- 1Department of Crop Sciences, Division of Crop Plant Genetics, Georg-August-University Göttingen, Göttingen, Germany
- 2Department of Crop Sciences, Division of Plant Breeding Methodology, Georg-August University Göttingen, Göttingen, Germany
Flowering is a critical life stage for plants, and the regulation of flowering is heavily influenced by environmental factors and is genetically very complex. In oilseed rape (Brassica napus L.), a major oil crop, yield is heavily dependent on successful flowering. Until now, the influences of day length and temperature on flowering time have mostly been studied in spring-type rape, although they also affect flowering in winter oilseed rape after vernalization, and changing climate conditions alter springtime temperatures. In this study, a doubled haploid population derived from a cross between a winter and a spring-type oilseed rape was examined for the effect of cool and warm temperatures (11°C and 22°C) in combination with long and short days (8/16-h light) on flowering time after vernalization. Quantitative trait locus (QTL) analysis revealed major QTLs for flowering time in two homologous regions on chromosomes C06 and A07, which were found to interact epistatically. It was found that temperature can either delay or promote flowering depending on day length and genotype, highlighting the complex interplay between these factors. Our study provides new insights into the genetic basis of flowering time regulation in B. napus, especially after vernalization, and highlights the importance of considering the interplay between temperature and day length in breeding programs for this crop, particularly in the context of climate change.
1 Introduction
Flowering time is regulated in a complex network with different pathways that interact with each other and are well studied in Arabidopsis thaliana (Blümel et al., 2015; Quiroz et al., 2021). The internal and external signals are thereby controlling the autonomous and gibberellin pathways, as well as the vernalization, temperature, and day length pathways. Most of the environmental cues are sensed in the leaves and lead to the expression of FLOWERING LOCUS T (FT) through signaling cascades. The FT protein travels to the apical meristem and initiates the generative phase (Jaeger et al., 2013). Oilseed rape (Brassica napus L.) is closely related to Arabidopsis. However, a genome triplication occurred in the evolution of the genus Brassica, and the hybridization of Brassica rapa and Brassica oleracea led to the allopolyploid species B. napus with the A and C subgenomes (Chalhoub et al., 2014). Therefore, genes occur with a high copy number variation, including flowering time genes, which makes flowering time regulation in the B. napus complex (Schiessl et al., 2017; Schiessl, 2020).
Vernalization is the initiation of flowering through a prolonged cold period. In crop plants, the requirement of a vernalization period to initiate flowering separates winter crops from spring crops; therefore, the vernalization pathway is well studied in B. napus (Ferreira et al., 1995; Raman et al., 2016; Richter and Möllers, 2018; Schiessl et al., 2019).
Studies in Arabidopsis have shown the complexity of the molecular mechanisms for the regulatory pathways of day length and temperature. The influence of day length is often regulated over the inner circadian clock with the central regulator CONSTANS, a direct FT activator (Takagi et al., 2023). They are known to interact with each other, as well as with the plant age and the gibberellin pathway, making this one of the most complex pathways for flowering (Song et al., 2013; Kim and Sung, 2014; Blümel et al., 2015). Even though this is the case, many studies on the influence of temperature on flowering have been done without considering other abiotic factors like day length (Schiessl, 2020; Jiang, 2023). In particular, the effect of temperature and day length after vernalization is not studied in B. napus. Like Arabidopsis (Amasino and Michaels, 2010), oilseed rape is a long-day plant, for which longer day length and higher temperature generally lead to earlier flowering (Major, 1980; King and Kondra, 1986; Mendham and Salisbury, 1995; Nanda et al., 1996; Robertson et al., 2002; Nelson et al., 2014). So far, quantitative trait locus (QTL) mapping studies identified chromosomes A02, A03, A10, C03, C04, C05, and C09 as carrying photoperiod-sensitive genes (Robert et al., 1998; Axelsson et al., 2001; Cai et al., 2008; Luo et al., 2014; Rahman et al., 2018). However, most of the molecular markers used at that time do not allow identification of their physical positions on current reference genomes (Chalhoub et al., 2014; Sun et al., 2017; Lee et al., 2020).
For the effect of temperature, only very few studies have been done, all of them on spring-type rapeseed. Abelenda et al. (2023) studied the difference in flowering time between 21°C and 28°C in different spring-type cultivars. Most cultivars delayed flowering time under the higher temperature, but one genotype accelerated flowering and showed different FT expression. Salisbury and Green (1991) reported interactions between temperature and day length on flowering time in spring genotypes of European, Canadian, and Australian origins. In a Canadian spring oilseed rape doubled haploid (DH) population, Rahman et al. (2018) detected QTLs for flowering time for different day lengths and temperature regimes. In B. rapa, Xiao et al. (2019) mapped flowering time QTLs for responses to ambient temperature and photoperiod on nearly all chromosomes.
In conclusion, all these studies have shown genotypic differences in response to day length and temperature regarding flowering time regulation. However, the majority of those studies in B. napus were done on spring types or the effect of different temperatures during or before vernalization in winter types. Even though rising temperatures during winter and early spring caused by climate change are evident in areas of winter oilseed rape cultivation, the reaction of flowering time in winter oilseed rape to temperature and its interaction with day length has been understudied. Therefore, the objectives of the present work were to test the impact of day length and temperature on flowering time in fully vernalized plants of the DH population DH4079 × Express617 and to assess the interaction between temperature and day length. To achieve these objectives, plants vernalized for 9 weeks were grown under four different controlled conditions with combinations of short and long days (8 and 16 h) and at two temperature regimes (11°C and 22°C) to determine days to flowering. A single-nucleotide polymorphism (SNP) marker-based linkage map was used to map QTLs and identify candidate genes.
2 Methods
2.1 Plant material
The inbred line 617 from the winter oilseed rape cultivar Express (Norddeutsche Pflanzenzucht Hans-Georg Lembke KG, Holtsee, Germany) and the doubled haploid line DH4079 (Ferrie, 2003) from the Swedish spring-type cultivar Topas were crossed to generate F1 seeds. A DH population consisting of 184 lines was developed from clonally propagated F1 plants as described by Valdés et al. (2018).
2.2 Day length and temperature experiment
The effect of day length and temperature on the flowering time of fully vernalized plants was determined in a split–split plot design with two-factor levels in temperature (11°C and 22°C) and two-factor levels in day length (8 and 16 h) with five replications. Seeds of 184 DH lines, the parental genotypes, and the F1 were sown in two 96 multi-pot trays (Quickpot 96, HerkuPlast Kubern GmbH, Ering, Germany) with a total size of 335 × 515 mm in four duplicates. Single pots had a size of 38 × 38 × 78 mm and were filled with soil (Fruhstorfer Erde type T25, HAWITA Gruppe GmbH, Vechta, Germany) and cultivated for 3 to 4 weeks in the greenhouse until the two- to three-leaf developmental stages (BBCH 12 to 13; Lancashire et al., 1991). Then, the multi-pot trays were transferred to a vernalization chamber adjusted to 4°C–5°C and 8 h cool white light (Schuch Typ 164/12 L96C 82W) for 9 weeks. After vernalization, the plants were transferred to two growth chambers with different temperatures, which were divided with sheets impervious to light to allow treatment with different day lengths. Therefore, the conditions consisted of 4 day length and temperature combinations of 8 h/11°C (SD11), 8 h/22°C (SD22), 16 h/11°C (LD11), and 16 h/22°C (LD22). For testing the effect of day lengths and temperatures, the positions of the genotypes on the multi-pot trays were randomized in each replication and condition. Growth chambers were equipped with Philips MASTER Green Power CG T 400 W, providing light intensities of 110–120 µmol·m−2·s−1. Plants were watered and fertilized on a regular basis and treated with fungicides and insecticides, when necessary. Days to flowering (DTF) was recorded starting from the day of transfer to the climate chamber. Replications were terminated at day 135. Genotypes that did not flower at day 135 but showed buds were recorded with a value of 150 DTF, and if they did not show buds, they were recorded with a value of 165 DTF. The means over all replications of each condition were used to calculate differences in days to flowering. Differences between DTF under short and long days at the same temperature (SD-LD11 and SD-LD22) and between low and high temperatures under the same day length (11-22LD and 11-22SD) were calculated. A full list of phenotypic data is available in Supplementary Table S1.
2.3 Statistical analysis
PLABSTAT 3A software (Utz, 2011) was used to calculate the analysis of variance and heritabilities. The ANOVA for day length and temperature experiment was performed using the model for a split–split plot design: , where Yijkl is the trait value of the genotype l in the day length condition k and the temperature condition j in replication i; μ is the general mean; tj and ri are the effects of temperature j and replication i, respectively; and ritj is the interaction between the ith replication and jth temperature, which is treated as the first stratum error. The effect of the kth day length is dk, and tjdk is the interaction between the jth temperature and kth day length, ritjdk is the second stratum error (interactions between the ith replication, jth temperature, and kth day length; gl is the effect of the lth genotype; gltj, gldk, and gltjdk are the interactions between the lth genotype with the jth temperature and kth day length, while gltjdkri is the third stratum error term. The factors genotypes and replications were taken as random. Broad-sense heritabilities were calculated using the formula , with coefficient T = 20 as the product of all factor levels.
Other statistical analyses were performed in R (R. Core Team, 2019). Figures of the descriptive statistics were created in R using the package ggplot2 (Wickham, 2016; R. Core Team, 2019). A Tukey’s test was used to test significant differences (p ≤ 0.01) between subgroups in figures with a box plot.
2.4 QTL analysis
A previously published full marker map consisting of 21,583 markers distributed over 19 linkage groups was used to develop a bin map of 1,883 markers (Valdés et al., 2018). Mean values over the five replications were used in QTL mapping for all traits. QTL mapping was performed using the WinQTL Cartographer software version 2.5 (Wang et al., 2012), and the composite interval mapping (CIM) algorithm was employed with the following specifications: independent logarithm of odds (LOD) significance thresholds (α = 0.05) were estimated for each trait by 1,000 permutation tests. Model 6 was employed; the forward and backward stepwise regression method was used to set cofactors. The genome was scanned at 1-cM intervals, and the window size was set to 10 cM. The 95% confidence interval for each QTL was determined by one LOD drop from the peak position. Additive effects, as well as the percentage of phenotypic variance explained by a QTL, were determined. A positive additive effect of a QTL is an additive effect by the allele of the winter oilseed rape parent Express617. To test epistasis, a multiple-interval mapping method was used. QTLs found in CIM were used as input and the BIC-M0 model with 1-cM walk speed and 10-cM window size. Additive × additive effects were significant with a LOD score of 2.4.
SNP marker sequences were provided by Isobel Parkin (AAFC, Saskatoon, SK, Canada), aligned using the BLAST algorithm against the reference genome sequence of “Express617” (Lee et al., 2020) by use of the Galaxy BLASTn-short algorithm (Cock et al., 2015), and used to create a physical map. Figures of the maps were drawn using MapChart (Voorrips, 2002). Gene annotation was provided by Schilbert et al. (2021).
3 Results
3.1 Quantifying the effect of day length and temperature on flowering time of fully vernalized plants
According to the analysis of variance, DTF is predominantly influenced by the effect of day length (Table 1). The size of the variance components for the effect of day length was almost 20 times that of the temperature and more than two times that of the genotype. The size of the variance components for the temperature × day length interaction was 1.5 times that of the effects of temperature. DTF showed a high broad-sense heritability of H2 = 95%. Short-day (SD) conditions (8-h light) delayed the mean DTF in the DH population, as well as for the parents and F1, but also increased the range (Table 2).
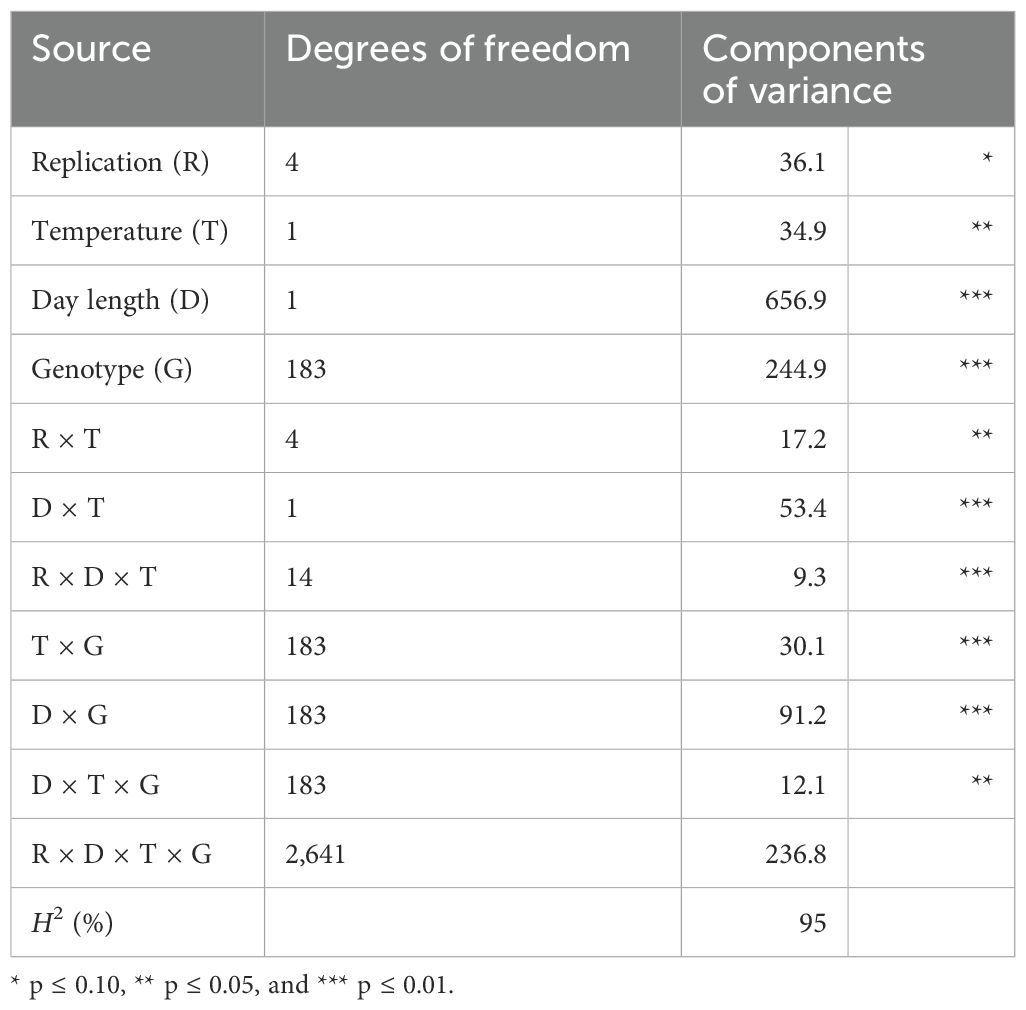
Table 1. Components of variance and broad-sense heritability (H2) for days to flowering for the DH population DH4079 × Express617 (n = 184) at two different temperatures T (11°C and 22°C) and two different day length conditions D (8 h and 16 h) after full vernalization treatment.
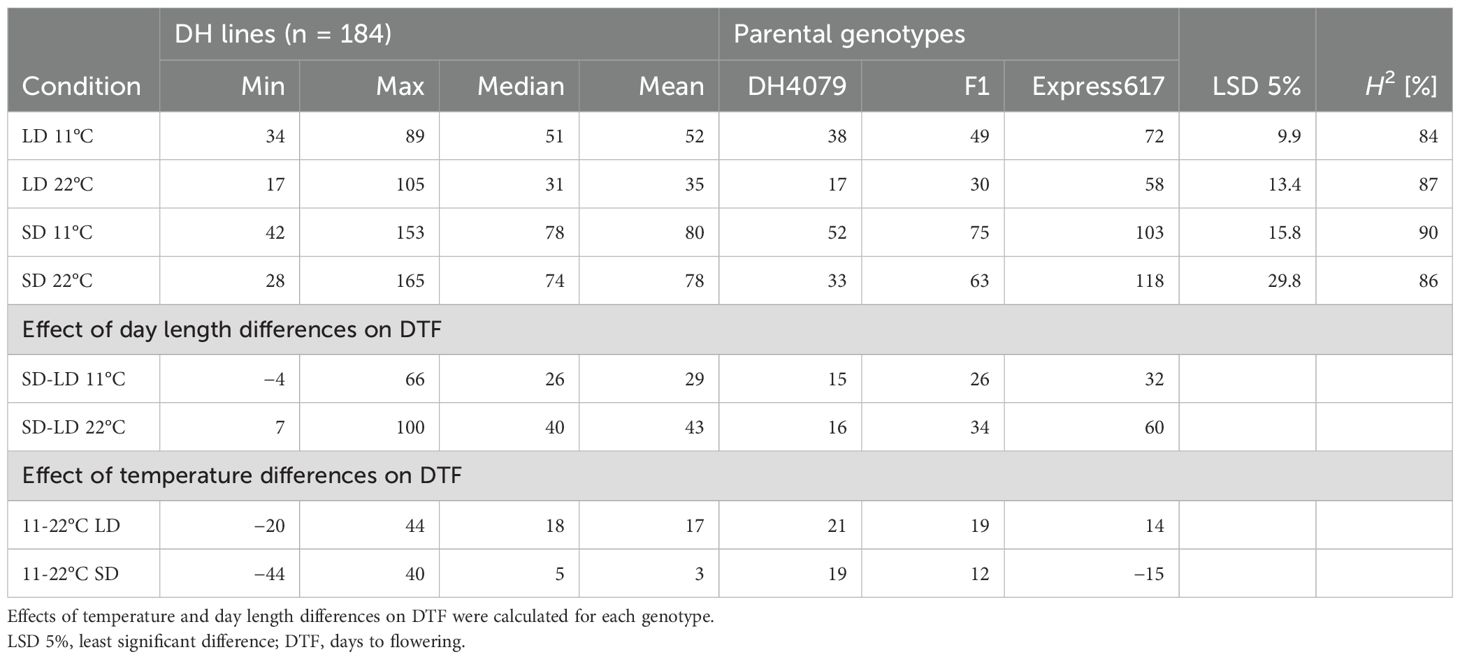
Table 2. Descriptive statistics for days to flowering of vernalized plants of the DH4079 × Express617 DH population grown under four different conditions, with different temperatures (11°C and 22°C) and different day lengths: short day (SD; 8 h) and long day (LD; 16 h).
The mean of the DH lines showed an earlier flowering time due to higher temperatures under long-day (LD) conditions (16-h light) from 52 days at 11°C to 35 days at 22°C. The range increased under 22°C and SD conditions (Table 2, Figure 1). Under SD conditions, the means for DTF under the two temperature regimes were no longer significantly different (Table 2, Figure 1). In all conditions, the winter oilseed rape parent Express617 flowered later than the spring-type parent DH4079, and the F1 showed an intermediate phenotype but had values slightly closer to those of the spring-type parent (Figure 1).
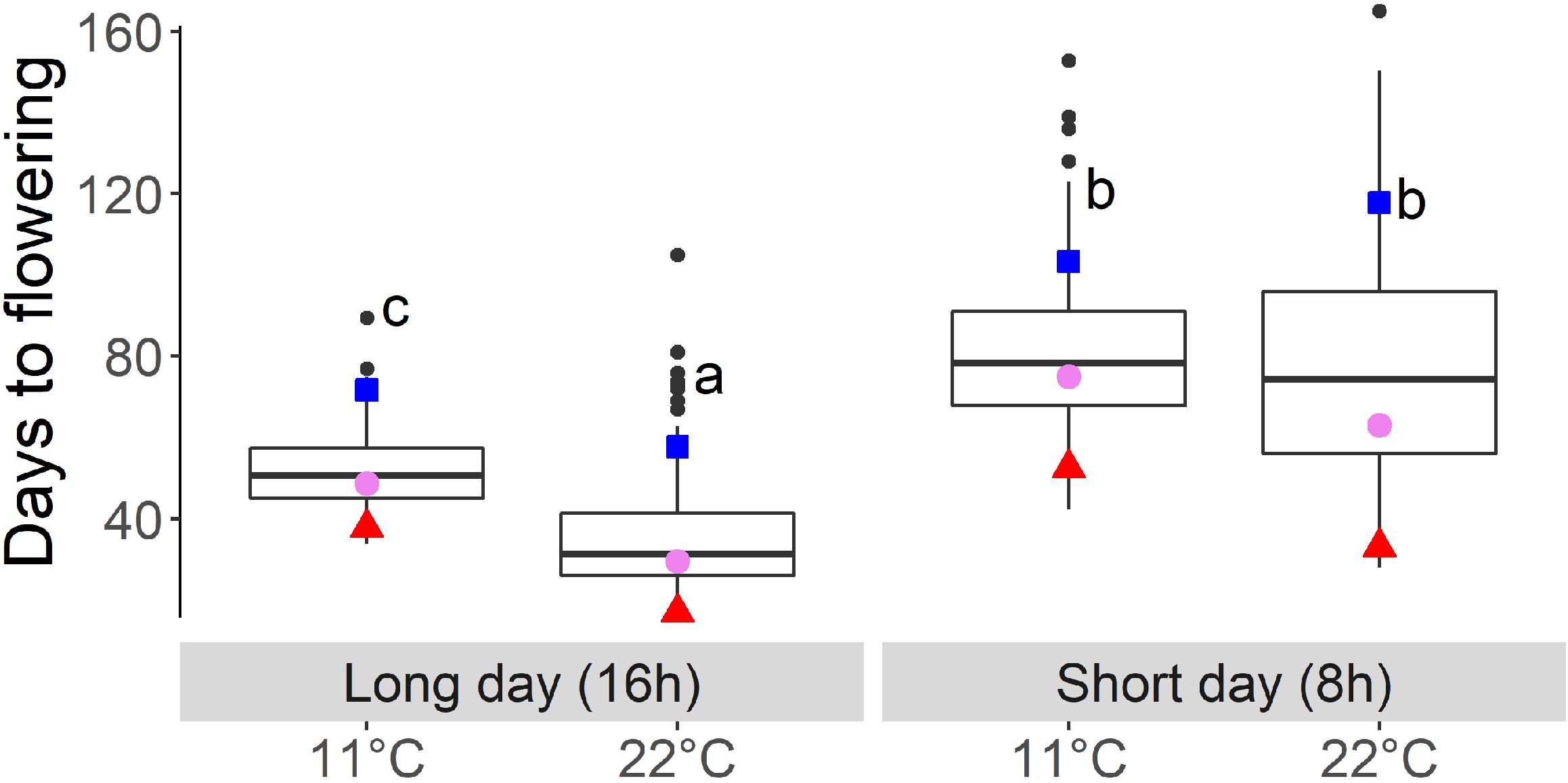
Figure 1. Days to flowering of the fully vernalized DH population growing under different temperatures (11°C and 22°C) and day length (short- and long-day) conditions. Letters indicate significantly different subgroups (p ≤ 0.01) tested with Tukey's test. Winter oilseed rape parent Express617 is indicated with blue square, spring-type parent DH4079 indicated with red triangle, and F1 indicated with violet circle.
The effect of day length differences on DTF in the DH population, calculated by subtracting DTF under LD from DTF under SD, had in a temperature of 22°C a population mean of SD-LD22 = 43 days and in 11°C only SD-LD11 = 29 days (Table 2). However, under both temperatures, the range was extensive from −4 up to 100 days of difference in flowering time. In the winter oilseed rape parent Express617, the effects of day length differences were SD-LD11 = 32 days and SD-LD22 = 60 days, while the effects in the spring-type parent DH4079 were lower and similar in both temperatures (SD-LD11 = 15 and SD-LD22 = 16 days).
The effect of temperature differences, calculated by subtracting DTF at 22°C from 11°C at the respective day lengths, showed under LD a population mean of 11-22LD = 17 days and under SD a mean of 11-22SD = 3 days differences in flowering (Table 2). The values for this effect of temperature differences on the DH lines ranged between −20 and 44 days under the long-day conditions (11-22LD) and between −44 and 40 days under the short-day conditions (11-22SD). This range showed the ability of warmer temperatures to either accelerate or delay DTF compared to cool temperatures, depending on the genotype and in interaction with the day length conditions. Under LD conditions, the effect of temperature differences on the spring-type parent DH4079 was 11-22LD = 21 days, with an acceleration of flowering through warmer temperatures. In the winter-type parent Express617, the value was lower with 11-22LD = 14 days, and the F1 showed an intermediate phenotype. Under SD, the warmer temperature led to a delayed flowering time in Express617 with 11-22SD = −15 days, while still accelerating in the F1 (11-22SD = 12 days) and DH4079 (11-22SD = 19 days, Table 2, Figure 1). For DH4079, the effects of temperature differences showed similar values with 11-22LD = 21 days and 11-22SD = 19 days as the effects of day length differences, indicating no interaction between temperature and day length in this parental genotype (Table 2).
3.2 Identification of major genomic regions with clusters of QTL
The QTL analysis (Table 3) revealed between four and seven QTLs for each trait, which when summed up could explain between TR2 = 38.6% and 65.2% of the phenotypic variance of the respective trait (Table 3). Most QTLs for days to flowering showed a positive effect, except for QTLs on A05 (LD22-3) and C06 (LD22-7, SD11-5, SD11-6, and SD22-5), indicating that the delay of flowering was caused mainly by Express617 alleles (Table 3). Certain regions of the genome showed an accumulation of QTLs. The major QTLs were forming clusters on chromosomes A07 and C06 (Figures 2, 3).
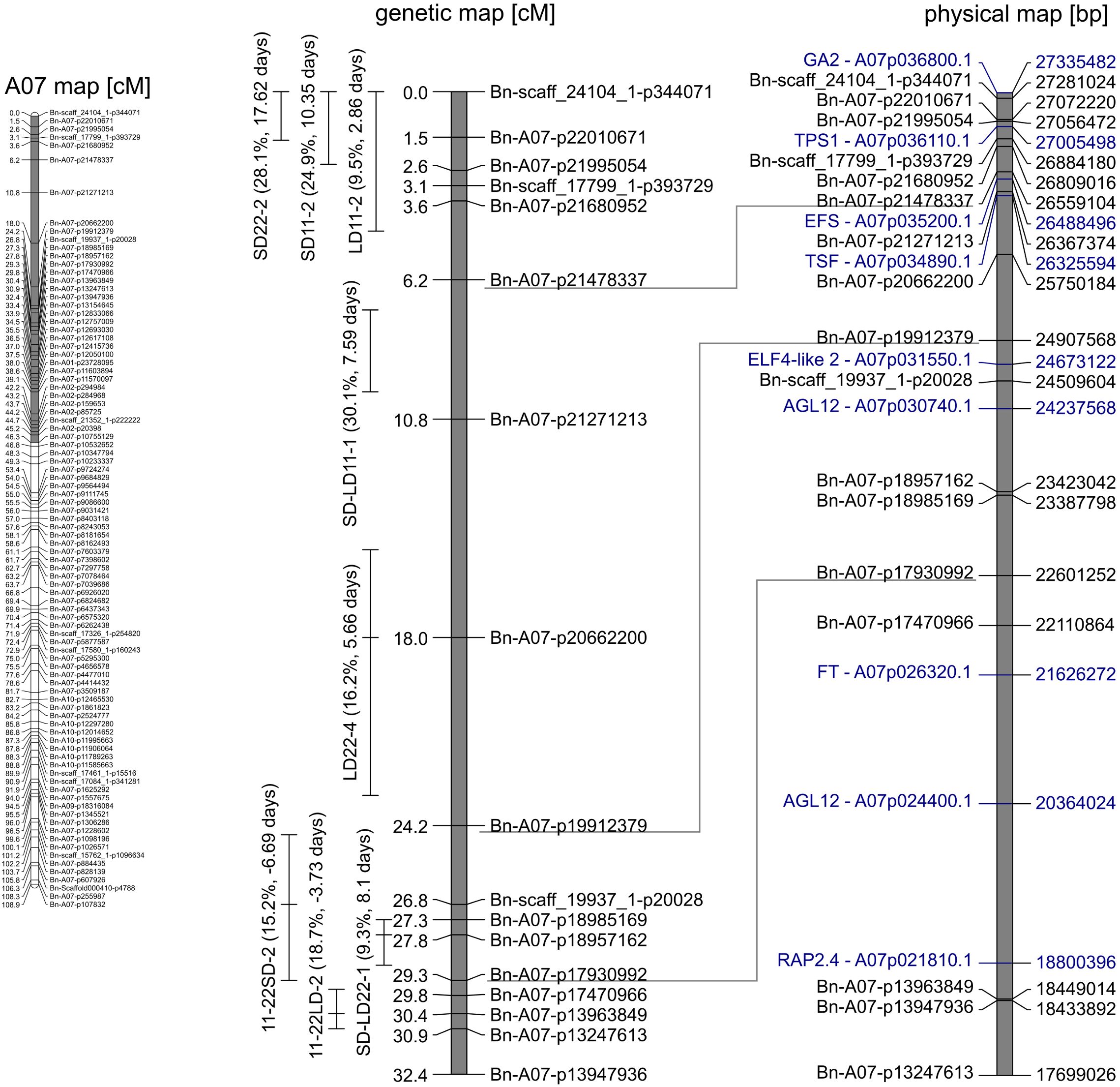
Figure 2. Gray marked in genetic map of A07 (left) is the section enhanced on the right. Genetic (middle) map depicts the quantitative trait loci (QTLs) clustering on A07. QTLs are given with peak and 95% confidence interval. The phenotypic variance explained (in percent) and additive effect (in days) of the QTL are shown in brackets. In the corresponding physical (left) map, candidate genes (blue) and the respective gene ID in the reference genome of “Express617” are shown.
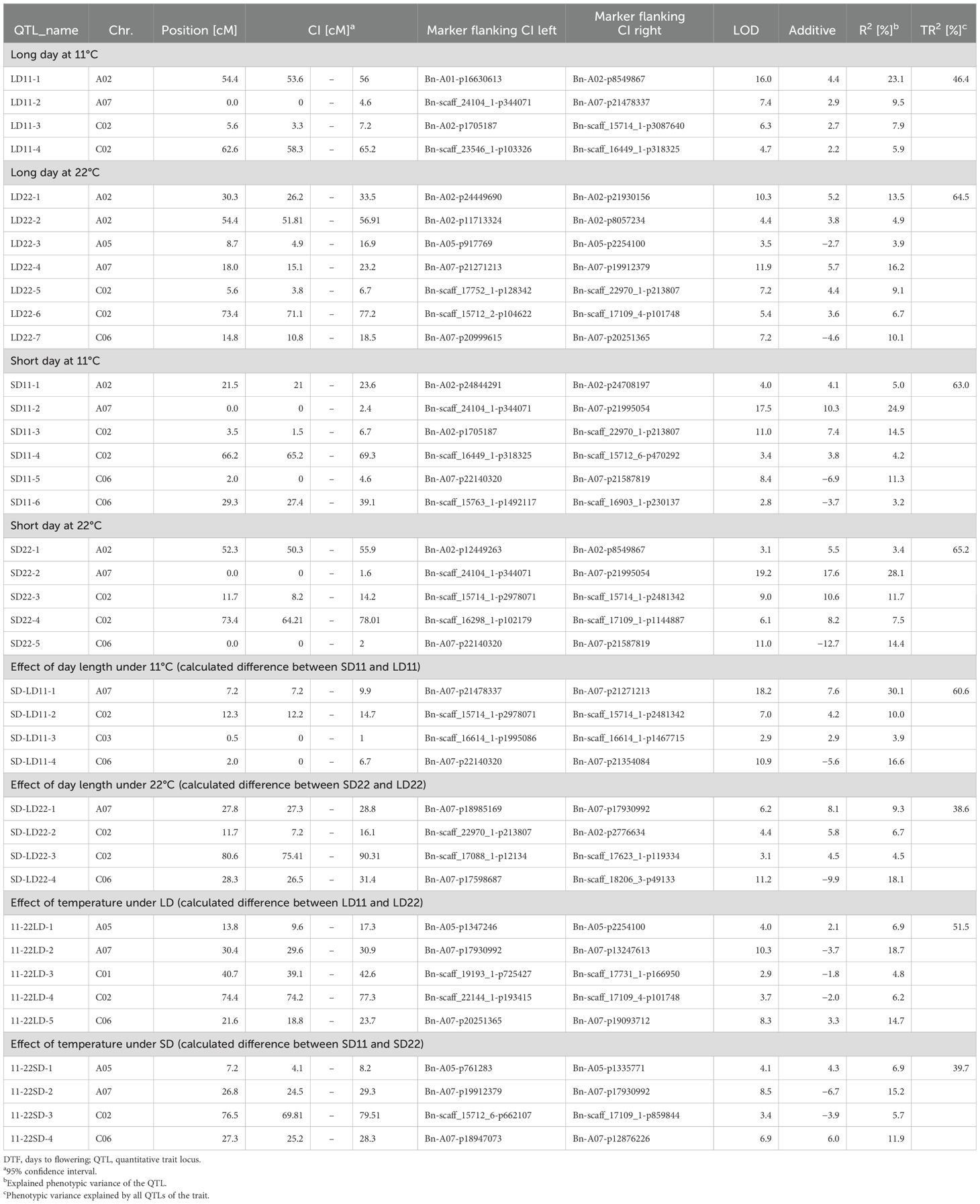
Table 3. Quantitative trait loci mapped for DTF under different temperature and day length conditions, the effects of temperature, and the effects of day length in the DH4079 × Express617 population.
3.2.1 QTLs on chromosome A07
On the linkage group A07, two QTL clusters were identified (Figure 2). At the beginning of the genetic map between 0 and 9.9 cM, four QTLs were located, three of which are related to flowering under short days (SD11-2, SD22-2, SD-LD11-1, and LD11-2). The major QTLs of both traits regarding DTF under SD conditions collocated here; QTL SD11-2 at 0 cM explained R2 = 24.9% of the variance with an additive effect of 10.3 days, and QTL SD22-2 at 0 cM explained R2 = 28.1% with an additive effect of a = 17.6 days. The second-largest QTL LD11-2 for the trait DTF under 11°C and LD was positioned at 0 cM with an explained variance of R2 = 9.5% and an additive effect of a = 2.9 days. Since the peaks for those three QTLs were located at 0 cM, the real QTLs may be located outside of the genetic map. The major QTL for the effect of day length differences at 11°C, SD-LD11-1, was located on A07 at 7.2 cM with an explained variance of R2 = 30.1% and an additive effect of a = 7.6 days. For all four QTLs, the Express617 allele delayed flowering. Between 24.5 and 30.9 cM on the same chromosome A07, three QTLs formed a second cluster; two major QTLs for the temperature differences were mapped here, even though the confidence intervals did not overlap (Figure 2), and one QTL for day length differences at 22°C was mapped. For temperature differences under LD, the largest QTL 11-22LD-2 at 30.4 cM explained a phenotypic variance of R2 = 18.7% and an additive effect of a = −3.7 days. For temperature differences under SD, the largest QTL 11-22SD-2 mapped at 26.8 cM and explained R2 = 15.2% of the phenotypic variance with an additive effect of a = −6.7 days. Their additive effects were negative, meaning that the Express617 allele made this effect smaller by either delaying DTF at 22°C or accelerating DTF under 11°C. The second-largest QTL for day length differences at 22°C (SD-LD22-1) was located on A07 at 27.8 cM, falling into the confidence interval of 11-22SD-2 (Figure 2), and explained R2 = 9.3% of the phenotypic variance (a = 8.1 days).
3.2.2 QTLs on chromosome C06
At the beginning of C06 from 0 to 6.7 cM, a QTL cluster for DTF in SD was located (Figure 3). The second-largest QTL for DTF in warm SD conditions, SD22-5, was located on C06 at 0 cM (R2 = 14.4%, a = −12.7 days). It had overlapping confidence intervals with the third largest QTL for DTF in cool SD conditions, SD11-5 (R2 = 11.3%, a = −6.9 days), and the second-largest QTL for the effect of day length difference under 11°C, SD-LD11-4 (R2 = 16.6%, a = −5.6 days), which were both collocated on C06 at 2 cM.
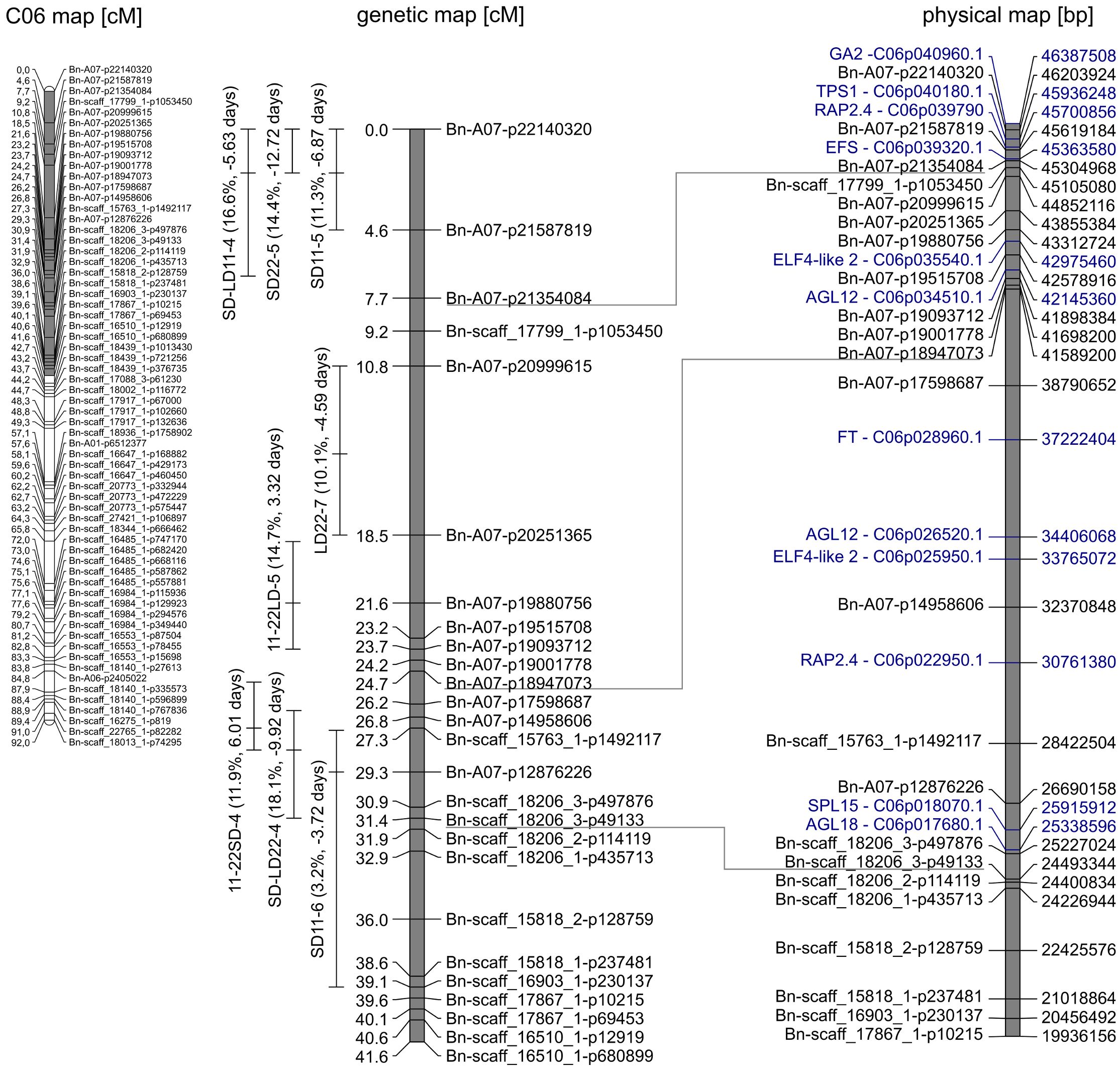
Figure 3. Gray marked in genetic map of C06 (left) is the section enhanced on the right. Genetic (middle) map depicts the quantitative trait loci (QTLs) clustering on C06. QTLs are given with peak and 95% confidence interval. The phenotypic variance explained (in percent) and additive effect (in days) of the QTL are shown in brackets. In the corresponding physical (right) map, candidate genes (blue) and the respective gene ID in the reference genome of “Express617” are shown.
On the same chromosome C06 between 18.8 and 39.1 cM, a QTL cluster with four QTLs regarding the reaction of DTF to day length and temperature was located. The effects of temperature difference under SD and LD were both mapped with their second-largest QTL in this cluster, although they showed no overlapping confidence intervals. QTL 11-22LD-5 on C06 at 21.6 cM explained R2 = 14.7% of the variance with a positive additive effect of a = 3.3 days. QTL 11-22SD-4 on C06 at 27.3 cM explained R2 = 11.9% of the variance with a positive additive effect of a = 6.0 days. The effect of day length differences at 22°C had its major QTL SD-LD22-4 on C06 at 28.3 cM with R2 = 18.1% explained variance and an additive effect of a = −9.9 days, meaning the DH4079 allele at this position delayed flowering under SD. The DTF under SD and 11°C mapped with the minor QTL SD11-6 at 29.3 cM and an explained variance of R2 = 3.2% and a = −3.7 days. The three QTLs 11-22SD-4, SD-LD22-4, and SD11-6 had overlapping confidence intervals. Interestingly, the Express617 allele accelerated flowering under short days and warm temperatures in both clusters, contrary to the cluster on A07.
3.2.3 Epistatic effects between QTLs on A07 and C06
Seven traits showed a QTL on both A07 and C06. For six of these traits, additive × additive epistatic effects were found between QTLs on chromosomes A07 and C06. The epistatic effect between QTL SD11-2 on A07 and QTL SD11-5 on C06 with an effect of a × a = −7.0 was the strongest epistatic effect in this study (Table 4). When grouping the DH population by the alleles of two markers located near the short day-sensitive clusters, one on A07 (Bn-A07-p21478337, 6.2 cM) and one on C06 (Bn-A07-p21354084, 7.7 cM), the epistatic effect can be observed in the phenotype (Figures 4, 5). Tukey’s test between the four haplotype groups showed no significant difference between the two groups that shared the DH4079 allele on A07 (ADHCDH and ADHCExp) under any condition. Therefore, the DH allele on A07 masked the allelic effect on C06. Except for cool long-day conditions at 11°C, the allele combination AExpCDH resulted in a late flowering group of genotypes.
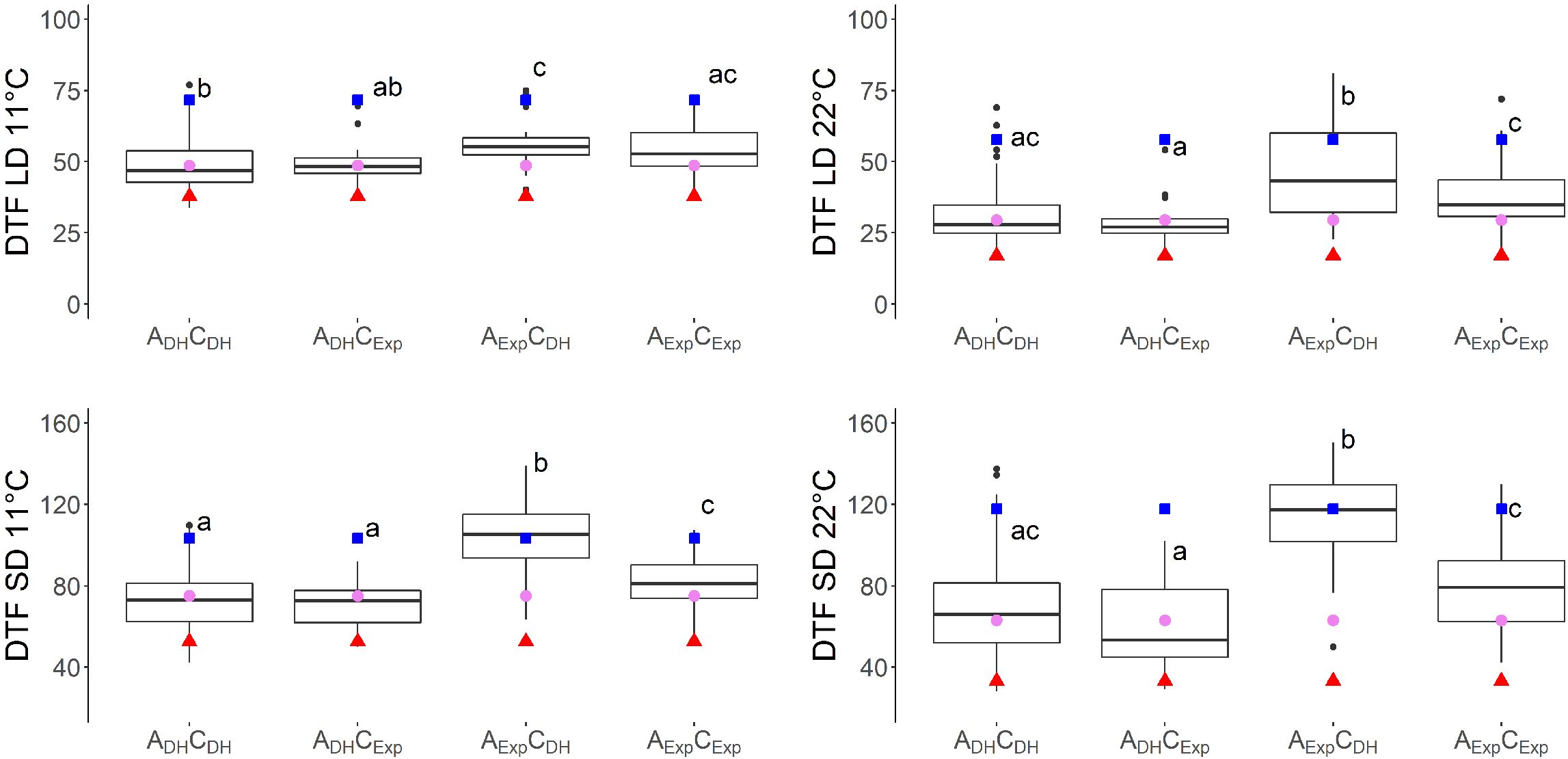
Figure 4. Days to flowering (DTF) in different temperatures (11°C and 22°C) and day length [short day (SD) and long day (LD)] conditions of vernalized DH population divided by alleles of two SNP markers: Bn-A07-p21478337 on A07 at 6.2 cM, indicated by A, and Bn-A07-p21354084 on C06 at 7.7 cM, indicated by C. Subscript “DH” indicates parental DH4079 allele, and subscript “Exp” indicates parental Express617 allele. Letters indicate significantly different subgroups (p ≤ 0.01) tested with Tukey's test within conditions. Phenotypic values of Express617 (blue square), F1 (pink circle), and DH4079 (red triangle) are given.
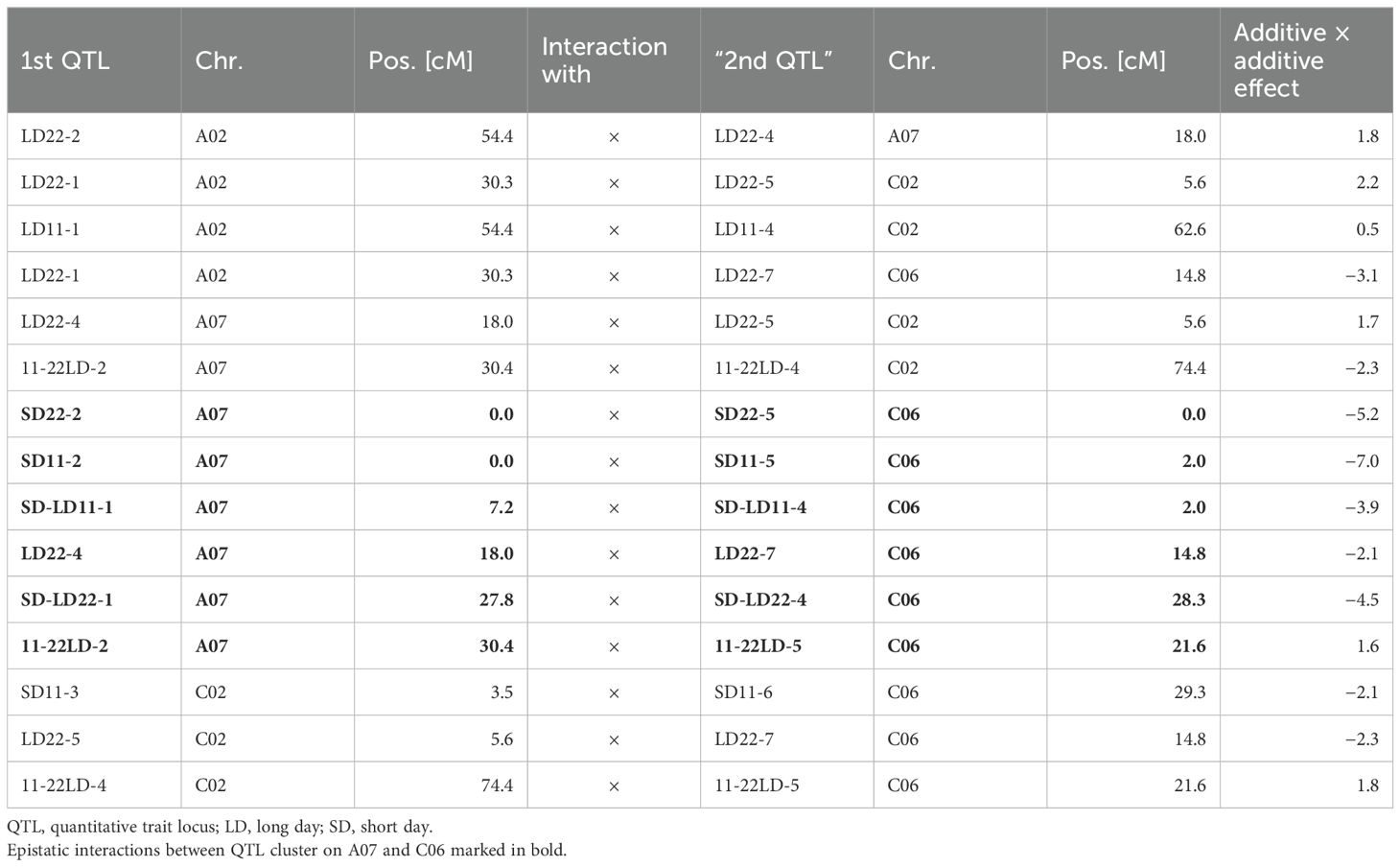
Table 4. Epistatic effects between QTLs (see Table 3) for days to flowering (DTF) under different day length and temperature conditions, as well as for the effect of day length and temperature in the DH4079 × Express617 population.
3.3 Presentation of known flowering time genes in the QTL regions on A07 and C06
The cluster for DTF under short days at the beginning of the genetic map of A07 consisted of three QTLs (SD11-2, SD22-2, and LD11-2). Since the peaks for those three QTLs were located at 0 cM, the real QTLs may be located outside of the genetic map. Two possible candidate genes were located in this genomic region: GA REQUIRING 2 (GA2) was located outside of the genetic map, and TREHALOSE-6-PHOSPHATE SYNTHASE 1 (TPS1) was located between two markers in the confidence interval of QTL LD11-2 (Figure 2). As a possible candidate gene, EARLY FLOWERING IN SHORT DAYS (EFS) was identified for the QTL for the effect of day length differences at 11°C, SD-LD11-1, at 7.2 cM (Figure 2).
The second QTL cluster formed between 24.5 and 30.9 cM on chromosome A07. Here, two major QTLs for the temperature differences were mapped (11-22SD-2 and 11-22LD-2), as well as one QTL for day length differences at 22°C (SD-LD22-1). No known flowering time gene could be found for the confidence interval of SD-LD22-1. With regard to the QTL for temperature difference, the confidence interval of QTL 11-22SD-2 covered a genomic region with AGAMOUS-LIKE 12 (AGL12) and EARLY FLOWERING4-like 2 (ELF4-like2) homologs. The confidence interval of QTL 11-22LD-2 included copies of FLOWERING LOCUS T (FT), AGL12, and RELATED TO AP2 4 (RAP2.4) as possible candidate genes (Figure 2).
At the beginning of C06, the QTL cluster for DTF in SD was located from 0 to 6.7 cM (Figure 3). The peak of SD22-5 and the confidence intervals of all three reached the end of the genetic map, which means that genes located outside the genetic map should be considered; therefore, GA2 is another possible candidate gene (Figure 3) similar to the cluster on A07. Homologs of TPS1 and RAP2.4 were identified as candidate genes for QTLs SD22-5, SD11-5, and SD-LD11-4; EFS was identified as an additional possible candidate gene for SD-LD11-4 (Figure 3). On chromosome C06 between 18.8 and 39.1 cM, a QTL cluster with four QTLs regarding the reaction of DTF to day length and temperature was located. For QTLs 11-22SD-4 and SD-LD22-4, FT, AGL12, ELF4-like2, and RAP2.4 were identified as possible candidate genes. The genes SPL15 and AGL18 are possible candidate genes for SD-LD22-4 and SD11-6.
The physical maps of A07 and C06 show many homologs of the same genes in between them, and QTLs of the same trait are often located near homologous genes on A07 and C06 (Figures 2, 3). According to Chalhoub et al. (2014), these regions are homoeologous in the reference genome Darmor-bzh. Our results indicate that the homology between the chromosomes is also true for the used reference genome Express617 (Lee et al., 2020) and our population.
4 Discussion
4.1 Short days delay flowering after vernalization
The aim of this study was to close a research gap in quantifying the effect of temperature and day length after vernalization on DTF, especially in material with a genetic background in both spring- and winter-type oilseed rape. Plants were vernalized for 9 weeks and grown in two different day length conditions (8 and 16 h) as well as two different temperature conditions (11°C and 22°C). With regard to day length, it was confirmed that short-day conditions delay flowering time. The genetic analysis showed that QTLs of the same trait mapped on homoeologous regions on A07 and C06, which, therefore, resulted in similar candidate genes and epistatic effects.
Previous studies in Arabidopsis and Brassica have shown that these long-day plants show a late flowering genotype under SD conditions. In Brassica species, a change of day length between 12 and 14 h showed the biggest response (King and Kondra, 1986; Nanda et al., 1996). Later flowering genotypes showed stronger responses to photoperiod than early flowering genotypes (King and Kondra, 1986; Robertson et al., 2002). The DH population of this study also showed a strong delay of DTF under SD conditions after the vernalization requirement was fulfilled, even to the point where some genotypes did not even start flowering at the end of the experiment (Figure 1). However, genetic variation in the amount of delay was observed as shown by the calculation of the effects of day length (SD-LD11°C and SD-LD22°C) and the range of DTF (Table 2, Figures 1, 5). The delay of flowering under short days was observed in both parental genotypes (Table 2; Figure 1). The QTL analysis revealed two important genomic regions, where several QTLs for DTF under SD conditions and the effects of day length differences formed clusters. The clusters were located on homoeologous regions on A07 and C06, which means that they were orthologous in the two ancestral species of B. napus: B. rapa and B. oleracea (Chalhoub et al., 2014). The cluster on chromosome A07 was located between 0 and 9.9 cM, where QTLs for DTF under short day (SD22-2 and SD11-2) collocated and a QTL for the effect of day length differences at 11°C SD-LD11-1 was located nearby (Figure 2). The cluster at the beginning of the genetic map of C06 was located between 0 and 6.7 cM, where the confidence interval of QTL SD22-5 overlapped with that of QTLs SD11-5 and SD-LD11-4 (Figure 3). However, the direction of the additive effect was different between the clusters on A07 and C06. For the QTL on A07, the winter oilseed rape Express617 allele delayed flowering in short-day conditions. In contrast to that, the additive effect was negative for the QTL on C06 with the spring-type DH4079 allele delaying flowering in short days. Additionally, epistatic effects were recorded between the respective QTLs (Table 4). The DH alleles on A07 masked the allelic effect on C06, as the group with the ADHCDH haplotype and the group with the ADHCExp haplotype showed no significant difference in their means according to Tukey’s test (Figures 4, 5). The allele combination AExpCDH resulted in the largest delay in flowering under short days and also warm long-day conditions (Figure 4), as can be seen especially in the effect of day length differences, where this allele combination was always significantly different from the others (Figure 5). In both homologous genomic regions, copies of the flowering time candidate genes GA2, EFS, and TPS1 were located. In A. thaliana, TPS1 is the protein responsible for the synthesis of trehalose-6-phosphate (T6P), a sugar signal. TPS1 is necessary for the expression of FT and other flowering-inducing genes (Wahl et al., 2013). However, there is no current knowledge about the influence of TPS1 on flowering through the day length pathways. The protein GA2 is part of the gibberellin synthesis (Berardini et al., 2015). Gibberellin (GA) is a phytohormone that activates flowering but is also involved in many other developmental and stress pathways. Variations in the gene GA2 would, therefore, influence more phenotypic traits than flowering time. EFS is known in Arabidopsis as an FLC activator (recruited by the PAF1-like complex), meaning its activity delays flowering (Kim et al., 2005). Kim et al. (2005) showed that a mutation in EFS accelerates flowering time under short days more than fri or flc mutations with an active EFS. Thus, there is an FLC-independent effect of EFS on flowering time under short days, whose mechanism is not yet known.
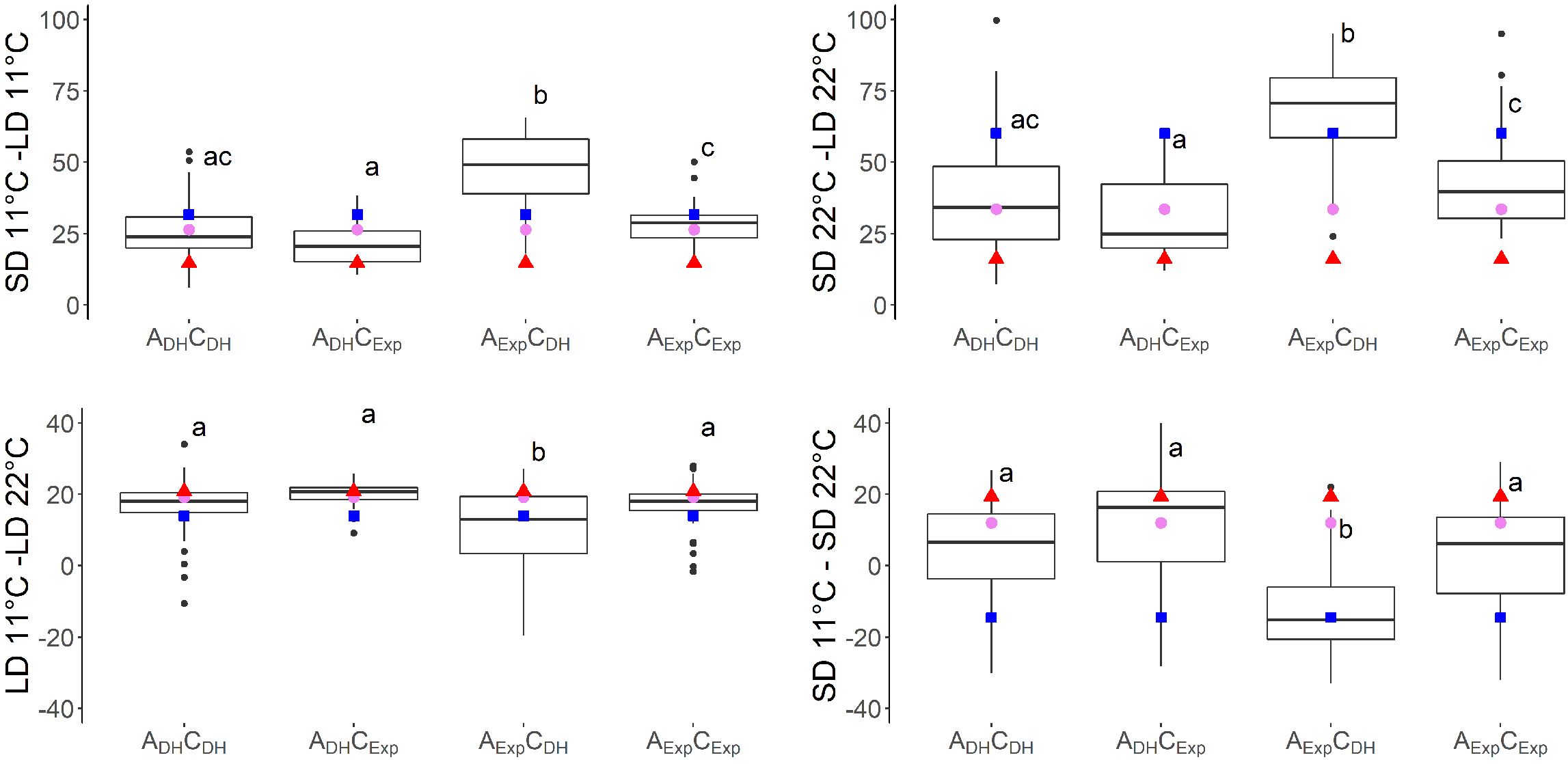
Figure 5. Effects of temperature and day length calculated by subtracting days to flowering (DTF) in different temperatures (11°C minus 22°C) and day length [short day (SD) minus long day (LD)] conditions of vernalized DH population divided by alleles of two SNP markers: Bn-A07-p21478337 on A07 at 6.2 cM, indicated by A, and Bn-A07-p21354084 on C06 at 7.7 cM, indicated by C. Subscript “DH” indicates parental DH4079 allele, and subscript “Exp” indicates parental Express617 allele. Letters indicate significantly different subgroups (p ≤ 0.01) tested with Tukey's test within conditions. Phenotypic values of Express617 (blue square), F1 (pink circle), and DH4079 (red triangle) are given.
Previous QTL studies in B. napus did not present photosensitive QTLs on A07 and C06 but on several other parts of the genome on chromosomes A02, A03, A10, C03, C04, C05, and C09 (Robert et al., 1998; Axelsson et al., 2001; Cai et al., 2008; Luo et al., 2014; Rahman et al., 2018). Therefore, the homoeologous regions on A07 and C06 are novel discoveries for day length-dependent flowering time regulation. This is not surprising considering the complexity of the flowering time regulation. Furthermore, DH4079 contributed an allele on A07, which masked the effect of the C06 alleles (Table 3; Figure 4). The C06 DH4079 allele could only delay flowering in the absence of the A07 DH4079 allele (Figure 4); hence, the C06 DH4079 allele is masked in the parent. In conclusion, epistatic effects should not be ignored, especially in a plant with a complex genome like B. napus, where several homolog copies of a gene exist. Further research is needed to reveal the complexity of flowering time regulation found in B. napus and in these two homolog regions.
4.2 The effect of temperature on flowering time is dependent on day length and genotype
We calculated the effect of temperature on DTF by subtracting DTF at 22°C from DTF at 11°C (11°–22°C); a positive value stands for earlier flowering at warmer temperatures and a negative number for later flowering. Surprisingly, higher temperatures could either delay or accelerate flowering, depending on the genotype (Table 2). While most plant species react with earlier flowering to higher temperatures due to warmer temperatures, it has been shown that high temperatures can also delay flowering (Fitter and Fitter, 2002; Jiang, 2023), something also observed in B. napus (Abelenda et al., 2023).
The ANOVA showed that the effect of temperature on DTF was much smaller than the effect of the interaction between temperature and day length (Table 1). In the phenotypic analysis, DH4079 as a Swedish spring type showed a reaction to temperature that was independent of day length and vice versa (Table 2), so when sown in spring, the cultivar can react to warm temperatures without a negative interaction with day length. In contrast, German winter oilseed rape Express617 reacted differently to warmer temperatures depending on the day length; under LD, the flowering was 14 days earlier at 22°C, but under SD warmer temperatures, the flowering was delayed by 15 days. This is the first study to show how important it is to research the combined effect of day length and temperature after vernalization in winter oilseed rape. It should be noted, that in this experiment, the temperatures were constant and did not change between night and day. Other conditions may have led to different results since thermosensing is discussed to vary between day and night (Xiao et al., 2013; Hayes et al., 2021).
We found that in our QTL analysis, homoeologous regions on the linkage groups C06 and A07 responsible for the regulation of flowering time with QTL clusters for traits related to flowering depended on temperature and day length (Table 3, Figures 2, 3). The cluster on A07 between 24.5 cM and 30.9 cM was comprised of QTLs 11-22LD-2, 11-22SD-2, and SD-LD22-1 (Figure 2). The cluster on C06 between 18.8 and 39.1 cM was comprised of QTLs 11-22LD-4, 11-22SD-5, SD-LD22-4, and SD11-6 (Figure 3). On A07, it was the Express617 allele and, on C06, the DH4079 allele that delayed DTF at 22°C or under short days. The two homoeologous clusters showed epistatic effects between QTLs 11-22LD2 and 11-22LD-5 (a × a = 1.6), SD-LD22-1 and SD-LD22-4 (a × a = −4.5), and LD22-4 and LD22-7 (a × a = −2.1). QTLs 11-22SD-2 and 11-22SD-4 showed no epistasis. In each of those genomic regions, homologous copies of the flowering regulator genes ELF4-LIKE 2, AGL12, RAP2.4, and FT were located. No details about the function of ELF4-LIKE 2 are known yet. In Arabidopsis, ELF4-LIKE 2 could not rescue elf4 mutants (Lin et al., 2019), which does not exclude another function in flowering time regulation. In Arabidopsis, AGL12 has been shown to positively regulate flowering specifically in the apical meristem due to long-day conditions or independently due to higher temperatures (Tapia-López et al., 2008; Rodríguez-Bolaños et al., 2023). However, vernalization has been reported to negate the influence of AGL12 (Tapia-López et al., 2008), and in our experiment, vernalization was applied. Transcription factor RAP2.4 is involved in many plant developmental processes. Its transcription is induced by abiotic stress, including heat stress, and is reduced by light, and overexpression has been shown to result in earlier flowering time in Arabidopsis (Lin et al., 2008). A temperature-dependent activity of the FT protein was shown, as low temperatures reduce the function of FT in Arabidopsis (Teper-Bamnolker and Samach, 2005). Under warm short-day conditions, PIF4 is positively regulating FT by binding to the promotor and activating transcription (Kumar et al., 2012). Changes in the promotor region of FT could cause genotype-specific differences in the regulation of flowering time by other transcription factors. For B. napus, differences in FT expression have been shown in spring-type cultivars between 21°C and 28°C (Abelenda et al., 2023) for FT homologs A02, A07, and C06. Ghanbari (2016) found FT on C06 as the candidate gene for a flowering time QTL in an autumn sown field trial of the DH population of Sansibar × Oase, two winter oilseed rape genotypes, thus influencing flowering time in spring after full vernalization. It can be concluded that there is a genotype-specific interaction between temperature and day length. When sown in the field in autumn, winter oilseed rape should not induce flowering on warm winter days, while spring types may induce flowering earlier if warm temperatures permit it. Due to the different regulations of FT through temperature under short days, FT is a viable candidate gene, already supported by other studies on chromosomes A07 and C06 (Abelenda et al., 2023; Ghanbari, 2016).
4.3 Outlook: more research on the effect of changing climate conditions in spring on winter rapeseed is needed
In conclusion, day length had an immense influence on flowering time in the DH4079 × Express617 population, and novel QTL regions were discovered on chromosomes A07 and C06. The gene EFS, which represses flowering under short days, was identified as a viable candidate gene. The influence of temperature × day length interactions on flowering time after vernalization is less studied for rapeseed, although with pending climate change, this may become an issue when warm spring temperatures shift to earlier months when the days are shorter. We found that the effects for temperature and day length interactions are greater than just the temperature effect and suggest that these two important abiotic factors should not be studied independently. The effect of temperature under short days is also genotype-specific, and the combination of higher temperatures and short-day conditions can either delay or accelerate flowering time.
Both parental genotypes had alleles, which suppressed flowering under short days and warm temperatures, but on different loci. On C06, the alleles derived from the spring-type parent DH4079 were responsible for this effect, while on A07, the alleles from the winter-type parent Express617 were responsible. In the presence of the DH4079 allele on A07, the effects of the alleles on C06 were masked, and the delay in flowering time through short-day conditions was not expressed. The QTLs on C06 and A07 were located in homoeologous regions and resulted consequently in the same candidate genes. This genetic diversity is a valuable basis for breeding B. napus to counter the environmental effects of climate change.
Data availability statement
The original contributions presented in the study are included in the article/Supplementary Material. Further inquiries can be directed to the corresponding author.
Author contributions
EH: Writing – original draft, Writing – review & editing, Conceptualization, Data curation, Formal analysis, Investigation, Methodology, Project administration, Validation. AS: Methodology, Supervision, Writing – review & editing. CM: Methodology, Supervision, Writing – review & editing, Conceptualization, Funding acquisition, Investigation, Project administration, Resources, Validation.
Funding
The author(s) declare that financial support was received for the research and/or publication of this article. This research was funded by the DFG (Deutsche Forschungsgemeinschaft) in the Priority Program 1530 “Flowering Time Control: from natural variation to crop improvement”, Project number 270184074.
Acknowledgments
We acknowledge support by the Open Access Publication Funds of the Göttingen University.
Conflict of interest
The authors declare that the research was conducted in the absence of any commercial or financial relationships that could be construed as a potential conflict of interest.
Generative AI statement
The author(s) declare that Generative AI was used in the creation of this manuscript. For editing the abstract and the title, Chat-AI (Meta LLaMA 3.1 8B Instruct) provided by the GWDG (Gesellschaft für wissenschaftliche Datenverarbeitung mbH Göttingen) was utilized with the prompts provided by the Generative AI as included in the Supplementary File S2.
Publisher’s note
All claims expressed in this article are solely those of the authors and do not necessarily represent those of their affiliated organizations, or those of the publisher, the editors and the reviewers. Any product that may be evaluated in this article, or claim that may be made by its manufacturer, is not guaranteed or endorsed by the publisher.
Supplementary material
The Supplementary Material for this article can be found online at: https://www.frontiersin.org/articles/10.3389/fpls.2025.1513353/full#supplementary-material
Supplementary File S2 | Chat log with Chat-AI to improve the abstract and title.
References
Abelenda, J. A., Trabanco, N., Del Olmo, I., Pozas, J., Del Martín-Trillo, M. M., Gómez-Garrido, J., et al. (2023). High ambient temperature impacts on flowering time in Brassica napus through both H2A.Z-dependent and independent mechanisms. Plant Cell Environ. 46, 1427–1441. doi: 10.1111/pce.14526
Amasino, R. M., Michaels, S. D. (2010). The timing of flowering. Plant Physiol. 154, 516–520. doi: 10.1104/pp.110.161653
Axelsson, T., Shavorskaya, O., Lagercrantz, U. (2001). Multiple flowering time QTLs within several Brassica species could be the result of duplicated copies of one ancestral gene. Genome 44, 856–864. doi: 10.1139/g01-082
Berardini, T. Z., Reiser, L., Li, D., Mezheritsky, Y., Muller, R., Strait, E., et al. (2015). The Arabidopsis information resource: Making and mining the “gold standard” annotated reference plant genome. Genesis 53, 474–485. doi: 10.1002/dvg.22877
Blümel, M., Dally, N., Jung, C. (2015). Flowering time regulation in crops—what did we learn from Arabidopsis? Curr. Opin. Biotechnol. 32, 121–129. doi: 10.1016/j.copbio.2014.11.023
Cai, C. C., Tu, J. X., Fu, T. D., Chen, B. Y. (2008). The genetic basis of flowering time and photoperiod sensitivity in rapeseed Brassica napus L. Russ J. Genet. 44, 326–333. doi: 10.1134/S1022795408030137
Chalhoub, B., Denoeud, F., Liu, S., Parkin, I. A. P., Tang, H., Wang, X., et al. (2014). Plant genetics. Early allopolyploid evolution in the post-Neolithic Brassica napus oilseed genome. Science 345, 950–953. doi: 10.1126/science.1253435
Cock, P. J. A., Chilton, J. M., Grüning, B., Johnson, J. E., Soranzo, N. (2015). NCBI BLAST+ integrated into Galaxy. Gigascience 4, 39. doi: 10.1186/s13742-015-0080-7
Ferreira, M. E., Satagopan, J., Yandell, B. S., Williams, P. H., Osborn, T. C. (1995). Mapping loci controlling vernalization requirement and flowering time in Brassica napus. Theor. Appl. Genet. 90, 727–732. doi: 10.1007/BF00222140
Ferrie, A. (2003). “Microspore culture of Brassica species,” in Doubled Haploid Production in Crop Plants. Eds. Maluszynski, M., Kasha, K. J., Forster, B. P., Szarejko, I. (Springer Netherlands, Dordrecht), 205–215.
Fitter, A. H., Fitter, R. S. R. (2002). Rapid changes in flowering time in British plants. Science 296, 1689–1691. doi: 10.1126/science.1071617
Ghanbari, M. (2016). Inheritance and genetic variation of shoot elongation before winter in oilseed rape (Brassica napus L.). Georg-August-University, Göttingen. Plant breeding.
Hayes, S., Schachtschabel, J., Mishkind, M., Munnik, T., Arisz, S. A. (2021). Hot topic: Thermosensing in plants. Plant Cell Environ. 44, 2018–2033. doi: 10.1111/pce.13979
Jaeger, K. E., Pullen, N., Lamzin, S., Morris, R. J., Wigge, P. A. (2013). Interlocking feedback loops govern the dynamic behavior of the floral transition in Arabidopsis. Plant Cell 25, 820–833. doi: 10.1105/tpc.113.109355
Jiang, D. (2023). Complex regulation of flowering by high temperatures. Plant Cell Environ. 46, 1423–1426. doi: 10.1111/pce.14574
Kim, S. Y., He, Y., Jacob, Y., Noh, Y.-S., Michaels, S., Amasino, R. (2005). Establishment of the vernalization-responsive, winter-annual habit in Arabidopsis requires a putative histone H3 methyl transferase. Plant Cell 17, 3301–3310. doi: 10.1105/tpc.105.034645
Kim, D.-H., Sung, S. (2014). Genetic and epigenetic mechanisms underlying vernalization. Arabidopsis Book 12, e0171. doi: 10.1199/tab.0171
King, J. R., Kondra, Z. P. (1986). Photoperiod response of spring oilseed rape (Brassica napus L. and B. campestris L.). Field Crops Res. 13, 367–373. doi: 10.1016/0378-4290(86)90037-7
Kumar, S. V., Lucyshyn, D., Jaeger, K. E., Alós, E., Alvey, E., Harberd, N. P., et al. (2012). Transcription factor PIF4 controls the thermosensory activation of flowering. Nature 484, 242–245. doi: 10.1038/nature10928
Lancashire, P. D., Bleiholder, H., van Boom, T., Langelüddeke, P., Stauss, R., Weber, E., et al. (1991). A uniform decimal code for growth stages of crops and weeds. Ann. Appl. Biol. 119, 561–601. doi: 10.1111/j.1744-7348.1991.tb04895.x
Lee, H., Chawla, H. S., Obermeier, C., Dreyer, F., Abbadi, A., Snowdon, R. (2020). Chromosome-scale assembly of winter oilseed rape brassica napus. Front. Plant Sci. 11. doi: 10.3389/fpls.2020.00496
Lin, R.-C., Park, H.-J., Wang, H.-Y. (2008). Role of Arabidopsis RAP2.4 in regulating light- and ethylene-mediated developmental processes and drought stress tolerance. Mol. Plant 1, 42–57. doi: 10.1093/mp/ssm004
Lin, K., Zhao, H., Gan, S., Li, G. (2019). Arabidopsis ELF4-like proteins EFL1 and EFL3 influence flowering time. Gene 700, 131–138. doi: 10.1016/j.gene.2019.03.047
Luo, Y. X., Luo, C. Y., Du, D. Z., Fu, Z., Yao, Y. M., Xu, C. C., et al. (2014). Quantitative trait analysis of flowering time in spring rapeseed (B. napus L.). Euphytica 200, 321–335. doi: 10.1007/s10681-014-1140-2
Major, D. J. (1980). Photoperiod response characteristics controlling flowering of nine crop species. Can. J. Plant Sci. 60, 777–784. doi: 10.4141/cjps80-115
Mendham, N. J., Salisbury, P. A. (1995). “Physiology: crop development, growth and yield,” in Brassica oilseeds: production and utilization. Eds. Kimber, D., McGregor, D. I. (CAB International, Wallingford), 11–64.
Nanda, R., Bhargava, S. C., Tomar, D., Rawson, H. M. (1996). Phenological development of Brassica campestris, B. juncea, B. napus and B. carinata grown in controlled environments and from 14 sowing dates in the field. Field Crops Res. 46, 93–103. doi: 10.1016/0378-4290(95)00090-9
Nelson, M. N., Rajasekaran, R., Smith, A., Chen, S., Beeck, C. P., Siddique, K. H. M., et al. (2014). Quantitative trait loci for thermal time to flowering and photoperiod responsiveness discovered in summer annual-type Brassica napus L. PloS One 9, e102611. doi: 10.1371/journal.pone.0102611
Quiroz, S., Yustis, J. C., Chávez-Hernández, E. C., Martínez, T., La Sanchez, M., d., P., et al. (2021). Beyond the genetic pathways, flowering regulation complexity in arabidopsis thaliana. Int. J. Mol. Sci. 22 (11), 5716. doi: 10.3390/ijms22115716
Rahman, H., Bennett, R. A., Kebede, B. (2018). Molecular mapping of QTL alleles of Brassica oleracea affecting days to flowering and photosensitivity in spring Brassica napus. PloS One 13, e0189723. doi: 10.1371/journal.pone.0189723
Raman, H., Raman, R., Coombes, N., Song, J., Prangnell, R., Bandaranayake, C., et al. (2016). Genome-wide association analyses reveal complex genetic architecture underlying natural variation for flowering time in canola. Plant Cell Environ. 39, 1228–1239. doi: 10.1111/pce.12644
R. Core Team (2019). R: A language and environment for statistical computing (Vienna, Austria). Available at: https://www.R-project.org/.
Richter, J. C., Möllers, C. (2018). Genetic variation for vernalization requirement of winter oilseed rape. Acta Hortic. 1202, 87–92. doi: 10.17660/ActaHortic.2018.1202.13
Robert, L. S., Robson, F., Sharpe, A., Lydiate, D., Coupland, G. (1998). Conserved structure and function of the Arabidopsis flowering time gene CONSTANS in Brassica napus. Plant Mol. Biol. 37, 763–772. doi: 10.1023/a:1006064514311
Robertson, M. J., Asseng, S., Kirkegaard, J. A., Wratten, N., Holland, J. F., Watkinson, A. R., et al. (2002). Environmental and genotypic control of time to flowering in canola and Indian mustard. Aust. J. Agric. Res. 53, 793. doi: 10.1071/AR01182
Rodríguez-Bolaños, M., Martínez, T., Juárez, S., Quiroz, S., Domínguez, A., Garay-Arroyo, A., et al. (2023). XAANTAL1 reveals an additional level of flowering regulation in the shoot apical meristem in response to light and increased temperature in arabidopsis. Int. J. Mol. Sci. 24 (16), 12773. doi: 10.3390/ijms241612773
Salisbury, P. A., Green, A. G. (1991). “Developmental responses in spring canola cultivars,” in GCIRC 1991 Congress, 1769–1774.
Schiessl, S. (2020). Regulation and subfunctionalization of flowering time genes in the allotetraploid oil crop brassica napus. Front. Plant Sci. 11. doi: 10.3389/fpls.2020.605155
Schiessl, S., Huettel, B., Kuehn, D., Reinhardt, R., Snowdon, R. (2017). Post-polyploidisation morphotype diversification associates with gene copy number variation. Sci. Rep. 7, 41845. doi: 10.1038/srep41845
Schiessl, S. V., Quezada-Martinez, D., Tebartz, E., Snowdon, R. J., Qian, L. (2019). The vernalisation regulator FLOWERING LOCUS C is differentially expressed in biennial and annual Brassica napus. Sci. Rep. 9, 14911. doi: 10.1038/s41598-019-51212-x
Schilbert, H. M., Schöne, M., Baier, T., Busche, M., Viehöver, P., Weisshaar, B., et al. (2021). Characterization of the brassica napus flavonol synthase gene family reveals bifunctional flavonol synthases. Front. Plant Sci. 12. doi: 10.3389/fpls.2021.733762
Song, Y. H., Ito, S., Imaizumi, T. (2013). Flowering time regulation: Photoperiod- and temperature-sensing in leaves. Trends Plant Sci. 18, 575–583. doi: 10.1016/j.tplants.2013.05.003
Sun, F., Fan, G., Hu, Q., Zhou, Y., Guan, M., Tong, C., et al. (2017). The high-quality genome of Brassica napus cultivar ‘ZS11’ reveals the introgression history in semi-winter morphotype. Plant J. 92, 452–468. doi: 10.1111/tpj.13669
Takagi, H., Hempton, A. K., Imaizumi, T. (2023). Photoperiodic flowering in Arabidopsis: Multilayered regulatory mechanisms of CONSTANS and the florigen FLOWERING LOCUS T. Plant Commun. 4, 100552. doi: 10.1016/j.xplc.2023.100552
Tapia-López, R., García-Ponce, B., Dubrovsky, J. G., Garay-Arroyo, A., Pérez-Ruíz, R. V., Kim, S.-H., et al. (2008). An AGAMOUS-related MADS-box gene, XAL1 (AGL12), regulates root meristem cell proliferation and flowering transition in Arabidopsis. Plant Physiol. 146, 1182–1192. doi: 10.1104/pp.107.108647
Teper-Bamnolker, P., Samach, A. (2005). The flowering integrator FT regulates SEPALLATA3 and FRUITFULL accumulation in Arabidopsis leaves. Plant Cell 17, 2661–2675. doi: 10.1105/tpc.105.035766
Utz, H. F. (2011). “PLABSTAT: Ein Computerprogramm zur statistischen Analyse von pflanzenzüchterischen Experimenten,” in Institute of Plant Breeding, Seed Science and Population Genetics (Hohenheim: University of Hohenheim).
Valdés, A., Clemens, R., Möllers, C. (2018). Mapping of quantitative trait loci for microspore embryogenesis-related traits in the oilseed rape doubled haploid population DH4069 × Express 617. Mol. Breed. 38, 73. doi: 10.1007/s11032-018-0822-1
Voorrips, R. E. (2002). MapChart: Software for the graphical presentation of linkage maps and QTLs. J. Heredity. 93, 77–78. doi: 10.1093/jhered/93.1.77
Wahl, V., Ponnu, J., Schlereth, A., Arrivault, S., Langenecker, T., Franke, A., et al. (2013). Regulation of flowering by trehalose-6-phosphate signaling in Arabidopsis thaliana. Science 339, 704–707. doi: 10.1126/science.1230406
Wang, S., Basten, C. J., Zeng, Z.-B. (2012). Windows QTL cartographer 2.5. (Raleigh, NC: Department of Statistics, North Carolina State University).
Xiao, D., Shen, H.-R., Zhao, J.-J., Wei, Y.-P., Liu, D.-R., Hou, X.-L., et al. (2019). Genetic dissection of flowering time in Brassica rapa responses to temperature and photoperiod. Plant Sci. 280, 110–119. doi: 10.1016/j.plantsci.2018.10.027
Keywords: epistasis, genetic variation, circadian rhythm, photoperiod, gene homology, winter rape, epigenetic, vernalization
Citation: Heinrich E, Schierholt A and Möllers C (2025) QTL mapping of flowering time in Brassica napus: a study on the interplay between temperature and day length after vernalization. Front. Plant Sci. 16:1513353. doi: 10.3389/fpls.2025.1513353
Received: 18 October 2024; Accepted: 27 March 2025;
Published: 09 May 2025.
Edited by:
Xiaodong Yang, Yangzhou University, ChinaReviewed by:
Md Mostofa Uddin Helal, Shanxi Agricultural University, ChinaKaushal Pratap Singh, Directorate of Rapeseed Mustard Research (DRMR), India
Copyright © 2025 Heinrich, Schierholt and Möllers. This is an open-access article distributed under the terms of the Creative Commons Attribution License (CC BY). The use, distribution or reproduction in other forums is permitted, provided the original author(s) and the copyright owner(s) are credited and that the original publication in this journal is cited, in accordance with accepted academic practice. No use, distribution or reproduction is permitted which does not comply with these terms.
*Correspondence: Eva Heinrich, ZXZhLmhlaW5yaWNoQGFnci51bmktZ29ldHRpbmdlbi5kZQ==