- College of Landscape Architecture and Art, Northwest A&F University, Xianyang, China
Introduction: In recent years, the visible light intensity of lawns has significantly decreased due to obstructions caused by urban shading objects. Carex has a competitive advantage over other turfgrass in low-light conditions and extensive management. Therefore, exploring their survival strategy in low-light environments is of great significance.
Methods: This study focuses on two species of Carex, Carex parva and Carex scabrirostris, and investigates their response to low-light conditions (150 μmol/m2/s) by simulating urban lawn conditions. Biomass allocation characteristics, leaf anatomical features, biochemical parameters, root morphology and photosynthetic parameters were measured.
Results: (a) Peroxidase activity, specific leaf area, and relative water content are key factors influencing the photosynthetic capacity of the two Carex species. (b) Under low-light conditions, photosynthetic parameters, leaf physiological indicators, and biomass allocation of the two Carex species were significantly affected (p<0.05). Both Carex species increased their investment in leaf biomass, maintained lateral root growth, and cleared reactive oxygen species to maintain their physiological balance. (c) In the simulated urban low-light environment, neither C. parva nor C. scabrirostris produced dauciform roots.
Discussion: In terms of response strategies, C. scabrirostris is a high-photosynthesis investing species with high productivity under low-light conditions, whereas C. parva exhibits minimal response, indicating a slow investment. C. scabrirostris has greater potential for application in low-light environments compared to C. parva. These results provide a theoretical basis for the cultivation and application of these two Carex species, as well as the expansion of turfgrass germplasm resources.
1 Introduction
In recent years, lawns have played an increasingly significant role in landscape greening (Thompson and Kao-Kniffin, 2019), providing not only social benefits and ecosystem services to the urban environment but also substantial economic and ecological benefits (Trammell et al., 2019). Currently, urban buildings, artificial structures, and dense tree canopies have created numerous shaded areas, resulting in a significant reduction in visible light intensity within cities (Francini et al., 2023). However, most types of turfgrass do not adapt well to excessively shaded environments (Zhang et al., 2016). Given that light conditions are an important factor restricting turfgrass growth in urban environments, selecting a shade-tolerant turfgrass species is imperative (Fu et al., 2020).
Carex, one of the most ecologically diverse genera (Jakovljević et al., 2014), is currently being used in urban lawns, and their growth status is being explored (Shokoya et al., 2022). Compared with other turfgrasses, the Carex genus has the advantage of growing in low-light or low-maintenance conditions (Więcław, 2017). It has been found that the Carex genus produces dauciform roots (Güsewell and Schroth, 2017), which are effective in absorbing water and nutrients from deeper soils and storing large amounts of nutrients in infertile soils, providing a stable source of nutrients for plant growth (Shane et al., 2005). Research has found that the Carex genus can produce dauciform roots (Güsewell and Schroth, 2017), which are capable of effectively absorbing water and nutrients from deep soil layers. As a result, dauciform roots can store large amounts of nutrients in infertile soils, providing a stable nutrient source for plant growth. Previous studies have found that Carex species with dauciform root systems are more likely to occur in areas with high light intensity and lower phosphorus availability (Playsted et al., 2006; Brundrett, 2009). However, the formation of dauciform roots under low-light conditions in urban lawns is mainly unknown.
Typical responses of plants to low-light environments include an increase in aboveground biomass, thinner leaves, and a larger specific leaf area (SLA) (Milla and Matesanz, 2017). Additionally, low-light conditions reduce the maximum carboxylation capacity (Vcmax), maximum electron transport rate (Jmax), light saturation point (LSP), and net photosynthetic rate (Pnmax) (Haque et al., 2017; Fu et al., 2017; Sun et al., 2023). These changes are the result of the long-term coordination between leaf functional traits and photosynthesis (Nam et al., 2017; Wright et al., 2004). Leaf anatomical structure reflects important photosynthetic physiological characteristics (Maza-Villalobos et al., 2022). Wang et al. (2023) studied factors influencing plant photosynthetic capacity based on leaf anatomy. It is important to select Carex species that can efficiently utilize light energy, considering that Carex species lack palisade tissue in mesophyll cells (Wang et al., 2023).
Plants also adapt to low-light conditions by altering their physiological and metabolic processes (Zhang et al., 2022; Kittipornkul et al., 2023). Specifically, the chlorophyll content decreases while osmoregulatory substances accumulate, and antioxidant enzyme activity increases (Wu, 2016; Wu et al., 2021). In the absence of light, plants secrete many osmoregulatory substances to maintain normal osmotic pressure in the cytoplasm (Huang et al., 2022). Lower light will imbalance the internal scavenging system of reactive oxygen species, leading to membrane lipid peroxidation (Liang et al., 2009; Zhang et al., 2022). Antioxidant enzymes, such as peroxidase (POD), can eliminate reactive oxygen species, maintaining redox homeostasis (Liu et al., 2021).
As a significant concept in ecology, the leaf economics spectrum reveals the coordination and trade-offs among various plant traits (Wright et al., 2004). Plants adopt different investment strategies in low-light environments (Wright et al., 2004). Plants that prioritize rapid return on investment often have larger and thinner leaves, whereas plants employing resource-storage strategies exhibit contrasting characteristics (Jiang et al., 2023). These traits result from the ongoing interactions between plants and their environments over time (Violle et al., 2007). Similarly, the same species may exhibit different structural characteristics in different environments (Hu et al., 2022). Studying the variability and plasticity of plant traits also contributes to understanding their growth strategies under different environmental conditions (Fajardo and Siefert, 2016; Lafont Rapnouil et al., 2023). Additionally, plant functional traits can be categorized into soft traits and hard traits. In order to obtain certain key physiological traits that are difficult to measure in real time in the field, such as indicators related to photosynthesis, other easily observable soft traits can be used as proxies (Cornelissen et al., 2003). Since different plant organs and tissues respond differently to environmental changes (Funk and Cornwell, 2013), it is of significant importance to identify functional trait indicators in Carex species that are associated with the efficient utilization of light energy.
C. parva is a perennial herb of the genus Carex in the family Cyperaceae. It has high resistance to drought and shaded conditions, making it suitable for extensive management. It is also a turfgrass species with excellent potential for urban areas (Dai et al., 2010). C. scabrirostris, an endemic species of high research value in China, commonly grows alongside C. parva (Dai et al., 2010). Currently, there are limited studies on the environmental adaptability and survival strategies of these two Carex species (Wang et al., 2023). Out of 865 species of sedge plants in China, only three, including C. parva, have been observed to develop dauciform roots in their natural habitats (Gao and Yang, 2016). Further exploring the excellent potential and application value of Carex species in low-light environments and providing parental materials for domesticating and introducing urban lawns in China.
This study aims to investigate the response changes of C. parva and C. scabrirostris under low-light environments, as well as their different growth strategies in heterogeneous environments. Specifically, we propose and explore the following questions: (a) Can we identify the key factors influencing the photosynthetic capacity of these two species of Carex? (b) How do the responses of these two Carex species change in simulated low-light urban environments, and how do they adapt to low-light environments? (c) Do these two species of Carex have different growth strategies under two different light environments, and which Carex has a broader range of potential applications in low-light environments? This study further explores the excellent potential and application value of Carex species in low-light environments, providing parental material and preliminary theoretical support for the introduction and domestication of these species in urban lawns in China.
2 Materials and methods
2.1 Study area
The plant materials used in this experiment were C. parva and C. scabrirostris, which belong to the genus Carex in the family Cyperaceae. They were collected from the Taibai Mountains (34°10’N, 107°58’E), a predominantly high-altitude grassland situated at an elevation of 3,620 m (Dai et al., 2010). The area experiences an annual precipitation of 751.8 mm, with a yearly relative humidity of 70% and an average annual temperature of 11°C (Cao et al., 2016). We selected three sample areas in the Taibai Mountains, with a distance of 20 m between each sample area. Each area was dug up to form three sample clusters measuring 20 cm × 20 cm × 20 cm (length, width, and depth) (Falcioni et al., 2017). Soil samples were taken at the four corners and the central area of the sample plot using the plum 5-point method (Falcioni et al., 2017).
The plant materials were stored in the climate chamber of Northwest A&F University (34°26’N, 108°06’E). The chamber was established under the following conditions: light duration of 14 h day/10 h night, day/night temperature of 25/18°C, light intensity of 150 μmol/m²/s, air relative humidity controlled at 65%–75%, and soil moisture maintained consistent with the original habitat. The parameters were chosen based on the shaded lawn environments in Shaanxi as the main reason (Wang et al., 2023). The experiment was conducted during the optimal growth season for the selected plants, which was late July.
2.2 Sampling and measurements
After being collected from the field, some plants were immediately processed upon arrival at the laboratory, while the remaining plants were kept in a growth chamber to simulate a low-light environment for 80 days before processing. Five randomly selected samples of each species were harvested for analysis. During the harvesting process, plant photosynthesis was measured, including the light response curve and the CO2 response curve, using the LI-6400XT (LI-COR, Lincoln, NE, USA). Photosynthetically active radiation (PAR) levels were set at 2000, 1500, 1200, 1000, 750, 500, 250, 200, 150, 100, 50, 25, and 0 μmol m−2 s−1, with a CO2 concentration of 400 μmol mol−1. The CO2 concentration in the sample chamber was varied between 400, 300, 200, 150, 100, and 50 μmol mol−1, and then set back to 400, 600, 800, 1000, 1200, 1500, and 2000 μmol mol−1, with a constant PAR of 1000 μmol mol−1. The hyperbolic tangent model was used to fit indices such as the LSP, light compensation point (LCP), dark respiration rate (RD), maximum carboxylation rate (Vcmax), and maximum electron transport rate (Jmax) (Wang et al., 2023).
Plant morphological indices were measured next. Leaf area was measured using the LI-3000C portable leaf area meter. Three mature leaves were randomly selected, and their length, average width, maximum width, and leaf area were measured. Using a caliper (De Antonio et al., 2023), the thickness of the leaf on the same side as the main vein was measured, and the fresh weight and dry weight of these leaves were recorded. Additionally, the anatomical and structural characteristics of leaf sections were observed using a MoticBA410 microscope (Jiang et al., 2023). Images were captured, and parameters such as upper epidermis thickness, lower epidermis (LET) thickness, and cuticle thickness (CUT) were documented. Root morphology, including root length, root volume, and root surface area, was measured using the Winrhizo software. Among the 175 specimens of C. parva and 76 specimens of C. scabrirostris collected in the field, 0 and 64 plants with dauciform roots (lateral roots with swollen axes) were observed, respectively. The aboveground parts of the plants were separated on a quartz surface, and their fresh weight and dry weight were measured individually using an electronic balance.
Last, physiological indices of the plants were measured. Chlorophyll a and b were extracted from fresh leaves using 95% (v/v) ethanol according to Lichtenthaler and Wellburn (1983). Malondialdehyde (MDA) content was determined using the thiobarbituric acid method, proline mass fraction using the sulfosalicylic acid extraction ninhydrin colorimetric method, Soluble protein (SP) content using the Coomassie Brilliant Blue method, and POD content using the guaiacol method (Weng et al., 2015).
2.3 Statistical analysis
Data analysis for this study was conducted using SPSS 19.0, and graphs were generated using Origin 22. The normality of all data was assessed using the Shapiro–Wilk test, and the homogeneity of variances was assessed using Levene’s test. In case the results did not follow a normal distribution, a square root transformation was applied to achieve normality. Fisher’s LSD analysis was used to determine the statistical significance of differences between treatments (p < 0.05). To assess the variability of plant functional traits between species, the coefficient of variation (CV) was calculated using the formula CV = (SD ÷ M) × 100%, where SD is the standard deviation and M is the mean. Traits with a CV exceeding 50% were considered ecologically adaptive traits, while traits with a lower CV served as indicators of systematic evolution, reflecting species’ adaptive potential (Zhang et al., 2021). Additionally, the plasticity index (PI) was used to characterize the response of two Carex species to different environments. It was calculated as PI = (max − min)/max, where max and min represent the maximum and minimum values of a certain trait. Traits with PI > 0.6 were defined as sensitive indicators of habitat response, while traits with PI < 0.2 were considered inert indicators of habitat response. Spearman correlation analysis was used to examine the relationships among plant functional traits. To further screen relevant indicators for the efficient utilization of light in two types of Carex, redundancy analysis (RDA) was performed on nine functional trait indicators. Detrended correspondence analysis (DCA) was conducted on RDA data (Grinn-Gofroń et al., 2018). Indicators were selected based on causality and correlation analysis, excluding those with poor response and indicators directly derived from basic indicators (Flexas et al., 2022). RDA was used to explore the associations between photosynthetic traits and plant physiological ecology, ranking the contribution values for each indicator (Liu et al., 2021). Finally, the RDA results were compared and validated with the corresponding correlation analysis to ensure the accuracy of the findings (Dong et al., 2022).
3 Result
3.1 Changes in photosynthetic parameters
The light response curves of C. parva and C. scabrirostris exhibited similar variations (Figure 1A). Overall, regardless of the environment, C. scabrirostris displayed greater photosynthetic capacity than C. parva (Figure 1A; Table 1). Under low-light conditions, the LSP of C. parva increased significantly, while that of C. scabrirostris decreased significantly (p < 0.05) (Table 1). Both C. parva and C. scabrirostris exhibited significant reductions in RD under low-light conditions. Compared to their natural habitats, both Carex species showed increased stomatal conductance (Gs) and transpiration rates (Tr) under low-light conditions (Figures 1C, E). However, in low-light conditions, the intercellular CO2 concentration (Ci) decreased in C. parva, while C. scabrirostris exhibited higher Ci levels at PAR < 800 (Figure 1D). Additionally, under PAR < 500, C. scabrirostris showed the highest light use efficiency (LUE) among the Carex species under low-light conditions (Figure 1F).
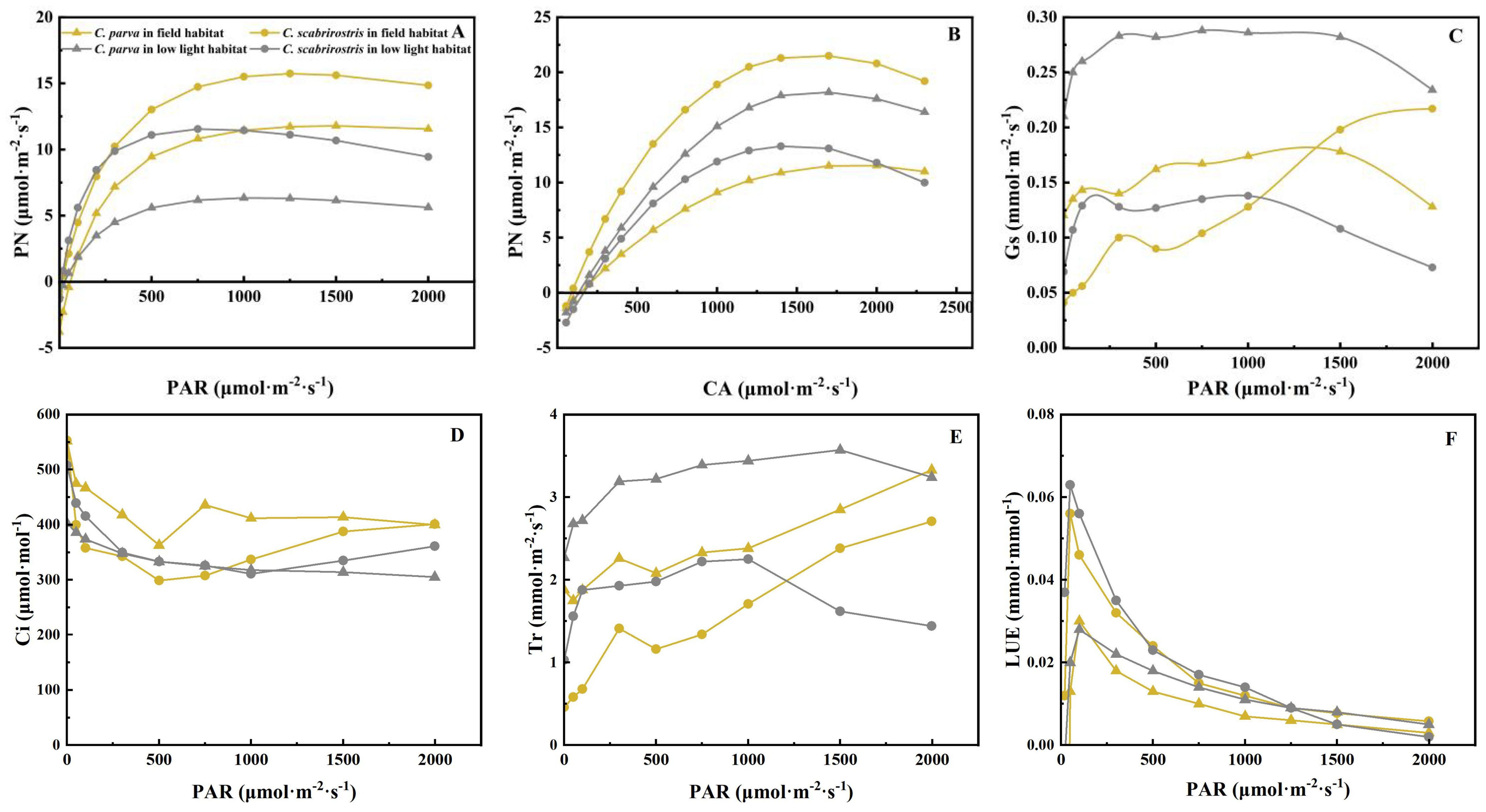
Figure 1. Light use efficiency of C. parva and C. scabrirostris with different photosynthetically active radiation. (A) Light response curves; (B) carbon dioxide response curves; (C) stomatal conductance; (D) transpiration rate; (E) intercellular CO2 concentration; (F) PN, net photosynthetic rate; PAR, photosynthetically active radiation; CA, air carbon dioxide concentration; Gs, Stomatal conductance; Tr, Transpiration rate; Ci, Intercellular CO2 concentration; LUE, light use efficiency.
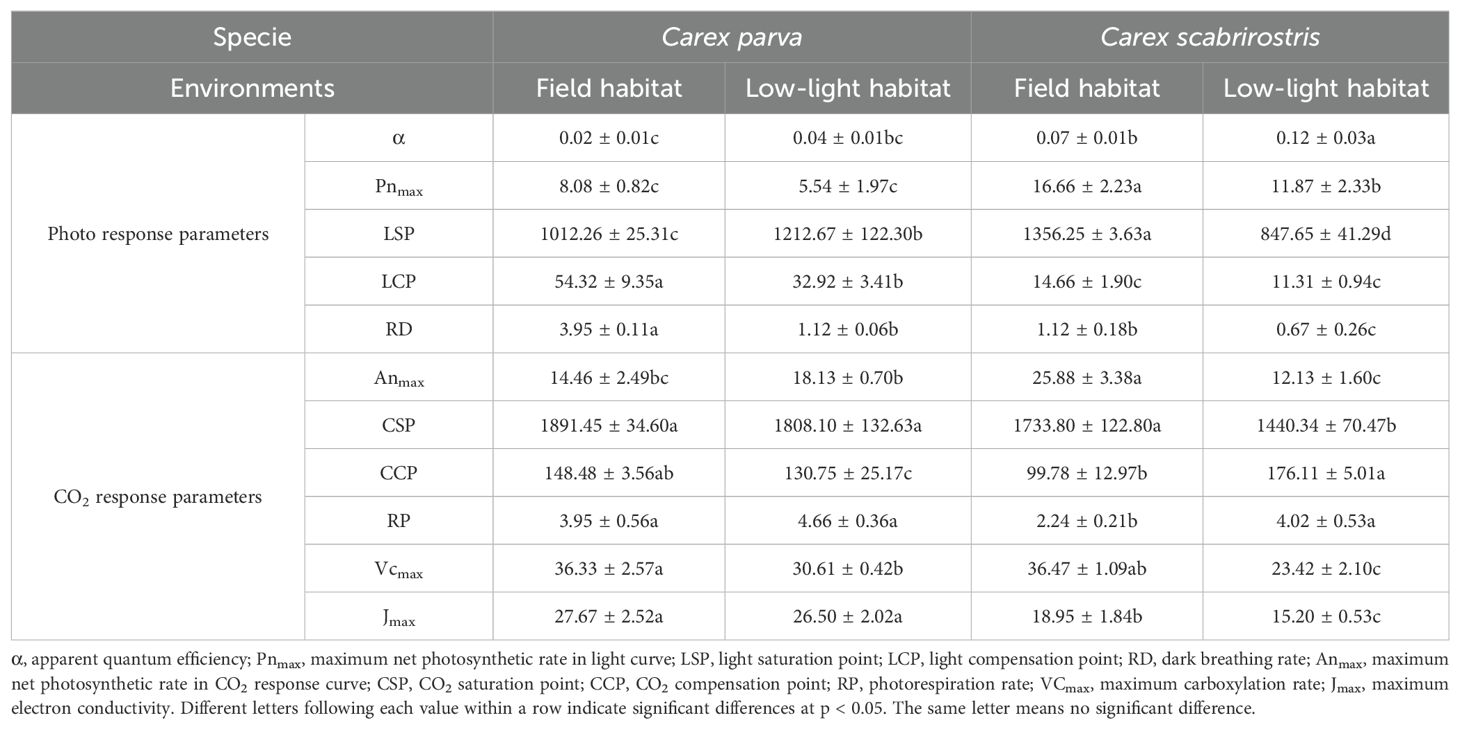
Table 1. Light response curve parameters, CO2 response curve parameters of Carex parva and Carex scabrirostris.
Additionally, akin to the changes observed in the light response curve, C. scabrirostris demonstrated a higher responsiveness to CO2 compared to C. parva (Figure 1B; Table 1). Anmax, CSP, VCmax, and Jmax showed significant reductions in C. scabrirostris, while CCP and RP exhibited significant increases. Conversely, in C. parva, CCP, and VCmax experienced significant decreases, while the other variables exhibited minimal or no response (Table 1).
3.2 Changes of plant morphology
Both Carex species significantly increased their aboveground biomass and decreased their belowground biomass under low-light conditions, leading to a reduced root-shoot ratio (Figures 2A, B). Morphologically, the leaves of both Carex species significantly elongate in response to low-light conditions (Figure 3C). In this setting, the leaf area of C. parva significantly increased, whereas the SLA remained relatively unchanged. Conversely, the leaf area of C. scabrirostris decreased significantly, accompanied by a notable increase in SLA (Figures 3A, B).
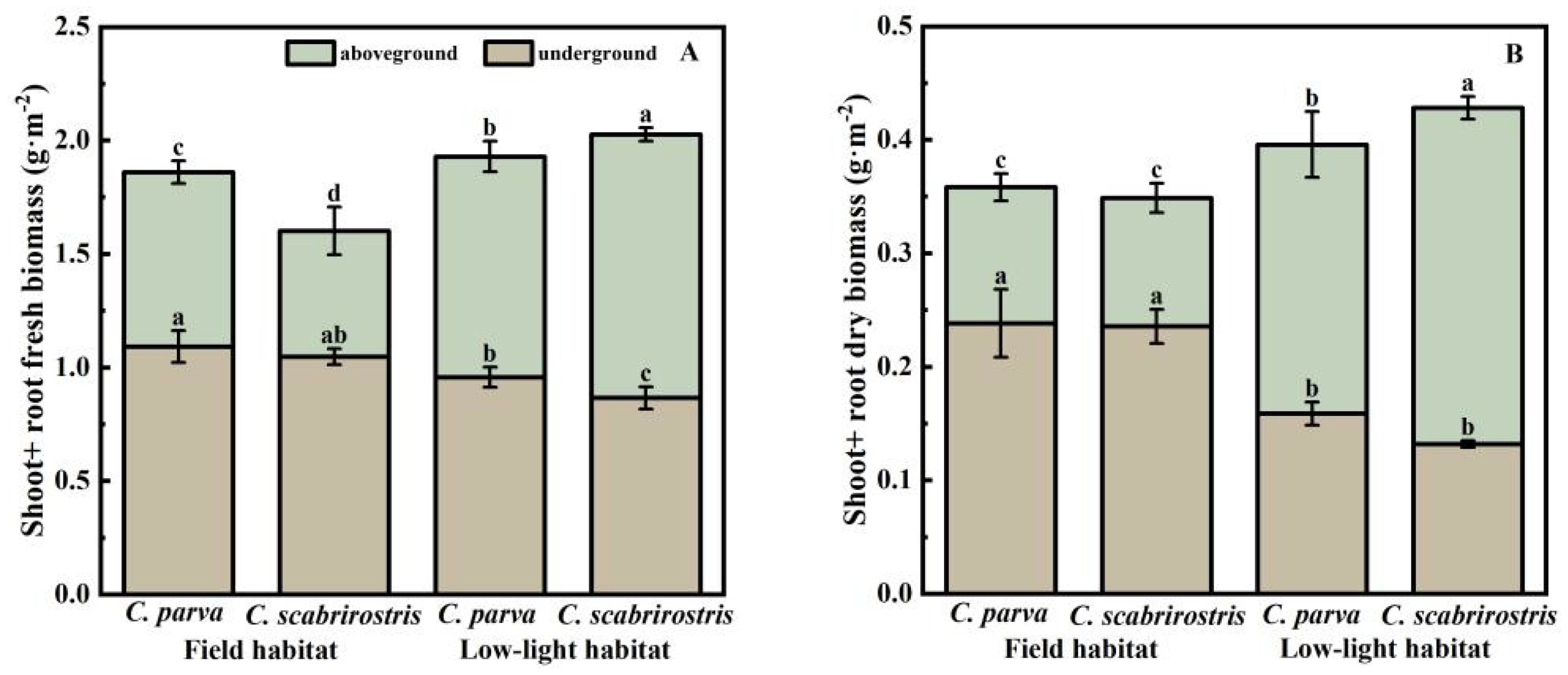
Figure 2. The effect of different environments on the aboveground and underground biomass changes in C. parva and C. scabrirostris. Different letters indicate significant differences in means between treatments based on ANOVA. Bars represent Means ± SE (standard errors). (A) is the aboveground and underground fresh biomass, (B) is the aboveground and underground dry biomass.
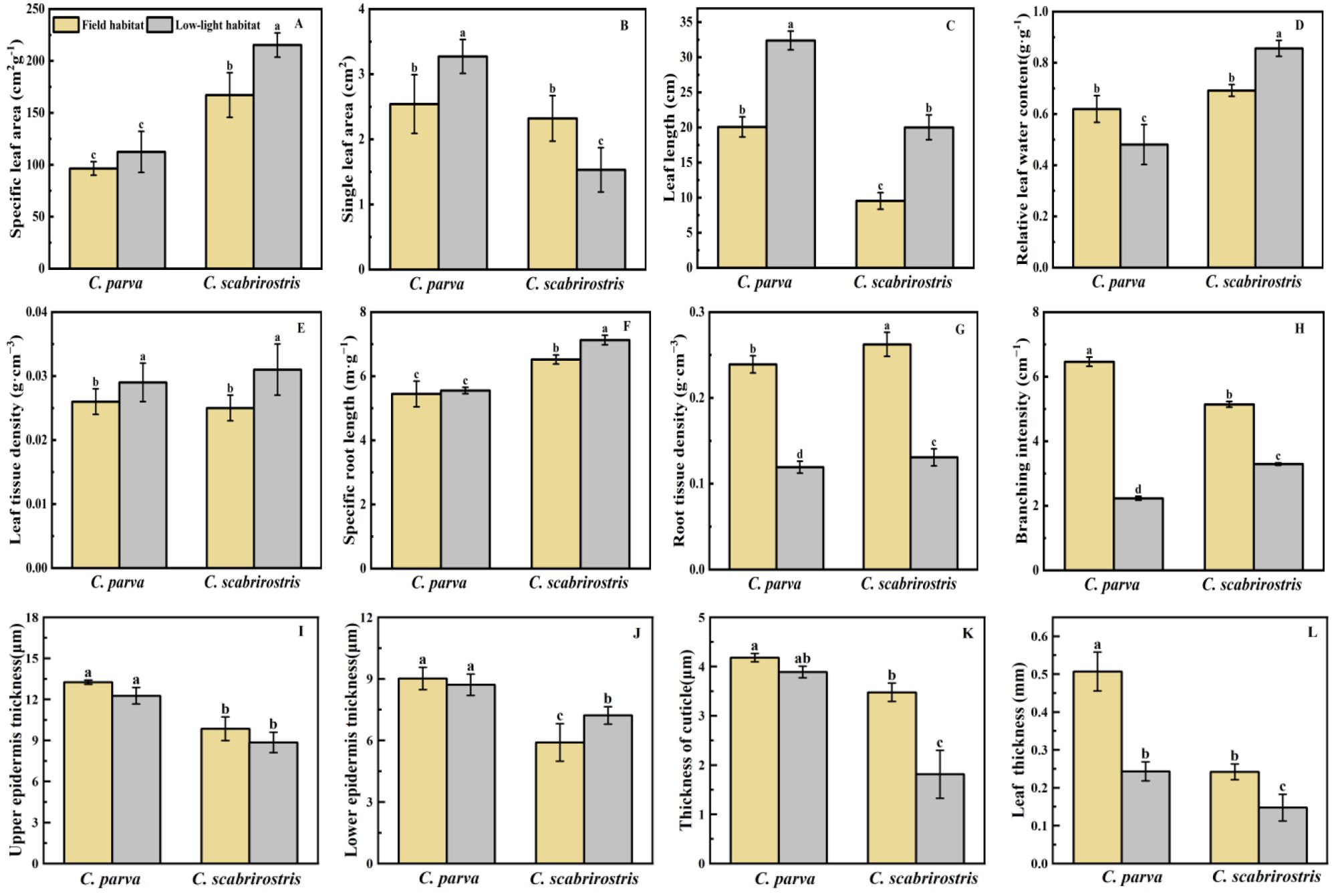
Figure 3. Effects of different environments on the morphological changes and leaf anatomical indices of C. parva and C. scabrirostris. Different letters indicate significant differences in means between treatments based on ANOVA. Bars represent Means ± SE (standard errors). (A) is the specific leaf area, (B) is the single leaf area, (C) is the leaf length, (D) is the relative leaf water content, (E) is the leaf tissue density, (F) is the specific root length, (G) is the root tissue density, (H) is the branching intensity, (I) is the upper epidermis thickness, (J) is the lower epidermis thickness, (K) is the thickness of cuticle, (L) is the leaf thickness.
Concerning leaf relative water content (LRWC), the two Carex species exhibited distinct trends. LRWC decreased significantly in C. parva, whereas it significantly increased in C. scabrirostris (Figure 3D). Leaf tissue density (LTD) significantly increased in both C. parva and C. scabrirostris under low-light conditions (Figure 3E). Figure 4 illustrates that under low-light conditions, the LET of C. scabrirostris increased significantly, while the CUT of both Carex species decreased significantly to varying degrees (p < 0.05). No significant changes were observed in the leaf dissection of C. parva (Figures 4A–C). Both C. parva and C. scabrirostris exhibited significantly thinner leaf thickness (LT) under low-light conditions (Figure 4D).
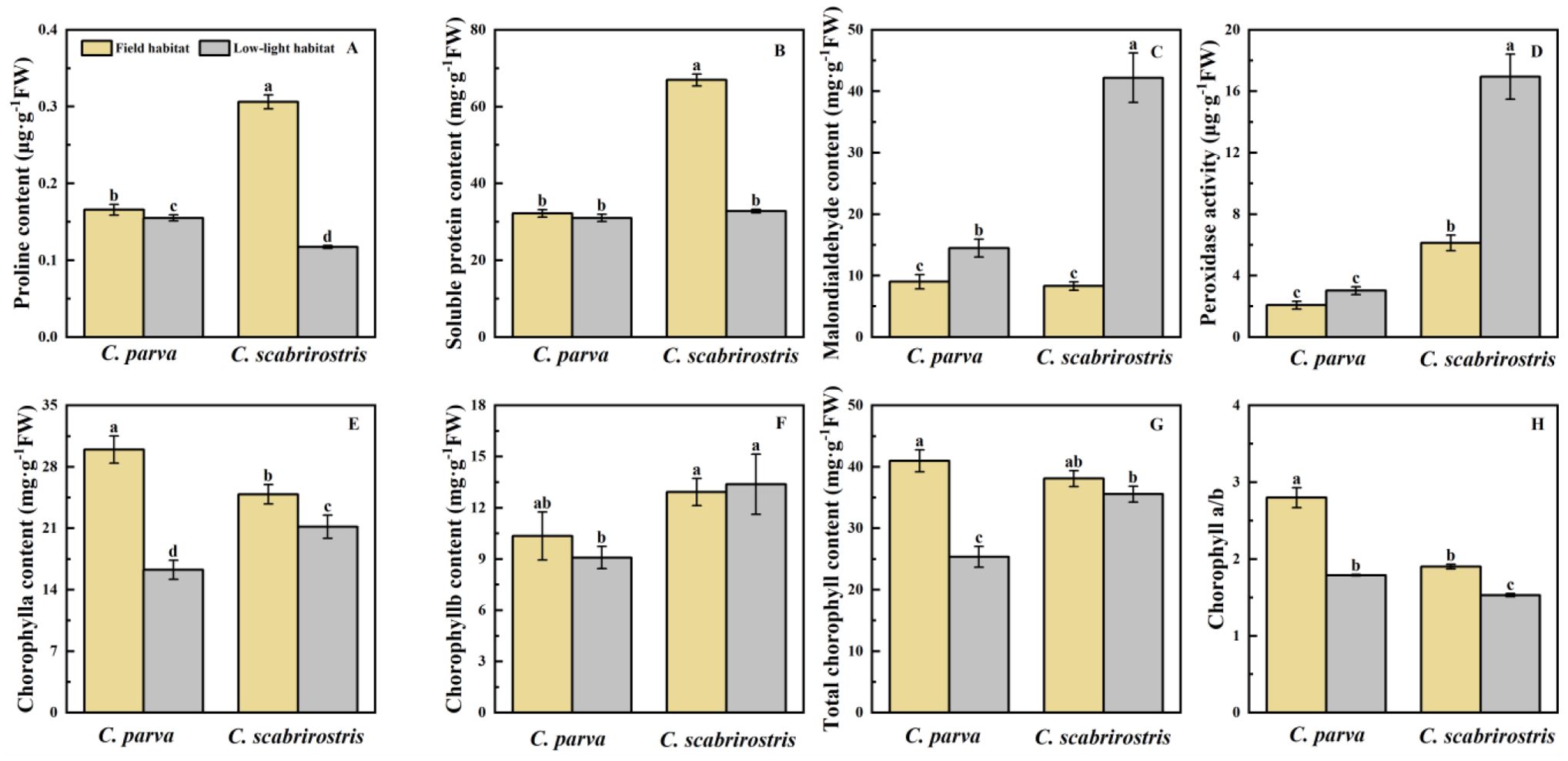
Figure 4. Effects of different environments on biochemical parameters of C. parva and C. scabrirostris leaves. Different letters indicate significant differences in means between treatments based on ANOVA. Bars represent Means ± SE (standard errors). (A) is the proline content, (B) is the soluble protein content, (C) is the malondialdehyde content, (D) is the peroxidase activity, (E) is the chorophylla content, (F) is the chorophyllb content, (G) is the total chorophyll content, (H) is the chorophya/b.
In terms of root morphology, the specific root length (SRL) of C. scabrirostris significantly increased under low-light conditions, while there was no significant change in C. parva (Figure 3G). However, both species of Carex showed a significant reduction in root tissue density (RTD) and branching intensity (BI) to varying degrees (Figures 2F, 3H). It is worth noting that C. scabrirostris was found to have dauciform roots with an average density of 50.5 (number·g-1DW) in the field habitat, whereas the original dauciform roots disappeared after a period of growth in a low-light environment (Table 2).
3.3 Changes of leaf biochemical parameters
The proline content in the leaves of both Carex species decreased significantly under low-light conditions (Figure 4A). SP levels significantly decreased in C. scabrirostris, whereas C. parva did not respond to SP (Figure 4B). Additionally, the MDA content in both Carex species significantly increased, with increases of 35.7% for C. parva and 80.9% for C. scabrirostris (Figure 4C). POD activity significantly increased by 62.5% in C. scabrirostris, (Supplementary Table), whereas no significant change was observed in C. parva (Figure 4D). Furthermore, under low-light conditions, both Carex species exhibited significant decreases in chlorophyll a and chlorophyll a/b (Figures 4E, H). Total chlorophyll content significantly decreased in C. parva, whereas no significant change was observed in C. scabrirostris (Figure 4G).
3.4 Plant plasticity, variability, and correlation between traits
Plants in field habitats exhibit high sensitivity (PI ≥ 0.6) in terms of LT, net photosynthetic rate (Pnmax), LET, POD, RD, and LCP response from the perspective of plant plasticity (Figures 5A, C). In terms of CUT, SRL, and chlorophyll a + b, they exhibit low sensitivity (PI < 0.2). In low-light environments, plants demonstrate high sensitivity (PI ≥ 0.6) in terms of LT, leaf dry matter content (LDWC), CUT, RD, Pnmax, leaf area (LA), LCP, and POD. However, they exhibit low sensitivity (PI < 0.2) in terms of RTD, SP, and chlorophyll a/b.
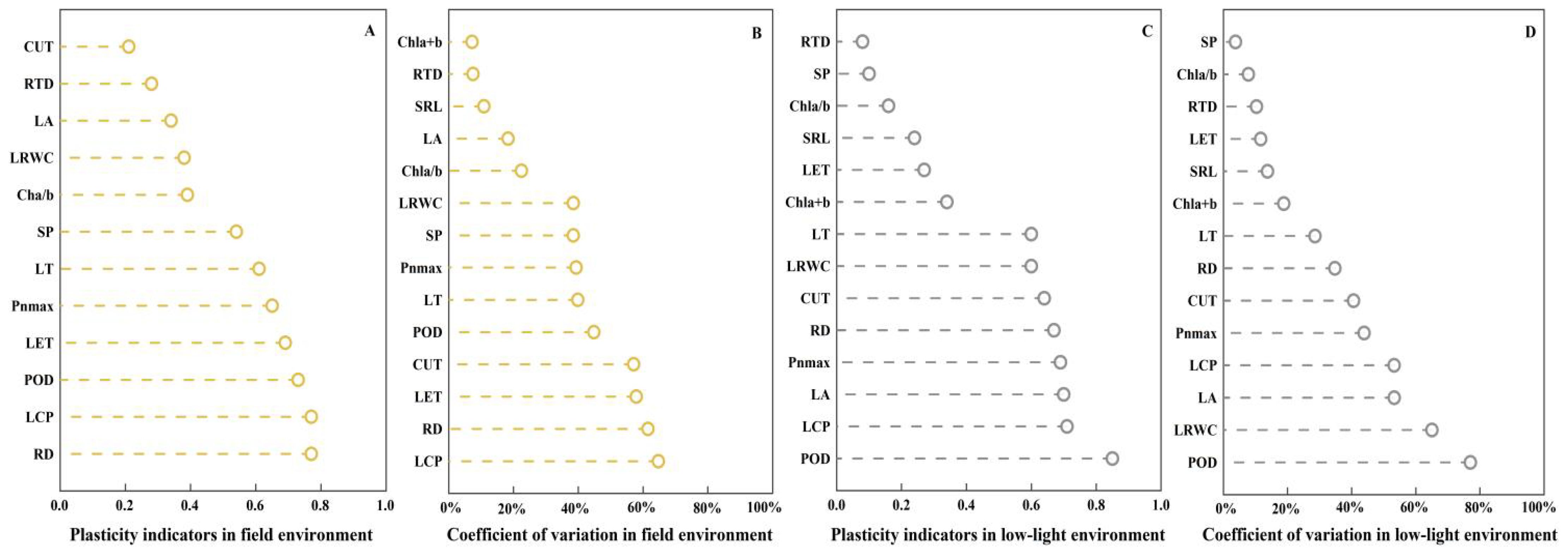
Figure 5. Plasticity index and coefficient of variation ranking of physiological and ecological indicators of C. parva and C. scabrirostris in different environments. LA, leaf area; LRWC, leaf relative water content; SRL, specific root length; RTD, root tissue density; CUT, cuticle thickness; LET, lower epidermal thickness; LT, leaf thickness; SP, soluble protein concentration; POD, peroxidase activity; Chla/b, chlorophyll a/b; Chla+b, total chlorophyll content; Pnmax, maximum net photosynthetic rate in the light curve; LCP, light compensation point; RD, rate of dark respiration. (A) is the plasticity indicators in field environment, (B) is the coeffcient of variation index in field environment, (C) is the plasticity indicators in low-light environment, (D) is the coeffcient of variation index in low-light environment.
From the perspective of plant variability (Figures 5B, D), plants in field habitats exhibit greater variability (CV > 50%) in terms of CUT, LET, RD, and LCP. In terms of LA, SRL, RTD, and chlorophyll a + b, they demonstrate smaller variability (CV <20%). However, in low-light environments, plants demonstrate greater variability (CV >50%) in terms of POD, LDWC, LA, and LCP. On the other hand, they show smaller variability (CV <20%) in terms of SP, chlorophyll a/b, SRL, LET, chlorophyll a + b, and RTD. The ranking of plant plasticity and variability remains consistent across different environments.
In terms of morphological indicators, morphologically, SLA shows a positive correlation with LRWC and a negative correlation with LET, irrespective of the environment (Figures 4D, 6C). Regarding intraspecific correlations of C. scabrirostris across different environments, dauciform root density (DRD) shows significant positive correlations with Pnmax and SP, and significant negative correlations with VCmax, Jmax, POD, and SLA (Figure 6B). In the natural habitat promoting dauciform root growth, the indicators that are significantly positively correlated with DRD remain consistent, while they show significant negative correlations with LCP, RD, chlorophyll a/b, CUT, and LET (Figure 6C). Regarding photosynthetic parameters, in natural habitats, Pnmax exhibits positive correlations with SRL, DRD, and SP, while significantly negatively correlated with LDMC and chlorophyll a/b (Figure 6C). In low-light environments, Pnmax shows a significant positive correlation with POD (Figure 6D). Regarding physiological characteristics, in natural habitats, POD exhibits significant positive correlations with SLA and LRWC, while significantly negatively correlated with LDMC and chlorophyll a/b. In low-light environments, POD shows a negative correlation with chlorophyll a/b.
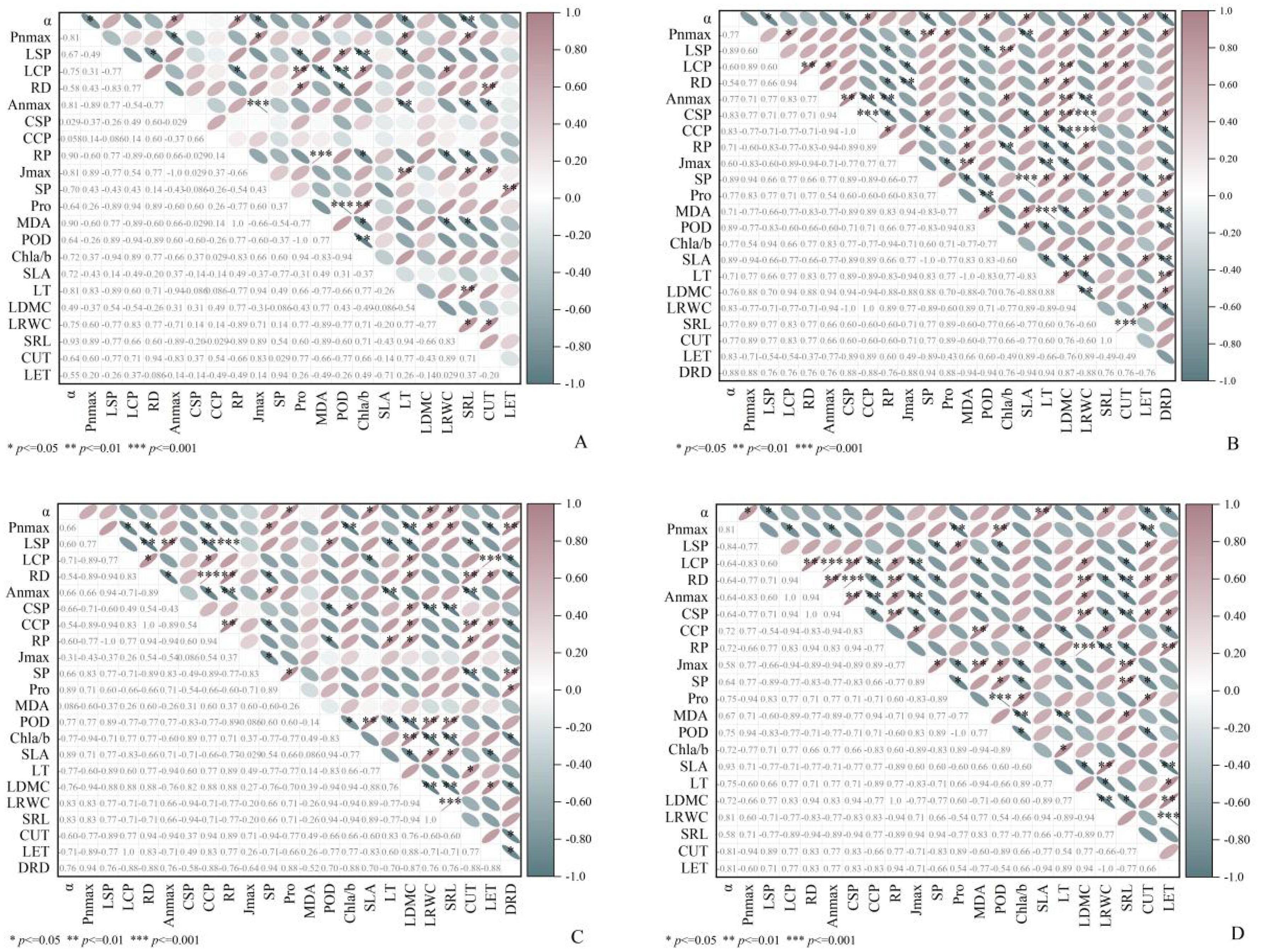
Figure 6. Spearman correlation analysis showed the relationship between functional traits of C. parva and C. scabrirostris. (A) is the relationship between the functional traits of C. parva; (B) is the relationship between the functional traits of C. scabrirostris; (C) is the relationship between the functional traits of two Carex species in the field environment; (D) is the relationship between the functional traits of two Carex species in the low-light environment. In the area in the lower left corner, the number represents the correlation coefficient. In the upper right corner, blue indicates a negative correlation, red indicates a positive correlation, and * indicates a significant correlation. α, apparent quantum efficiency; Pnmax, maximum net photosynthetic rate in the light curve; LSP, light saturation point; LCP, light compensation point; RD, rate of dark respiration; Anmax, maximum net photosynthetic rate (CO2—response curves); CSP, CO2 saturation point; CCP, CO2 compensation point; RP, photorespiration rate; Jmax maximum electron conductivity; SP, soluble protein; Pro, proline content; MDA, malondialdehyde content; POD, peroxidase activity; Chla/b, Chlorophyll a/b; SLA, specific leaf area; LT, leaf thickness; LDWC, leaf dry weight/fresh weight; LRWC leaf relative water content; SRL, specific root length; CUT, cuticle thickness; LET, lower epidermal thickness.
3.5 Redundancy analysis
The explanatory variables of the first and second axes were 39.83% and 34.13%, respectively, indicating that the first and second axes accounted for 73.96% of the variation in the photosynthetic characteristics of the two Carex species (Figure 7). Among the explanatory variables, POD had the longest arrow and the largest projected area, explaining 37.8% of the variation with a significant p-value of 0.002. This indicates a strong correlation with photosynthetic characteristics, significantly affecting them (p < 0.05). Additionally, POD had the highest contribution value of 39.2% and showed a positive correlation (acute angle) with α and Pnmax, while exhibiting a negative correlation (obtuse angle) with LCP and RD. DRD explained 31.10% of the variation with a p-value of 0.010, significantly affecting photosynthetic characteristics, positively correlating with Pnmax, Anmax, and LSP, and negatively correlating with RP. SLA accounted for 15.5% of the variation with a p-value of 0.004, significantly affecting photosynthetic characteristics and positively correlating with Pnmax and Jmax. Among these variables, SLA and α showed the smallest angle. LRWC explained 3.80% of the variation with a p-value of 0.042, significantly affecting photosynthetic characteristics, positively correlating with α and Vcmax, and negatively correlating with CSP. The results of RDA were consistent with the correlation values obtained from Pearson analysis.
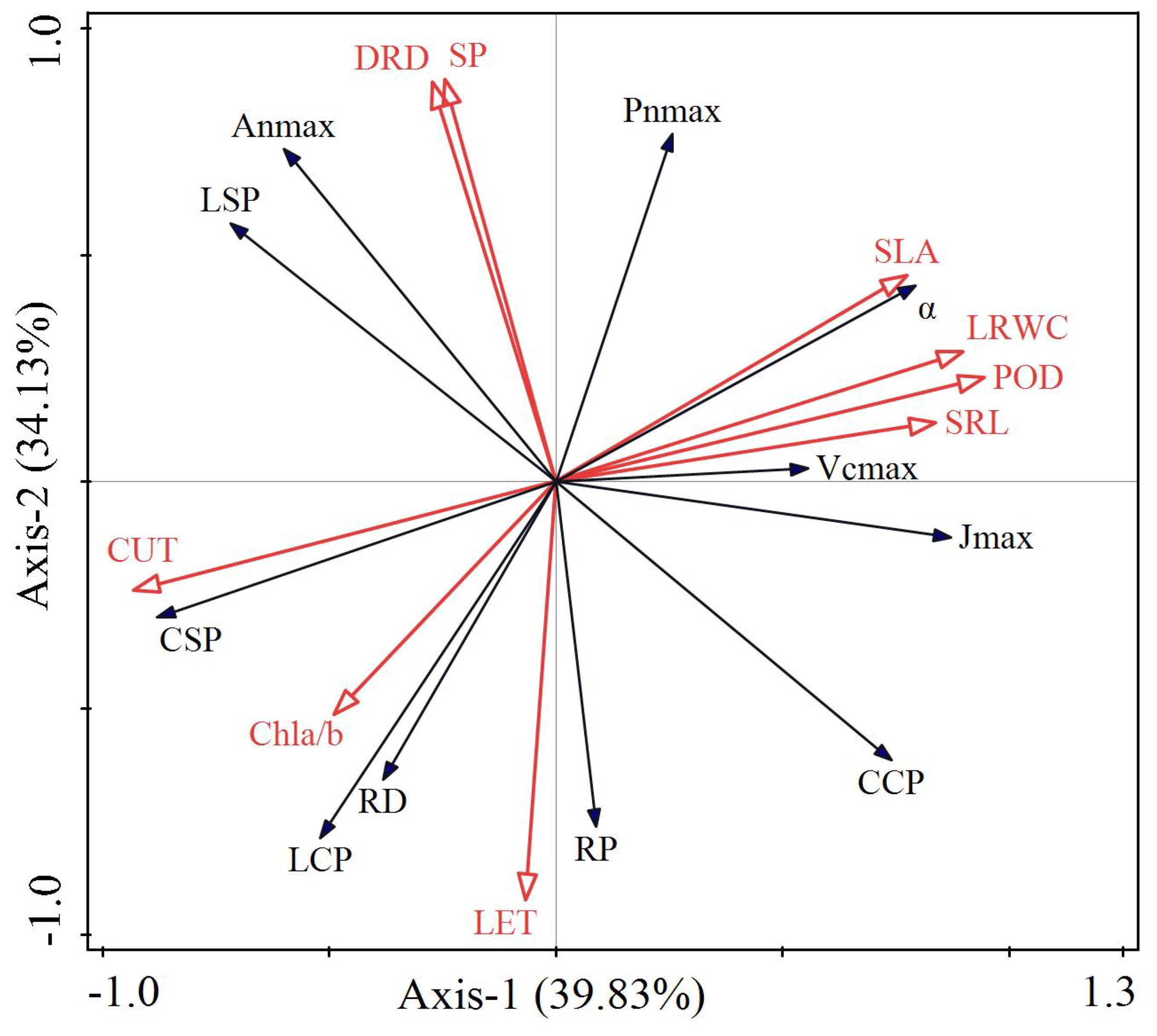
Figure 7. RDA analysis between photosynthetic and ecophysiological properties of two Carex species. α, apparent quantum efficiency; Pnmax, maximum net photosynthetic rate in light curve; LSP, light saturation point; LCP, light compensation point; RD, dark respiration rate; Anmax, maximum net photosynthetic rate in CO2 response curve; CSP, CO2 saturation point; CCP, CO2 compensation point; RP, photorespiration rate; VCmax, maximum carboxylation rate; Jmax, maximum electronic conductivity; POD, peroxidase activity; DRD, dauciform root density; SLA, specific leaf area; LRWC, leaf relative water content; Chla/b, chlorophylla/b; SP, soluble protein; LET, lower epidermal thickness; CUT, cuticle thickness; SRL, specific root length.
4 Discussion
4.1 Key factors affecting the photosynthetic capacity of two Carex species
In this experiment, immediately after sampling the field habitat plants, we simulated the native environments of C. parva and C. scabrirostris in an artificial climate chamber in order to obtain plants acclimated in low-light conditions. By controlling environmental factors, we minimized phenological differences among the two groups (Rosbakh et al., 2021) and reduced growth differences to the lowest possible level. We used the same soil substrate as in their field habitats, maintained humidity and soil moisture according to their native conditions, and strictly regulated the diurnal temperature variations in the climate chamber. This approach ensured that light intensity was the major variable, allowing us to investigate the acclimation changes and key factors influencing the photosynthetic capacity of the two Carex species under low-light conditions.
This study found that the POD activity, SLA, DRD, and LRWC of the two Carex species contributed 88.2% to their photosynthetic capacity (Table 3) and were significantly correlated with photosynthesisrelated indicators (p < 0.05). Among these, POD activity made the largest contribution to the photosynthesis-related indicators of the two Carex species. Under low-light stress conditions, the two Carex species produce reactive oxygen species that damage chloroplasts, resulting in decreased photosynthetic capacity. In order to maintain the redox balance and preserve the photosynthetic function of chloroplasts, both Carex species exhibited high POD activity to eliminate reactive oxygen species, stabilizing cell membranes and the photosystem (Liang et al., 2009; Zhang et al., 2022). Further analysis reveals that the plasticity and variability of POD activity showed strong regulatory potential under different environmental conditions, reinforcing the role of POD as a key factor in the response of plants to environmental variation. Therefore, they are key indicators influencing the habitat adaptability of Carex species. This also confirms that POD will change accordingly with environmental changes to help plants cope with adverse environments. Shading increased the POD activity of Cedrela fissilis, consistent with the findings of this study (Barbosa et al., 2022).
SLA and LRWC are essential indicators of leaf structure and morphology in plants. SLA is closely related to plant growth and survival strategies, associated with light capture and photosynthetic capacity. A larger leaf area facilitates capturing more light energy and enables faster plant growth potential. Plants with effective photosynthesis maintain high LRWC, preserving chloroplast structure and function. Both Carex species exhibited low SLA in fully illuminated environments, indicating that excessive investment in leaf morphology for efficient light absorption was unnecessary. In low-light conditions, C. scabrirostris actively increases its leaf area ratio to maximize light capture, maintaining relatively high LRWC, effectively preserving chloroplast structure and photosystem II function, enabling efficient photosynthesis. In this environment, C. parva showed a non-significant increase in SLA and a decrease in LRWC. This may be due to C. parva’s weaker ability to adapt to low light or the different ways in which the leaf morphology of the two Carex species adapts to light intensity. Meanwhile, both SLA and LRWC showed strong plasticity and variability under low light, serving as sensitive indicators of habitat response. This is also supporting evidence that the two species of Carex adapted to different light environments by regulating these indicators. In summary, plants adapt to different light environments by adjusting the morphological structure and physiological changes in their leaves.
As not all Carex species produce dauciform roots, this study finds that POD activity and LRWC as the most crucial physiological indicators affecting the photosynthetic capacity of the two Carex species during the transition from full light to low-light environments. Additionally, SLA is considered one of the most important indicators from a morphological perspective affecting the photosynthetic characteristics of these two Carex species. Valladares et al. (2006) considers the PI and CV to be relatively simple and effective methods, noting a strong correlation between each method. In this study, the rankings of the PI and CV are generally consistent, reflecting the sensitivity of each index to habitat responses. In summary, POD significantly influences the photosynthetic characteristics of both Carex. Under low-light conditions, the POD, SLA, and LRWC of C. scabrirostris are all significantly greater than those of C. parva, indicating that C. scabrirostris possesses higher photosynthetic efficiency and greater light energy utilization.
4.2 Responses of two Carex species to low-light environment
In this experiment, both C. parva and C. scabrirostris showed increased stomatal conductance, transpiration rate, and aboveground dry matter content under low-light conditions (PAR = 150). These results suggest that in such an environment, the water vapor exchange between the leaves of both Carex species and the external environment is promoted, thereby enabling the accumulation of photosynthetic products. C. parva reduces its LCP under low-light conditions but accumulates organic matter by increasing its LSP. Under low-light conditions, both the Pnmax and LSP of C. scabrirostris significantly decreased, indicating a reduction in its photosynthetic capacity. This reduction, in turn, enhances its adaptability to low-light environments (Fu et al., 2017). The Pnmax of both Carex species under low-light conditions is lower than in the field environment, possibly indicating acclimation to low light. Furthermore, regardless of the light environment, Pnmax of C. scabrirostris is significantly higher than that of C. parva, demonstrating its superior photosynthetic ability. Further analysis of LUE indicates that C. scabrirostris exhibits the highest LUE under low-light conditions, suggesting strong adaptability and survival capabilities in such environments. Both Vcmax and Jmax limit plant photosynthesis, and under low-light conditions compared to their natural environments, both Carex species exhibited varying degrees of reduction in Vcmax and Jmax. This could be one of the reasons for the weakened photosynthetic capacity, consistent with the findings of this study (Choi et al., 2021).
Plants have been shown to adapt to low-light conditions by increasing their chlorophyll content and reducing the a/b ratio under shaded conditions (Yao et al., 2016; Hirano et al., 2019). In low-light environments, the chlorophyll a/b ratio significantly decreases in both Carex species. C. scabrirostris displayed no significant alterations in total chlorophyll content, while C. parva significantly decreased. This also confirms that C. scabrirostris is more shade tolerant. Additionally, the SP content of both Carex species is positively correlated with Pnmax and Anmax. Pnmax and Anmax decrease significantly under low-light conditions. This indicates that photosynthesis in both Carex species is hindered compared to their native habitat in such low-light conditions, potentially reducing the photosynthetic yield of plants, thus limiting their ability to produce more proteins (Miao et al., 2023). This finding is important for understanding the growth strategies and physiological mechanisms of Carex species in low-light environments. C. scabrirostris exhibits the highest levels of SP and Pnmax in its habitat, providing nutrients for plant growth and aiding in better acclimation to the environment (Wang et al., 2021). Additionally, the excessive accumulation of lipid peroxidation products, measured as MDA, in both Carex species under low-light environments can cause damage to chloroplasts through the generation of reactive oxygen species, leading to a decline in plant photosynthetic capacity. To maintain internal redox homeostasis and sustain chloroplast function, both Carex species demonstrate higher POD activity to scavenge reactive oxygen species and maintain intracellular redox balance, thus keeping the cell membrane and the photosystem stable (Smirnoff and Arnaud, 2019).
Plants can maximize their photosynthetic efficiency and capacity by adjusting leaf area, and the increase in leaf area determines the light interception capacity (Weraduwage et al., 2015; Yao et al., 2016). Under full sunlight, two species of Carex actively regulate leaf area and aboveground biomass, exhibiting the lowest SLA and aboveground biomass. This acclimation avoids excessive light absorption and inhibition (Yao et al., 2016). In low-light environments, C. parva increases its individual leaf area to obtain more light energy. On the contrary, leaf thickness is significantly negatively correlated with individual leaf area. These morphological changes can optimize the leaf’s ability to capture light and alleviate potential light inhibition effects in C. parva. Leaf thickness and SLA of C. scabrirostris show a similar trend to C. parva. However, the individual leaf area of C. scabrirostris decreases significantly, although this decrease may be indicative of the plant’s capability to transport resources from aboveground to underground. This may explain why C. scabrirostris has decreased underground biomass while increasing aboveground biomass. The biomass of aboveground organisms is inversely proportional to leaf area, further indicating that the allocation of aboveground and belowground biomass is influenced by leaf area. Upper epidermal cell thickness in two Carex species is found to display a significant negative correlation with SLA while demonstrating a noteworthy positive correlation with the chlorophyll a/b ratio. Thinning of the epidermis thickness of two Carex species increases the light-exposed surface area, enhancing the light capture capacity of the leaves. It facilitates light penetration through the leaf surface, promotes photochemical reactions within leaf cells, and ultimately improves photosynthetic efficiency (Rôças et al., 2001).
In low-light environments, the RTD and BI of both C. parva and C. scabrirostris are significantly reduced, which decreases their competitiveness in the underground (Cheng et al., 2009). The SRL of C. scabrirostris significantly increases in low-light environments, indicating an active enhancement of root absorption for water and nutrients, thereby improving its adaptability to low-light conditions (Bordron et al., 2021). Consequently, C. scabrirostris exhibits a high level of competitiveness in terms of nutrient and water resources, promoting rapid growth even in low-light conditions (Birouste et al., 2014).
4.3 Growth strategies of two Carex species in two different environments
In addressing the third research question, it is possible to answer from both the perspective of trait variation, plasticity, and the leaf economic spectrum. Analyzing CV and PI is necessary to accurately reflect a species’ response to environmental changes and resource competition during community assembly (Donovan et al., 2011; Navarro and Hidalgo-Triana, 2021). Compared with roots, leaves exhibit greater plasticity and variability (Figure 4). This suggests that the root systems of the two Carex species remain relatively stable in heterogeneous environments. Meanwhile, plants trade-off various traits and make corresponding changes with environmental variations (Römermann et al., 2016). The different sensitivities of morphology, leaf anatomical structures, and photosynthetic parameters in this study to environmental changes indicate that plants also trade-off among different morphologies, leaf anatomical structures, and photosynthetic capacities to achieve optimal survival strategies, which benefit individual survival and population development. On the other hand, plants face the combined influences of various habitat factors, and a positive response of a certain trait to one environmental factor may be a negative response to another environmental factor (Langley et al., 2022). Plants also balance their responses to different habitat factors. In low-light environments, light is the main environmental factor limiting the growth of both Carex species. After weighing the pros and cons, both Carex species prioritize increasing leaf area and accumulating aboveground biomass to cope with the stressful conditions of low light. In field habitats, the CV values of LCP, LET, CUT, and RD are all above 50%, indicating that plants maintain internal leaf moisture through thicker cuticles and epidermal layers (Guo et al., 2023) and increase their photosynthetic capacity with higher LCP and faster RDs. Under low-light conditions, the CV values of LA, LRWC, and POD are all above 50%, which is related to the reduction in photosynthetic capacity. Plants strategically increase leaf area and utilize POD to eliminate reactive oxygen species, thereby optimizing light absorption and addressing limitations under low-light conditions.
Theoretical analysis of leaf economics spectra reveals that the investment strategies of both Carex species remain unchanged, whether in field habitats or low-light environments. C. parva, with weaker photosynthetic ability, a smaller SLA, and higher leaf dry matter accumulation, is categorized as a “slow-investment-high-return” species. In contrast, C. scabrirostris is a “fast-investment-low-return” species due to its larger SLA, higher photosynthetic capacity, and lower leaf dry matter accumulation (Stearns, 1998). C. scabrirostris employs a rapid investment strategy, enabling it to quickly occupy space and resources, though it may be less stable in long-term competition compared to species with slower investment strategies (Wright and Grier, 2012). Notably, C. scabrirostris shows significant variation in its investment strategy depending on the environment. In natural habitats, it allocates more resources underground, enhancing root expansion and nutrient absorption to address nutrient limitations. This strategy likely reduces leaf maintenance costs and increases resource-use efficiency, helping the species cope with the potential stress of high light intensity. Conversely, under low-light conditions, C. scabrirostris prioritizes aboveground growth, such as leaf development and optimization of photosynthetic structures, to maximize the capture of limited light resources. This flexible investment strategy underscores C. scabrirostris’s high adaptability to environmental changes and the diversity of its survival strategies. C. parva maintains consistent investment strategies in both environments, possibly due to its slower investment strategy or lack of response within a short time frame. Through in-depth analysis, this study further reveals that these species employ different response strategies in their aboveground parts when facing low-light conditions. C. parva enhances light capture efficiency by increasing leaf area, while C. scabrirostris reduces individual leaf area and leaf mass but significantly increases aboveground biomass, potentially due to an increased number of blades. This illustrates the resource allocation and compromise strategies employed by plant species in environments with limited resources, aligning with their trait adaptations and functional demands (Westoby and Wright, 2006; Heberling and Fridley, 2012). Under low-light conditions, C. scabrirostris allocates more resources to aboveground growth, altering its growth strategy, while C. parva remains unchanged in this experiment. These different responses reflect the long-term coexistence strategies of the two Carex species in relation to environmental conditions and resource competition.
From the perspective of the root economics spectrum, the two Carex species adopted a rapid investment strategy in a simulated urban low-light environment, whereas a conservative investment strategy was observed in their natural habitat (Martín-Robles et al., 2019). This finding is consistent with the conclusions drawn from the leaf economics spectrum. Notably, under simulated urban low-light conditions, C. scabrirostris was unable to produce dauciform roots. RDA and correlation analysis revealed a significant association between the presence of dauciform roots and the plant’s photosynthetic capacity. In low-light conditions, although the investment strategy for roots and leaves is rapid investment, C. scabrirostris prioritizes resources to the leaves to optimize light capture and improve photosynthetic efficiency. As a result, this leads to reduced investment in the belowground components, including the formation and maintenance of dauciform roots. Consequently, after growing in the same soil environment for a period of time, the dauciform roots of C. scabrirostris gradually disappeared.
5 Conclusion
This experiment significantly advanced our understanding of the response mechanisms of C. parva and C. scabrirostris to low-light conditions. First, indicators such as POD activity, SLA, and relative water content significantly influenced the photosynthetic capacity of the two Carex species. Secondly, under low-light conditions, C. parva exhibited a slow investment-return response strategy, while C. scabrirostris adopted a fast investment-return response strategy. Both Carex scabrirostris and Carex parva allocate more resources to aboveground growth in low-light environments to better adapt to the reduced light conditions. Third, in the simulated urban low-light environment, neither C. parva nor C. scabrirostris produced dauciform roots. The study found a strong correlation between dauciform root formation in Carex species and light intensity. Within the scope and conditions of these experiments, POD activity emerged as a key player in maintaining plant growth and photosynthetic capacity under low-light conditions. Delving deeper into the regulatory mechanisms of POD to light will provide valuable insights for optimizing plant light-use efficiency and enhancing adaptability to stressful environments.
Furthermore, both species showcased superior shade tolerance under simulated low-light urban environments, particularly C. scabrirostris. These findings hold promise for their potential as excellent turfgrass varieties for low-light environments in cities, laying a solid research foundation for their future cultivation and domestication. To safeguard species diversity in lawn grass and bolster the stability of urban lawns, this study advocates for further research focusing on the developmental potential of Carex species and their tolerance to high shade conditions.
Data availability statement
The raw data supporting the conclusions of this article will be made available by the authors, without undue reservation.
Author contributions
WL: Conceptualization, Data curation, Formal analysis, Investigation, Methodology, Writing – original draft, Writing – review & editing. RF: Investigation, Methodology, Writing – review & editing. SY: Formal analysis, Investigation, Writing – review & editing. SC: Software, Writing – review & editing. YH: Investigation, Writing – review & editing. WJ: Conceptualization, Funding acquisition, Investigation, Project administration, Resources, Writing – review & editing.
Funding
The author(s) declare financial support was received for the research, authorship, and/or publication of this article. This work was supported by the Natural Science Foundation of China (32071859), China’s Ministry of Science and Technology’s Basic Science Resources Survey Special Project (2019FY101604) and Shaanxi Academy of Forestry Technology Innovation Plan (SXLK2021-0203).
Acknowledgments
We would like to thank the reviewers for their valuable suggestions on our study.
Conflict of interest
The authors declare that the research was conducted in the absence of any commercial or financial relationships that could be construed as a potential conflict of interest.
Publisher’s note
All claims expressed in this article are solely those of the authors and do not necessarily represent those of their affiliated organizations, or those of the publisher, the editors and the reviewers. Any product that may be evaluated in this article, or claim that may be made by its manufacturer, is not guaranteed or endorsed by the publisher.
Supplementary material
The Supplementary Material for this article can be found online at: https://www.frontiersin.org/articles/10.3389/fpls.2024.1432539/full#supplementary-material
References
Barbosa, L. D. O., Dresch, D. M., Scalon, L., Scalon, S. D., Paula., Q. (2022). Ecophysiological Strategies of Cedrela fissilis Vell. Seedlings under Conditions of Flooding and Light Availability. J. Sustain. Forestry 41, 783–798. doi: 10.1080/10549811.2020.1867183
Birouste, M., Zamora-Ledezma, E., Bossard, C., Pérez-Ramos, I. M., Roumet, C. (2014). Measurement of fine root tissue density: a comparison of three methods reveals the potential of root dry matter content. Plant Soil 374, 299–313. doi: 10.1007/s11104-013-1874-y
Bordron, B., Germon, A., Laclau, J.-P., Oliveira, I. R., Robin, A., Jourdan, C., et al. (2021). Nutrient supply modulates species interactions belowground: dynamics and traits of fine roots in mixed plantations of Eucalyptus and Acacia mangium. Plant Soil 460, 559–577. doi: 10.1007/s11104-020-04755-2
Brundrett, M. C. (2009). Mycorrhizal associations and other means of nutrition of vascular plants: understanding the global diversity of host plants by resolving conflicting information and developing reliable means of diagnosis. Plant Soil 320, 37–77. doi: 10.1007/s11104-008-9877-9
Cao, X., Ma, Q., Zhong, C., Yang, X., Zhu, L., Zhang, J., et al. (2016). Elevational variation in soil amino acid and inorganic nitrogen concentrations in Taibai Mountain, China. PLoS One 11, e0157979. doi: 10.1371/journal.pone.0157979
Cheng, X., Huang, M., Shao, M., Warrington, D. N. (2009). A comparison of fine root distribution and water consumption of mature Caragana korshinkii Kom grown in two soils in a semiarid region, China. Plant Soil 315, 149–161. doi: 10.1007/s11104-008-9739-5
Choi, D., Jang, W., Toda, H., Yoshikawa, M. (2021). Differences in characteristics of photosynthesis and nitrogen utilization in leaves of the black locust (Robinia pseudoacacia L.) according to leaf position. Forests 12, 348. doi: 10.3390/f12030348
Cornelissen, J. H. C., Lavorel, S., Garnier, E., Díaz, S., Buchmann, N., Gurvich, D. E., et al. (2003). A handbook of protocols for standardised and easy measurement of plant functional traits worldwide. Aust. J. Bot. 51, 335–380. doi: 10.1071/bt02124
Dai, L., Liang, S.-Y., Zhang, S., Tang, Y., Koyama, T., Tucker, G. C., et al. (2010). Flora of China (Acoraceae through Cyperaceae), Vol. 23. Available online at: http://www.iplant.cn/info/Cyperaceae?t=foc.
De Antonio, A. C., Scalon, M. C., Rossatto, D. R. (2023). Leaf size and thickness are related to frost damage in ground layer species of Neotropical savannas. Flora 299, 152208. doi: 10.1016/j.flora.2022.152208
Dong, P. B., Wang, L. Y., Wang, L. J., Jia, Y., Li, Z. H., Bai, G., et al. (2022). Distributional response of the rare and endangered tree species abies chensiensis to climate change in East Asia. Biology-Basel 11, 1659. doi: 10.3390/biology11111659
Donovan, L. A., Maherali, H., Caruso, C. M., Huber, H., De Kroon, H. (2011). The evolution of the worldwide leaf economics spectrum. Trends Ecol. Evol. 26, 88–95. doi: 10.1016/j.tree.2010.11.011
Fajardo, A., Siefert, A. (2016). Phenological variation of leaf functional traits within species. Oecologia 180, 951–959. doi: 10.1007/s00442-016-3545-1
Falcioni, R., Moriwaki, T., Bonato, C. M., De Souza, L. A., Nanni, M. R., Antunes, W. C. (2017). Distinct growth light and gibberellin regimes alter leaf anatomy and reveal their influence on leaf optical properties. Environ. Exp. Bot. 140, 86–95. doi: 10.1016/j.envexpbot.2017.06.001
Flexas, J., Zhang, Y., Gulías, J., Xiong, D., Carriquí, M., Baraza, E., et al. (2022). Leaf physiological traits of plants from the Qinghai-Tibet Plateau and other arid sites in China: Identifying susceptible species and well-adapted extremophiles. J. Plant Physiol. 272, 153689. doi: 10.1016/j.jplph.2022.153689
Francini, A., Toscano, S., Ferrante, A., Romano, D. (2023). Method for selecting ornamental species for different shading intensity in urban green spaces. Front. Plant Sci. 14. doi: 10.3389/fpls.2023.1271341
Fu, B., Wang, S., Liu, Y., Liu, J., Liang, W., Miao, M., et al. (2017). Hydrogeomorphic ecosystem responses to natural and anthropogenic changes in the loess plateau of China. Annu. Rev. Earth Planet. Sci. 45, 223–243. doi: 10.1146/annurev-earth-063016-020552
Fu, J., Luo, Y., Sun, P., Gao, J., Zhao, D., Yang, P., et al. (2020). Effects of shade stress on turfgrasses morphophysiology and rhizosphere soil bacterial communities. BMC Plant Biol. 20, 92. doi: 10.1186/s12870-020-2300-2
Funk, J. L., Cornwell, W. K. L. (2013). Leaf traits within communities: context may affect the mapping of traits to function. Ecology 94, 1893–1897. doi: 10.1890/12-1602.1
Gao, Q., Yang, Z. L. (2016). Diversity and distribution patterns of root-associated fungi on herbaceous plants in alpine meadows of southwestern China. Mycologia 108, 281–291. doi: 10.3852/14-324
Guo, Y., Qiu, Y., Hu, H., Wang, Y., Mi, Z., Zhang, S., et al. (2023). Petal morphology Is correlated with floral longevity in Paeonia suffruticosa. Agronomy 13, 1372. doi: 10.3390/agronomy13051372
Grinn-Gofroń, A., Bosiacka, B., Bednarz, A., Wolski, T. (2018). A comparative study of hourly and daily relationships between selected meteorological parameters and airborne fungal spore composition. Aerobiologia 34, 45–54. doi: 10.1007/s10453-017-9493-3
Güsewell, S., Schroth, M. H. (2017). How functional is a trait? Phosphorus mobilization through root exudates differs little between Carex species with and without specialized dauciform roots. New Phytol. 215, 1438–1450. doi: 10.1111/nph.14674
Haque, M. S., De Sousa, A., Soares, C., Kjaer, K. H., Fidalgo, F., Rosenqvist, E., et al. (2017). Temperature variation under continuous light restores tomato leaf photosynthesis and maintains the diurnal pattern in stomatal conductance. Front. Plant Sci. 8. doi: 10.3389/fpls.2017.01602
Heberling, J. M., Fridley, J. D. (2012). Biogeographic constraints on the world-wide leaf economics spectrum. Global Ecol. Biogeography 21, 1137–1146. doi: 10.1111/j.1466-8238.2012.00761.x
Hirano, I., Iida, H., Ito, Y., Park, H.-D., Takahashi, K. (2019). Effects of light conditions on growth and defense compound contents of Datura inoxia and D. stramonium. J. Plant Res. 132, 473–480. doi: 10.1007/s10265-019-01111-z
Hu, Y., Yang, L., Gao, C., Liao, D., Long, L., Qiu, J., et al. (2022). A comparative study on the leaf anatomical structure of camellia oleifera in a low-hot valley area in guizhou province, China. PLoS One 17, e262509. doi: 10.1371/journal.pone.0262509
Huang, Y., Zhai, Y., Huang, Y., Huang, Y., Liu, K., Zhang, J., et al. (2022). Effects of light intensity on physiological characteristics and expression of genes in coumarin biosynthetic pathway of angelica dahurica. IJMS 23, 15912. doi: 10.3390/ijms232415912
Jakovljević, K. M., Šinžar-Sekulić, J. B., Vukojičić, S. S., Kuzmanović, N. V., Lakušić, D. V. (2014). Leaf anatomy of Carex humilis does not correlate with orographic, geological and bioclimatic habitat conditions in C&SE Europe. Biologia 69, 332–340. doi: 10.2478/s11756-013-0312-1
Jiang, S., Tang, Y., Fan, R., Bai, S., Wang, X., Huang, Y., et al. (2023). Response of Carex breviculmis to phosphorus deficiency and drought stress. Front. Plant Sci. 14. doi: 10.3389/fpls.2023.1203924
Kittipornkul, P., Treesubsuntorn, C., Kobthong, S., Yingchutrakul, Y., Julpanwattana, P., Thiravetyan, P. (2023). The potential of proline as a key metabolite to design real-time plant water deficit and low-light stress detector in ornamental plants. Environ. Sci. Pollut. Res. 31, 36152–36162. doi: 10.1007/s11356-023-27990-3
Lafont Rapnouil, T., Gallant Canguilhem, M., Julien, F., Céréghino, R., Leroy, C. (2023). Light intensity mediates phenotypic plasticity and leaf trait regionalization in a tank bromeliad. Ann. Bot. 132, 443–454. doi: 10.1093/aob/mcad126
Langley, J. A., Grman, E., Wilcox, K. R., Avolio, M. L., Komatsu, K. J., Collins, S.L., et al. (2022). Do trade - offs govern plant species’ responses to different global change treatments? Ecology 103, e3626. doi: 10.1002/ecy.3626
Liang, W., Wang, M., Ai, X. (2009). The role of calcium in regulating photosynthesis and related physiological indexes of cucumber seedlings under low light intensity and suboptimal temperature stress. Scientia Hortic. 123, 34–38. doi: 10.1016/j.scienta.2009.07.015
Lichtenthaler, H. K., Wellburn, A. R. (1983). Determinations of total carotenoids and chlorophylls a and b of leaf extracts in different solvents. Available online at: https://portlandpress.com/biochemsoctrans/article-abstract/11/5/591/57549 (Accessed November 25, 2023).
Liu, X., Xu, Y., Liu, F., Pan, Y., Miao, L., Zhu, Q., et al. (2021). The feasibility of antioxidants avoiding oxidative damages from reactive oxygen species in cryopreservation. Front. Chem. 9. doi: 10.3389/fchem.2021.648684
Martín-Robles, N., Morente-López, J., Freschet, G. T., Poorter, H., Roumet, C., Milla, R. (2019). Root traits of herbaceous crops: Pre-adaptation to cultivation or evolution under domestication?. Funct. Ecol. 33, 273–285. doi: 10.1111/1365-2435.13231
Maza-Villalobos, S., García-Ramírez, P., Endress, B. A., Lopez-Toledo, L. (2022). Plant functional traits under cattle grazing and fallow age scenarios in a tropical dry forest of Northwestern Mexico. Basic Appl Ecol. 64, 30–44. doi: 10.1016/j.baae.2022.06.006
Miao, C., Yang, S., Xu, J., Wang, H., Zhang, Y., Cui, J., et al. (2023). Effects of light intensity on growth and quality of lettuce and spinach cultivars in a plant factory. Plants 12, 3337. doi: 10.3390/plants12183337
Milla, R., Matesanz, S. (2017). Growing larger with domestication: a matter of physiology, morphology or allocation? Plant Biol. 19, 475–483. doi: 10.1111/plb.12545
Nam, J. M., Kim, J. H., Kim, J. G. (2017). Effects of light intensity and plant density on growth and reproduction of the amphicarpic annual Persicaria thunbergii. Aquat. Bot. 142, 119–122. doi: 10.1016/j.aquabot.2017.03.004
Navarro, T., Hidalgo-Triana, N. (2021). Variations in leaf traits modulate plant vegetative and reproductive phenological sequencing across arid mediterranean shrublands. Front. Plant Sci. 12. doi: 10.3389/fpls.2021.708367
Playsted, C. W. S., Johnston, M. E., Ramage, C. M., Edwards, D. G., Cawthray, G. R., Lambers, H. (2006). Functional significance of dauciform roots: exudation of carboxylates and acid phosphatase under phosphorus deficiency in Caustis blakei (Cyperaceae). New Phytol. 170, 491–500. doi: 10.1111/j.1469-8137.2006.01697.x
Rôças, G., Scarano, F. R., Barros, C. F. (2001). Leaf anatomical variation in Alchornea triplinervia (Spreng) Müll. Arg. (Euphorbiaceae) under distinct light and soil water regimes. Botanical J. Linn. Soc. 136, 231–238. doi: 10.1111/j.1095-8339.2001.tb00568.x
Römermann, C., Bucher, S. F., Hahn, M., Bernhardt-Römermann, M. (2016). Plant functional traits – fixed facts or variable depending on the season? Folia Geobot. 51, 143–159. doi: 10.1007/s12224-016-9250-3
Rosbakh, S., Hartig, F., Sandanov, D. V., Bukharova, E. V., Miller, T. K. (2021). Siberian plants shift their phenology in response to climate change. Glob. Change Biol. 27, 4435–4448. doi: 10.1111/gcb.15744
Shane, M. W., Dixon, K. W., Lambers, H. (2005). The occurrence of dauciform roots amongst Western Australian reeds, rushes and sedges, and the impact of phosphorus supply on dauciform-root development in Schoenus unispiculatus (Cyperaceae). New Phytol. 165, 887–898. doi: 10.1111/j.1469-8137.2004.01283.x
Shokoya, G., Fontanier, C., Martin, D. L., Dunn, B. L. (2022). Evaluation of sedges and nimblewill as low-input, shaded lawns in Oklahoma, USA. HortTechnology 32, 567–577. doi: 10.21273/HORTTECH05107-22
Smirnoff, N., Arnaud, D. (2019). Hydrogen peroxide metabolism and functions in plants. New Phytol. 221, 1197–1214. doi: 10.1111/nph.15488
Stearns, S. C. (1998). The evolution of life histories (Oxford university press). Available online at: https://academic.oup.com/book/53266 (Accessed November 25, 2023).
Sun, Z., Geng, W., Ren, B., Zhao, B., Liu, P., Zhang, J. (2023). Responses of the photosynthetic characteristics of summer maize to shading stress. J. Agron. Crop Sci. 209, 330–344. doi: 10.1111/jac.12630
Thompson, G. L., Kao-Kniffin, J. (2019). Urban grassland management implications for soil C and N dynamics: A microbial perspective. Front. Ecol. Evol. 7. doi: 10.3389/fevo.2019.00315
Trammell, T. L. E., Pataki, D. E., Still, C. J., Ehleringer, J. R., Avolio, M. L., Bettez, N., et al. (2019). Climate and lawn management interact to control C4 plant distribution in residential lawns across seven US cities. Ecol. Appl. 29, e01884. doi: 10.1002/eap.1884
Valladares, F., Sanchez gomez, D., Zavala, M. A. (2006). Quantitative estimation of phenotypic plasticity: bridging the gap between the evolutionary concept and its ecological applications. J. Ecol. 94, 1103–1116. doi: 10.1111/j.1365-2745.2006.01176.x
Violle, C., Navas, M.-L., Vile, D., Kazakou, E., Fortunel, C., Hummel, I., et al. (2007). Let the concept of trait be functional! Oikos 116, 882–892. doi: 10.1111/j.2007.0030-1299.15559.x
Wang, X., Feng, Y., Feng, X., Liu, W., Huang, Y., Jiang, S., et al. (2023). Environmental adaptability of the genus Carex-A case study of Carex heterostachya and Carex breviculmis in northwest China. Plant Ecol. 224, 617–634. doi: 10.1007/s11258-023-01328-y
Wang, J., Shi, K., Lu, W., Lu, D. (2021). Effects of post-silking shading stress on enzymatic activities and phytohormone contents during grain development in spring maize. J. Plant Growth Regul. 40, 1060–1073. doi: 10.1007/s00344-020-10164-7
Weng, M., Cui, L., Liu, F., Zhang, M., Shan, L., Yang, S., et al. (2015). Effects of drought stress on antioxidant enzymes in seedlings of different wheat genotypes. Pakistan J. Bot. 47, 49–56.
Weraduwage, S. M., Chen, J., Anozie, F. C., Morales, A., Weise, S. E., Sharkey, T. D., et al. (2015). The relationship between leaf area growth and biomass accumulation in arabidopsis thaliana. Front. Plant Sci. 6. doi: 10.3389/fpls.2015.00167
Westoby, M., Wright, I. J. (2006). Land-plant ecology on the basis of functional traits. Trends Ecol. Evol. 21, 261–268. doi: 10.1016/j.tree.2006.02.004
Więcław, H. (2017). Within-species variation among populations of the Carex flava complex as a function of habitat conditions. Plant Ecol. Diversity 10, 443–451. doi: 10.1080/17550874.2018.1440442
Wright, J. P., Grier, A. S. (2012). Does the leaf economic spectrum hold within local species pools across varying environmental conditions? Funct. Ecol. 26, 1390–1398. doi: 10.1111/1365-2435.12001
Wright, I. J., Reich, P. B., Westoby, M., Ackerly, D. D., Baruch, Z., Bongers, F., et al. (2004). The worldwide leaf economics spectrum. Nature 428, 821–827. doi: 10.1038/nature02403
Wu, H. (2016). Effect of different light qualities on growth, pigment content, chlorophyll fluorescence, and antioxidant enzyme activity in the red alga pyropia haitanensis (Bangiales, rhodophyta). BioMed. Res. Int. 2016, 1–8. doi: 10.1155/2016/7383918
Wu, H., Tang, H., Liu, L., Shi, L., Zhang, W., Jiang, C. (2021). Local weak light induces the improvement of photosynthesis in adjacent illuminated leaves in maize seedlings. Physiologia Plantarum 171, 125–136. doi: 10.1111/ppl.13220
Yao, H., Zhang, Y., Yi, X., Zhang, X., Zhang, W. (2016). Cotton responds to different plant population densities by adjusting specific leaf area to optimize canopy photosynthetic use efficiency of light and nitrogen. Field Crops Res. 188, 10–16. doi: 10.1016/j.fcr.2016.01.012
Zhang, J., Ge, J., Dayananda, B., Li, J. (2022). Effect of light intensities on the photosynthesis, growth and physiological performances of two maple species. Front. Plant Sci. 13. doi: 10.3389/fpls.2022.999026
Zhang, J., Liu, Y., Zheng, T., Zhao, X., Liu, H., Zhang, Y. (2021). Nutrient and stoichiometric characteristics of aggregates in a sloping farmland area under different tillage practices. Sustainability 13, 890. doi: 10.3390/su13020890
Keywords: Carex, low-light environment, photosynthesis, ecological strategy, dauciform root
Citation: Liu W, Fan R, Yang S, Chen S, Huang Y and Ji W (2024) Carex parva and Carex scabrirostris adopt diverse response strategies to adapt to low-light conditions. Front. Plant Sci. 15:1432539. doi: 10.3389/fpls.2024.1432539
Received: 14 May 2024; Accepted: 16 September 2024;
Published: 14 October 2024.
Edited by:
Alexander G. Ivanov, Bulgarian Academy of Sciences, BulgariaReviewed by:
Georgios Liakopoulos, Agricultural University of Athens, GreeceCaner Ünlü, Istanbul Technical University, Türkiye
Copyright © 2024 Liu, Fan, Yang, Chen, Huang and Ji. This is an open-access article distributed under the terms of the Creative Commons Attribution License (CC BY). The use, distribution or reproduction in other forums is permitted, provided the original author(s) and the copyright owner(s) are credited and that the original publication in this journal is cited, in accordance with accepted academic practice. No use, distribution or reproduction is permitted which does not comply with these terms.
*Correspondence: Wenli Ji, aml3ZW5saUBud2FmdS5lZHUuY24=