- College of Agriculture, Guangxi University, Nanning, China
Abscisic acid (ABA) and nitric oxide (NO), as unique signaling molecules, are involved in plant growth, developmental processes, and abiotic stresses. However, the interaction between ABA and NO under abiotic stresses has little been worked out at present. Therefore, this paper reviews the mechanisms of crosstalk between ABA and NO in the regulation of plants in response to environmental stresses. Firstly, ABA-NO interaction can alleviate the changes of plant morphological indexes damaged by abiotic stresses, for instance, root length, leaf area, and fresh weight. Secondly, regulatory mechanisms of interaction between ABA and NO are also summarized, such as reactive oxygen species (ROS), antioxidant enzymes, proline, flavonoids, polyamines (PAs), ascorbate-glutathione cycle, water balance, photosynthetic, stomatal movement, and post−translational modifications. Meanwhile, the relationships between ABA and NO are established. ABA regulates NO through ROS at the physiological level during the regulatory processes. At the molecular level, NO counteracts ABA through mediating post-translational modifications. Moreover, we also discuss key genes related to the antioxidant enzymes, PAs biosynthesis, ABA receptor, NO biosynthesis, and flavonoid biosynthesis that are regulated by the interaction between ABA and NO under environmental stresses. This review will provide new guiding directions for the mechanism of the crosstalk between ABA and NO to alleviate abiotic stresses.
1 Introduction
Abiotic stresses such as drought, salt, heavy metal, extreme light, ultraviolet-B (UV-B), and extreme temperature are usually caused by changes in environmental factors (Gujjar et al., 2014; Kreszies et al., 2018; Stromme et al., 2019). When plants are exposed to extreme environments, it often results in morphological and physiological changes, for instance, growth inhibition (Pontin et al., 2021), chlorophyll degradation (Sahay et al., 2019), stomatal closure (Peng et al., 2022), and redox imbalance (Lu et al., 2009). In response to these adverse effects, plants often stimulate reactive oxygen species (ROS) generation, including hydrogen peroxide (H2O2), superoxide radicals (O2-), and hydroxyl radicals (OH–). Interestingly, ROS is considered a double-edged sword: promotion of plant growth at low concentrations and inhibition at high levels (Li et al., 2022). When the stress exceeds the tolerance capacity of plants, excess ROS is produced in plant cells (Gill and Tuteja, 2010; Mullineaux et al., 2018; Zhang et al., 2018a). High levels of ROS lead to excessive oxidation of the plasma membrane, frequently producing high levels of malondialdehyde (MDA) and thiobarbituric acid reactive substances (TBARS), resulting in plant cell poisoning (Xu et al., 2013; Berni et al., 2019; Iqbal et al., 2022). To scavenge excess ROS, plants can regulate antioxidant enzymes, antioxidants, phytohormones, and signaling molecules in response to stress (Muneer et al., 2014; Peng et al., 2022; Saha et al., 2022). Moreover, abscisic acid (ABA) and nitric oxide (NO) significantly enhance antioxidant enzyme activities to eliminate excess ROS, thus increasing the survival percentage of maize seedlings under chilling stress (Li and Zhang, 2012). The polyethylene glycol (PEG)-induced reduction in length, fresh weight, and dry weight of roots are reversed by exogenous ABA and NO (Sahay and Gupta, 2018; Sahay et al., 2019). The authors also found that ABA and NO can increase the levels of antioxidant enzymes and reduce ROS and MDA contents (Sahay and Gupta, 2018; Sahay et al., 2019). The above studies imply that ABA and NO play a crucial role in inhibiting ROS and reducing plasma membrane oxidation under abiotic stresses.
ABA is an essential plant hormone, which is closely linked to seed germination (Kermode, 2005), stomatal closure (Raschke et al., 1975), and defoliation (Gao et al., 2017), especially abiotic stresses (Sah et al., 2016). It has been reported that ABA regulates stomatal movement and abscission by binding to specific receptors under abiotic stresses (Iqbal et al., 2021). Meanwhile, the regulatory mechanisms of ABA under stresses are complex, because ABA interacts with other signaling molecules, such as ROS, calcium ion (Ca2+), hydrogen sulfide (H2S), and NO (Desikan et al., 2004). As a signaling molecule, NO is involved in chlorophyll biosynthesis (Zhang et al., 2018b), flowering time (Grun et al., 2006), crop yield (Hou and Tsuruta, 2003), stomatal movement (Castillo et al., 2015), and abiotic stresses (Iqbal et al., 2022). Simultaneously, NO also acts in conjunction with other signaling molecules or hormones under abiotic stresses. For instance, our previous research has shown that NO is involved in melatonin-induced antioxidant response in the leaves of tomato seedlings under cadmium stress (Xu et al., 2023). Moreover, NO modulates seed germination, stomatal movement, and environmental tolerance by post-translational modifications (PTMs), including S-nitrosylation and tyrosine nitration (Albertos et al., 2015; Wang et al., 2015; Yang et al., 2015). For S-nitrosylation, it is produced by covalent binding between the NO group and thiol (–SH) of cysteine (Prakash et al., 2019; Iqbal et al., 2021). In addition, susceptible amino acids are bonded with a nitro group to develop tyrosine nitration (Prakash et al., 2019; Iqbal et al., 2021).
A growing number of reports indicate that the interaction between ABA and NO participates in abiotic tolerance (Zhang et al., 2019; Santos et al., 2020; Iqbal et al., 2022). For example, the growth of wheat is altered by ABA and NO under heat stress, including increase of the length, fresh weight, dry weight, and leaf area (Iqbal et al., 2022). ABA and NO solution treatments can maintain firmness and peel color as well as improve antioxidant capacity in peach fruit under low-temperature stress, however, ABA-induced cold tolerance is blocked by NO scavenger 2-(4-carboxyphenyl)-4,4,5,5-tetramethylimidazoline-1-oxyl-3 oxide (cPTIO) (Zhang et al., 2019). Zhang et al. (2018b) imply that low-light stress can cause a decrease in plant height, leaf width, tiller number, and dry weight of tall fescue seedlings, whereas ABA alleviates the reduction, following an increase in endogenous NO concentration. Interestingly, ABA biosynthesis inhibitor fluridone (Flu) can suppress endogenous NO generation. These results indicate that ABA promotes plant growth by increasing NO response under low-light stress. Under chilling stress, ABA and NO donor sodium nitroprusside (SNP) enhance the activities of antioxidant enzymes to reduce ROS levels in maize seedlings (Li and Zhang, 2012). Nevertheless, ABA-activated antioxidant responses are reversed by NO scavenger cPTIO, suggesting that ABA enhances chilling tolerance relying on NO.
The above results show that crosstalk between ABA and NO is involved in abiotic tolerance, but few reviews have summarized and discussed it. Thus, this paper presents a comprehensive review of the interaction between ABA and NO under abiotic tolerance and their regulatory pathways. In addition, the expressions of key genes mediated by interaction between ABA and NO are briefly discussed.
2 ABA-NO interaction confers tolerance to plants under environmental stresses
2.1 Drought
Drought stress affects the growth, development, and survival of plants (Wang et al., 2018). However, it has been shown that ABA, NO, and/or ROS contribute/contributes to stress mitigation. For example, the roots tips of wheat seedlings are detached, then the shed root tips are exposed to drought stress by evaporating (Zhao et al., 2001). The activities of superoxide synthase and NO synthase-like (NOS-like, EC 1.14.13.39) are enhanced at 20 min after drought treatment, and the content of endogenous ABA is accumulated after 60 min. It is interesting that ABA-induced drought stress response is inhibited by ROS scavenger, NOS-like inhibitor, or NO scavenger, indicating that ROS and NO are involved in ABA-induced drought tolerance in root tips of wheat seedlings (Zhao et al., 2001; Table 1). Tari et al. (2010) reveal that the root length of drought-tolerant wheat cultivar is lower than that of drought-sensitive wheat cultivar under drought stress. Meanwhile, high levels of ROS, ABA, and NO are detected in drought-tolerant wheat cultivar, showing that the generations of ROS and NO are induced by ABA under osmotic stress. Under osmotic tolerance, the leaf water loss is reduced by SNP and H2O2 in wheat seedlings (Xing et al., 2004; Table 1). Meanwhile, SNP and H2O2 can improve ABA content during the process. However, the effects are reversed by NO scavenger and NOS inhibitors. The results demonstrate that there is a crosstalk between ABA and NO under drought stress. Exogenous ABA can improve drought tolerance by regulating antioxidant systems and relative water content (RWC) as well as generating NO and H2O2 in triploid bermudagrass (Lu et al., 2009). The authors further found that NO and H2O2 also activate antioxidant systems in response to drought stress (Lu et al., 2009). Moreover, ABA-induced drought tolerance is reduced by NO scavenger/inhibitor and H2O2 scavenger/inhibitor. Furthermore, H2O2 scavenger/inhibitor can suppress NO-induced drought tolerance. The above results show that ROS is linked between ABA and NO under drought stress. For tomato seeds under osmotic stress, 3 μM ABA accelerates seed dormancy, and NO donor S-nitrosoglutathione (GSNO) can reverse the effects (Piterkova et al., 2012). Endogenous ABA, NO, H2O2 in the leaves of Bromeliaceae are accumulated, and RWC is reduced during drought stress, suggesting that ABA, NO, and H2O2 play an important role in the response to drought stress by osmotic adjustment (Mioto and Mercier, 2013; Table 1). Planchet et al. (2014) report that PEG-induced accumulation of NO in Medicago seedlings is reduced by the ABA biosynthesis inhibitor norflurazon. Interestingly, under normal conditions, NO content is increased by exogenous ABA, but this effect is reversed by NO scavenger cPTIO (Planchet et al., 2014). These results indicate that NO may also be involved in ABA-induced drought stress response. Drought stress stimulates the generation of superoxide radicals (O2-), H2O2, and MDA in the leaves of seedlings, thus the growths of Pusa Jagannath and Varuna Indian mustards are inhibited (Sahay et al., 2019). However, ABA and NO markedly promote growth by enhancing the activities of antioxidant enzymes, mediating proline (Pro) metabolism, and maintaining water content (Sahay et al., 2019; Table 1). Pontin et al. (2021) also conclude that ABA and NO can promote drought tolerance by improving antioxidant enzyme activities and flavonoid metabolism and maintaining water balance in the leaves of grapevines.
Overall, previous reports exhibit that the involvement of NO in ABA-induced tolerance is linked to H2O2. At the same time, crosstalk between ABA and NO confers drought tolerance in plants by maintaining water balance, enhancing antioxidant-related enzymes, and promoting flavonoid metabolism. Nevertheless, there are few studies on the role of ABA-NO interaction in drought tolerance at the molecular aspects, this topic needs to be further investigated.
2.2 Temperature stress
Temperature stress, including high-temperature stress and low-temperature stress, restricts the growth and development of plants. High temperature induces ABA and NO accumulation in reed plants (Song et al., 2008). In addition, the effects of NO scavenger/inhibitor cPTIO/Nw-nitro-L-arginine (LNNA) on endogenous ABA content are not significant, however, endogenous NO content and NOS-like activity are decreased by ABA inhibitor Flu. The results suggest that NO production is involved in ABA-induced high-temperature stress tolerance and acts downstream of ABA (Song et al., 2008; Table 1). Iqbal et al. (2022) conclude that heat tolerance is enhanced by ABA and 100 µM SNP by improving photosynthesis and antioxidant capacity and up-regulating the expression of antioxidant enzyme-related genes. Applying 100 µM ABA and SNP can alleviate low-temperature-induced growth inhibition of maize seedlings via enhancing antioxidant enzyme activities and reducing H2O2 and MDA levels (Li and Zhang, 2012; Tables 1, 2). Furthermore, the effects of ABA are blocked by NO scavenger, indicating that ABA enhances chilling tolerance by NO generation. Similarly, Dong et al. (2017) report that ABA and NO significantly enhance antioxidant response in the leaves of walnut shoots under low-temperature stress. Nevertheless, co-treatment with cPTIO and ABA can reverse the effects. The exposure of peach fruits to low temperatures significantly increases endogenous ABA and NO contents, therefore enhancing antioxidant capacities to eliminate excess ROS and preventing over-oxidation of the plasma membrane (Zhang et al., 2018b; Table 1). In addition, the effect is inhibited by NO scavenger/inhibitor, suggesting that ABA induces the antioxidant response to confer low-temperature tolerance through NO production.
Low temperature up-regulates the expression level of the MfSAMS1 gene (related to S-adenosylmethionine synthetase, the enzyme catalyzing the formation of polyamines and ethylene), manifesting that MfSAMS1 may be involved in the response to cold stress in Medicago (Guo et al., 2014; Tables 1, 2). Additionally, ABA, H2O2, or NO treatment alone significantly up-regulates MfSAMS1 expression. And its expression is decreased by ABA biosynthesis inhibitor naproxen, H2O2 scavenger dimethylthiourea (DMTU), or NO scavenger cPTIO treatment under cold stress, respectively, indicating that ABA, H2O2, or NO influences MfSAMS1 expression and they may alleviate cold stress by up-regulating MfSAMS1 expression. In order to further verify the role of MfSAMS1 under cold stress, overexpressing MfSAMS1 transgenic tobacco is obtained (Guo et al., 2014). The overexpression of MfSAMS1 in tobacco increases the survival and net photosynthetic rate (Pn) as well as decreases ion leakage. The contents of spermidine (Spd) and putrescine (Put) are also improved, following high levels of enzyme activities and gene expression related to polyamine biosynthesis and antioxidant enzyme (Guo et al., 2014). These results reveal that SAMS alleviates cold stress through up-regulating polyamine oxidation. Likewise, the concentrations of NO and H2O2 are raised by Spd or Spm treatment alone in tomato seedlings under low-temperature stress (Diao et al., 2017). Furthermore, Spd or Spm can enhance the activities of nitrate reductase (NR, EC 1.7.99.4), NOS-like, diamine oxidase (DAO, EC 1.4.3.22), and polyamine oxidase (PAO, EC 1.5.3.11), up-regulating LeNR expression, and down-regulating LeNOS1 expression (Diao et al., 2017). Endogenous NO content is decreased when H2O2 inhibitor or scavenger is added, while endogenous H2O2 content is not significantly changed when NO inhibitor or scavenger is added (Diao et al., 2017). The results mentioned above indicate that Spd and Spm enhance the production of NO by the NR pathway depending on H2O2. SNP treatment increases Put, Spd, and Spm contents and up-regulates polyamine biosynthesis-related gene expression, so it can be concluded that NO can increase the contents of Put and Spd under low-temperature stress. ABA content is increased by Put treatment, following the reduction of electrolyte leakage. However, the effects are reversed by Put synthesis inhibitor D-arginine. The expression of the ABA biosynthesis-related gene is up-regulated by Put treatment, but it is not observably changed by D-Arg, showing that ABA is involved in Put-induced low-temperature tolerance (Diao et al., 2017). These results demonstrate that interaction between NO, ABA, and H2O2 can improve the PAs-induced cold tolerance by up-regulating the expression of polyamine and ABA biosynthesis-related gene expression (Tables 1, 2). In CPK27-silenced (related to calcium-dependent protein kinases) tomatoes, photosynthetic and antioxidant capacity, ABA, NO, and H2O2 contents, and the activities of nicotinamide adenine dinucleotide phosphate (NADPH) oxidase, NR, and mitogen-activated protein kinases1/2 (MPK1/2) are decreased under cold stress (Lv et al., 2018). However, the levels of NO, H2O2, and MPK1/2 are increased when ABA is added during the process. To study the relationship among ABA, NO, H2O2, and MPK1/2, the mutants are obtained. The results indicate that low level of ABA is detected in the silence of gene related to H2O2-synthesis RBOH1 (NADPH-dependent respiratory burst oxidase homolog), MPK1/2, or NR mutant, separately. Furthermore, low levels of CPK27, NO, and MPK1/2 are found in the deficiency of ABA mutant notabilis (Lv et al., 2018; Tables 1, 2). The results reflect that ABA is involved in the upregulation of CPK27 expression-induced low-temperature tolerance by increasing NO, H2O2, and MPK1/2 levels. After cold-acclimated treatment, creeping lilyturf leaves have higher water retention capacity, photosynthetic efficiency, and antioxidant enzyme activity (Peng et al., 2022; Tables 1, 2). The authors use transcriptomics and metabolomics to find that genes related to cellular processes, environmental information processing, genetic information processing, metabolism, and organismal systems are considerably enriched, with 4,620 upregulation and 5,824 downregulation (Peng et al., 2022). The authors identify that the contents of 10 flavonoids increased significantly under low-temperature stress, following regulation of the expression of flavonol biosynthesis pathway genes (Peng et al., 2022). Likewise, the contents of intermediate products and genes associated with the ABA biosynthesis pathway are significantly regulated, following high ABA concentration and low stomatal aperture. The sequence of ABA signaling is sequentially from PYR1/PYL, PP2C, SnRK2 to ABF. In addition, NO content is increased, with NOS up-regulation and PAO down-regulation (Peng et al., 2022; Table 2). The intermediates of the NO biosynthesis pathway are increased. These results exhibit that the interaction between ABA, NO, and flavonoids can improve low-temperature tolerance via reducing stomatal aperture.
Similar to drought tolerance, ABA-NO interaction can improve tolerance through enhancing antioxidant response and photosynthetic capacity, up-regulating MfSAMS1 and CPK27 expression, promoting polyamine and flavonoid biosynthesis, and accelerating stomatal closure.
2.3 Salt
Excessive salt is one of the most important factors restricting crop growth (Elsiddig et al., 2022). Santos et al. (2020) found that endogenous NO content is increased in the roots of wild-type (WT) tomato but reduced in the roots of ABA-insensitive mutant sitiens under salt stress. The concentration of NO is reduced in WT under salt stress when NO scavenger/inhibitor is added separately, but there is no significant change in sitiens mutant (Santos et al., 2020). Meanwhile, SNP treatment can enhance antioxidant enzyme activity in sitiens mutant, but WT type is not detected, implying that ABA-mediated NO improves sodium chloride (NaCl) tolerance through enhancing antioxidant enzyme activities (Table 1). Ruan et al. (2004) showed that 0.1 mM SNP can promote RWC in the leaves of wheat seedlings under 150 mM NaCl stress. Endogenous ABA content is increased by SNP treatment, but it also can be arrested by NO scavengers (Ruan et al., 2004). Simultaneously, ABA and NO increase Pro content, however NO scavengers suppress it. The results suggest that ABA increases Pro level dependent on NO production under NaCl stress (Table 1). The application of ABA and NO can improve the growth of rice by maintaining water homeostasis, sodium ion/potassium ion (Na+/K+) ratio, elevating antioxidant enzyme activities and antioxidant contents under 200 mM NaCl stress (Saha et al., 2022; Table 1). Hsu and Lee, 2018 reveal that methionine sulfoxide reductase (MSR; catalyzed the reduction of methionine sulfoxide to methionine residues and alleviated ROS damage to proteins)-related gene expression is up-regulated in rice roots under salt stress, but when 0.3 mM Flu or 300 μM cPTIO is added, the effect is reversed (Hsu and Lee, 2018; Table 2). In this process, NO content is decreased by Flu treatment (Hsu and Lee, 2018), implying that ABA-induced NO responds to salt stress through the regulation of MSR-related genes.
Overall, ABA-induced NO enhances the tolerance against salt stress by maintaining water homeostasis, increasing antioxidant enzyme activity and antioxidant contents, and regulating MSR-related gene expression. There are fewer studies in the molecular direction, and our future research should pay attention to this aspect.
2.4 Heavy metal
Excessive heavy metal ions can be absorbed by plants, thus causing damage to the plant cell membrane system (Yu et al., 2023). Wu et al. (2018) found that ABA synthesis is activated by aldehyde oxidase involved in the ABA biosynthesis pathway under molybdenum (Mo) condition. However, NO scavenger cPTIO can constrain ABA synthesis, following the reduction of antioxidant enzyme activities and associated gene expression. The results show that ABA-dependent NO improves Mo tolerance by activation of the antioxidant systems. Lead (Pb) results in a reduction of stomatal conductance, leaf area, and seed yield of cowpeas (Sadeghipour, 2017). Besides, the levels of auxin (IAA), cytokinin (CTK), and gibberellic acid (GA) are reduced and ABA is increased. However, 0.5 mM SNP can reverse the above effects. These results show that NO modulates ABA to inhibit Pb uptake, thus enhancing Pb tolerance. SNP treatment promotes root elongation as well as ABA and IAA generations in the root apices of rye and wheat under aluminum (Al) stress (He et al., 2012). The effect is reversed by NO scavenger. At present, there are relatively few studies on the interaction between ABA and NO in heavy metal stress tolerance, thus we still need to focus on heavy metal stress.
2.5 Light
Generally, light stress includes high-light and low-light stresses (Xu et al., 2013; Zhang et al., 2018b). High-light stress [500 μmol m−2 s−1 photosynthetic photon flux density (PPFD); the control is set to 100 μmol m−2 s−1 PPFD] results in an increase in ion leakage and an accumulation of MDA in the leaves of tall fescue seedlings (Xu et al., 2013). These negative effects are alleviated by ABA, SNP, or SNP+Flu treatment and can be worsened by cPTIO, Flu, ABA+cPTIO, LNNA, or LNNA+ABA treatment. Furthermore, exogenous ABA increases NOS activity resulting in NO production and antioxidant enzyme activation, implying that ABA alleviates high-light stress depending on NO via enhancing antioxidant capacity (Table 1). Zhang et al. (2018b) found that NO content and NOS-like activity in tall fescue seedlings are increased by exogenous ABA and are reduced by Flu under low-light stress (40 μmol m−2 s−1 PPFD; the control was set to 200 μmol m−2 s−1 PPFD). Meanwhile, the expression of the NO biosynthesis-related gene is up-regulated by ABA and is descended by Flu. The authors further found that exogenous ABA can raise photosynthetic capacity and antioxidant enzyme activity and up-regulate antioxidant enzyme-related gene expression (Zhang et al., 2018b; Tables 1, 2). To sum up, ABA-activated NOS-like signaling induces NO production and enhances light tolerance through increasing antioxidant and photosynthetic capacity and regulating their gene expression.
2.6 UV-B
When the environment tends to continue to deteriorate, more and more ultraviolet irradiation is not absorbed by the ozone layer, resulting in plant exposure to high levels of UV-B radiation, which reduces crop yields. Ion leakage is increased by UV-B in maize seedlings, and this negative effect is alleviated by using exogenous ABA (Tossi et al., 2009). Moreover, the concentration of H2O2 is increased in maize seedlings of WT, and viviparous 14 (vp14), a mutant defective in ABA synthesis, only slightly changed. NO is detected in the WT but not in the vp14 mutant (Tossi et al., 2009). In addition, when 100 µM ABA is supplied, NO production is restored in the vp14 mutant. Catalase (CAT, EC 1.11.1.6, as H2O2-degrading enzyme), H2O2 inhibitor diphenylene iodonium (DPI), and the NO inhibitor Nw-nitro-L-arginine methyl ester (LNAME) partially inhibit UV-B-induced NO accumulation, indicating that H2O2 and NOS-like activity play an important role in plant response to UV-B (Tossi et al., 2009). The results as mentioned show that UV-B improves ABA accumulation, which activates NADPH oxidase and H2O2 production, and that a NOS-like-dependent mechanism increases NO production, suggesting that ABA alleviates UV-B by increasing H2O2 generation and NO production-dependent on NOS-like (Table 1). Thus, detailed molecular insights into the underpinning mechanisms remain to be established, for example, UV-B irradiation stress regulates the change of endogenous ABA and NO levels.
2.7 Alkali
Plants are subject to alkali stress resulting in growth inhibition. For instance, when Nitraria tangutorum seedlings are exposed to alkali stress, plant height, fresh weight, and RWC are reduced (Zhang et al., 2023). Nevertheless, exogenous ABA and NO can promote plant growth of Nitraria tangutorum seedlings. The authors further found that exogenous ABA and NO can enhance antioxidant capacity and photosynthetic capacity, regulate transcript levels of ion transporter genes and the biosynthesis of NO and ABA genes, and reduce the stomatal aperture (Zhang et al., 2023). It can be concluded that crosstalk between ABA and NO can improve alkali tolerance by the above pathways.
3 Regulatory pathways of NO-ABA interaction alleviating abiotic stress in plants
3.1 Reactive oxygen species
Low concentrations of ROS can act as signaling molecules to be involved in ABA and NO interaction under stresses. For example, ROS, ABA, and NO are induced by osmotic stress in drought-sensitive wheat cultivar, implying that there is an interaction among ROS, ABA, and NO under drought stress (Tari et al., 2010; Table 1). ABA-induced osmotic tolerance is weakened by NO scavenger/inhibitor and ROS scavenger in the root tips of wheat seedlings (Zhao et al., 2001). Lu et al. (2009) point out that NO and H2O2 are generated by exogenous ABA in triploid bermudagrass under drought stress. Moreover, ABA-induced oxidative response is inhibited by H2O2 scavenger/inhibitor and NO scavenger/inhibitor. However, H2O2 scavenger/inhibitor reduces NO-induced oxidative tolerance. Under UV-B stress, NO and H2O2 are lower in ABA synthesis-deficient mutant vp14 compared to WT (Tossi et al., 2009). In addition, NO synthesis is inhibited by H2O2 inhibitors during the process. The results imply that interaction between ABA and NO is linked by ROS under abiotic stress.
3.2 Antioxidant enzymes
Drought-induced oxidative stress results in an elevation of ion leakage, MDA, and H2O2 in triploid bermudagrass (Lu et al., 2009). To avoid high levels of drought-induced ROS damage to plant cells, plants depend on the enzymatic antioxidant system to scavenge excess ROS. Consequently, the activities of superoxide dismutase (SOD, EC 1.15.1.1), CAT, ascorbate peroxidase (APX, EC 1.11.1.11), and peroxidase (POD, EC 1.11.1.7) are enhanced by NO and ABA to scavenge excess ROS. In addition, ABA and NO-induced antioxidant enzyme activities are inhibited by NO scavenger/inhibitor and H2O2 scavenger/inhibitor (Lu et al., 2009; Table 1; Figure 1). The above results show that ABA activates ROS signaling resulting in NO accumulation to enhance antioxidant enzyme activities under drought stress. Drought-induced high levels of O2-, H2O2, and MDA are reversed by ABA and SNP alone or co-treatment in Pusa Jagannath and Varuna types of Indian mustard (Sahay et al., 2019; Figure 1). The activities of SOD, CAT, and APX in the roots and leaves of Pusa Jagannath are enhanced by NO and ABA during the process. When ABA and SNP are applied, APX and POD activities are elevated in the leaves of grapevines under drought stress (Pontin et al., 2021). Under salt stress, NO is not generated in the root of ABA-insensitive mutant sitiens. However, salt resistance is elevated by the activities of APX and CAT compared to WT-type tomato when NO is added (Santos et al, 2020). The results can be seen that NO acts cooperatively in ABA-induced antioxidant enzymes under salt tolerance. Using 200 mM NaCl-induced salt stress triggers the accumulation of H2O2 and O2- in rice, but when SNP and ABA are applied, these negative effects are reversed, following high levels of APX and glutathione S-transferase (GST, EC 2.5.1.18; Saha et al., 2022). Wu et al. (2018) found that the concentrations of O2- and MDA are increased in wheat seedlings under Mo condition, but these adverse effects can be relieved by exogenous ABA and SNP. During the process, ABA and SNP also enhance the activities of antioxidant enzymes, including SOD, CAT, POD, and APX (Wu et al., 2018). Nevertheless, cPTIO also can block ABA biosynthesis and antioxidant enzyme activities (Wu et al., 2018; Figure 1C). Under high-light stress, ion leakage, MDA, H2O2, and O2- levels increase significantly in the leaves of tall fescue seedlings, whereas ABA and SNP suppress these negative effects (Xu et al., 2013). However, ABA-induced antioxidant responses are inhibited by NO scavenger/inhibitor (Xu et al., 2013; Figure 1C). Furthermore, ABA stimulates NO accumulation via the NOS pathway, following the high levels of SOD, CAT, APX, and glutathione reductase (GR, EC 1.8.1.7) (Xu et al., 2013). Likewise, ABA-dependent on NO increases significantly the activities of POD, SOD, CAT, and APX, thus decreasing remarkably the levels of ion leakage, MDA, H2O2, and O2- contents in tall fescue seedlingsunder low-light stress (Zhang et al., 2018b; Figure 1C). Iqbal et al. (2022) found that the activities of SOD, CAT, APX, and GR are enhanced by ABA and NO to decrease TBARS and H2O2 productions in wheat under heat stress. ABA and NO prevent ROS accumulation and MDA production against low-temperature stress, accompanied by an increase in SOD and CAT activities (Li and Zhang, 2012; Figure 1C). The levels of SOD, CAT, APX, and GR are increased by ABA and NO caused by decreasing the contents of electrolyte leakage, MDA, H2O2, O2-, and OH– in the leaves of walnut shoots under chilling stress (Dong et al., 2017). Furthermore, NO scavenger cPTIO and ABA inhibitor Flu can inhibit these effects. Low-temperature stress triggers the increase of electrolyte leakage, MDA, and ROS, which lead to lipid peroxidation in peach fruit. Nevertheless, the adverse effect is reversed by ABA and NO through enhancing the activities of SOD, POD, APX, GR, monodehydroascorbate reductase (MDHAR), and dehydroascorbate reductase (DHAR, EC 1.8.5.1) (Zhang et al., 2019; Figure 1C). Similarly, Zhang et al. (2023) suggest that ABA and NO can eliminate ROS, MDA, and electrical leakage to improve alkali tolerance by enhancing the levels of GR, CAT, SOD, GST, and APX in Nitraria tangutorum seedlings. Together, all these results show that the crosstalk between ABA and NO has an essential role in the activation of antioxidant enzymes response to abiotic stress.
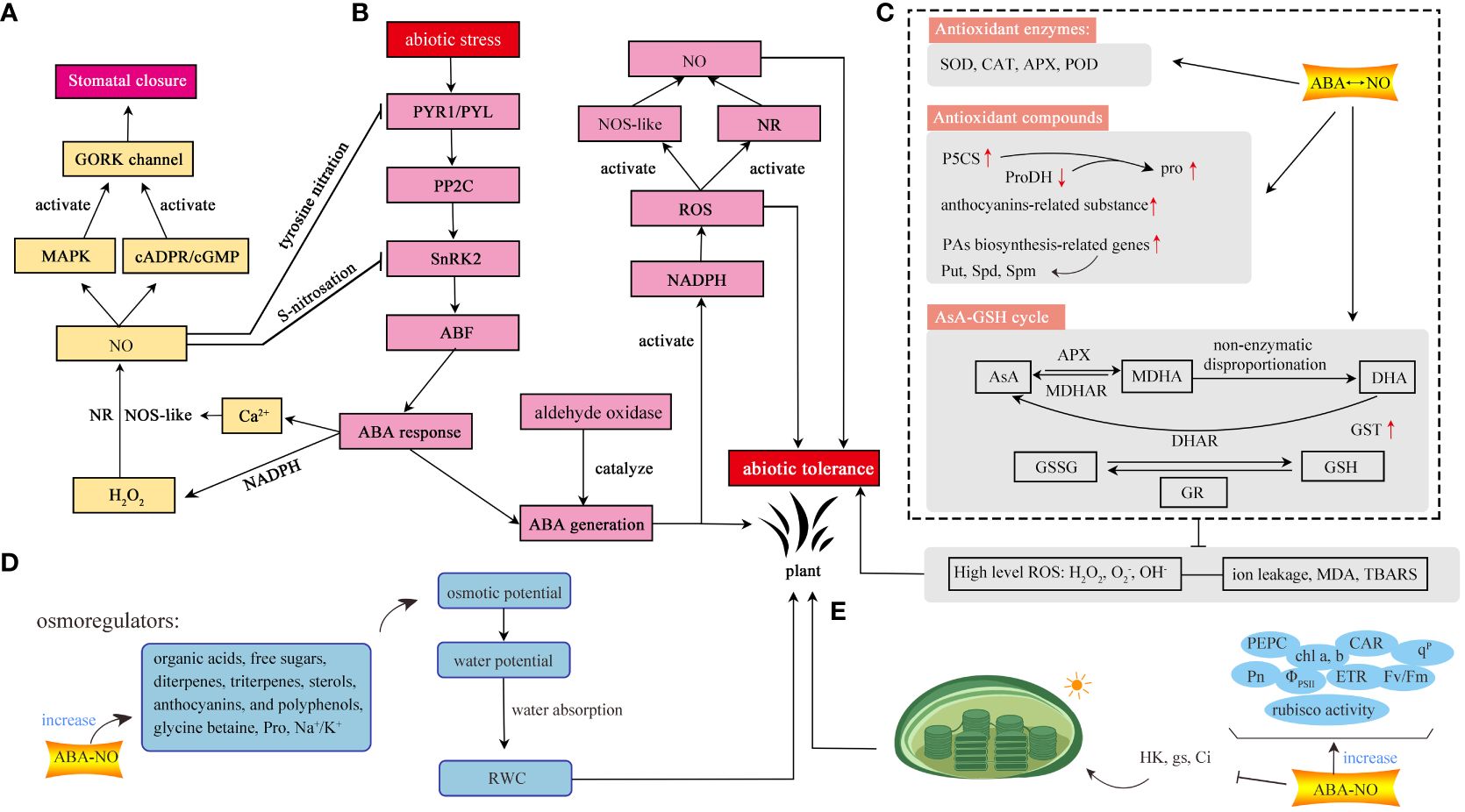
Figure 1 The regulatory mechanism of ABA-NO interaction under abiotic stress in plants. (A) Stomatal movement. (B) Regulatory pathways among ABA, NO, ROS. (C) Antioxidant systems. Plants scavenge excess ROS through three pathways, including antioxidant enzymes, antioxidants, and AsA-GSH cycle. (D) Water balance. (E) Photosynthesis. The interaction between ABA and NO can mediate photosynthesis to enhance environmental tolerance. GORK, outward-rectifying K+; MAPK, mitogen-activated protein kinase; cGMP, 3’,5’-cyclic guanosine monophosphate; NO, nitric oxide; ABA, abscisic acid; NR, nitrate reductase; NOS-like, NO synthase-like; H2O2, hydrogen peroxide; Ca2+, calcium ion; NADPH, nicotinamide adenine dinucleotide phosphate; PYR/PYL, pyrabactin resistance/PYR1-like; PP2C, type 2C protein-phosphatase; SnRK2, sucrose non-fermenting 1-related protein kinase subfamily 2; ABF, ABA-responsive element binding factors; SOD, superoxide dismutase; CAT, catalase; APX, ascorbate peroxidase; POD, peroxidase; P5CS, pyrroline-5-carboxylate synthetase; ProDH, recombinant proline dehydrogenase; PAs, polyamines; Put, putrescine; Spd, spermidine; Spm, spermine; AsA, ascorbic acid; MDHAR, monodehydroascorbate reductase; MDHA, monodehydroascorbate; DHAR; dehydroascorbate reductase; DHA; dehydroascorbate; GST, glutathione S-transferase; GSH, glutathione; GR, glutathione reductase; GSSG, oxidized glutathione; O2-, superoxide radicals; H2O2, OH-, hydroxyl radicals;hydrogen peroxide; MDA, malondialdehyde; TBARS, thiobarbituric acid reactive substances; hexose kinase; gs, stomatal conductance; Ci, intercellular CO2 concentration; PEPC, phosphoenolpyruvate carboxylase; Pn, net photosynthetic rate; chl a, b, chlorophyll a, b; ΦPSII, actual photochemical efficiency of PSII; CAR, total carotenoids; ETR, apparent electron transport rate; Fv/Fm, the maximum quantum yield of PSII photochemistry; qP, photochemical quenching; Pro, proline; RWC, relative water content; Na+/K+, sodium ion/potassium ion.
3.3 Ascorbate-glutathione cycle
The ascorbate-glutathione (AsA-GSH) cycle is a multistep enzymatic catalytic system for ROS elimination in plant tissues (Sahay et al., 2019; Zhang et al., 2019; Xu et al., 2023; Zhang et al., 2023). Four enzymes cooperatively burst ROS to maintain cellular redox balance, including, monodehydroascorbate reductase (MDAR, EC 1.6.5.4), DHAR, GR, and APX. The application of ABA and NO not only improves ascorbic acid (AsA) content but also elevates APX activity in the leaves of Indian mustard exposed to drought stress (Sahay et al., 2019; Figure 1C). Alkali stress-induced levels of reduced glutathione (GSH), GR, and GST are increased by ABA and NO, following the increments of the reduced glutathione/oxidized glutathione (GSH/GSSG) ratio in Nitraria tangutorum seedlings (Zhang et al., 2023). Under chilling resistance, the activities of APX, GR, and MDAR are promoted by ABA and NO in peach fruits (Zhang et al., 2019). ABA and NO also induce AsA and GST generations as well as high AsA/DHA and GSH/GSSG ratios, but these effects are inhibited by ABA+cPTIO treatment. Similarly, the levels of AsA, GSH, APX, and GR are raised by ABA and NO in the leaves of walnut shoots under low-temperature stress (Dong et al., 2017; Figure 1C). In addition, these effects are reversed by ABA+cPTIO treatment. The results imply that the ABA-induced AsA-GSH cycle exists NO-dependent mechanism.
3.4 Proline
Pro is an antioxidant compound that is considered a ROS scavenger (Pontin et al., 2021). Under salt stress, both ABA and NO improve the activity of pyrroline-5-carboxylate synthetase (P5CS, a Pro synthesis enzyme) and inhibit the activity of recombinant proline dehydrogenase (ProDH, a Pro degradation enzyme) in the leaves of wheat seedlings, indicating that Pro participates in the interaction between ABA and NO-induced salt tolerance (Ruan et al., 2004; Figure 1C). Sahay et al. (2019) report that ABA and NO increase the content of Pro in the leaves of Pusa Jagannath type Indian mustard under drought stress. Heat stress-induced Pro in wheat is raised by ABA and SNP treatments (Iqbal et al., 2022). When NO scavenger cPTIO is added, the Pro content induced by ABA is reduced. Interestingly, the Pro concentration of Medicago seedlings is reduced by NO under drought stress (Planchet et al., 2014). Similarly, the concentration of Pro in grapevines is also reduced by ABA or NO (Pontin et al., 2021). In short, the above results show that Pro may be modulated by ABA-NO interaction under environmental stresses. However, there is an urgent need for further explorations to understand the potential mechanisms. For example, the regulation pathway of Pro content by the interaction between ABA and NO.
3.5 Flavonoids
PEG-induced total flavonoid accumulation is reduced by ABA and NO (Sahay et al., 2019), implying that ABA and NO are involved in drought tolerance through flavonoid metabolism (Table 1). However, Pontin et al. (2021) show that ABA and NO can improve anthocyanins-related substance contents such as cyanidin 3-O-glucoside, petunidin 3-O-glucoside, malvidin 3-O-glucoside, peonidin 3-O-p-coumaroylglucoside, malvidin 3-O-p-coumaroylglucoside, revealing that ABA and NO can alleviate drought stress by the flavonoid accumulation pathway (Table 1; Figure 1C). Peng et al. (2022) identify 10 individual flavonoids (Cinnarnoyl-CoA, P-Cinnarnoyl-CoA, Naringenin chalcone, Naringenin, Dihydrokaempferol, Dihydroquercetin, Dihydromyricetin, Kaempferol, Quercetin, Myricetin) and flavonol biosynthesis pathway-related genes under freezing stress by using transcriptomics and metabolomics. Meanwhile, ABA biosynthesis-related genes and intermediates (β-Carotene, β-Cryptoxanthin, Zeaxanthin, Antheraxanthin, Violaxanthin, Neoxanthin, 9’-cis-Violaxanthin, 9’-cis-Neoxanthin, Xanthoxin, Abscisic aldehyde, Xanthoxic acid, and Abscisic alcohol) are significantly up-regulated as well as ABA production and low stomatal aperture. The expression level of NOS is also up-regulated, following the production of NO, arginine, and Nω-Hydroxy-arginine, implying that ABA-NO interaction relied on flavonoid metabolism to improve low-temperature tolerance (Table 1). In general, the detailed response mechanisms of flavonoids by crosstalk between ABA and NO remain to be understood.
3.6 Polyamines
PAs are considered antioxidant compounds, including Put, Spd, and Spm in plants. ABA and SNP treatments can increase the content of total PAs in rice seedlings, implying that ABA and NO can respond to salt stress through PAs pathway (Saha et al., 2022; Table 1; Figure 1C). Under cold stress, the expression level of MfSAMS1 in Medicago is promoted by ABA, H2O2, or NO, following the productions of Put, Spd, and Spm (Guo et al., 2014). Simultaneously, the above results are suppressed by ABA inhibitor naproxen, H2O2 scavenger DMTU, and NO scavenger cPTIO (Guo et al., 2014). The results suggest that the interaction between ABA and NO upregulates the expression of key gene for PAs to promote PAs generation under stressful conditions (Guo et al., 2014; Table 1; Figure 1C). Diao et al. (2017) reveal that the concentrations of Put, Spd, and Spm are increased by SNP treatment in tomato seedlings, following up-regulation of PAs biosynthesis-related gene expression. Moreover, when Spd and Spm are applied, NO and H2O2 contents are increased as well as LeNR upregulation (Diao et al., 2017; Table 1). Put treatment can improve ABA concentration and the ABA biosynthesis-related gene is also up-regulated, showing that PAs depend on NO, ABA, and H2O2 to improve cold tolerance. However, the specific mechanisms by which crosstalk between ABA and NO can affect endogenous PAs accumulation are still not clear and need further study.
3.7 Osmotic adjustment
Abiotic stresses usually induce water tolerance and accumulation for various organic and inorganic compounds contributing to osmotic adjustment in plants (Sahay et al., 2019; Pontin et al., 2021). ABA treatment is able to block the RWC reduction and promote the production of NO and H2O2 in triploid bermudagrass under drought stress, showing that ABA maintains water balance through NO and H2O2 (Lu et al., 2009; Table 1; Figure 1D). PEG induces the production of ABA, NO, and H2O2, following the low water content in Guzmania monostachia (Mioto and Mercier, 2013; Figure 1D). Planchet et al. (2014) observe that PEG promotes NO production as well as low water content in Medicago seedlings. The authors further found that cPTIO increases the water content by osmoregulators accumulation, such as amino acid, glutamate, and Pro (Planchet et al., 2014). In addition, NO is induced by ABA during the process. ABA and NO improve leaf RWC by Pro generation in drought-tolerance of Indian mustard seedlings (Sahay et al., 2019). Under drought stress, the water potential (Ψw) of grapevine seedlings is significantly reduced, which is reversed by ABA and NO (Pontin et al., 2021; Table 1; Figure 1D). Meanwhile, ABA and NO stimulate the production of organic acids, free sugars, diterpenes, triterpenes, sterols, anthocyanins, and polyphenols. The ratio of leaf water loss is reduced with the accumulation of ABA in wheat seedlings under mannitol-induced osmotic stress (Xing et al., 2004). In this process, when NO inhibitor LNAME or LNNA is applied, ABA content is decreased and water loss is elevated. The results suggest that ABA-induced NO promotes osmosis tolerance by maintaining water balance. ABA and NO can increase osmotic potential by promoting the accumulation of gamma aminobutyric acid, glycine betaine, alanine, and sucrose as well as Na+/K+ ratio, thus RWC enhancement in rice (Saha et al., 2022; Table 1). Similarly, ABA and NO decrease leaf water and stomatal aperture through improving K+, betaine, soluble protein, sugar, flavonoid, and Pro contents as well as reducing Na+ content under alkali stress (Zhang et al., 2023). Heat tolerance-induced osmolyte contents are raised by ABA and NO, including Pro, glycine betaine, trehalose, and soluble sugar in the leaves of wheat seedlings (Iqbal et al., 2022). However, ABA-induced osmolytes are inhibited by NO scavenger cPTIO. Although the studies described above suggest that crosstalk between ABA and NO can maintain water balance by regulating osmolytes, the mechanisms underlying the regulation of osmolytes by interaction between ABA and NO need to be further explored.
3.8 Photosynthesis
ABA, NO, and H2O2 can enhance phosphoenolpyruvate carboxylase (PEPC, an enzyme involved in photosynthesis) activity under environmental stress, revealing that they play a vital function in photosynthesis under abiotic tolerance (Lu et al., 2009; Mioto and Mercier, 2013). Under osmotic stress, chlorophyll (chl) content is elevated by ABA and NO (Pontin et al., 2021). This is the result of ABA and NO increasing water potential and thus promoting stomatal closure. ABA improves the low-light tolerance of tall fescue seedlings via enhancing photosynthesis, such as chl a, chl b, total carotenoids (CAR) contents, Pn, the maximum quantum yield of PSII photochemistry (Fv/Fm), actual photochemical efficiency of PSII (ΦPSII), photochemical quenching (qP), apparent electron transport rate (ETR), consequently, decreasing intercellular CO2 concentration (Ci) (Zhang et al., 2018b; Figure 1E). More importantly, NO and H2O2 are generated by ABA treatment during the process. The above results imply that NO and H2O2 contribute to ABA-activated photosynthesis. Iqbal et al. (2022) also report that heat-induced decrease chl content, Pn, stomatal conductance (gs), Ci, Fv/Fm, and rubisco activity, but ABA and NO reverse the effects, showing that ABA and NO can improve the heat-tolerance by enhancing photosynthetic capacity in wheat. However, the effects are worsened by ABA inhibitor Flu or NO scavenger cPTIO. Under alkali stress, photosynthetic capacity is reduced in Nitraria tangutorum seedlings (Zhang et al., 2023). However, ABA and NO increase in the levels of Chl a, Chl b, CAR contents, Fv/Fm, ETR, non-photochemical quenching (NPQ), ΦPSII, Pn and reduce levels of qP and Ci, therefore enhancement of photosynthesis. To summarize, the interaction between ABA and NO increases photosynthetic capacity by altering photosynthetic indexes, however, the relevant mechanisms are missing.
3.9 Stomatal movement
As a first line of defense, stomatal closure plays a vital in stress. It has been reported that ABA promotes stomatal closure in many plant species. Furthermore, previous studies demonstrate that ABA-induced stomatal closure is reversed by NO scavengers (Garcia-Mata and Lamattina, 2002; Neill et al., 2002b), thus NO is also involved in stomatal movement. However, this is a complex process in guard cells, with many signaling cascade involvement. For example, H2O2-induced stomatal closure is blocked by NO scavengers in Arabidopsis (Bright et al., 2006). Moreover, the functions of ABA and H2O2 in triggering stomatal closure are deficient in NO-deficient double mutant nia1nia2 in Arabidopsis. Interestingly, in NADPH oxidase deficient double mutant atrbohD/F, ABA-induced stomatal closure is inhibited with diminishing of NO accumulation (Bright et al., 2006). These results make it clear that ABA mediates H2O2-trigger NO to regulate stomatal closure. NO biosynthesis is enhanced by ABA thereby activating cADPR and cGMP (3’,5’-cyclic guanosine monophosphate) leading to stomatal closure in pea guard cells (Neill et al., 2002a; Figure 1A). The stomatal movement induced by ABA and calcium ions (Ca2+) is inhibited by BAPTA-AM (Ca2+ chelating agent) in broad bean guard cells (Garcia-Mata and Lamattina, 2007). However, NO-induced stomatal movement is not significantly inhibited during this process. In addition, Ca2+ depends on the enhancement of NOS-like activity to increase NO synthesis. ABA can activate the outward-rectifying K+ (GORK) channel causing stomatal closure in guard cells (Desikan et al., 2004; Xie et al., 2014). This is a result of ABA-induced H2O2 production, which is then involved in RbohF-dependent ROS and NR making NO accumulation. On the contrary, ABA signaling can be regulated negatively by NO through S-nitrosation that inhibits open stomatal 1 (OST1)/sucrose nonfermentable 1 (SNF1)-associated protein kinase 2.6 (SnRK2.6) in Arabidopsis guard cells (Wang et al., 2015; Figure 1A). Under alkali stress, crosstalk between ABA and NO can be reduced stomatal aperture by water regulation (Zhang et al., 2023). Gayatri et al. (2013) report that a signaling cascade under drought: ABA is the first accumulated; then RBOHD and RBOHF (respiratory burst oxidase homologs D and F) are activated leading to an increase in ROS; NO is generated through the activation of NR; mitogen-activated protein kinase (MAPK) signaling is activated by NO eventually resulting in stomatal closure (Figure 1A). In short, NO is essential for ABA-induced stomatal movement and requires the involvement of multiple signaling molecules. Nevertheless, we need to concentrate on the mechanisms by which crosstalk between ABA and NO regulates stomatal movement under more abiotic stresses, especially signaling cascades.
3.10 Post−translational modifications
S-nitrosylated proteins are detected in Arabidopsis, tobacco, and maize under drought or salt stress (Wawer et al., 2010; Mengel et al., 2013; Santisree et al., 2015), implying NO contributes to abiotic tolerance by mediating PTMs. Moreover, the effects of ABA on stomatal movement, growth inhibition, and seed dormancy are modulated by PTMs. For example, NO inhibits ABA-induced stomatal closure by activating S-nitrosation of SnRK2.6/OST1 at the cysteine137 site in Arabidopsis guard cells (Wang et al., 2015). NO reverses the effects of ABA-induced growth inhibition and seed dormancy of Arabidopsis via generating S-nitrosation of abscisic acid insensitive 5 (ABI5, the basic leucine zipper transcriptional factor, involved in the ABA synthesis) at cysteine153 (Albertos et al., 2015). Similarly, ABA is degraded by the tyrosine nitration of PYR/PYL/RCAR (ABA receptors) at tyrosine23 and tyrosine58 in Arabidopsis (Castillo et al., 2015). More importantly, NO drives S-nitrosation of APX1 (ascorbate peroxidase1) at cysteine32 to enhance ROS elimination, therefore improving abiotic tolerance in Arabidopsis (Yang et al., 2015). The above results imply that NO-mediated PTMs regulate the protein structure and function of ABA and antioxidant enzymes in response to abiotic stress. Additionally, the mechanism of linkage between ABA and NO is PTMs under abiotic stress. In fact, research that ABA-modulated abiotic tolerance involving S-nitrosation and tyrosine nitration is very poorly known. Therefore, we should focus on developing these areas of NO-mediated transcriptomics, proteomics, and PTMs.
4 Regulation of gene expression levels by ABA-NO interaction under environmental stresses
ABA-NO interaction alleviates environmental stresses via changing the levels of gene expression. For example, at the transcript level, ABA and NO up-regulate the relative expression of MSR-related genes OsMSRA4, OsMSRA5, OsMSRB1.1, OsMSRB3, and OsMSRB5 to prevent ROS from harming proteins and improve tolerance of NaCl in rice (Hsu and Lee, 2018; Figure 2). Furthermore, ABA inhibitor Flu and NO scavenger cPTIO can reverse the effects (Hsu and Lee, 2018). ABA relies on NO to notably up-regulate the expression of the antioxidant enzyme-related genes TaSOD, TaCAT, and TaAPX in wheat seedlings under heavy metal stress (Table 2; Figure 2). When ABA inhibitors are applied, their expression is down-regulated (Wu et al., 2018). Under low-light-induced oxidative stress, ABA also can improve levels of the antioxidant enzyme-related genes POD, CnZn-SOD, Mn-SOD, CAT-A, CAT-B, APX2, APX4, ABA receptor genes FaPYR1 and FaPYL1, but CAT-C is down-regulation (Zhang et al., 2018b; Table 2; Figure 2). The authors further found that the expression of a NOS-associated gene FaNOA1 is up-regulated by ABA and down-regulated by ABA inhibitor Flu, suggesting that ABA-mediated NO improves low-light tolerance by changing the expression of genes related to antioxidant enzymes (Table 2). Guo et al. (2014) report that the expression associated with PAs and ethylene biosynthesis gene MfSAMS1 is significantly up-regulated by ABA, SNP, and H2O2 treatments in Medicago under low-temperature stress. The transcript levels of PAs biosynthesis-related genes including PAO, CuAO1, CuAO2, SAMDC1, SAMDC2, SPDS1, SPDS2, SPMS, antioxidant enzyme-related genes Cu-SOD, Zn-SOD, CAT1, cAPX, cpAPX are up-regulated in overexpression of MfSAMS1 tobacco, indicating that MfSAMS1 gene is improved low-temperature tolerance through up-regulating antioxidant enzymes and polyamine oxidation (Guo et al., 2014; Table 2; Figure 2). The exogenous Spd or Spm can up-regulate the expression of the NO-related gene LeNR and downregulation of LeNOS along with NO generation in tomato seedlings under low-temperature stress (Diao et al., 2017; Table 2). Furthermore, Put treatment can up-regulate the expression of the ABA biosynthesis gene LeNCED1 as well as ABA production. When SNP is applied, LeADC, LeADC1, LeODC, and LeSPDS associated with polyamine biosynthesis are up-regulated (Diao et al., 2017; Table 2). The above results reveal that that PAs depend on ABA and NO to improve low-temperature tolerance by upregulating ABA and NO-related genes. When CPK27 (calcium-dependent protein kinases) is silenced in tomato under low-temperature stress, the activities of NADPH oxidase, NR, and MPK1/2 are suppressed, and the NO, ABA, and H2O2 contents are decreased (Lv et al., 2018; Table 2; Figure 2). But when exogenous ABA is used, the effects are overturned, showing that CPK27-dependent ABA responds to low-temperature stress via elevating the activity of NADPH oxidase, NR, and generating NO, H2O2. Peng et al. (2022) indicate that the gene expression associated with flavonol biosynthesis pathways such as C4H, CHS, CHI, F3H, F3’5’H, F3’H, and FLS are significantly up-regulated in the leaves of creeping lilyturf under freezing stress. Likewise, ABA biosynthesis pathway gene expression levels, for example, LUT5, ZEP, NCED, ABA2, and AAO3 are up-regulated as well as VED downregulation. In addition, NO content is increased, with NOS up-regulation and pheophorbide a oxygenase (PAO) down-regulation (Table 2; Figure 2). Exogenous ABA and NO enhance the levels of expression of genes related to NO biosynthesis NtNOA1, NtNR2, ABA biosynthesis NtNCED1/3/4/5, NtAAO, NtSDR, ABA signaling pathway NtPYL2/6, NtPP2C, NtABF1/3, and NtSnRK2.2/2.3 to increase endogenous ABA and NO contents in Nitraria tangutorum seedlings under alkali conditions (Zhang et al., 2023). Moreover, the expression of genes involved in plasma membrane-localized Na+/H+ antiporter NtSOS1, vacuolar Na+/H+ antiporter NtNHX1/2/3, K+/H+ reverse transporter NtKEA 3/5, other ion transporter NtKUP4, NtKCO, and NtHAK6/12 in roots are up-regulated by exogenous ABA and NO, following the down-regulation of the expression of NtHKT1, NtHAK6/12, NtKCO, and NtKUP4 in leaves. The results show that exogenous ABA and NO can promote the increase of endogenous ABA and NO contents, which further regulate ion transporters to alleviate alkali tolerance. Even though these specific genes are involved in the interaction between ABA and NO, the regulatory network is not understood.
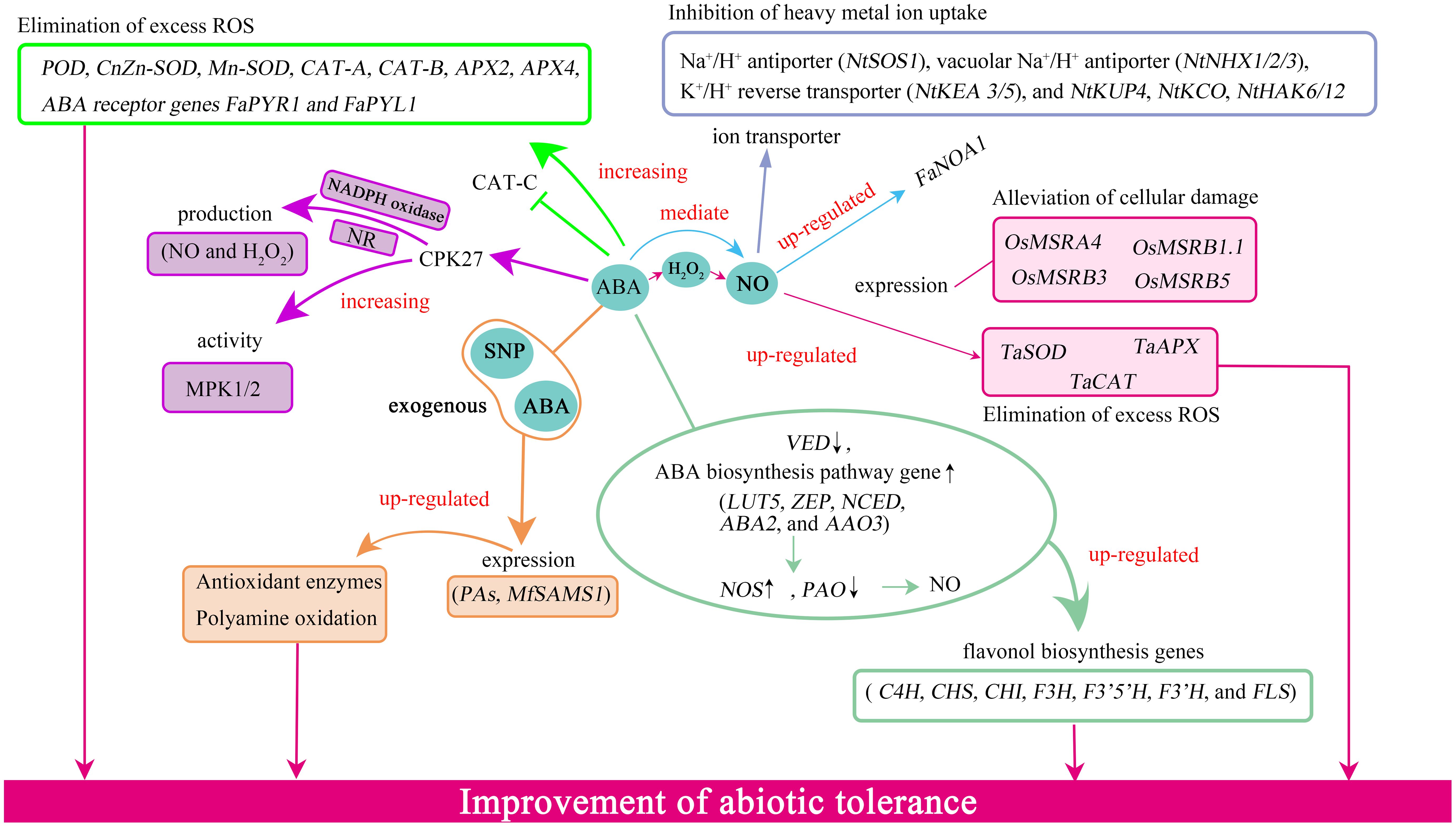
Figure 2 The interaction between ABA and NO regulated genes in plants under environmental stresses. ABA increased the expression levels of POD, CnZn-SOD, Mn-SOD, CAT-A, CAT-B, APX2, APX4, FaNOA1, FaPYR1, and FaPYL1 as well as NO and H2O2 production and NADPH oxidase, NR, and MPK 1/2 activities. ABA also up-regulated the expression of flavonol biosynthesis pathways- and ABA biosynthesis-related genes, down-regulated VED, reduced PAO activity, enhanced NOS-like activity as well as NO production. The interaction among ABA, NO, and H2O2 could up-regulate the expression of PAs-related genes as well as MfSAMS1. ABA-mediate NO up-regulated the expression of genes related to MSR and antioxidant enzymes. ABA, abscisic acid; NO, nitric oxide; SNP, sodium nitroprusside dihydrate; H2O2, hydrogen peroxide; PAs, polyamines; NADPH, nicotinamide adenine dinucleotide phosphate; NR, nitrate reductase-like; NOS-like, NO synthase; PAO, polyamine oxidase; MSR, methionine sulfoxide reductase A and B.
5 Conclusions and perspectives
Abiotic stresses present a great threat to the growth and development of plants. Growing attention is devoted to the role of interaction between ABA and NO in environmental stresses. The crosstalk between ABA and NO to alleviate multiple environmental stresses is discussed in this review, including drought, extreme temperature, salt, heavy metal, extreme light, UV-B, and alkali. ABA-NO crosstalk is potentially a strategy to enhance abiotic tolerance by modulating ROS, antioxidant enzymes, Pro, flavonoids, polyamines, ascorbate-glutathione cycle, water maintenance, photosynthesis, stomatal movement, and PTMs. Importantly, the review summarizes in detail that ABA-NO interaction can regulate some related genes to improve stress tolerance. These genes included those associated with MSR, antioxidant enzyme, ABA receptor, ABA biosynthesis, NO biosynthesis, PAs biosynthesis, calcium-dependent protein kinases, and flavonoid biosynthesis.
Although it is now known that crosstalk between ABA and NO plays a crucial role in conferring abiotic resistance in plants, many parts of this modulation are still missing. In the first place, the mechanisms of environmental perception by interaction between ABA and NO remain a topic of great interest. In addition, the regulatory mechanisms in multiple and simultaneous stresses need to be further explored. Thirdly, further studies are needed to investigate the signaling cascade mechanisms involved in abiotic and biotic stresses in plants, for example, Ca2+, K+, other signaling molecules, and plant hormones. Finally, the topic of NO-involved PTMs of specific proteins and specific sites is also a crucial subject. For the above, we will still face great challenges in solving these existential problems.
Author contributions
JX: Writing – original draft, Writing – review & editing. XL: Visualization, Writing – original draft. YL: Visualization, Writing – original draft. WL: Visualization, Writing – original draft. ZW: Visualization, Writing – original draft. WY: Funding acquisition, Project administration, Supervision, Writing – review & editing. CL: Project administration, Supervision, Writing – review & editing.
Funding
The author(s) declare financial support was received for the research, authorship, and/or publication of this article. This work was supported by National Nature Science Foundation of China (31660568), Guangxi science and technology major project (GuikeAA22068088), Science and technology major project of Guangxi (AA22068088-2), and start-up funding for introduced talents in Guangxi University (to CL).
Conflict of interest
The authors declare that the research was conducted in the absence of any commercial or financial relationships that could be construed as a potential conflict of interest.
Publisher’s note
All claims expressed in this article are solely those of the authors and do not necessarily represent those of their affiliated organizations, or those of the publisher, the editors and the reviewers. Any product that may be evaluated in this article, or claim that may be made by its manufacturer, is not guaranteed or endorsed by the publisher.
References
Albertos, P., Romero-Puertas, M. C., Tatematsu, K., Mateos, I., Sánchez-Vicente, I., Nambara, E., et al. (2015). S-Nitrosylation triggers ABI5 degradation to promote seed germination and seedling growth. Nat. Commun. 6, 8669. doi: 10.1038/ncomms9669
Berni, R., Luyckx, M., Xu, X., Legay, S., Sergeant, K., Hausman, J. F., et al. (2019). Reactive oxygen species and heavy metal stress in plants: impact on the cell wall and secondary metabolism. Environ. Exp. Bot. 161, 98–106. doi: 10.1016/j.envexpbot.2018.10.017
Bright, J., Desikan, R., Hancock, J. T., Weir, I. S., Neill, S. J. (2006). ABA-induced NO generation and stomatal closure in Arabidopsis are dependent on H2O2 synthesis. Plant J. 45, 113–122. doi: 10.1111/j.1365-313X.2005.02615.x
Castillo, M. C., Lozano-Juste, J., González-Guzmán, M., Rodriguez, L., Rodriguez, P. L., León, J. (2015). Inactivation of PYR/PYL/RCAR ABA receptors by tyrosine nitration may enable rapid inhibition of ABA signaling by nitric oxide in plants. Sci. Signal. 8, ra89. doi: 10.1126/scisignal.aaa7981
Desikan, R., Cheung, M. K., Bright, J., Henson, D., Hancock, J. T., Neill, S. J. (2004). ABA, hydrogen peroxide and nitric oxide signalling in stomatal guard cells. J. Exp. Bot. 55, 205–212. doi: 10.1093/jxb/erh033
Diao, Q. N., Song, Y. J., Shi, D. M., Qi, H. Y. (2017). Interaction of polyamines, abscisic acid, nitric oxide, and hydrogen peroxide under chilling stress in tomato (Lycopersicon esculentum Mill.) seedlings. Front. Plant Sci. 8. doi: 10.3389/fpls.2017.00203
Dong, N. G., Qi, J. X., Li, Y. F., Chen, Y. H., Hao, Y. B. (2017). Effects of abscisic acid and nitric oxide on chilling resistance and activation of the antioxidant system in walnut shoots in vitro. J. Am. Soc Hortic. Sci. 142, 376–384. doi: 10.21273/JASHS04197-17
Elsiddig, A. M. I., Zhou, G. S., Zhu, G. L., Nimir, N. E. A., Suliman, M. S. E., Ibrahim, M. E. H., et al. (2022). Nitrogen fertilizer promoting salt tolerance of two sorghum varieties under different salt compositions. Chil. J. Agr. Res. 83, 3–13. doi: 10.4067/S0718-58392023000100003
Gao, X. X., Liu, S. C., Zhang, Y. B., Guo, J. W., Fang, Z. C., Dao, J. M., et al. (2017). Differential analysis of endogenous hormone levels and natural defoliation traits during sugarcane (Saccharum spp.) maturation. Sugar Tech. 19, 41–50. doi: 10.1007/s12355-016-0441-2
Garcia-Mata, C., Lamattina, L. (2002). Nitric oxide and abscisic acid cross talk in guard cells. Plant Physiol. 128, 790–792. doi: 10.1104/pp.011020
Garcia-Mata, C., Lamattina, L. (2007). Abscisic acid (ABA) inhibits light-induced stomatal opening through calcium-and nitric oxide-mediated signaling pathways. Nitric. Oxide 17, 143–151. doi: 10.1016/j.niox.2007.08.001
Gayatri, G., Agurla, S., Raghavendra, A. S. (2013). Nitric oxide in guard cells as an important secondary messenger during stomatal closure. Front. Plant Sci. 4. doi: 10.3389/fpls.2013.00425
Gill, S. S., Tuteja, N. (2010). Reactive oxygen species and antioxidant machinery in abiotic stress tolerance in crop plants. Plant Physiol. Biochem. 48, 909–930. doi: 10.1016/j.plaphy.2010.08.016
Grun, S., Lindermayr, C., Sell, S., Durner, J. (2006). Nitric oxide and gene regulation in plants. J. Exp. Bot. 57, 507–516. doi: 10.1093/jxb/erj053
Gujjar, R. S., Akhtar, M., Singh, M. (2014). Transcription factors in abiotic stress tolerance. Ind. J. Plant Physiol. 19, 306–316. doi: 10.1007/s40502-014-0121-8
Guo, Z. F., Tan, J. L., Zhuo, C. L., Wang, C. Y., Xiang, X., Wang, Z. Y. (2014). Abscisic acid, H2O2 and nitric oxide interactions mediated cold-induced S-adenosylmethionine synthetase in Medicago sativa subsp. falcata that confers cold tolerance through up-regulating polyamine oxidation. Plant Biotechnol. J. 12, 601–612. doi: 10.1111/pbi.12166
He, H. Y., He, L. F., Gu, M. H., Li, X. F. (2012). Nitric oxide improves aluminum tolerance by regulating hormonal equilibrium in the root apices of rye and wheat. Plant Sci. 183, 123–130. doi: 10.1016/j.plantsci.2011.07.012
Hou, A. X., Tsuruta, H. (2003). Nitrous oxide and nitric oxide fluxes from an upland field in Japan: effect of urea type, placement, and crop residues. Nutr. Cycl. Agroecosys. 65, 191–200. doi: 10.1023/A:1022149901586
Hsu, Y. T., Lee, T. M. (2018). Abscisic acid-dependent nitric oxide pathway and abscisic acid-independent nitric oxide routes differently modulate NaCl stress induction of the gene expression of methionine sulfoxide reductase A and B in rice roots. J. Plant Physiol. 231, 374–382. doi: 10.1016/j.jplph.2018.10.019
Iqbal, N., Sehar, Z., Fatma, M., Umar, S., Sofo, A., Khan, N. A. (2022). Nitric oxide and abscisic acid mediate heat stress tolerance through regulation of osmolytes and antioxidants to protect photosynthesis and growth in wheat plants. Antioxidants 11, 372. doi: 10.3390/antiox11020372
Iqbal, N., Umar, S., Khan, N. A., Corpas, F. J. (2021). Crosstalk between abscisic acid and nitric oxide under heat stress: exploring new vantage points. Plant Cell Rep. 20, 1429–1450. doi: 10.1007/s00299-021-02695-4
Kermode, A. R. (2005). Role of abscisic acid in seed dormancy. J. Plant Growth Regul. 24, 319–344. doi: 10.1007/s00344-005-0110-2
Kreszies, T., Shellakkutti, N., Osthoff, A., Yu, P., Baldauf, J. A., Zeisler-Diehl, V. V., et al. (2018). Osmotic stress enhances suberization of apoplastic barriers in barley seminal roots: analysis of chemical, transcriptomic and physiological responses. New. Phytol. 221, 180–194. doi: 10.1111/nph.15351
Li, C. X., Yu, W. J., Wu, Y. C., Li, Y. Q. (2022). Roles of hydrogen gas in plants under abiotic stress: current knowledge and perspectives. Antioxidants 11, 1999. doi: 10.3390/antiox11101999
Li, H. Y., Zhang, W. Z. (2012). Abscisic acid-induced chilling tolerance in maize seedlings is mediated by nitric oxide and associated with antioxidant system. Appl. Materials Electron. Eng. Pts. 378-379, 423–427. doi: 10.4028/www.scientific.net/AMR.378-379.423
Lu, S. Y., Su, W., Li, H. H., Guo, Z. F. (2009). Abscisic acid improves drought tolerance of triploid Bermudagrass and involves H2O2- and NO-induced antioxidant enzyme activities. Plant Physiol. Biochem. 47, 132–138. doi: 10.1016/j.plaphy.2008.10.006
Lv, X. Z., Li, H. Z., Chen, X. X., Xiang, X., Guo, Z. X., Yu, J. Q., et al. (2018). The role of calcium-dependent protein kinase in hydrogen peroxide, nitric oxide and ABA-dependent cold acclimation. J. Exp. Bot. 69, 4127–4139. doi: 10.1093/jxb/ery212
Mengel, A., Chaki, M., Shekariesfahlan, A., Lindermayr, C. (2013). Effect of nitric oxide on gene transcription- S-nitrosylation of nuclear proteins. Front. Plant Sci. 4. doi: 10.3389/fpls.2013.00293
Mioto, P. T., Mercier, H. (2013). Abscisic acid and nitric oxide signaling in two different portions of detached leaves of guzmania monostachia with cam up-regulated by drought. J. Plant Physiol. 170, 996–1002. doi: 10.1016/j.jplph.2013.02.004
Mullineaux, P. M., Exposito-Rodriguez, M., Laissue, P. P., Smirnoff, N. (2018). ROS-dependent signalling pathways in plants and algae exposed to high light: comparisons with other eukaryotes. Free Radical Bio. Med. 122, 52–64. doi: 10.1016/j.freeradbiomed.2018.01.033
Muneer, S., Kim, T. H., Choi, B. C., Lee, B. S., Lee, J. H. (2014). Effect of CO, NOx and SO2 on ROS production, photosynthesis and ascorbate-glutathione pathway to induce Fragaria×annasa as a hyperaccumulator. Redox Biol. 16, 123–132. doi: 10.1016/j.redox.2013.12.006
Neill, S. J., Desikan, R., Clarke, A., Hancock, J. T. (2002a). Nitric oxide is a novel component of abscisic acid signaling in stomatal guard cells. Plant Physiol. 128, 13–16. doi: 10.1104/pp.010707
Neill, S. J., Desikan, R., Clarke, A., Hurst, R. D., Hancock, J. T. (2002b). Hydrogen peroxide and nitric oxide as signalling molecules in plants. J. Exp. Bot. 53, 1237–1247. doi: 10.1093/jxb/53.372.1237
Peng, Z., Wang, Y., Zuo, W. T., Gao, Y. R., Li, R. Z., Yu, C. X., et al. (2022). Integration of metabolome and transcriptome studies reveals flavonoids, abscisic acid, and nitric oxide comodulating the freezing tolerance in Liriope spicata. Front. Plant Sci. 12. doi: 10.3389/fpls.2021.764625
Piterkova, J., Luhova, L., Hofman, J., Tureckova, V., Novak, O., Petrivalsky, M., et al. (2012). Nitric oxide is involved in light-specific responses of tomato during germination under normal and osmotic stress conditions. Ann. Bot. 110, 767–776. doi: 10.1093/aob/mcs141
Planchet, E., Verdu, I., Delahaie, J., Cukier, C., Girard, C., Morere-Le Paven, M. C., et al. (2014). Abscisic acid-induced nitric oxide and proline accumulation in independent pathways under water-deficit stress during seedling establishment in Medicago truncatula. J. Exp. Bot. 65, 2161–2170. doi: 10.1093/jxb/eru088
Pontin, M., Murcia, G., Bottini, R., Fontana, A., Bolcato, L., Piccoli, P. (2021). Nitric oxide and abscisic acid regulate osmoprotective and antioxidative mechanisms related to water stress tolerance of grapevines. Aust. J. Grape Wine Res. 27, 392–405. doi: 10.1111/ajgw.12485
Prakash, V., Vi., P., Tripathi, D. K., Sharma, S., Corpasd., F. J. (2019). Crosstalk between nitric oxide (NO) and abscisic acid (ABA) signalling molecules in higher plants. Environ. Exp. Bot. 161, 41–49. doi: 10.1016/j.envexpbot.2018.10.033
Raschke, K., Firn, R. D., Pierce, M. (1975). Stomatal closure in response to xanthoxin and abscisic acid. Planta 125, 149–160. doi: 10.1007/BF00388701
Ruan, H. H., Shen, W. B., Xu, L. L. (2004). Nitric oxide involved in the abscisic acid induced proline accumulation in wheat seedling leaves under salt stress. Acta Bot. Sin. 46, 1307–1315.
Sadeghipour, O. (2017). Nitric oxide increases Pb tolerance by lowering pb uptake and translocation as well as phytohormonal changes in cowpea (Vigna unguiculata (L.) Walp.). Sains Malays. 46, 19–195. doi: 10.17576/jsm-2017-4602-02
Sah, S. K., Reddy, K. R., Li, J. (2016). Abscisic acid and abiotic stress tolerance in crop plants. Front. Plant Sci. 7. doi: 10.3389/fpls.2016.00571
Saha, I., Ghosh, A., Dolui, D., Fujita, M., Hasanuzzaman, M., Adak, M. K. (2022). Differential impact of nitric oxide and abscisic acid on the cellular and physiological functioning of sub1A QTL bearing rice genotype under salt stress. Plants 11, 1084. doi: 10.3390/plants11081084
Sahay, S., Gupta, M. (2018). Effect of nitric oxide and abscisic acid on growth determinants in Brassica juncea cultivars subjected to PEG-induced water stress. Plant Arch. 18, 737–744.
Sahay, S., Khan, E., Gupta, M. (2019). Nitric oxide and abscisic acid protects against PEG-induced drought stress differentially in Brassica genotypes by combining the role of stress modulators, markers and antioxidants. Nitric. Oxide 89, 81–92. doi: 10.1016/j.niox.2019.05.005
Santisree, P., Bhatnagar-Mathur, P., Sharma, K. K. (2015). NO to drought-multifunctional role of nitric oxide in plant drought: Do we have all the answers? Plant Sci. 239, 44–45. doi: 10.1016/j.plantsci.2015.07.012
Santos, M. P., Zandonadi, D. B., de Sa, A. F. L., Costa, E. P., de Oliveira, C. J. L., Perez, L. E. P., et al. (2020). Abscisic acid-nitric oxide and auxin interaction modulates salt stress response in tomato roots. Theor. Exp. Plant Physiol. 32, 301–313. doi: 10.1007/s40626-020-00187-6
Song, L. L., Ding, W., Shen, J., Zhang, Z. G., Bi, Y. R., Zhang, L. X. (2008). Nitric oxide mediates abscisic acid induced thermotolerance in the calluses from two ecotypes of reed under heat stress. Plant Sci. 175, 826–832. doi: 10.1016/j.plantsci.2008.08.005
Stromme, C., Sivadasan, U., Nissinen, K., Lavola, A., Randriamanana, T., Julkunen-Tiitto, R., et al. (2019). Interannual variation in UV-B and temperature effects on bud phenology and growth in Populus tremula. Plant Physiol. Biochem. 134, 31–39. doi: 10.1016/j.plaphy.2018.08.029
Tari, I., Guoth, A., Benyo, D., Kovacs, J., Poor, P., Wodala, B. (2010). The roles of ABA, reactive oxygen species and nitric oxide in root growth during osmotic stress in wheat: comparison of a tolerant and a sensitive variety. Acta Biol. Hung. 61, 189–196. doi: 10.1556/ABiol.61.2010.Suppl.18
Tossi, V., Lamattina, L., Cassia, R. (2009). An increase in the concentration of abscisic acid is critical for nitric oxide-mediated plant adaptive responses to UV-B irradiation. New. Phytol. 181, 871–879. doi: 10.1111/j.1469-8137.2008.02722.x
Wang, L., Zhao, R. R., Li, R., Yu, W. Q., Yang, M. J., Sheng, J. P., et al. (2018). Enhanced drought tolerance in tomato plants by overexpression of SlMAPK1. Plant Cell Tiss. Org. 133, 27–38. doi: 10.1007/s11240-017-1358-5
Wang, P., Du, Y., Hou, Y. J., Zhao, Y., Hsu, C. C., Yuan, F., et al. (2015). Nitric oxide negatively regulates abscisic acid signaling in guard cells by S-nitrosylation of OST1. Proc. Natl. Acad. Sci. U. S. A. 112, 613–618. doi: 10.1073/pnas.1423481112
Wawer, I., Bucholc, M., Astier, J., Anielska-Mazur, A., Wendehenne, D. (2010). Regulation of Nicotiana tabacum osmotic stress-activated protein kinase and its cellular partner GAPDH by nitric oxide in response to salinity. Biochem. J. 429, 73–83. doi: 10.1042/BJ20100492
Wu, S. W., Hu, C. X., Tan, Q. L., Zhao, X. H., Xu, S. J., Xia, Y. T., et al. (2018). Nitric oxide acts downstream of abscisic acid in molybdenum-induced oxidative tolerance in wheat. Plant Cell Rep. 37, 599–610. doi: 10.1007/s00299-018-2254-0
Xie, Y., Mao, Y., Zhang, W., Lai, D., Wang, Q., Shen, W. (2014). Reactive oxygen speciesdependent nitric oxide production contributes to hydrogen-promoted stomatal closure in Arabidopsis. Plant Physiol. 165, 759–773. doi: 10.1104/pp.114.237925
Xing, H., Tan, L. L., An, L. H., Zhao, Z. G., Wang, S. M., Zhang, C. L. (2004). Evidence for the involvement of nitric oxide and reactive oxygen species in osmotic stress tolerance of wheat seedlings: inverse correlation between leaf abscisic acid accumulation and leaf water loss. J. Plant Growth Regul. 42, 61–68. doi: 10.1023/B:GROW.0000014894.48683.1b
Xu, Y. F., Fu, J. J., Chu, X. T., Sun, Y. F., Zhou, H., Hu, T. M. (2013). Nitric oxide mediates abscisic acid induced light-tolerance in leaves of tall fescue under high-light stress. Sci. Hortic. 162, 1–10. doi: 10.1016/j.scienta.2013.07.042
Xu, J. R., Wei, Z. E., Lu, X. F., Liu, Y. Z., Yu, W. J. (2023). Involvement of nitric oxide and melatonin enhances cadmium resistance of tomato seedlings through regulation of the ascorbate–glutathione cycle and ROS metabolism. Int. J. Mol. Sci. 24, 9526. doi: 10.3390/ijms24119526
Yang, H., Mu, J., Chen, L., Feng, J., Hu, J., Li, L., et al. (2015). S-nitrosylation positively regulates ascorbate peroxidase activity during plant stress responses. Plant Physiol. 167, 1604–1615. doi: 10.1104/pp.114.255216
Yu, X. F., Yang, Z. H., Xu, Y. H., Wang, Z. W., Fan, C. Y., Zeng, X. X., et al. (2023). Effect of chromium stress on metal accumulation and cell wall fractions in Cosmos bipinnatus. Chemosphere 315, 137677. doi: 10.1016/j.chemosphere.2022.137677
Zhang, J., Cheng, K., Liu, X. Y., Dai, Z. C., Zheng, L. L., Wang, Y. C. (2023). Exogenous abscisic acid and sodium nitroprusside regulate flavonoid biosynthesis and photosynthesis of Nitraria tangutorum Bobr in alkali stress. Front. Plant Sci. 14. doi: 10.3389/fpls.2023.1118984
Zhang, X. H., Liu, Y. H., Liu, Q., Zong, B., Yuan, X. P., Sun, H. E., et al. (2018b). Nitric oxide is involved in abscisic acid-induced photosynthesis and antioxidant system of tall fescue seedlings response to low-light stress. Environ. Exp. Bot. 155, 226–238. doi: 10.1016/j.envexpbot.2018.07.001
Zhang, W., Lu, L. Y., Hu, L. Y., Cao, W., Sun, K., Sun, Q. B., et al. (2018a). Evidence for the involvement of auxin, ethylene and ROS signaling during primary root inhibition of Arabidopsis by the allelochemical benzoic acid. Plant Cell Physiol. 59, 1889–1904. doi: 10.1093/pcp/pcy107
Zhang, Q. T., Zhang, L. L., Geng, B., Feng, J. R., Zhu, S. H. (2019). Interactive effects of abscisic acid and nitric oxide on chilling resistance and active oxygen metabolism in peach fruit during cold storage. J. Sci. Food Agric. 99, 3367–3380. doi: 10.1002/jsfa.9554
Keywords: environmental stresses, regulatory pathways, metabolic pathways, abscisic acid, nitric oxide, crosstalk
Citation: Xu J, Lu X, Liu Y, Lan W, Wei Z, Yu W and Li C (2024) Interaction between ABA and NO in plants under abiotic stresses and its regulatory mechanisms. Front. Plant Sci. 15:1330948. doi: 10.3389/fpls.2024.1330948
Received: 31 October 2023; Accepted: 25 April 2024;
Published: 17 May 2024.
Edited by:
Khalid Rehman Hakeem, King Abdulaziz University, Saudi ArabiaReviewed by:
M. Nasir Khan, University of Tabuk, Saudi ArabiaHamid Manzoor, Bahauddin Zakariya University, Pakistan
Copyright © 2024 Xu, Lu, Liu, Lan, Wei, Yu and Li. This is an open-access article distributed under the terms of the Creative Commons Attribution License (CC BY). The use, distribution or reproduction in other forums is permitted, provided the original author(s) and the copyright owner(s) are credited and that the original publication in this journal is cited, in accordance with accepted academic practice. No use, distribution or reproduction is permitted which does not comply with these terms.
*Correspondence: Changxia Li, bGljeEBneHUuZWR1LmNu
†ORCID: Changxia Li, orcid.org/0000-0001-8570-4653