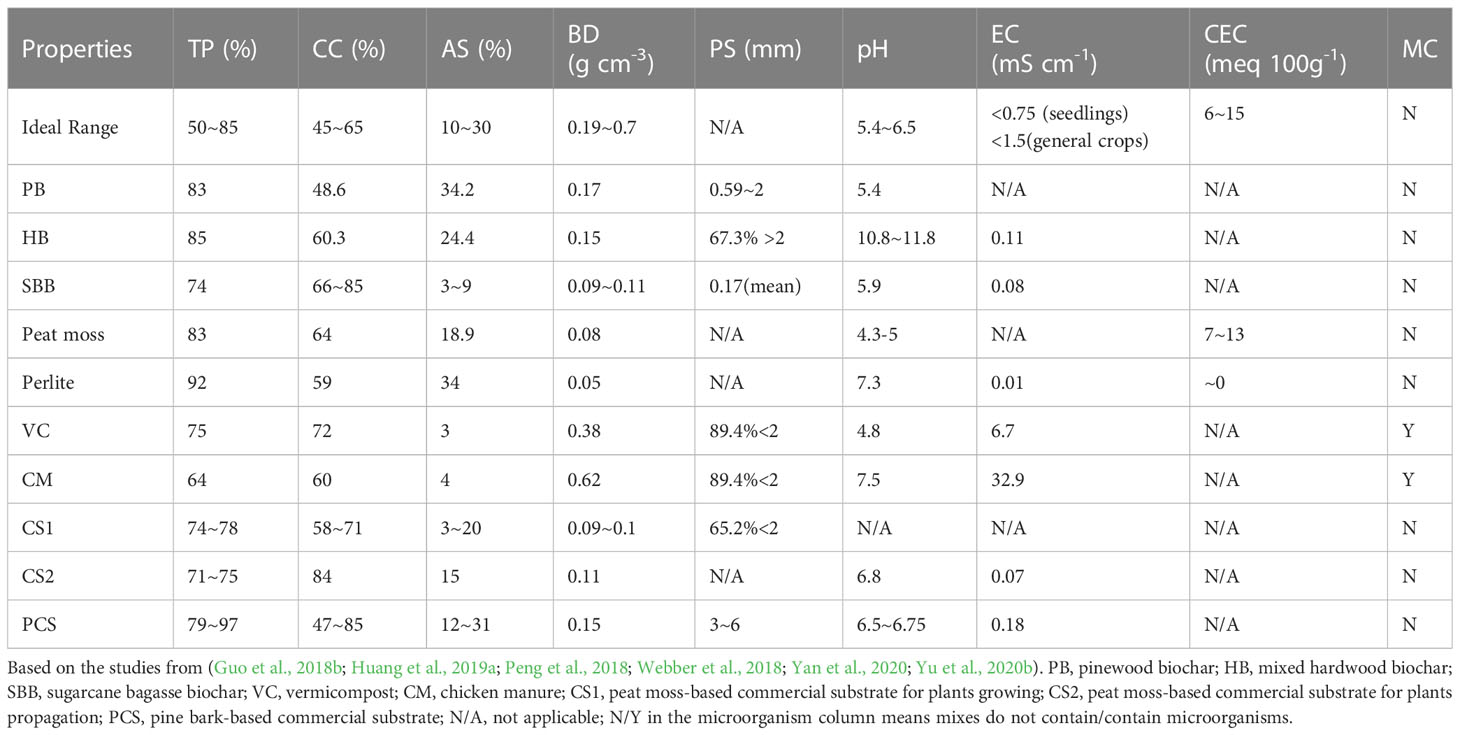
Table 1 The physical properties including total porosity (TP, %), container capacity (CC, %), air space (AS, %), bulk density (BD, g cm-3), and particle size (PS, mm); chemical properties including pH, electrical conductivity (EC, mS cm-1), cation exchange capacity (CEC, meq 100g-1) and biological properties (microorganisms, MC) of several types of biochar and peat moss-based commercial substrate from our previous studies.
Unlike peat moss, which may encounter rewetting difficulties, certain types of biochar used in containers are easy to rewet due to its larger surface areas and pore size distribution (Lehmann et al, 2011). Biochar made from organic materials at 400 ~1,200°C, has larger surface area than peat moss because its higher micropores content (Lee et al., 2015). The surface area of biochar increased because high temperatures changed more macropores into mesopores/micropores in biochar (Lee et al., 2015). Micropores contributed largely to biochar surface area, endowing high adsorptive capabilities on the biochar and allowing small dimension molecules, such as gases and solvents to be absorbed (Lehmann et al, 2011). Thus, when the same irrigation practice applied, biochar would encounter less difficulties in rewetting than peat moss or peat moss-based substrate (Drzal et al., 1997).
3.2 Biochar has benefits on nutrients supply and absorption
Biochar was proposed to be beneficial to plant nutrient absorption because it could provide nutrient resources depending on its feedstock and production method. Lin et al. mentioned that acacia saligna biochar produced from at 380°C and sawdust at 450°C contained 17.7 and 16.2% of humics (humic-like and fluvic-like materials), which can serve as biostimulant and be assimilated by plants (Lin et al., 2012; Ding et al., 2016). Similarly, biochar made from gasified rice hulls at 815 ~ 871°C could be used as P and K fertilizers as the 5.4 g (0.19 oz) biochar sample released 35.2 mg (0.0012 oz) P and 50.1 mg (0.0018 oz) K in water solution for container crops over a short production cycle of 6 weeks (Altland and Locke, 2013). Pine bark biochar produced from 450°C fast pyrolysis increased mint growth due to its high K and P contents (Yan et al., 2020).
Also, biochar benefits plant nutrient due to its various properties. Adding green waste biochar to the substrate decreased the available N, resulting from biochar’s porous structure induced N binding effects (Altland and Locke, 2012; Tian et al., 2012). Applying sugarcane bagasse biochar or mix hardwood biochar (pH 5.4 and 10.1 respectively) could adjust the substrate pH to around 6~8 (Yu et al., 2020b). The suitable substrate pH range (6~8) could promote K content, causing Mg and Ca deficiency due to the antagonism and/or synergism relationships among nutrients (Landis, 2005; Taiz and Zeiger, 2010).
3.3 Biochar effects on plant diseases
Soil-borne diseases affect potted plants’ marketability and are hard to control (Katan, 1997; Graber et al., 2014; Puertolas et al., 2018). There are 10~20% of attainable crop yields loss caused by soil-borne diseases and the economic losses in USA are more than $4 billion (Graber et al., 2014). Soil-borne diseases control becomes more challenging due to trade globalization (Daughtrey and Benson, 2005; Puertolas et al., 2018). For instance, Phytophthora ramorum has survived for eight months in root balls and potting substrates of rhododendron plants, affecting the plants marketability worldwide (Appiah et al, 2004; Vercauteren et al., 2013). Fusarium oxysporum f. sp papaveris, a fungi pathogen attacking Papaveraceae plants, largely affected Papaveraceae plants marketability in Italy (Bertetti et al., 2018).
As a container substrate to replace peat moss, the effects of biochar on soil-borne pathogen has been less reported than that of plant growth, which had positive, neutral, and negative effects (Huang and Gu, 2019; Yu et al, 2020b). To date, there aren’t enough studies about the biochar effect on plant health (Figure 2.), based on the Scientific Report database, among the 36,474 biochar publications (Figure 1.), only 3,997 were pathogen related, less than 11%. The majority of those pathogen studies were conducted in field, only 84 were conducted in containers. The dose of biochar is relatively low (ranging in most cases between 0.5~5%, Table 2) and most of the studies were conducted on edible crops such as tomato, pepper, strawberry, asparagus, lettuce, cucumber, beans etc (Copley et al., 2015; Mehari et al., 2015; Caroline et al., 2016; Jaiswal et al., 2017). The highest dose of biochar used in those studies is testing balsam fir bark and spruce bark biochar (475°C) on Pythium ultimum on sweet pepper, lettuce, basil, geranium and coriander at 50% (Gravel et al., 2013). Among those studies in Table 2, there were only two studies tested biochar effects on disease for ornamental crops which was red maple, red oak and geranium (Zwart and Kim, 2012; Gravel et al., 2013).
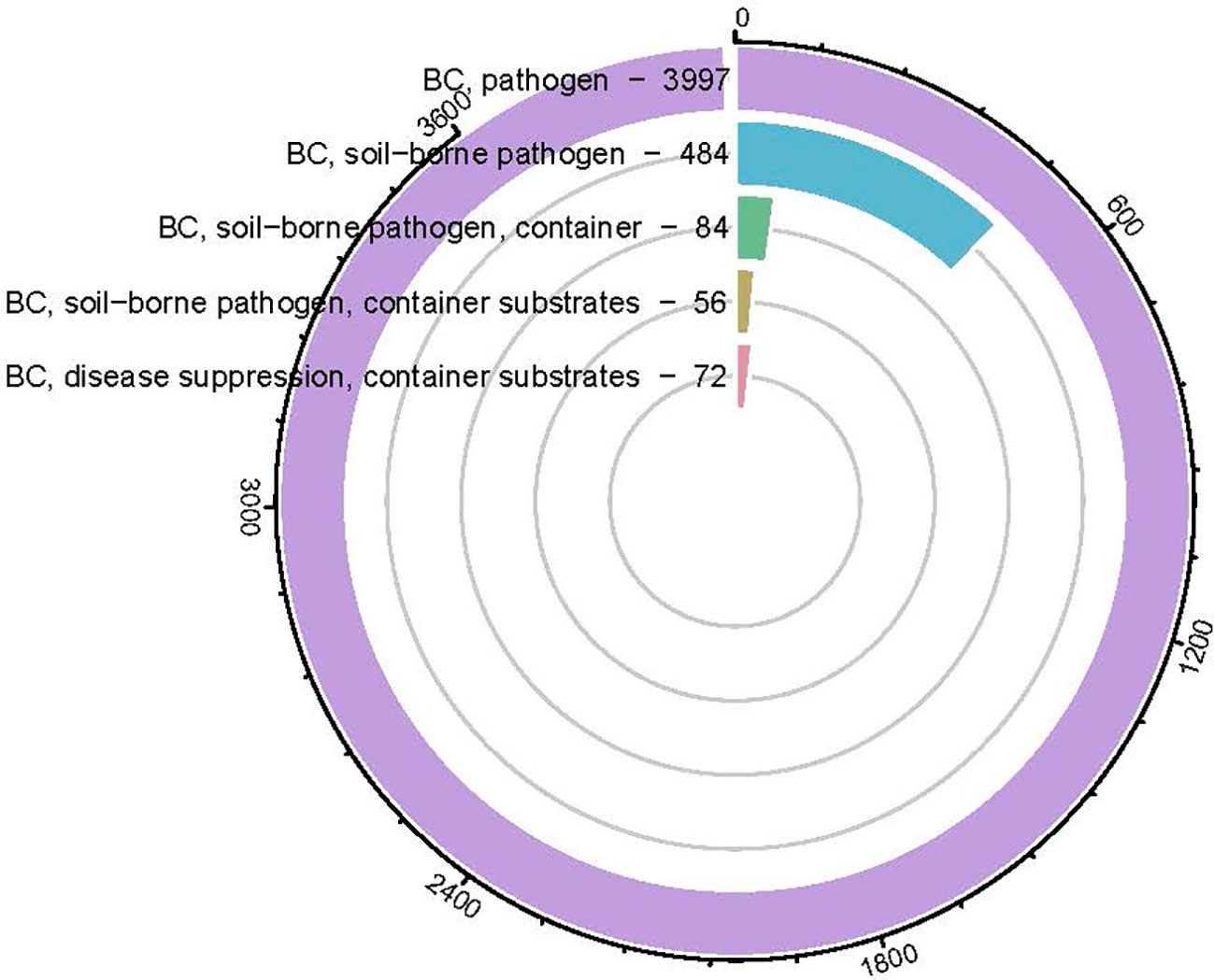
Figure 2 Circular bar-plot indicating the number of biochar (BC) pathogen-related articles published from 2010~2023 based on key words searching in Science Direct data base.
Similar to its effects on plant growth, biochar effects on plant health vary depending on plant species, biochar rates and types (Frenkel et al., 2017). Gravel et al. (2013) found that adding 50% of balsam fir/spruce bark biochar caused higher pathogen root colonization rate in all other crops except for coriander. Adding 30% coconut biochar increased plant health (Graber et al., 2014). Kadota and Niimi claimed that maple bark biochar improved the quality of several plant species, shortened the number of days needed for flowering, and increased plants survival rates (Kadota and Niimi, 2004). Adding 3% (w/w) wood-derived biochar with pre-conditioning such as pre-planting fertigation of the media reduced pre-emergence damping off caused by Pythium aphanidermatum by 71% for cucumber seedlings (Jaiswal et al., 2019). Incorporating biochar at rates of 10-30% (by vol.) increased strawberry fresh weight by 5-10% and reduced Phytophthora presents (Blok et al., 2019). Earthworm, microalgae biomass and 6% biochar mix increased tomato, pepper and eggplant seeds’ resistance for Pythium sp., increased germination rate by 34% (Alshehrei et al., 2021). Adding 20% and 50% of mixed hardwood biochar decreased poinsettia root rot disease caused Pythium aphanidermatum and pepper blight disease caused by Phytophthora capsica, respectively (Yu et al., 2021; Yu et al., 2023).
The potential mechanisms on how biochar may influence plant disease include both direct and indirect influence on pathogen: 1) biochars’ chemical compounds affect pathogen growth; 2) biochars’ physicochemical properties improve soil nutrients availability and abiotic conditions; 3) biochars’ physical properties help absorb toxins and enzymes produced by pathogens, reducing virulence; 4) biochars’ presence induces systemic resistance in host plants; 5) biochars’ physical properties enhance abundance and/or activities of beneficial microbes; 6) biochar induced disease suppression related gene expression (Graber et al., 2014; Bonanomi et al., 2015; Jaiswal et al., 2018; Jaiswal et al., 2020; Rasool et al., 2021; Ji et al., 2022; Liu et al., 2022).
4 Environmental benefits of biochar as a container substrate
4.1 Biochar protects peatland
The horticulture industry demands a large amount of peat moss as container substrates. Around 0.15 M m3 of peat moss were used in container plants production, accounting for 86.5% of the total imported peat moss in the United States (USDA-NASS, 2018). In the United Kingdom, 0.06 M m3 peat moss were used in horticulture, including container plants, bedding plants, vegetables, soft fruit, and cut flower production. In Europe, around 2.6 M m3 peat moss were used in horticulture, with the total ratio of peat in media for plant growth being 99% in Estonia, 99% in Lithuania, 92% in Latvia, 88% in Finland, 87% in Ireland, 87% in Denmark, 87 in Sweden, and 81% in Germany (Kitir et al., 2018).
Replacing peat moss with biochar protects peatland from further disturbance. The highest rate for biochar replacing peat moss as a container substrate is 80% with pine bark biochar (Guo et al., 2018b; Huang et al., 2019b). If 80% of peat moss can be replaced by pine bark biochar, 0.12 M m3, 0.05 M m3 and 2.08 M m3 peat moss can be saved annually in the United States, in the United Kingdom, and in Europe, respectively. Global average dry biomass Sphagnum production is around 260 g m-2 yr-1, depending on species and locations (Gunnarsson, 2005). Considering the commercial peat moss bulk density is 0.1 g cm-3, if 80% of peat moss substrate can be replaced by pine bark biochar, 46.2 M m2, 19.2 M m2, and 800 M m2 of peatland can be saved annually from being disturbed for the United States, the United Kingdom, and Europe, respectively.
4.2 Biochar reduces chemical leaching
4.2.1 Biochar reduces nutrient leaching
As aforementioned, fertilizer tends to be over-used in greenhouse/nursery production and plants can only use 50% of fertilizers applied (Sönmez et al., 2008; Savci, 2012). The rest of the other half of fertilizers were either lost in evaporation and/or reactions with organic compounds (Savci, 2012). Moreover, since the majority of fertilizers haven’t been absorbed by plants, they can reach ground water and contaminate ground water (Power and Schepers, 1989; Zhu et al., 2004).
Biochar replacing peat moss as a container substrate reduces nutrient runoff either by providing additional nutrient content or alternating substrates’ properties. Adding 15-20% gasified rice hull biochar (815 ~871 °C) in a peat-based substrate reduced nutrients such as NH4+, NO3-, H2PO4-, HPO42-, and K+ leaching as it provided sufficient potassium (K) amount for geranium and tomato plants growing in containers (Altland and Locke, 2017). Jahromi et al. (2018) found that switchgrass (1,000 °C) biochar-amended substrates reduced the total nutrients lost from hydrangea containers because biochar addition increased substrate water holding capacity. Altland and Locke (2013) demonstrated that adding 10% saw dust biochar to peat moss-based substrate increased nitrate and phosphate retention and subsequently reduced their leaching. Adding conifers wood biochar (500 °C) into container substrate for lavender production reduced K leaching as it increased K content of the growing substrates significantly (Fascella et al., 2020). Woodchip biochar (450~600 °C) decreased more extractable total N including NO3-N than peat moss substrates with similar seedlings growth (Prasad et al., 2018). Similarly, adding forest wood biochar (700 °C) at 7.5% with additional fertilizer reduced NO3-N, K and P leaching compared to the peat substrate. Adding fresh wood screening at 7.5% and 15% (500-600 °C) decreased NH4-N and K leaching compared to the peat substrate under both 1-fold and 1.5-fold fertilizer conditions (Chrysargyris et al., 2019).
4.2.2 Biochar decreases pesticides usage and leaching
The over-use of pesticides in greenhouse production also caused environmental concerns (Ayoub, 1999; Bolognesi, 2003). In the United States, among the total usage of pesticide, around 90% of pesticide comes from agricultural production (Atwood and Paisley-Jones, 2017). Pesticides contaminate the environment via surface runoff, spray drift, and subsurface flow, which is the major pathway for pesticides entering water bodies (Zhang et al., 2018). Leaching can rapidly transport pesticides to surface and subsurface receiving waters (Roseth and Haarstad, 2010). The best management practices are recommended for nurseries to reduce pesticide contamination, yet, the best management practices alone may not completely remove pesticides contamination (Grant et al., 2019).
Biochar has been reported as a good sorbent for efficient removal of chemicals, and its efficacy depends on many factors including biochar types, effect of time, adsorbent dosage, chemical concentration and pH. Taha et al. (2014) demonstrated that biochar made from corn stover and rice straw adsorbed many types of pesticides including organophosphates (diazinon and malathion) and neonicotinoids (imidacloprid and acetamiprid). Mandal et al. (2017) reported that rice straw biochar had the highest adsorption rate for atrazine and imidacloprid. Baharum et al. (2020) found that activated coconut fiber biochar (700°C) removed 98.96% and 87.93% of diazinon respectively when modified with phosphorus acid and sodium hydroxide at pH 7. Ponnam et al. (2020) described that biochar produced from the neem tree bark (300°C) provided a 95.2% desirability on removal Bentazone with response (adsorption uptake) of 79.40 mg/g, for initial concentration of insecticide (50 mg/L), adsorbent dosage (0.448 g), time 30.0 min and pH 2. Gámiz et al. (2019) demonstrated that aged oak wood biochar (550°C) had a significantly higher removal rate (>85%) of three highly persistent and ionizable pesticides (imazamox, picloram, terbuthylazine) than the fresh biochar (<16%).
5 Economic benefits of biochar as a container substrate
Biochar provides large potential economic values as the market of biochar and biochar supply companies are growing. According to the transparency market research (Doe, 2014; Natural-Resources, 2017), the evaluated worth of global biochar market reached $0.44 M in 2016, and it is expected to experience a Compound Annual Growth Rate of 14.5% from 2017 to 2025 and reach a valuation of $1.48 M by 2025. Also, the number of biochar supply companies increased. There were approximately 150 biochar supply companies in 2013, mostly of them were small garden and specialty retailers, however, the number of biochar companies doubled in 2015 (Cedergreen et al., 2009; Jirka and Tomlinson, 2015).
5.1 Biochar decreases peatland restoration costs
Peatland restoration requires high economic costs such as techniques costs, rewetting and recurring costs, as well as maintenance costs (Glenk and Martin-Ortega, 2018; Humpenöder et al., 2020; Karofeld et al., 2020). The costs associated with restoration range from $280 ha-1 to $14,016 ha-1 (Moxey and Moran, 2014). A one-time cost of $7,000 ha-1 for initial rewetting and recurring was estimated, with another cost of $200 ha-1 yr-1 maintenance and/or $140 ha-1 yr-1 management costs (Glenk and Martin-Ortega, 2018).
Replacing peat moss with biochar as a container substrate largely reduces peatland restoration costs because biochar production does not degrade the peatland ecosystem. With around 10.3 M ha peatland area needs to be restored (Humpenöder et al., 2020), an estimated $72.1 billion one-time rewetting and recurring costs with another $2.06 billion and/or $1.44 billion maintenance and management costs could be saved annually by replacing peat moss with biochar.
5.2 Biochar reduces substrate costs
Replacing peat moss with biochar as a container substrate can bring large economic benefits due to its potential low price and large demand. The average customer price for sphagnum peat increased from $ 22 m-3 in 1986 to $172 m-3 in 2018 (Yu et al., 1990; Bwi, 2018). Customers may have to pay higher prices based on the distributors they chose, for instance, the price of peat moss in Greenhouse Megastore is $ 310.7 m-3 (Megastore, 2019). Comparing to peat moss, however, the average biochar price is $100 m-3, half the price of peat moss from BWI, and one third the price of peat moss from Megastore. Aforementioned, 0.15 M m-3, 0.057 M m-3, and 2.6 M m-3 of peat moss were used in horticulture in the United States, United Kingdom, and Europe, respectively (Kitir et al., 2018; USDA-NASS, 2018). With 80% of biochar being able to replace peat moss as a container substrate (Guo et al., 2018b; Huang et al., 2019b), $8.64 M, $3.6 M, and $149.76 M can be saved annually in the United States, United Kingdom, and Europe, respectively if consumers get peat moss from a cheaper distributor. If consumers get peat moss from a more expensive distributor, $25.2 M, $10.5 M, $436.8 M can be saved annually in the United States, United Kingdom, and Europe, respectively. The actual economic benefits of using biochar to replace peat moss as a container substrate could be even larger if biochar were produced locally, which may lead to an even lower price than the average.
Also, using biochar to replace peat moss as a container substrate brings large economic benefits due to several reasons (Table 3). Firstly, peat moss needs a specific condition to growth such as waterlogged, acidic and anaerobic areas while biochar material can be grown anywhere. Secondly, peat moss regrowth rate ranges from 30-40% while biochar materials can reach to100%. Moreover, the price for commercially available peat moss is around $172 m-3, if been purchased from wholesale such as BWI, 72% higher than that of biochar. Additionally, peat moss can only be harvested when the depth is more than 2m while biochar materials can be harvest or collected anytime. Peat requires thousands of years to be generated, making it a unrenewable resource (Hugron et al., 2013). With the restoration practices, the average rate of peat moss vertical growth was around 1 mm year-1 in the peatland (Savichev et al., 2020). If no restoration practices are launched, the spontaneous revegetation of abandoned peatlands will take even longer (Karofeld et al., 2020). The best suggested harvesting depth for peat moss is 0.25 m from the top soil, meaning after harvesting, peatland needs 25 years or even longer to be able to harvest again (Savichev et al., 2020). The 25 years are more than enough to grow pine trees to merchantable size for biochar production (Butler et al., 2017; Guo et al., 2018b). If we grow other biomass such as sugarcane (or other herbs), miscanthus, and shrubs, the generation of biochar can be 25 times faster than peat moss, providing 25 times the economic benefits of peat moss (Webber et al., 2018; Roy et al., 2020).
5.3 Biochar reduces chemical costs
Chemical costs in agriculture are high due to large demands and high prices. Global fertilizer demands were projected to 208 M tons with the United States consuming 22 M tons in 2015 at an average price $719 ton-1 (Baanante and I.F.P.R. Insitute, 1996; Schnitkey, 2017; EPA, 2019). Global pesticides use in agriculture was 4.12 M tons with USA using 408,000 tons, with the trade reached approximately 5.9 M tons valuing $37.6 billion in 2018 (FAO, 2020). The United States was the top five countries for pesticides imports with trade values ranging $1.4~3.0 billion in 2018 (Wanner et al, 2020).
Replacing peat moss with biochar as a container substrate significantly reduces chemical costs by adding extra nutrients, increasing nutrient use efficiency, and reducing disease incidence. Biochar produced from nutrient-rich raw materials could serve as a source of P and K, reducing the total amount of fertilizer needed for plant growth (Huang et al., 2019a). If using biochar could increase nutrient use efficiency by 50% (Jahromi et al., 2018), $7.91 billion can be saved in the United States, and $74.78 billion worldwide (assuming the average price was $719 ton-1) (EPA, 2019). Also, mixed hardwood biochar used in our previous study could reduce 25% disease incidence, leading to less pesticide consumption (Unpublished Data). If using biochar could reduce pesticide usage by 25%, $9.4 billion could be saved globally.
5.4 Biochar decrease agricultural waste handling costs
Large amounts of agricultural waste contributed to high waste handling costs. Around 3.9 billion tons of waste were generated annually worldwide with 2.01 billion tons (expected to grow to 3.4 billion tons by 2050) being municipal solid waste (North America contributed 289 M tons) (Kaza et al, 2018). The operating costs for integrated municipal solid waste management, including collection, transport, treatment, and disposal, generally exceed $100 ton-1 yr-1 (USDA-EPA, 1997).
Using biochar to replace peat moss as a container substrate could significantly reduce agricultural waste handling costs. With pyrolysis for bio-oil purposes, the yield of biochar is from 20%~47% (Ok et al., 2015) (taking the average as 30%). To produce enough biochar for the horticulture industry in USA alone (0.15 M m3), assuming all the wastes have similar density as municipal waste, 350 kg m-3(USDA, 2008), nearly 0.18 M tons of agricultural waste can be converted, saving $18 M yr-1. Similarly, to produce enough biochar for horticulture industry in United Kingdom (0.057 M m3), and Europe (2.6 M m3), 0.67 M tons, 3.03 M tons of agricultural waste can be converted, respectively, saving millions of dollars on agricultural waste handling.
6 Limitations and possible solutions for biochar as a container substrate
Using biochar as a replacement for peat moss as a container substrate provides many benefits, yet it has several limitations. Biochar limitations are mainly from the varied properties and potential toxic substances it may contain, the non-continuous biochar supply-demand loop, and the lack of awareness and production practice of using it as container substrates (Huang and Gu, 2019). Although the number of biochar literature has increased dramatically, there is still little awareness of biochar application among modern farmers (Wu et al., 2017). These limitations may be addressed by providing finically and nonfinancial policy support to motivate business practice change, improving biochar commercial availability, to educate consumers, extending biochar demand, and to establish good production and application practice, exploring more biochar application options (Pourhashem et al., 2019).
6.1 Biochar various properties and production
Unlike the well-established sphagnum peat moss, biochar properties vary widely depending on feedstocks, production temperature, and pre- and post-treatment, bringing application difficulties for consumers (Huang and Gu, 2019). Biochar may contain potential toxic compounds such as heavy metals, polycyclic aromatic hydrocarbons (PAHs) and dioxin depending on the raw material and producing conditions (Shackley et al., 2010). When incorporating biochar with heavy metals, PAHs and dioxin into container substrates, plant growth could be decreased.
Biochar’s various properties could be addressed by implementing standard production practices such as using the same feedstock and temperature every time. Currently, most biochar is produced as a by-product from bio-oil-focused process, leading to various properties and toxic compounds (Huang and Gu, 2019; Yu et al., 2020a). Also, biochar made from feedstocks containing toxic compounds, either heavy metal, PAHs or chlorine could contain toxic compounds (Huang and Gu, 2019). As such, businesses can avoid producing toxic containing biochar by selecting feedstock material cautiously. Additionally, biochar various properties can be adjusted to an ideal range for container plants growth by incorporating other components such as bark, perlite, and peat (Guo et al., 2018b).
6.2 Biochar non-continuous supply-demand loop
Biochar supply and demand have not created a full loop for the industry yet. Consumers are reluctant to switch from peat moss to biochar due to their lack of awareness and poor biochar availability. Because of the unawareness of using biochar as container substrates, consumers tend to use the well-established and well-supplied peat moss as a major container substrate component, lowering biochar demand. In return, the low biochar demand discourages biochar producing companies due to the low financial benefits. Currently, there are only around 300 biochar companies worldwide, and most of them are small-scale companies, not being able to supply commercial biochar sustainably (Jirka and Tomlinson, 2015). Also, due to the lack of financial motivation, companies are not able to invest in biochar facilities, producing large-scale of container substrate-targeted/grade biochar (Pourhashem et al., 2019).
The non-continuous biochar supply-demand loop can be addressed by establishing related policies to encourage capital investment, providing technology support to reduce the initial production costs (Pourhashem et al., 2019). Academic world needs to pay more attention to the profitability of biochar application in their work (Maroušek et al., 2019). Also, non-financial programs, including extension programs can help educate consumers on biochar economic and environmental benefits and biochar application practices, increasing biochar demand. Additionally, more funding needs to be assigned to biochar research and development programs, exploring more biochar application options to enlarge biochar market margin.
7 Conclusions
As summarized in Figure 3, using biochar to replace peat moss as a container substrate for plant production provides an environmentally friendly way to address the environmental concerns associated with peatland mining and drainage, and additionally yields multiple benefits. Switching peat moss to biochar as a container substrate for plant production protects peatland ecosystem, increases water and fertilizer use efficiency, reduces greenhouse gas emission, and brings economic benefits. However, to reach biochar’s full potential, biochar limitations such as the lack of awareness, potential toxic compounds, and the non-continuous supply-demand loop need to be addressed soon by establishing both financial and non-financial supports from governments, companies, and research agencies.
Specifically, many container studies have been published on using biochar as an alternative for peat moss, however, most of the studies focused on crop production and the effect of biochar on disease control needs to be explored more. Studies testing the effect of the combination of bio-stimulants and biochar need to be explored for horticulture production. More molecular and physiology studies need to be included to enhance biochar application in horticulture. Also, nano-form of biochar products need to be developed and explored in horticulture. With many studies concentrated on edible crops, testing different biochar sources especially materials that may contain heavy metals such as sewage sledge and municipal waste is essential for safe food production. The facilities for biochar production needs high initial cost, preventing many companies from investing in biochar production, thus, appropriate technology for small to medium sized companies needs to be developed. Furthermore, the appropriate protocols that has been tested need to be shared to establish a uniform guideline for biochar production. Additionally, standardized biochar substrate mixes need to be commercialized for sustainable horticulture production. available, specifically for peat moss alternative growing substrate. In conclusion, using biochar in horticulture as a peat moss alternative can benefit environment economy significantly.
Author contributions
PY conducted the literature searching, collected, and analysed the data, and wrote the manuscript with the assistance of KQ, GN, and MG.
Conflict of interest
The authors declare that the research was conducted in the absence of any commercial or financial relationships that could be construed as a potential conflict of interest.
Publisher’s note
All claims expressed in this article are solely those of the authors and do not necessarily represent those of their affiliated organizations, or those of the publisher, the editors and the reviewers. Any product that may be evaluated in this article, or claim that may be made by its manufacturer, is not guaranteed or endorsed by the publisher.
References
Ahmad, M., Rajapaksha, A. U., Lim, J. E., Zhang, M., Bolan, N., Mohan, D., et al. (2014). Biochar as a sorbent for contaminant management in soil and water: a review. Chemosphere 99, 19–33. doi: 10.1016/j.chemosphere.2013.10.071
Albert, H. A., Li, X., Jeyakumar, P., Wei, L., Huang, L., Huang, Q., et al. (2021). Influence of biochar and soil properties on soil and plant tissue concentrations of Cd and Pb: A meta-analysis. Sci. Total Environ. 755, 142582. doi: 10.1016/j.scitotenv.2020.142582
Alexander, P., Bragg, N., Meade, R., Padelopoulos, G., Watts, O. (2008). Peat in horticulture and conservation: the UK response to a changing world. Mires Peat 3, 1–8.
Alshehrei, F., Al-Enazi, N. M., Ameen, F. (2021). Vermicomposting amended with microalgal biomass and biochar produce phytopathogen-resistant seedbeds for vegetables. Biomass Conversion Biorefinery, 1–8. doi: 10.1007/s13399-021-01770-w
Altland, J. E., Locke, J. C. (2012). Biochar affects macronutrient leaching from a soilless substrate. HortScience 47, 1136–1140. doi: 10.21273/HORTSCI.47.8.1136
Altland, J. E., Locke, J. C. (2013). Gasified rice hull biochar is a source of phosphorus and potassium for container-grown plants. J. Environ. Horticulture 31, 138–144. doi: 10.24266/0738-2898.31.3.138
Altland, J. E., Locke, J. C. (2017). High rates of gasified rice hull biochar affect geranium and tomato growth in a soilless substrate. J. Plant Nutr. 40, 1816–1828. doi: 10.1080/01904167.2016.1249800
Appiah, A., Jennings., P., Turner, J. (2004). Phytophthora ramorum: One pathogen and many diseases, an emerging threat to forest ecosystems and ornamental plant life. Mycologist 18, 145–150. doi: 10.1017/S0269915X04004136
Atwood, D., Paisley-Jones, C. (2017). “Pesticide and industry sales and usage 2008–2012 market estimates,”. Biological and Economic Analysis Division, Office of Pesticide Programs, Office of Chemical Safety and Pollution Prevention, US Environmental Protection Agency (Washington, DC: U.S.E.P. Agency).
Ayoub, A. T. (1999). Fertilizers and the environment. Nutrient Cycling Agroecosystems 55, 117–121. doi: 10.1023/A:1009808118692
Baharum, N. A., Nasir, H. M., Ishak, M. Y., Isa, N. M., Hassan, M. A., Aris, A. Z. (2020). Highly efficient removal of diazinon pesticide from aqueous solutions by using coconut shell-modified biochar. Arabian J. Chem. 13 (7), 6106–6121. doi: 10.1016/j.arabjc.2020.05.011
Barthelmes, A. (2016). “The global potential and perspectives for paludiculture. Paludiculture-Productive Use of Wet Weatlands: Climate Protection, Biodiversity, Regional Economic Benefits,”. Eds. Wichtmann, W., Schröder, C., Joosten, H. (Stuttgart: Schweizerbart Science Publishers), 200–203.
Basak, B. B., Sarkar, B., Saha, A., Sarkar, A., Mandal, S., Biswas, J. K., et al. (2022). Revamping highly weathered soils in the tropics with biochar application: What we know and what is needed. Sci. Total Environ. 822, 153461. doi: 10.1016/j.scitotenv.2022.153461
Beardsell, D., Nichols, D. (1982). Wetting properties of dried-out nursery container media. Scientia Hortic. 17, 49–59. doi: 10.1016/0304-4238(82)90061-9
Belonogova, N., Rudakova, M., Vasserman, D., Galitskaya, P., Selivanovskaya, S. (2018). Biochar as a potential carrier for agricultural beneficial microbes. Int. Multidiscip. Sci. GeoConference: SGEM: Surveying Geology Min. Ecol. Manage. 18, 145–150.
Bertetti, D., Gullino, M. L., Garibaldi, A. (2018). Susceptibility of some Papaveraceae plants to Fusarium oxysporum f. sp papaveris. J. Plant Dis. Prot. 125, 103–108. doi: 10.1007/s41348-017-0095-7
Blok, C., Diaz, A., Oud, N., Streminska, M., Huisman, M., Khanh, P., et al. (2019). “Biochar as a carrier: Trichoderma harzianum on biochar to promote disease suppression in strawberry (No. WPR-893),” (BU Greenhouse Horticulture: Wageningen University & Research).
Bolan, N., Hoang, S. A., Beiyuan, J., Gupta, S., Hou, D., Karakoti, A., et al. (2022a). Multifunctional applications of biochar beyond carbon storage. Int. Materials Rev. 67 (2), 150–200. doi: 10.1080/09506608.2021.1922047
Bolan, S., Hou, D., Wang, L., Hale, L., Egamberdieva, D., Tammeorg, P., et al. (2023). The potential of biochar as a microbial carrier for agricultural and environmental applications. Sci. Total Environ. 886, 163968. doi: 10.1016/j.scitotenv.2023.163968
Bolan, N., Sarmah, A. K., Bordoloi, S., Bolan, S., Padhye, L., Van Zwieten, L., et al. (2022b). Soil acidification and the liming potential of biochar. Environ. pollut. 317 (120632), 120632. doi: 10.1016/j.envpol.2022.120632
Bolognesi, C. (2003). Genotoxicity of pesticides: a review of human biomonitoring studies. Mutat. Research/Reviews Mutat. Res. 543, 251–272. doi: 10.1016/S1383-5742(03)00015-2
Bonanomi, G., Ippolito, F., Scala, F. (2015). A" black" future for plant pathology? Biochar as a new soil amendment for controlling plant diseases. J. Plant Pathol. 97 (2), 223–234.
Brioche, A. S. (2020). “PEAT,” in U. United states geological survey (ed.), mineral commodity summaries (Reston, VA: National Minerals Information Center), 118–119.
Butler, M. A., Dahlen, J., Eberhardt, T. L., Montes, C., Antony, F., Daniels, R. F. (2017). Acoustic evaluation of loblolly pine tree-and lumber-length logs allows for segregation of lumber modulus of elasticity, not for modulus of rupture. Ann. For. Sci. 74, 20. doi: 10.1007/s13595-016-0615-9
Bwi, C. (2018). Available at: https://www.bwicompanies.com/ (Accessed 03, 31 2018).
Cahn, M. D., Phillips, B. (2019). “Best management practices for mitigating pesticides in runoff from vegetable systems in California Pesticides in surface water: monitoring, modeling, risk assessment, and management,” (ACS Publications), 519–539.
Carlile, B., Coules, A. (2011). Towards sustainability in growing media. In International Symposium on Growing Media, Composting and Substrate Analysis 1013, 341–349.
Caroline, A., Debode, J., Vandecasteele, B., D’Hose, T., Cremelie, P., Haegeman, A., et al. (2016). Biological, physicochemical and plant health responses in lettuce and strawberry in soil or peat amended with biochar. Appl. Soil Ecol. 107, 1–12. doi: 10.1016/j.apsoil.2016.05.001
Cedergreen, N., Felby, C., Porter, J. R., Streibig, J. C. (2009). Chemical stress can increase crop yield. Field Crops Res. 114, 54–57. doi: 10.1016/j.fcr.2009.07.003
Chrysargyris, A., Prasad, M., Kavanagh, A., Tzortzakis, N. (2019). Biochar type and ratio as a peat additive/partial peat replacement in growing media for cabbage seedling production. Agronomy 9 (11), 693. doi: 10.3390/agronomy9110693
Conversa, G., Bonasia, A., Lazzizera, C., Elia, A. (2015). Influence of biochar, mycorrhizal inoculation, and fertilizer rate on growth and flowering of Pelargonium (Pelargonium zonale L.) plants. Front. Plant Sci. 6. doi: 10.3389/fpls.2015.00429
Copley, T. R., Aliferis, K. A., Jabaji, S. (2015). Maple bark biochar affects Rhizoctonia solani metabolism and increases damping-off severity. Phytopathology 105, 1334–1346. doi: 10.1094/PHYTO-08-14-0231-R
Cornelissen, G., Martinsen, V., Shitumbanuma, V., Alling, V., Breedveld, G., Rutherford, D., et al. (2013). Biochar effect on maize yield and soil characteristics in five conservation farming sites in zambia. Agronomy 3, 256–274. doi: 10.3390/agronomy3020256
Dargie, G. C., Lewis, S. L., Lawson, I. T., Mitchard, E. T., Page, S. E., Bocko, Y. E., et al. (2017). Age, extent and carbon storage of the central Congo Basin peatland complex. Nature 542, 86–90. doi: 10.1038/nature21048
Das, S. K., Ghosh, G. K., Avasthe, R. (2020). Biochar application for environmental management and toxic pollutant remediation. Biomass Convers. Biorefinery 2020 (13), 5585–556. doi: 10.1007/s13399-020-01078-1
Daughtrey, M. L., Benson, D. M. (2005). Principles of plant health management for ornamental plants. Annu. Rev. Phytopathol. 43, 141–169. doi: 10.1146/annurev.phyto.43.040204.140007
Dekker, L., Ritsema, C. (2000). Wetting patterns and moisture variability in water repellent Dutch soils. J. Hydrology 231, 148–164. doi: 10.1016/S0022-1694(00)00191-8
Demirbas, A., Arin, G. (2002). An overview of biomass pyrolysis. Energy sources 24, 471–482. doi: 10.1080/00908310252889979
Denevan, W., Woods, W. I. (2004). Discovery and awareness of anthropogenic Amazonian dark earths (terra preta). Energy and Agricultural Carbon Utilization: Sustainable Alternatives to Sequestration. (Athens, GA (USA): University of Georgia), 10–11.
Ding, Y., Liu, Y., Liu, S., Li, Z., Tan, X., Huang, X., et al. (2016). Biochar to improve soil fertility. A review. Agron. Sustain. Dev. 36, 1–18. doi: 10.1007/s13593-016-0372-z
Doe, J. (2014). Commercial satellite imaging market-global industry analysis, size, share, growth, trends, and forecast, 2013-2019,Transparency Market Research 1.
Drzal, M., Cassel, D.K., Fonteno, W. (1997). Pore fraction analysis: A new tool for substrate testing. In International Symposium on Growing Media and Hydroponics 481 (pp. 43–54).
Duan, M., Li, H., Gu, J., Tuo, X., Sun, W., Qian, X., et al. (2017). Effects of biochar on reducing the abundance of oxytetracycline, antibiotic resistance genes, and human pathogenic bacteria in soil and lettuce. Environ. pollut. 224, 787–795. doi: 10.1016/j.envpol.2017.01.021
Elad, Y., David, D. R., Harel, Y. M., Borenshtein, M., Kalifa, H. B., Silber, A., et al. (2010). Induction of systemic resistance in plants by biochar, a soil-applied carbon sequestering agent. Phytopathology 100, 913–921. doi: 10.1094/PHYTO-100-9-0913
Elmer, W. H., Pignatello, J. J. (2011). Effect of biochar amendments on mycorrhizal associations and Fusarium crown and root rot of asparagus in replant soils. Plant Dis. 95, 960–966. doi: 10.1094/PDIS-10-10-0741
EPA (2019). “Agricultural fertilizer,” in E.R.S. Unied state department of agriculture (United States Environmental Protection Agency).
Fascella, G., D’Angiolillo, F., Ruberto, G., Napoli, E. (2020). Agronomic performance, essential oils and hydrodistillation wastewaters of Lavandula angustifolia grown on biochar-based substrates. Ind. Crops Products 154, 112733. doi: 10.1016/j.indcrop.2020.112733
Ferlito, F., Torrisi, B., Allegra, M., Stagno, F., Caruso, P., Fascella, G. (2020). Evaluation of conifer wood biochar as growing media component for citrus nursery. Appl. Sci. 10, 1618. doi: 10.3390/app10051618
Frenkel, O., Jaiswal, A. K., Elad, Y., Lew, B., Kammann, C., Graber, E. R. (2017). The effect of biochar on plant diseases: what should we learn while designing biochar substrates? J. Environ. Eng. Landscape Manage. 25, 105–113. doi: 10.3846/16486897.2017.1307202
Gámiz, B., Velarde, P., Spokas, K. A., Celis, R., Cox, L. (2019). Changes in sorption and bioavailability of herbicides in soil amended with fresh and aged biochar. Geoderma 337, 341–349. doi: 10.1016/j.geoderma.2018.09.033
Gascó, G., Álvarez, M. L., Paz-Ferreiro, J., Miguel, M. S., Méndez, A. (2018). Valorization of biochars from pinewood gasification and municipal solid waste torrefaction as peat substitutes. Environ. Sci. pollut. Res. 25, 26461–26469. doi: 10.1007/s11356-018-2703-x
Gaudig, G., Krebs, M., Prager, A., Wichmann, S., Barney, M., Caporn, S., et al. (2017). Sphagnum farming from species selection to the production of growing media: a review. Mires Peat. 20, 1–30. doi: 10.19189/MaP.2018.OMB.340
George, C., Kohler, J., Rillig, M. C. (2016). Biochars reduce infection rates of the root-lesion nematode Pratylenchus penetrans and associated biomass loss in carrot. Soil Biol. Biochem. 95, 11–18. doi: 10.1016/j.soilbio.2015.12.003
Glenk, K., Martin-Ortega, J. (2018). The economics of peatland restoration. J. Environ. Economics Policy 7, 345–362. doi: 10.1080/21606544.2018.1434562
Graber, E., Frenkel, O., Jaiswal, A., Elad, Y. (2014). How may biochar influence severity of diseases caused by soilborne pathogens? Carbon Manage. 5, 169–183. doi: 10.1080/17583004.2014.913360
Grant, G. A., Fisher, P. R., Barrett, J. E., Wilson, P. C. (2019). Removal of agrichemicals from water using granular activated carbon filtration. Water Air Soil pollut. 230, 1–12. doi: 10.1007/s11270-018-4056-y
Gravel, V., Dorais, M., Ménard, C. (2013). Organic potted plants amended with biochar: its effect on growth and Pythium colonization. Can. J. Plant Sci. 93, 1217–1227. doi: 10.4141/cjps2013-315
Gunnarsson, U. (2005). Global patterns of Sphagnum productivity. J. Bryology 27, 269–279. doi: 10.1179/174328205X70029
Guo, Y., Niu, G., Starman, T., Gu, M. (2018a). Growth and development of Easter lily in response to container substrate with biochar. J. Hortic. Sci. Biotechnol. 94 (1), 1–7. doi: 10.3390/horticulturae4010001
Guo, Y., Niu, G., Starman, T., Volder, A., Gu, M. (2018b). Poinsettia growth and development response to container root substrate with biochar. Horticulturae 4, 1. doi: 10.3390/horticulturae4010001
Hansen, V., Hauggaard-Nielsen, H., Petersen, C. T., Mikkelsen, T. N., Müller-Stöver, D. (2016). Effects of gasification biochar on plant-available water capacity and plant growth in two contrasting soil types. Soil Tillage Res. 161, 1–9. doi: 10.1016/j.still.2016.03.002
Hansen, V., Müller-Stöver, D., Ahrenfeldt, J., Holm, J. K., Henriksen, U. B., Hauggaard-Nielsen, H. (2015). Gasification biochar as a valuable by-product for carbon sequestration and soil amendment. Biomass Bioenergy 72, 300–308. doi: 10.1016/j.biombioe.2014.10.013
Hao, W., Richardson, P., Hong, C. (2010). Foliar blight of annual vinca (Catharanthus roseus) caused by Phytophthora tropicalis in Virginia. Plant Dis. 94, 274–274. doi: 10.1094/PDIS-94-2-0274A
Harel, Y. M., Elad, Y., Rav-David, D., Borenstein, M., Shulchani, R., Lew, B., et al. (2012). Biochar mediates systemic response of strawberry to foliar fungal pathogens. Plant Soil 357, 245–257. doi: 10.1007/s11104-012-1129-3
Heck, M. A., Lüth, V. M., van Gessel, N., Krebs, M., Kohl, M., Prager, A., et al. (2021). Axenic in vitro cultivation of 19 peat moss (Sphagnum L.) species as a resource for basic biology, biotechnology, and paludiculture. New Phytol. 229, 861–876. doi: 10.1111/nph.16922
Hina, K., Bishop, P., Arbestain, M. C., Calvelo-Pereira, R., Maciá-Agulló, J. A., Hindmarsh, J., et al. (2010). Producing biochars with enhanced surface activity through alkaline pretreatment of feedstocks. Soil Res. 48, 606–617. doi: 10.1071/SR10015
Huang, L., Gu, M. (2019). Effects of biochar on container substrate properties and growth of plants—A review. Horticulturae 5, 14. doi: 10.3390/horticulturae5010014
Huang, W.-k., Ji, H.-l., Gheysen, G., Debode, J., Kyndt, T. (2015). Biochar-amended potting medium reduces the susceptibility of rice to root-knot nematode infections. BMC Plant Biol. 15, 267. doi: 10.1186/s12870-015-0654-7
Huang, L., Niu, G., Feagley, S. E., Gu, M. (2019a). Evaluation of a hardwood biochar and two composts mixes as replacements for a peat-based commercial substrate. Ind. Crops Products 129, 549–560. doi: 10.1016/j.indcrop.2018.12.044
Huang, L., Yu, P., Gu, M. (2019b). Evaluation of biochar and compost mixes as substitutes to a commercial propagation mix. Appl. Sci. 9, 4394. doi: 10.3390/app9204394
Hugelius, G., Loisel, J., Chadburn, S., Jackson, R. B., Jones, M., MacDonald, G., et al. (2020). Large stocks of peatland carbon and nitrogen are vulnerable to permafrost thaw. Proc. Natl. Acad. Sci. 117, 20438–20446. doi: 10.1073/pnas.1916387117
Humpenöder, F., Karstens, K., Lotze-Campen, H., Leifeld, J., Menichetti, L., Barthelmes, A., et al. (2020). Peatland protection and restoration are key for climate change mitigation. Environ. Res. Lett. 15, 104093. doi: 10.1088/1748-9326/abae2a
Jahromi, N. B., Walker, F., Fulcher, A., Altland, J., Wright, W. C. (2018). Growth response, mineral nutrition, and water utilization of container-grown woody ornamentals grown in biochar-amended pine bark. HortScience 53, 347–353. doi: 10.21273/HORTSCI12643-17
Jaiswal, A. K., Alkan, N., Elad, Y., Sela, N., Philosoph, A. M., Graber, E. R., et al. (2020). Molecular insights into biochar-mediated plant growth promotion and systemic resistance in tomato against fusarium crown and root rot disease. Sci. Rep. 10, 13934. doi: 10.1038/s41598-020-70882-6
Jaiswal, A. K., Elad, Y., Graber, E. R., Frenkel, O. (2014). Rhizoctonia solani suppression and plant growth promotion in cucumber as affected by biochar pyrolysis temperature, feedstock and concentration. Soil Biol. Biochem. 69, 110–118. doi: 10.1016/j.soilbio.2013.10.051
Jaiswal, A. K., Elad, Y., Paudel, I., Graber, E. R., Cytryn, E., Frenkel, O. (2017). Linking the belowground microbial composition, diversity and activity to soilborne disease suppression and growth promotion of tomato amended with biochar. Sci. Rep. 7, 44382. doi: 10.1038/srep44382
Jaiswal, A. K., Frenkel, O., Elad, Y., Lew, B., Graber, E. R. (2015). Non-monotonic influence of biochar dose on bean seedling growth and susceptibility to Rhizoctonia solani: the “Shifted R max-Effect”. Plant Soil 395, 125–140. doi: 10.1007/s11104-014-2331-2
Jaiswal, A. K., Frenkel, O., Tsechansky, L., Elad, Y., Graber, E. R. (2018). Immobilization and deactivation of pathogenic enzymes and toxic metabolites by biochar: a possible mechanism involved in soilborne disease suppression. Soil Biol. Biochem. 121, 59–66. doi: 10.1016/j.soilbio.2018.03.001
Jaiswal, A. K., Graber, E. R., Elad, Y., Frenkel, O. (2019). Biochar as a management tool for soilborne diseases affecting early-stage nursery seedling production. Crop Protec 120, 34–42. doi: 10.1016/j.cropro.2019.02.014
James, A. R. S., Yavitt, J. B., Zinder, S. H., Richardson, R. E. (2021). Linking microbial Sphagnum degradation and acetate mineralization in acidic peat bogs: from global insights to a genome-centric case study. ISME J. 15, 293–303. doi: 10.1038/s41396-020-00782-0
Ji, C., Cheng, K., Nayak, D., Pan, G. (2018). Environmental and economic assessment of crop residue competitive utilization for biochar, briquette fuel and combined heat and power generation. J. Cleaner Production 192, 916–923. doi: 10.1016/j.jclepro.2018.05.026
Ji, C., Ye, R., Yin, Y., Sun, X., Ma, H., Gao, R. (2022). Reductive soil disinfestation with biochar amendment modified microbial community composition in soils under plastic greenhouse vegetable production. Soil. Tillage Res. 218, 105323. doi: 10.1016/j.still.2022.105323
Jirka, S., Tomlinson, T. (2015). State of the biochar industry 2014: A survey of commercial activity in the biochar sector. Int. Biochar Initiative.
Kadota, M., Niimi, Y. (2004). Effects of charcoal with pyroligneous acid and barnyard manure on bedding plants. Scientia Hortic. 101, 327–332. doi: 10.1016/j.scienta.2004.01.002
Karofeld, E., Kaasik, A., Vellak, K. (2020). Growth characteristics of three Sphagnum species in restored extracted peatland. Restor. Ecol. 28, 1574–1583. doi: 10.1111/rec.13245
Katan, J. (1997). “Soil disinfestation: environmental problems and solutions,” in Modern Agriculture and the Environment: Proceedings of an International Conference, held in Rehovot, Israel, 2–6 October 1994, under the auspices of the Faculty of Agriculture, the Hebrew University of Jerusalem. Eds. Rosen, D., Tel-Or, E., Hadar, Y., Chen, Y. (Dordrecht: Springer Netherlands), 41–45. doi: 10.1007/978-94-011-5418-5_4
Kaza, S. Y., Lisa, C., Bhada-Tata, P., Van Woerden, F. (2018). What a Waste 2.0 : A Global Snapshot of Solid Waste Management to 2050 (Washington, DC: World Bank.).
Kitir, N., Yildirim, E., Şahin, Ü., Turan, M., Ekinci, M., Ors, S., et al. (2018). Peat use in horticulturePeat. IntechOpen. Peat; Topcuoglu, B., Turan, M., Eds. (London, UK: IntechOpen) pp.75–90. doi: 10.5772/intechopen.79171
Kumar, S. (2017). Sphagnum moss as a growing media constituent: some effects of harvesting, processing and storage. Mires Peat 20 (7), 1–11. doi: 10.19189/MaP.2016.OMB.232
Lee, S.-J., Park, J. H., Ahn, Y.-T., Chung, J. W. (2015). Comparison of heavy metal adsorption by peat moss and peat moss-derived biochar produced under different carbonization conditions. Water Air Soil pollut. 226, 1–11. doi: 10.1007/s11270-014-2275-4
Lehmann, J., Rillig, M. C., Thies, J., Masiello, C. A., Hockaday, W. C., Crowley, D. (2011). Biochar effects on soil biota – A review. Soil Biol. Biochem. 43, 1812–1836. doi: 10.1016/j.soilbio.2011.04.022
Leifeld, J., Menichetti, L. (2018). The underappreciated potential of peatlands in global climate change mitigation strategies. Nat. Commun. 9, 1–7. doi: 10.1038/s41467-018-03406-6
Liebner, S., Zeyer, J., Wagner, D., Schubert, C., Pfeiffer, E. M., Knoblauch, C. (2011). Methane oxidation associated with submerged brown mosses reduces methane emissions from Siberian polygonal tundra. J. Ecol. 99, 914–922. doi: 10.1111/j.1365-2745.2011.01823.x
Lin, Y., Munroe, P., Joseph, S., Henderson, R., Ziolkowski, A. (2012). Water extractable organic carbon in untreated and chemical treated biochars. Chemosphere 87, 151–157. doi: 10.1016/j.chemosphere.2011.12.007
Liu, C., Xia, R., Tang, M., Chen, X., Zhong, B., Liu, X., et al. (2022). Improved ginseng production under continuous cropping through soil health reinforcement and rhizosphere microbial manipulation with biochar: a field study of panax ginseng from northeast China. Hortic. Res. 9, uhac108. doi: 10.1093/hr/uhac108
Locke, J. C., Altland, J. E., Ford, C. W. (2013). Gasified rice hull biochar affects nutrition and growth of horticultural crops in container substrates. J. Environ. horticulture 31, 195–202. doi: 10.24266/0738-2898.31.4.195
Mandal, A., Singh, N., Purakayastha, T. (2017). Characterization of pesticide sorption behaviour of slow pyrolysis biochars as low cost adsorbent for atrazine and imidacloprid removal. Sci. Total Environ. 577, 376–385. doi: 10.1016/j.scitotenv.2016.10.204
Maroušek, J., Strunecký, O.&, Stehel, V. (2019). Biochar farming: defining economically perspective applications. Clean Techn Environ. Policy 21, 1389–1395. doi: 10.1007/s10098-019-01728-7
Megastore, G. (2019). Available at: https://www.greenhousemegastore.com/search?q=peat+moss.
Mehari, Z. H., Elad, Y., Rav-David, D., Graber, E. R., Harel, Y. M. (2015). Induced systemic resistance in tomato (Solanum lycopersicum) against Botrytis cinerea by biochar amendment involves jasmonic acid signaling. Plant Soil 395, 31–44. doi: 10.1007/s11104-015-2445-1
Méndez, A., Paz-Ferreiro, J., Gil, E., Gascó, G. (2015). The effect of paper sludge and biochar addition on brown peat and coir based growing media properties. Scientia Hortic. 193, 225–230. doi: 10.1016/j.scienta.2015.07.032
Méndez, A., Paz-Ferreiro, J., Gil, E., Gascó, G. (2017). The effect of sewage sludge biochar on peat-based growing media. Biol. Agric. Hortic. 33 (1), 40–51. doi: 10.1080/01448765.2016.1185645
Michel, J. C., Rivière, L. M., Bellon-Fontaine, M. N. (2001). Measurement of the wettability of organic materials in relation to water content by the capillary rise method. Eur. J. Soil Sci. 52, 459–467. doi: 10.1046/j.1365-2389.2001.00392.x
Moxey, A., Moran, D. (2014). UK peatland restoration: Some economic arithmetic. Sci. total Environ. 484, 114–120. doi: 10.1016/j.scitotenv.2014.03.033
Nartey, O. D., Zhao, B. (2014). Biochar preparation, characterization, and adsorptive capacity and its effect on bioavailability of contaminants: an overview. Adv. Materials Sci. Eng 2014, 1–13. doi: 10.1155/2014/715398
Natural-Resources (2017) BIocahar market :Global industry Analysis, size, share, growth, trends and forecast 2017-2025. Available at: https://www.transparencymarketresearch.com/biochar-market.html.
Nature (2017). Peatlands and climate change (Gland, Switzerland: .U.f.C.o. Nature). Available at: https://www.iucn.org/resources/issues-briefs/peatlands-and-climate-change.
Nelson, P. (2012). Root substrate. Greenhouse operation and management. 7th ed (NJ: Prentice Hall, Upper Saddle River), 161–194.
Nerome, M., Toyota, K., Islam, T., Nishijima, T., Matsuoka, T., Sato, K., et al. (2005)Suppression of bacterial wilt of tomato by incorporation of municipal biowaste charcoal into soil. Soil Microorganisms (Japan).
Ok, Y. S., Uchimiya, S. M., Chang, S. X., Bolan, N. (2015). Biochar: Production, characterization, and applications (FL, USA: CRC press, Boca Raton).
Orru, H., Orru, M. (2006). Sources and distribution of trace elements in Estonian peat. Global planetary Change 53, 249–258. doi: 10.1016/j.gloplacha.2006.03.007
Peng, D. H., Gu, M. M., Zhao, Y., Yu, F., Choi, H. S. (2018). Effects of biochar mixes with peat-moss based substrates on growth and development of horticultural crops. Hortic. Sci. Technol. 36, 501–512. doi: 10.12972/kjhst.20180050
Ponnam, V., Katari, N. K., Mandapati, R. N., Nannapaneni, S., Tondepu, S., Jonnalagadda, S. B. (2020). Efficacy of biochar in removal of organic pesticide, Bentazone from watershed systems. J. Environ. Sci. Health Part B 55 (4), 396–405. doi: 10.1080/03601234.2019.1707008
Poudyal, S., Cregg, B. M. (2019). Irrigating nursery crops with recycled run-off: A review of the potential impact of pesticides on plant growth and physiology. HortTechnology 29, 716–729. doi: 10.21273/HORTTECH04302-19
Pourhashem, G., Hung, S. Y., Medlock, K. B., Masiello, C. A. (2019). Policy support for biochar: Review and recommendations. GCB Bioenergy 11, 364–380. doi: 10.1111/gcbb.12582
Power, J., Schepers, J. (1989). Nitrate contamination of groundwater in North America. Agriculture Ecosyst. Environ. 26, 165–187. doi: 10.1016/0167-8809(89)90012-1
Prasad, M., Tzortzakis, N., McDaniel, N. (2018). Chemical characterization of biochar and assessment of the nutrient dynamics by means of preliminary plant growth tests. J. Environ. Manage. 216, 89–95. doi: 10.1016/j.jenvman.2017.04.020
Puertolas, A., Boa, E., Bonants, P. J. M., Woodward, S. (2018). Survival of Phytophthora cinnamomi and Fusarium verticillioides in commercial potting substrates for ornamental plants. J. Phytopathol. 166, 484–493. doi: 10.1111/jph.12708
Rasool, M., Akhter, A., Haider, M. S. (2021). Molecular and biochemical insight into biochar and Bacillus subtilis induced defense in tomatoes against Alternaria solani. Sci. Hortic. 285, 110203. doi: 10.1016/j.scienta.2021.110203
Renou-Wilson, F., Moser, G., Fallon, D., Farrell, C., Müller, C., Wilson, D. (2019). Rewetting degraded peatlands for climate and biodiversity benefits: Results from two raised bogs. Ecol. Eng. 127, 547–560. doi: 10.1016/j.ecoleng.2018.02.014
Reumer, M., Harnisz, M., Lee, H. J., Reim, A., Grunert, O., Putkinen, A., et al. (2018). Impact of peat mining and restoration on methane turnover potential and methane-cycling microorganisms in a northern bog. Appl. Environ. Microbiol. 84 (3), e02218-17. doi: 10.1128/AEM.02218-17
Rizzuti, A. M., Cohen, A. D., Stack, E. M. (2004). Using hydraulic conductivity and micropetrography to assess water flow through peat-containing wetlands. Int. J. coal geology 60, 1–16. doi: 10.1016/j.coal.2004.03.003
Ro, K., Novak, J., Bae, S., Flora, J., Berge, N. (2010). Greenhouse gas emission from soil amended with biochar made from hydrothermally carbonizing swine solids. In Paper presented at: American Chemical Society National Meeting. March 21-25, 2010, San Francisco, California.
Roseth, R., Haarstad, K. (2010). Pesticide runoff from greenhouse production. Water Sci. Technol. 61, 1373–1381. doi: 10.2166/wst.2010.040
Roy, P., Dutta, A., Gallant, J. (2020). Evaluation of the life cycle of hydrothermally carbonized biomass for energy and horticulture application. Renewable Sustain. Energy Rev. 132, 110046. doi: 10.1016/j.rser.2020.110046
Saunois, M., Stavert, A. R., Poulter, B., Bousquet, P., Canadell, J. G., Jackson, R. B., et al. (2020). The global methane budget 2000–2017. Earth System Sci. Data 12, 1561–1623. doi: 10.5194/essd-12-1561-2020
Savci, S. (2012). An agricultural pollutant: chemical fertilizer. Int. J. Environ. Sci. Dev. 3, 73. doi: 10.7763/IJESD.2012.V3.191
Savichev, O., Soldatova, E., Rudmin, M., Mazurov, A. (2020). Geochemical barriers in oligotrophic peat bog (Western Siberia). Appl. Geochemistry 113, 104519. doi: 10.1016/j.apgeochem.2019.104519
Savvas, D., Gianquinto, G., Tuzel, Y., Gruda, N. (2013). “Soilless culture. Good agricultural practices for greenhouse vegetable crops,” in Principles for Mediterranean climate areas, 303–354. Available at: https://hdl.handle.net/11588/596699
Scharlemann, J. P., Tanner, E. V., Hiederer, R., Kapos, V. (2014). Global soil carbon: understanding and managing the largest terrestrial carbon pool. Carbon Manage. 5, 81–91. doi: 10.4155/cmt.13.77
Schnitkey, G. (2017). “Nitrogen fertilizer prices and costs lower for 2018,” in Farmdoc daily (Illinois: Department of Agricultural and Consumer Economics, University of Illinois at Urbana-Champaign), 210.
Shackley, S., Sohi, S., Brownsort, P., Carter, S., Cook, J., Cunningham, C., et al. (2010). An assessment of the benefits and issues associated with the application of biochar to soil (London: Department for Environment, Food and Rural Affairs, UK Government).
Shotyk, W. (1988). Review of the inorganic geochemistry of peats and peatland waters. Earth-Science Rev. 25, 95–176. doi: 10.1016/0012-8252(88)90067-0
Sönmez, İ., Kaplan, M., Sönmez, S. (2008). Kimyasal gübrelerin çevre kirliliği üzerine etkileri ve çözüm önerileri. Batı Akdeniz Tarımsal Araştırma Enstitüsü Derim Dergisi 25 (2) 24–34.
Spokas, K. A., Baker, J. M., Reicosky, D. C. (2010). Ethylene: potential key for biochar amendment impacts. Plant Soil 333, 443–452. doi: 10.1007/s11104-010-0359-5
Spokas, K., Koskinen, W., Baker, J., Reicosky, D. (2009). Impacts of woodchip biochar additions on greenhouse gas production and sorption/degradation of two herbicides in a Minnesota soil. Chemosphere 77, 574–581. doi: 10.1016/j.chemosphere.2009.06.053
Taha, S. M., Amer, M. E., Elmarsafy, A. E., Elkady, M. Y. (2014). Adsorption of 15 different pesticides on untreated and phosphoric acid treated biochar and charcoal from water. J. Environ. Chem. Eng. 2, 2013–2025. doi: 10.1016/j.jece.2014.09.001
Temmink, R. J., Fritz, C., van Dijk, G., Hensgens, G., Lamers, L. P., Krebs, M., et al. (2017). Sphagnum farming in a eutrophic world: The importance of optimal nutrient stoichiometry. Ecol. Eng. 98, 196–205. doi: 10.1016/j.ecoleng.2016.10.069
The United Nations Sustainable Development Goals (2015). The 2030 Agenda for Sustainable Development. United Nations.
Tian, Y., Sun, X., Li, S., Wang, H., Wang, L., Cao, J., et al. (2012). Biochar made from green waste as peat substitute in growth media for Calathea rotundifola cv. Fasciata. Scientia Hortic. 143, 15–18. doi: 10.1016/j.scienta.2012.05.018
USDA (2008). “Agricultural Waste Management Field Handbook-Chapter 4 Agricultural Waste Characteristics,” in N.R.C.S. Unied State Department of Agriculture (Washington DC: USDA-NRCS).
USDA-EPA (1997). “Full cost accouting for municipal soild waste management: A handbook,” in U.S.E.P. Agency (Washington DC: USDA-EPA).
USDA-NASS (2018). “Agricultural Statistics,” in U.S.D.o. Agriculture (WA, USA: United States Government Printing Office Seattle), 202–210.
Valat, B., Jouany, C., Riviere, L. M. (1991). Characterization of the wetting properties of air-dried peats and composts. Soil Sci. 152, 100–107. doi: 10.1097/00010694-199108000-00006
Vaughn, S. F., Dinelli, F. D., Jackson, M. A., Vaughan, M. M., Peterson, S. C. (2018). Biochar-organic amendment mixtures added to simulated golf greens under reduced chemical fertilization increase creeping bentgrass growth. Ind. Crops Products 111, 667–672. doi: 10.1016/j.indcrop.2017.11.036
Vaughn, S. F., Kenar, J. A., Eller, F. J., Moser, B. R., Jackson, M. A., Peterson, S. C. (2015). Physical and chemical characterization of biochars produced from coppiced wood of thirteen tree species for use in horticultural substrates. Ind. Crops Products 66, 44–51. doi: 10.1016/j.indcrop.2014.12.026
Vaughn, S. F., Kenar, J. A., Thompson, A. R., Peterson, S. C. (2013). Comparison of biochars derived from wood pellets and pelletized wheat straw as replacements for peat in potting substrates. Ind. Crops products 51, 437–443. doi: 10.1016/j.indcrop.2013.10.010
Vercauteren, A., Riedel, M., Maes, M., Werres, S., Heungens, K. (2013). Survival of Phytophthora ramorum in Rhododendron root balls and in rootless substrates. Plant Pathol. 62, 166–176. doi: 10.1111/j.1365-3059.2012.02627.x
Wanner, N., Tubiello, F. N., DeSantis, G., Fuell, C., Gu, B. (2020). “Pesticides Trade 1990 - 2018. Global, regional and country trends,” in F.A.B. Series (Rome, Italy: FAO).
Webber, C. L., III, White, P. M., Jr., Gu, M., Spaunhorst, D. J., Lima, I. M., Petrie, E. C. (2018). Sugarcane and pine biochar as amendments for greenhouse growing media for the production of bean (Phaseolus vulgaris L.) seedlings. J. Agric. Sci. 10, 58. doi: 10.5539/jas.v10n4p58
Woolf, D., Amonette, J. E., Street-Perrott, F. A., Lehmann, J., Joseph, S. (2010). Sustainable biochar to mitigate global climate change. Nat. Commun. 1, 56. doi: 10.1038/ncomms1053
Wu, H., Lai, C., Zeng, G., Liang, J., Chen, J., Xu, J., et al. (2017). The interactions of composting and biochar and their implications for soil amendment and pollution remediation: a review. Crit. Rev. Biotechnol. 37 (6), 754–764. doi: 10.1080/07388551.2016.1232696
Xu, X., Cheng, K., Wu, H., Sun, J., Yue, Q., Pan, G. (2018). Greenhouse gas mitigation potential in crop production with biochar soil amendment-a carbon footprint assessment for cross-site field experiments from China. GCB Bioenergy 11, 592–605. doi: 10.1111/gcbb.12561
Xu, G., Zhang, Y., Sun, J., Shao, H. (2016). Negative interactive effects between biochar and phosphorus fertilization on phosphorus availability and plant yield in saline sodic soil. Sci. Total Environ. 568, 910–915. doi: 10.1016/j.scitotenv.2016.06.079
Yan, J., Yu, P., Liu, C., Li., Q., M., Gu. (2020). Replacing peat moss with mixed hardwood biochar as container substrates to produce five types of mint (Mentha spp.). Ind. Crops Products 155, 112820. doi: 10.1016/j.indcrop.2020.112820
Yeager, T., Fare, D., Lea-Cox, J., Ruter, J., Bilderback, T., Gilliam, C., et al. (2007). Best management practices: Guide for producing container-grown plants (Marietta, GA: Southern Nursery Association).
Yu, Z., Akridge, J. T., Dana, M. N., Lowenberg-DeBoer, J. (1990). An Economic evaluation of horticultural alfalfa as a substitute for sphagnum peat moss. Agribusiness 6, 443–462. doi: 10.1002/1520-6297(199009)6:5<443::AID-AGR2720060504>3.0.CO;2-J
Yu, P., Huang, L., Li, Q., Lima, I. M., White, P. M., Gu, M. (2020a). Effects of mixed hardwood and sugarcane biochar as bark-based substrate substitutes on container plants production and nutrient leaching. Agronomy 10, 156. doi: 10.3390/agronomy10020156
Yu, P., Li, Q., Huang, L., Qin, K., Niu, G., Gu, M. (2020b). The effects of mixed hardwood biochar, mycorrhizae, and fertigation on container tomato and pepper plant growth. Sustainability 12, 7072. doi: 10.3390/su12177072
Yu, Z., Loisel, J., Brosseau, D. P., Beilman, D. W., Hunt, S. J. (2010). Global peatland dynamics since the Last Glacial Maximum. Geophysical Res. Lett. 37 (13), L13402. doi: 10.1029/2010GL043584
Yu, P., Ong, K., Crosby, K., Gentry, T., Gu, M. (2021). Using poinsettia and pepper as model plants to investigate biochar and Trichoderma suppressing effects on plant diseases. Int. Plant Propagators’ Assoc. South. Region Proc. 7), 130–137.
Yu, P., Ong, K., Ueckert, J., Gu, M. (2023). Biochar and trichoderma reduce containerized poinsettia root rot caused by pythium aphanidermatum. HortScience 58 (8), 846–854. doi: 10.21273/HORTSCI17203-23
Zhang, X., Luo, Y., Goh, K. S. (2018). Modeling spray drift and runoff-related inputs of pesticides to receiving water. Environ. pollut. 234, 48–58. doi: 10.1016/j.envpol.2017.11.032
Zhu, J., Li, X., Zhang, F., Li, J., Christie, P. (2004). Responses of greenhouse tomato and pepper yields and nitrogen dynamics to applied compound fertilizers. Pedosphere 14 (2), 213–222.
Keywords: peat moss, substrate properties, pathogens, economic benefits, potted plant
Citation: Yu P, Qin K, Niu G and Gu M (2023) Alleviate environmental concerns with biochar as a container substrate: a review. Front. Plant Sci. 14:1176646. doi: 10.3389/fpls.2023.1176646
Received: 28 February 2023; Accepted: 10 July 2023;
Published: 27 July 2023.
Edited by:
V. Mohan Murali Achary, International Centre for Genetic Engineering and Biotechnology, IndiaReviewed by:
Giancarlo Fascella, Council for Agricultural and Economics Research (CREA), ItalyJunaid Ghani, University of Bologna, Italy
Copyright © 2023 Yu, Qin, Niu and Gu. This is an open-access article distributed under the terms of the Creative Commons Attribution License (CC BY). The use, distribution or reproduction in other forums is permitted, provided the original author(s) and the copyright owner(s) are credited and that the original publication in this journal is cited, in accordance with accepted academic practice. No use, distribution or reproduction is permitted which does not comply with these terms.
*Correspondence: Ping Yu, pingyu@uga.edu