- 1Aquatic and Crop Resource Development, National Research Council of Canada (NRC), Saskatoon, SK, Canada
- 2Graduate School of Agriculture and Life Sciences, The University of Tokyo, Tokyo, Japan
- 3Relica Genomics, Saskatoon, SK, Canada
Chloroplast transformation technology has become a powerful platform for generating plants that express foreign proteins of pharmaceutical and agricultural importance at high levels. Chloroplasts are often chosen as attractive targets for the introduction of new agronomic traits because they have their own genome and protein synthesis machinery. Certain valuable traits have been genetically engineered into plastid genomes to improve crop yield, nutritional quality, resistance to abiotic and biotic stresses, and the production of industrial enzymes and therapeutic proteins. Synthetic biology approaches aim to play an important role in expressing multiple genes through plastid engineering, without the risk of pleiotropic effects in transplastomic plants. Despite many promising laboratory-level successes, no transplastomic crop has been commercialized to date. This technology is mostly confined to model species in academic laboratories and needs to be expanded to other agronomically important crop species to capitalize on its significant commercial potential. However, in recent years, some transplastomic lines are progressing in field trials, offering hope that they will pass regulatory approval and enter the marketplace. This review provides a comprehensive summary of new and emerging technologies employed for plastid transformation and discusses key synthetic biology elements that are necessary for the construction of modern transformation vectors. It also focuses on various novel insights and challenges to overcome in chloroplast transformation.
1 Introduction
In plants, DNA is located in three distinct compartments: the nucleus, chloroplasts, and mitochondria, each of which carries its own genome and expresses heritable traits (Sugiura, 1992). Chloroplasts are endosymbiotically derived from once free-living bacteria and possess largely prokaryotic gene expression machinery and regulatory mechanisms (Barkan and Goldschmidt-Clermont, 2000). Owing to their origins as independent photosynthetic microbes, plastids serve as biosynthetic centers that carry out important metabolic activities including photosynthesis, sequestration of carbon, production of starch, and synthesis of amino acids, fatty acids, and pigments. They are also key compartments for sulfur and nitrogen metabolic processes (Kang et al., 2003). Chloroplasts have become attractive alternative targets for genetic engineering approaches, largely because of their capacity for overexpressing foreign genes and their maternal mode of inheritance (Gosal et al., 2009). Furthermore, chloroplast engineering technology has advantages over nuclear transformation techniques, as it enables the precise targeting of expression cassettes within the chloroplast genome (Daniell et al., 2016). It also enables polycistronic expression and eliminates the position effects by integrating transgenes through homologous recombination (Bock, 2007). The ability to produce stable chloroplast transformants has progressed considerably, from initial successes in tobacco (Nicotiana tabacum L.) to more than 20 species of flowering plants, including both dicots and monocots (Svab et al., 1990) (Table 1). These advances have opened up exciting possibilities for introducing novel genes of pharmaceutical (Davoodi-Semiromi et al., 2010), industrial (Verma et al., 2010), and agronomic (De Cosa et al., 2001) importance into crop plants. Despite these successes, there are still challenges that must be overcome to expand the usefulness of this powerful technology. Limited protocols for efficient plant regeneration and antibiotic selection in many crop species remain significant obstacles to the expansion of reliable chloroplast transformation systems across the plant kingdom (Bock, 2007). This review discusses novel approaches developed in chloroplast genetic transformation in recent years and highlights advancements in the understanding of the synthetic elements required for designing and constructing transformation vectors for multiple gene expression. New insights and challenges associated with chloroplast engineering and transformation are also summarized.
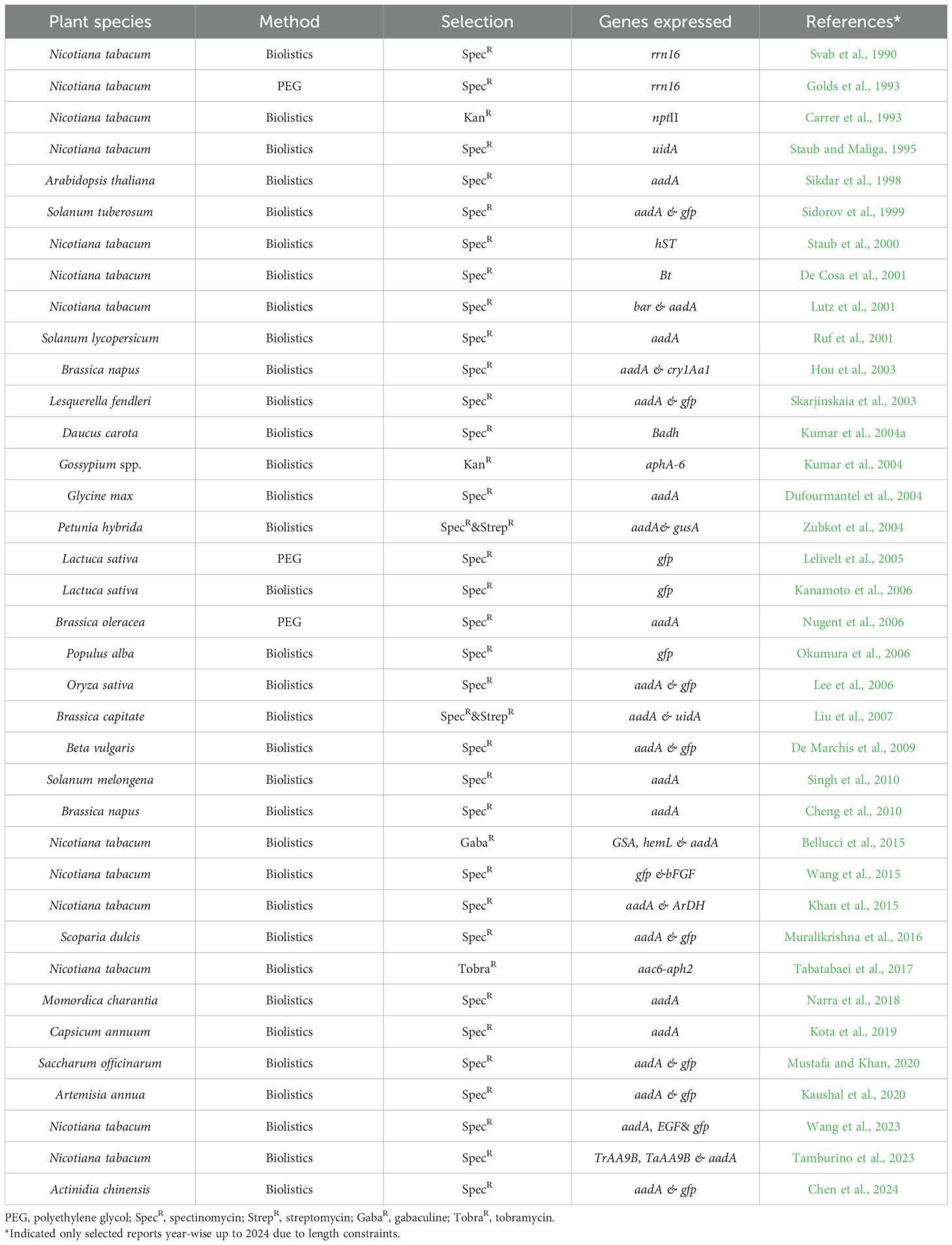
Table 1. Plastid transformation methods and selection conditions developed for different plant species.
2 Plastid transformation technology
There are two key aspects necessary for chloroplast genome engineering: the construction of transformation vectors, which typically include flanking sequences for homologous recombination and regulatory elements such as promoters, 5’ untranslated regions (UTRs), 3’ UTRs, terminators, intercistronic expression elements (IEEs), and cognate binding sites in plastid vectors for pentatricopeptide repeat (PPR) RNA-binding proteins, all of which regulate the expression of gene cassettes; and gene delivery methods for inserting exogenous genes along with selectable markers into the native chloroplast genome.
2.1 Construction of chloroplast transformation vectors
2.1.1 Flanking sequences
The insertion of foreign gene sequences into plastid DNA is typically carried out at the genetic level via homologous recombination, where the expression cassette is flanked by DNA sequences (usually between 1 and 1.5 kb in size) that are homologous to the native chloroplast genome (Iamtham and Day, 2000; Klaus et al., 2004). Many intergenic sites for transgene incorporation have been explored, but limited research has been done to examine the expression of similar transgenes at different integration sites (Maliga, 2004). The selection of target loci in the plastome may have an effect on the level of protein accumulation (Bock, 2014). The trnI-trnA (Daniell et al., 1998) and trnV-3’rps12 (Skarjinskaia et al., 2003) sites, located in the inverted repeat (IR) region of the plastome, allow rapid gene copying into IRA and IRB regions. Integration of the transgene into these transcriptionally active spacer regions was found to be effective due to gene dosage, which resulted in increased expression levels in chloroplasts (Herz et al., 2005). The rbcL-accD (Svab and Maliga, 1993) and trnfM-trnG (Ruf et al., 2001) sites are other commonly used locations, situated in the large single copy region (LSC) of the plastome, and a gene inserted into these regions should have only one copy present per ptDNA (plastid DNA) (Figure 1A). Details of chloroplast vector systems mentioned in previously published research articles provide additional information regarding the factors to consider while choosing and designing a vector; interested readers can find more details in those articles (Table 1).
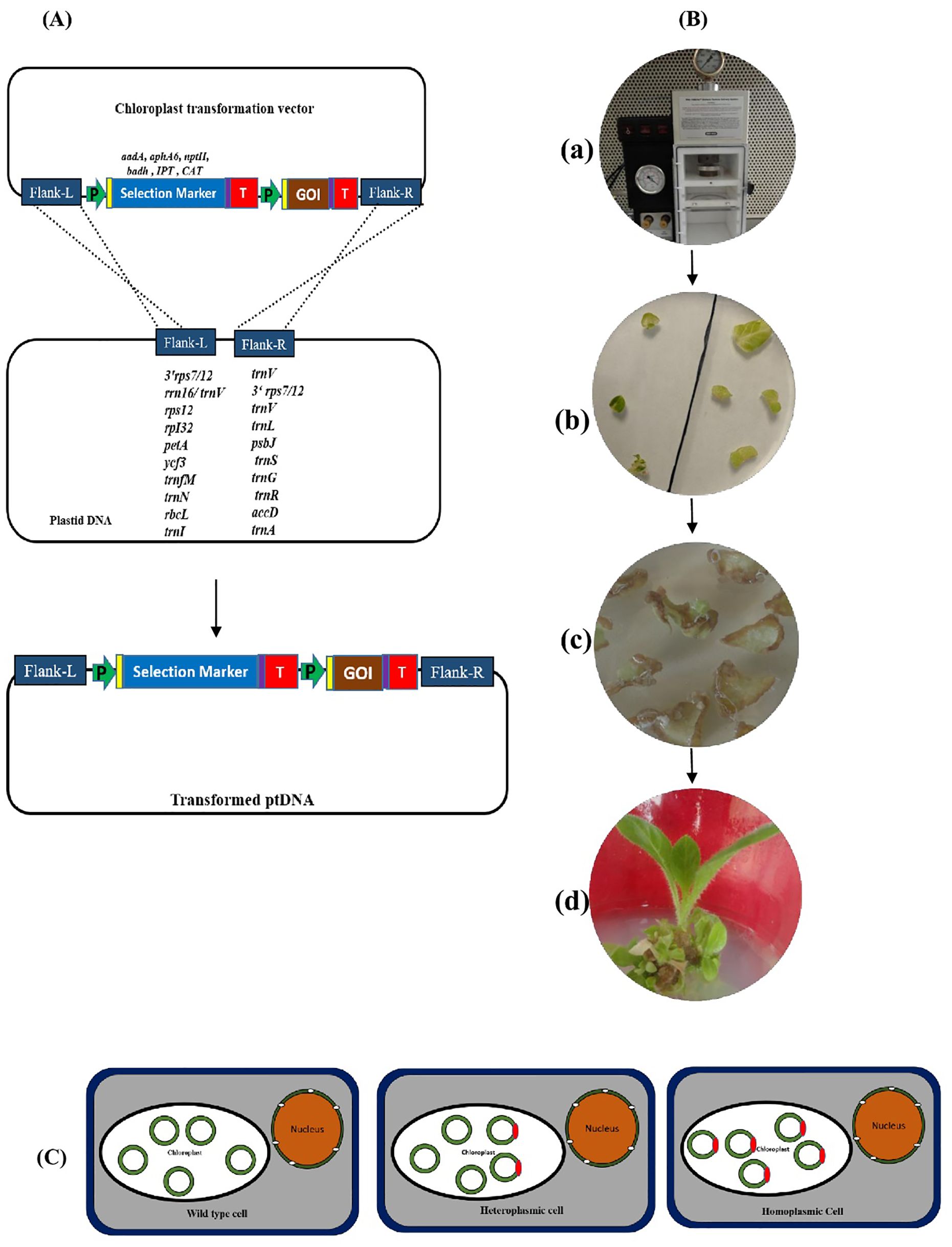
Figure 1. Schematic representation of the plastid transformation method. (A) Process of homologous recombination for integration of the gene of interest (GOI) and selection marker (SM) cassette into the native plastid genome. A typical cassette comprises a promoter (P), 5’ UTR (yellow boxes), coding region, 3’ UTR (blue boxes), and terminator (T). (B) Gene delivery into chloroplasts in leaf explants (tobacco as an example; Source: unpublished photographs of Muralikrishna Narra) using a biolistic delivery system (a) The gold or tungsten microparticles coated with desired plasmid DNA were shot on to the abaxial surface of explants using a gene gun. (b) The bombarded leaves were cut into small discs and placed on regeneration media with the appropriate concentration of antibiotics. (c) The explants were subjected to two or three rounds of selection to obtain homoplasmic shoots within 2–3 months. (d) The regenerated transplastomic plantlet. (C) Different stages of plant cells that have undergone plastid transformation (Wild-type cell with native plastid genome, heteroplasmic cell with a mixture of both transformed and untransformed copies of plastid genomes, and homoplasmic cell with fully transformed plastid genomes).
2.1.2 Selectable marker genes
Selectable marker genes are crucial for the identification and positive selection of transformants, enabling the generation of plants with uniformly transformed plastids (Maliga, 2003) (Figure 1A). Point mutations in the 16S rRNA sequence that cause spectinomycin and streptomycin insensitivity have led to the creation of “binding-type” vectors, which contrast with more commonly used heterologous marker genes, such as aminoglycoside adenylyl transferase (aadA) (Kavanagh et al., 1999; Nugent et al., 2005; Svab et al., 1990). The aadA gene is the most efficient for chloroplast transformation due to its strong dual resistance to spectinomycin and streptomycin, making the selection relatively unambiguous across a variety of tissue types (Svab and Maliga, 1993; Ye et al., 2001). “Binding-type” markers eliminate the need for foreign antibiotic resistance genes and provide a different means of selection for studying antibiotic insensitivity. This has been successfully demonstrated in tobacco protoplast systems (Craig et al., 2008). Alternative marker genes employed for selection include npt II (Carrer et al., 1993), aphA-6 (Huang et al., 2002), cat (Li et al., 2011), and tobramycin (Tabatabaei et al., 2017). The integration of nuclear genetics and plastid genome manipulation has enabled the development of a novel selection method, which was recently applied in ACC2-knockout lines (Acetyl-CoA carboxylase) of Arabidopsis through plastid transformation. Elimination of nuclear ACC2 function simplifies the procedure for efficient selection, resulting in the generation of spectinomycin-resistant plastid transformants at an ~100-fold enhanced frequency (Yu et al., 2017). In another study, fertile transplastomic Arabidopsis plants were generated using root-derived microcalli via biolistic transformation at high frequency in CRISPR/Cas9-generated ACC2-knockout explants. Null mutations in ACC2, which encodes a plastid-targeted acetyl-coenzyme A carboxylase, cause hypersensitivity to spectinomycin, making the selection procedure more efficient (Ruf et al., 2019). Okuzaki et al. (2020) recently reported a new strategy of using the barnase–barstar system for the production of homoplasmic plastid transformants. This system uses both nuclear-transformed estradiol-inducible barnase with a plastid transit signal and a plastid targeting vector with barstar. Heteroplasmic plants that contain wild-type plastids will be damaged when treated with estradiol due to barnase activity, but transformed plastids expressing barstar will be rescued and remain active, promoting a homoplasmic condition.
2.1.3 Marker excision elements
Introduced selectable marker genes may need to be eliminated from transplastomic plants in order to address biosafety, commercial applicability, and public acceptance issues (Maliga, 2003). From a productivity perspective, expression of the marker gene in transplastomic lines should impose minimal metabolic burden once homoplasmy is achieved. Several strategies have been developed to eliminate antibiotic-resistant markers in the final products (Daniell et al., 1998; Maliga, 2003). Homology-based excision is the simplest approach for excising a marker gene, relying on the plastid’s native homologous recombination machinery, which excises any sequence between two directly oriented repeats (Iamtham and Day, 2000). Excision by phage site-specific recombinases relies on the removal of a marker gene from transplastomic plants flanked by two directly oriented recombinase target sites through the introduction of a nuclear-encoded, plastid-targeted recombinase gene (Kittiwongwattana et al., 2007). Formation of a transient cointegrate structure for the marker gene, which is positioned outside the targeting region during the initial recombination with one of the targeting sequences, causes the marker gene to be subsequently lost during a second recombination event, which occurs after the removal of antibiotic selection (Klaus et al., 2004). The co-transformation segregation approach involves target insertions at two different locations in the plastid genome with two constructs: one carrying a selective marker and the other carrying a non-selected gene. The selection of transplastomic clones for the marker gene will also carry an insertion of the non-selected gene (Ye et al., 2003). Although these advancements in the development of antibiotic resistance gene (ARG)-free crops offer hope for their commercialization in the agricultural market, they still all have undesirable limitations, such as the requirement for additional culturing, low transformation frequency rates, and a lack of testing beyond model crops.
2.1.4 Regulatory sequences
In chloroplast transformation, genes can be expressed in operons that encompass entire metabolic pathways, with regulation occurring at the translational level (Stern et al., 1997). Understanding the interplay between multigene operons and modified regulatory elements is essential for advancing synthetic biology-enabled plastid engineering (Figure 2). There are several factors that should be considered, including:
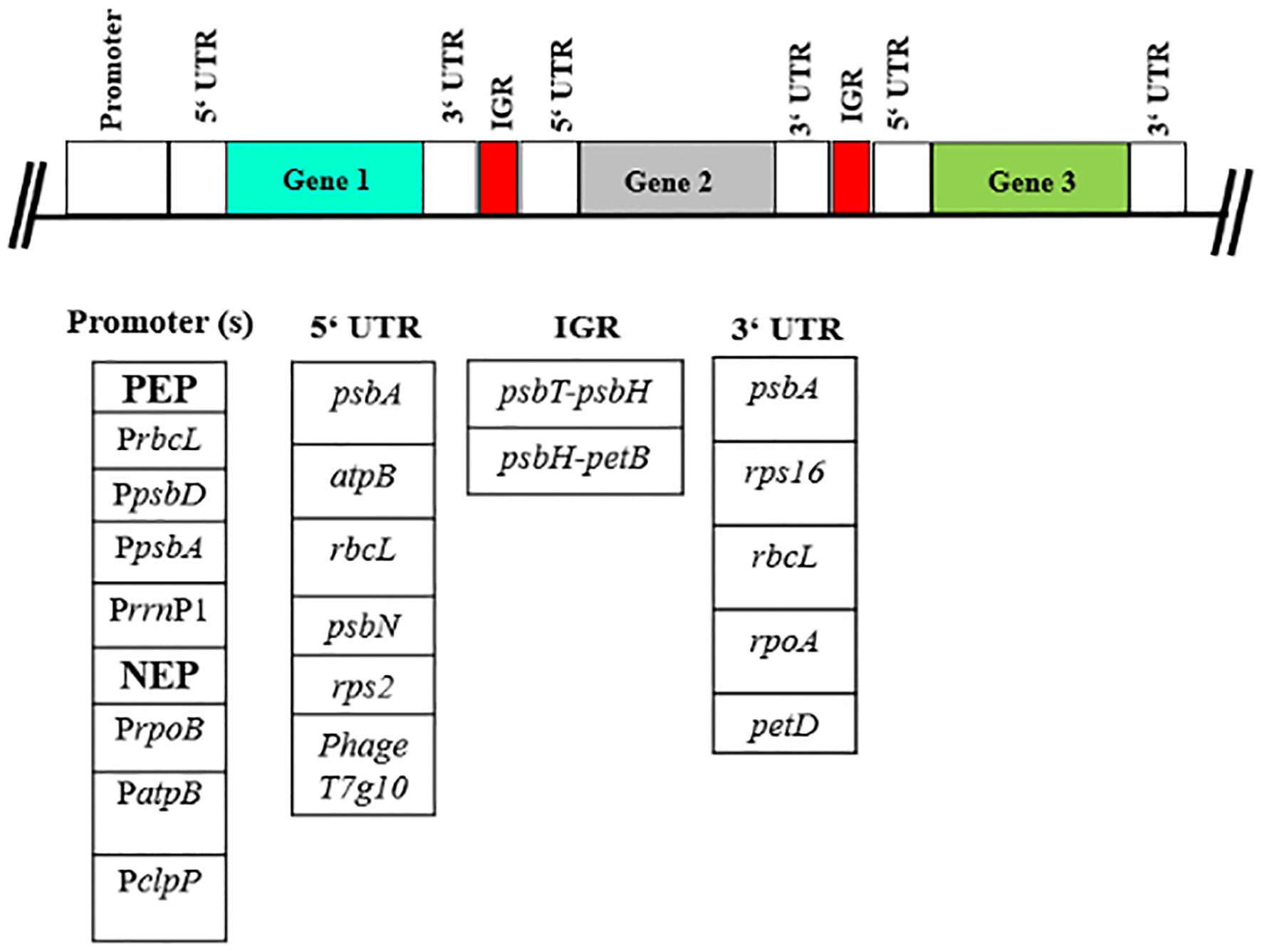
Figure 2. Segmental design of a synthetic plastid operon for plastid metabolic engineering. Schematic representation to show the variety of regulatory elements that can be employed for the construction of multigene operon. (IGR, intragenic plastid regions; UTR, untranslated Regions).
(a) PEP promoters
Most plastid-encoded RNA polymerase (PEP) promoters have coevolved with bacterial-type RNA (σ70) polymerases, which contain -35 (TTGACA) and -10 (TATAAT) consensus sequences as core motifs (Shiina et al., 2005) (Table 2). One of the best-studied examples of PEP promoters is one that ensures transcription of the psbA gene (PpsbA), which is developmentally activated by light in vivo. This promoter has an additional TATA box and a prokaryotic type TGn motif in between the -35 and -10 elements. In vitro characterization of the mustard and barley psbA promoter revealed that the presence of the -35 element was essential for enhanced transcription rates (Eisermann et al., 1990). However, studies in wheat showed that removal of the −35 consensus sequence does not affect transcription in mature chloroplasts if the TGn motif is present, but rather inhibits transcription in immature chloroplasts (Satoh et al., 1999). Hayashi et al. (2003) studied alterations at the −35 consensus sequence and found that these significantly decreased the transcription rate in tobacco. Another light-activated PEP promoter, PpsbD, has two additional regulatory elements: the AAG box and the PGT box, located upstream of the −35 consensus sequence (Thum et al., 2001). The transcription process was abolished when the AAG box was removed, even if the PGT box was retained. Likewise, alterations within the AAG box have reduced transcription by five times without affecting light inducibility. However, the removal of the PGT box did not affect the promoter’s response to light in initiating transcription (Allison and Maliga, 1995). The plastid rRNA operon promoter PrrnP1 is another commonly used PEP promoter for transgene expression in plastids. The essential role of the -35 promoter element in PrrnP1 was investigated through mutagenesis of the core promoter region (-37 to -8), which had a significant effect on transcription. Mutation of ACG significantly reduced the transcription rate to 8.19%, while alteration of TTG nearly abolished it (reducing transcription to 1.77%). However, mutations affecting the -10 promoter element (-16/-8 region) only moderately reduced in vitro transcription. Additionally, mutagenesis of the G-rich sequence (G-patch) between nucleotides -28 and -23 decreased transcription activity by 30% (Suzuki et al., 2003). With the application of improved bioinformatics algorithms, there is a need to mine additional functional plastid promoter motifs beyond those listed above. Experimental testing and identification of different PEP promoters will increase the availability of resources for developing new regulatory approaches in chloroplast engineering.
(b) NEP promoters
Nuclear-encoded polymerase (NEP) promoter activity has been identified at transcription initiation sites for several plastid genes in plants (Table 2). This activity is capable of driving transgene expression preferentially in non-green plastids, as found in fruits and tubers (Zhang et al., 2012; Valkov et al., 2011). The NEP promoters analyzed thus far are categorized into three types based on their sequence properties. Type-I promoters, characterized by a conserved YRTA-motif critical for promoter recognition, are located upstream of the transcription initiation site (+1) at -15 to +5 for PrpoB-345 and at -99 to +23 for PaccD-129 in tobacco (Liere and Maliga, 1999a, b; Kahlau and Bock, 2008). These fall under the Ia-type category. Another subset, the Ib-type, carries a second conserved sequence motif (ATAGAAATAGAA) at ~18 to 20 bp upstream of the YRTA-motif (PatpB-289 at -43 to +38 in tobacco) (Kapoor and Sugiura, 1999). The type-II NEP promoter PclpP-53 from tobacco, which lacks the YRTA-motif, has been characterized and found to be primarily located downstream of the transcription initiation site at –5 to +25 (Sriraman et al., 1998a). The most notable feature of this promoter, studied in tobacco roots and amyloplasts of potato, is its capacity to lead expression with higher mRNA accumulation in non-green plastids (Zhang et al., 2012; Valkov et al., 2011). Lastly, there is a non-YRTa-type (Pc) promoter that shows NEP transcription activity at rrn operon sequences. However, the sites relevant for transcription initiation from Pc have yet to be identified (Baeza et al., 1991). These findings may contribute to the future development of chloroplast expression systems, which are anticipated to address the ineffective expression of transgenes in non-green chloroplasts.
(c) 5’ Regulatory UTRs
The 5′ UTRs of plastid genes contain a conserved motif, such as the Shine–Dalgarno sequence or ribosomal-binding site (RBS) (AGGAGG), which regulates mRNA stability (Figure 2). The best-studied 5’ UTR is that of psbA (encoding D1 protein of PSII) from tobacco, which includes three conserved RBS sites, an AU-box, and a 5’ stem-loop structure. Removal of the first 17 bp in the 5’ stem-loop reduces mRNA levels by 50% and translation efficiency by 75% (Staub and Maliga, 1994b). The structure of the 5’ UTR of rbcL (encoding Rubisco large subunit) contains a single RBS site and two 5’ stem-loops, which are essential for increased mRNA stability. Mutations in the first 30 bp downstream of the translation initiation site reduce translation efficiency by 35-fold (Kuroda and Maliga, 2001b). The 5’ UTR of atpB (encoding ATP synthase β-subunit) lacks a conserved RBS sequence, and a mutation in the -25 to +18 region was shown to reduce translation by more than 20% (Hirose and Sugiura, 2004). Similarly, the psbN (encoding N-subunit of PSII) 5’ UTR, which also lacks a conserved RBS site, requires an unknown RNA-binding protein to initiate translation (Kuroda and Sugiura, 2014). The 5’ UTR of rps2 (encoding ribosomal protein S2) operates through an opposing mechanism, in which the binding of an RNA-binding protein limits translation efficiency by 13–20 fold (Plader and Sugiura, 2003). Finally, the Escherichia coli phage T7 gene (T7g10) and downstream box (DB) region are the most commonly used 5’ UTRs in plastid transgene expression. Protein expression levels have been shown to increase by up to 23% of the total soluble protein (TSP) when the expression is under the control of T7g10 (Kuroda and Maliga, 2001a). Various studies have suggested that the combination of various ribosome-binding sites and their manipulations can be used to design and test new synthetic plastid 5’ UTRs that regulate gene expression (Drechsel and Bock, 2011).
(d) 3’ Regulatory UTRs
The most common features of 3’ UTRs include transcription termination, mRNA transcript stability, and cleavage of polycistronic mRNAs into monocistronic protein-coding sequences by stem-loops (Monde et al., 2000) (Figure 2). The 3’ UTRs of the rbcL, rps16 (encoding ribosomal protein S16), and psbA genes are the most extensively used for plastid transgene expression. A study comparing the 3’ UTRs of psbA and rpl32 (encoding ribosomal protein L32) in tobacco have shown a fivefold difference in mRNA abundance (rpl32 > psbA) (Eibl et al., 1999). Similarly, a study comparing different 3’ UTRs, including those from plastid genes rbcL, psbA, petD, and rpoA, with the E. coli rrnB terminator, found that genes with the psbA and petD (encoding subunit IV of cyt b6f) 3’ UTRs exhibited two to three times higher transcript abundance compared to those with the rbcL and rpoA (encoding RNA polymerase α-subunit) 3’ UTRs (Tangphatsornruang et al., 2011). The examples above provide interesting insights, and future research may reveal more about the interplay between 3’ UTRs and transgenic plastid expression.
(e) Plastid intercistronic expression elements
The majority of chloroplast genes are expressed as polycistronic mRNAs. Primary transcripts in plastids are post-transcriptionally processed in the 5’-3’ direction into monocistronic or oligocistronic mRNAs through a complex series of maturation steps mediated by nuclear-encoded prokaryotic-type ribonucleases (Sakamoto et al., 1994). Stem-loop-like secondary structures within the 5’ and 3’ UTRs of plastid mRNA serve as recognition elements for RNA processing enzymes and act as protective elements against RNA degradation (Hayes et al., 1996; Stern and Gruissem, 1987). The transcripts from most plastid operons undergo essential intercistronic processing (RNA cleavage) to form monocistronic units, which in turn makes translation more efficient. One example is the psbE operon, which is transcribed as a single tetracistronic mRNA containing the psbE, psbF, psbL and psbJ genes for photosystem II (Carrillo et al., 1986; Willey and Gray, 1990). Stacking of multiple transgenes is often employed in chloroplast biotechnology and remains one of the unique attractions of chloroplast transformation technology, allowing the expression of multiple genes under a single promoter. IEEs, which are small sequence elements that mediate intercistronic cleavage (Zhou et al., 2007), are effective for stacking multiple foreign genes in operons and help to expand the range of applications for transplastomic technology. Two intercistronic cleavage sites were identified in the tobacco psbB operon of photosystem II, located at the central position of a putative RNA stem-loop secondary structure: psbT-psbH and psbH-petB (Figure 3). In vivo testing of these two elements, placed between two transgenes, revealed that only the complete stem-loop structure surrounding the psbT–psbH processing site conferred expression of the downstream cistron and thus acted as a functional IEE (Zhou et al., 2007). The IEE (50 bp) greatly simplifies the vector construction for co-expression of multiple genes and eliminates the need for individual gene promoters, reducing the risk of unusual recombination events and partial genomic deletions. The use of IEEs in tobacco and tomato chloroplasts has been evaluated in the construction of a synthetic operon for the expression of homogentisate phytyltransferase (SyHPT), tocopherol cyclase (SyTCY) and c-tocopherol methyltransferase (AtTMT), which are genes involved in the vitamin E (tocochromanol) biosynthesis pathway. This experiment resulted in a tenfold increase in the accumulation of transgene-derived foreign proteins compared to levels without IEEs (Lu et al., 2013). Another significant study in C. reinhardtii identified psbN-psbH and tscA-chlN as functional intercistronic regions and their role in the expression of foreign genes was identified (Macedo-Osorio et al., 2018).
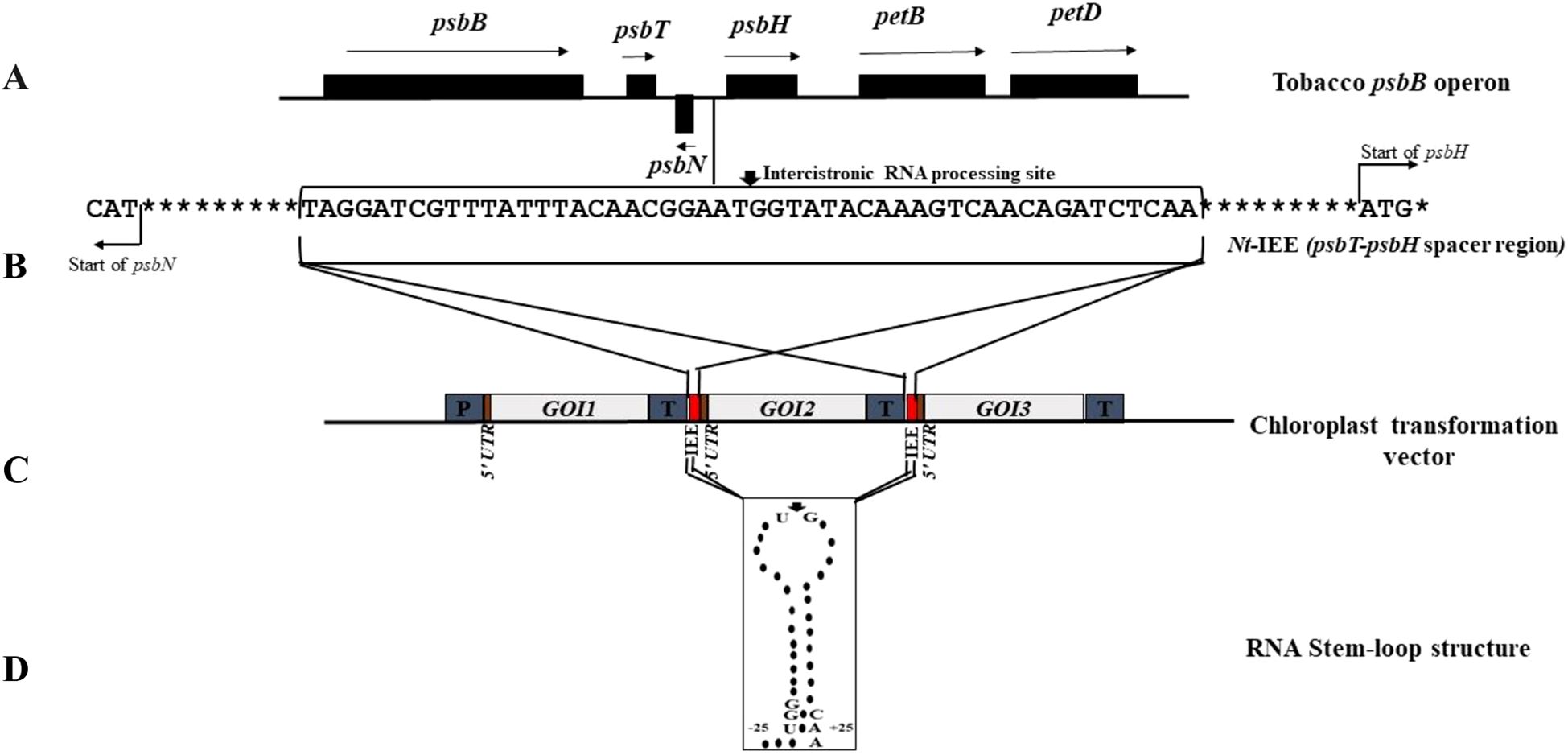
Figure 3. Intercistronic expression elements (IEEs) in the tobacco psbB operon. (A) Structure of the psbB operon with arrows above the genes indicating expression from left to right and arrow below the gene (psbN) indicating expression in the opposite direction. (B) Partial sequence of the psbT–psbH (Nt-IEE) (Zhou et al., 2007) spacer region from tobacco, used as a putative processing sequence in a plastid transformation vector. The position of the bold-headed arrow indicates the intercistronic RNA processing site (* indicates the next proceeding base). (C) A typical characteristic arrangement of IEEs inserted between the three cistrons (GOI: Gene of interest) to support plastid multicistron transgene expression. (D) Putative RNA stem–loop structure showing endonucleolytic cleavage site (at the bold headed arrow) for intercistronic processing of the operon.
(f) Pentatricopeptide repeat binding sites
PPR proteins are sequence-specific RNA-binding proteins involved in RNA maturation in plastids (Barkan et al., 2012). PPR proteins are of particular interest to chloroplast biotechnologists due to their potential for engineering the regulated expression of transgenes in chloroplasts. This regulatory mechanism involves the interaction between a nuclear-controlled variant of the PPR protein and a cognate binding site upstream of a chloroplast transgene, enabling its regulated expression. PPRs interact with RNA in a repeat-to-nucleotide binding mode via a superhelical RNA-binding surface that contains a lengthy stack of repeating motifs (Zhou et al., 2017) (Figure 4). Engineered PPR proteins can be generated by altering the PPR sequence or redesigning the protein based on the consensus PPR motif for custom RNA binding (Yan et al., 2019). An ethanol-inducible on/off switch for regulating chloroplast transgenes has been developed based on the characterized maize PPR10, which stimulates atpH expression by blocking 5′→3′ exoribonucleolytic degradation of mRNA (Rojas et al., 2019). This system has enhanced the translational efficiency of GFP and increased transgene expression in leaf tissues (Yu et al., 2019). Another PPR protein, chlororespiratory reduction 2 (CRR2), was employed to express vaccine-candidate genes against the human papillomavirus (HPV). Immunological studies showed that a post-transcriptional expression element (ndhB sequence) was helpful in expressing L1 proteins, though the yield was low (Legen et al., 2023). Overall, PPR protein-mediated strategies provide one of the few options available to chloroplast biotechnologists to regulate and optimize the expression of foreign genes (genes of interest) in chloroplasts.
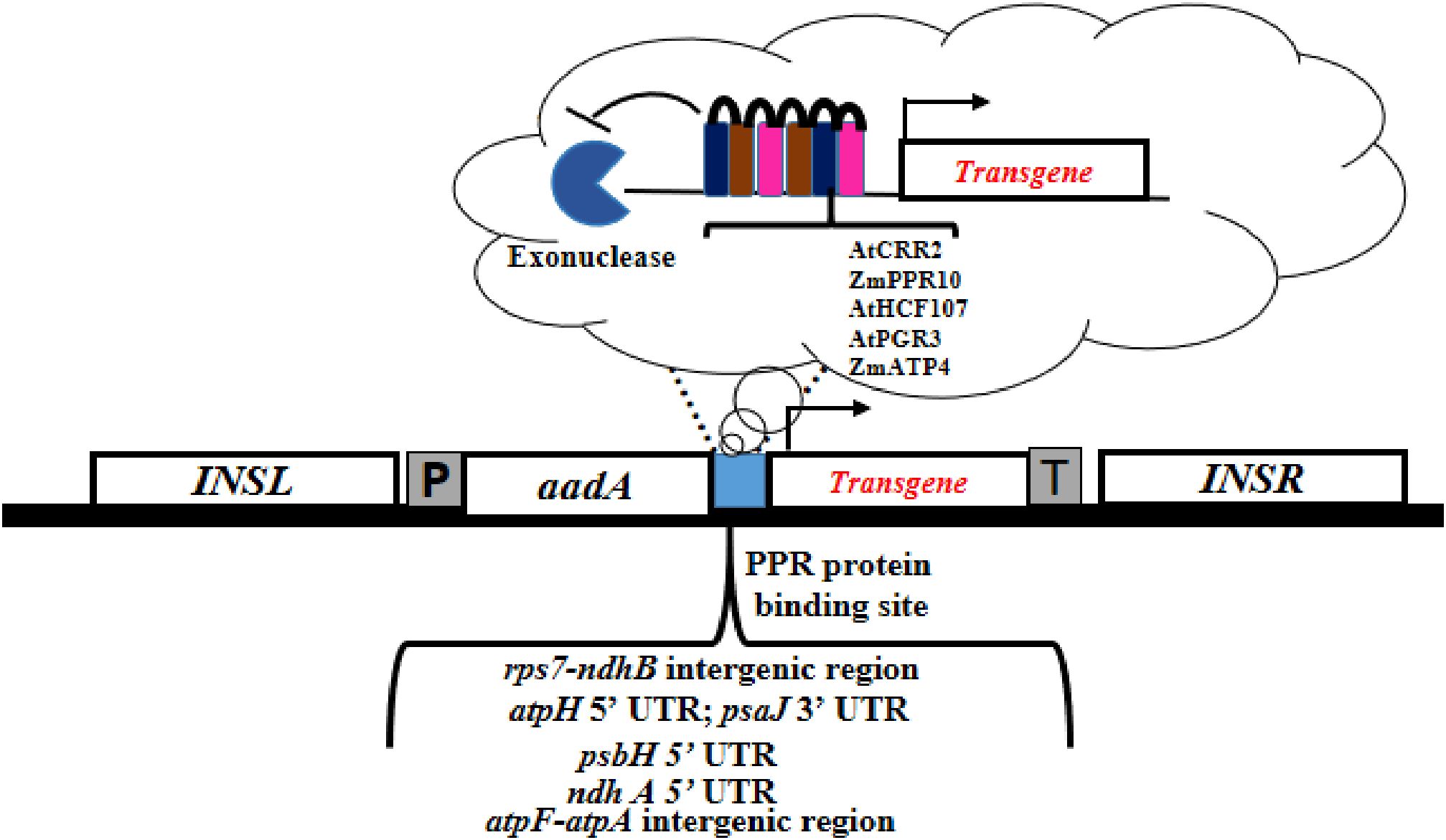
Figure 4. Experimental strategy for pentatricopeptide repeat (PPR) protein-mediated transgene expression in chloroplasts. Schematic overview of different PPR proteins and their binding sites. The incorporation of PPR protein binding sequences upstream of the transgene will enhance its expression by blocking exonuclease-mediated RNA degradation and increasing translational efficiency (in enlarged view).
(g) Elements for inducible expression
Overexpression of foreign proteins may result in severe mutant phenotypes. This can occur due to the complete depletion of gene expression capacity or interference of the recombinant protein(s) with chloroplast biogenesis. Pigment deficiencies and impaired photosynthetic activities typically result in negative effects during chloroplast transgene expression (Lössl et al., 2003; Hennig et al., 2007). Unfortunately, studies on endogenous mechanisms that control gene expression in chloroplasts have been limited. It is highly anticipated that an inducible transgene expression system will be developed to facilitate the accumulation of foreign proteins in response to an external stimulus. Nuclear control for regulating plastid transgene expression was initially investigated in tobacco. This approach uses a plastid-targeted T7 RNA polymerase, encoded in the nucleus, with its transcription regulated by ethanol-inducible nuclear promoters (Lössl et al., 2005) (Figure 5A). Two other inducible techniques that rely on nuclear control have been created. A hybrid transcription system was developed to utilize a eubacterial promoter, under which the transgene is positioned for expression. A chimeric transcription sigma factor that interacts with the PEP is required for this action (Buhot et al., 2006). The use of nuclear-encoded and plastid-targeted site-specific recombinase (Cre) for excision of an expression-blocking sequence that drives transgene expression has also been developed (Tungsuchat et al., 2006). These two methods involve the nuclear control for plastid transgene expression, which nullifies the advantage of abolishing transgene containment conferred by maternal inheritance—one of the most striking features of plastid engineering technology (Barkan and Goldschmidt-Clermont, 2000).
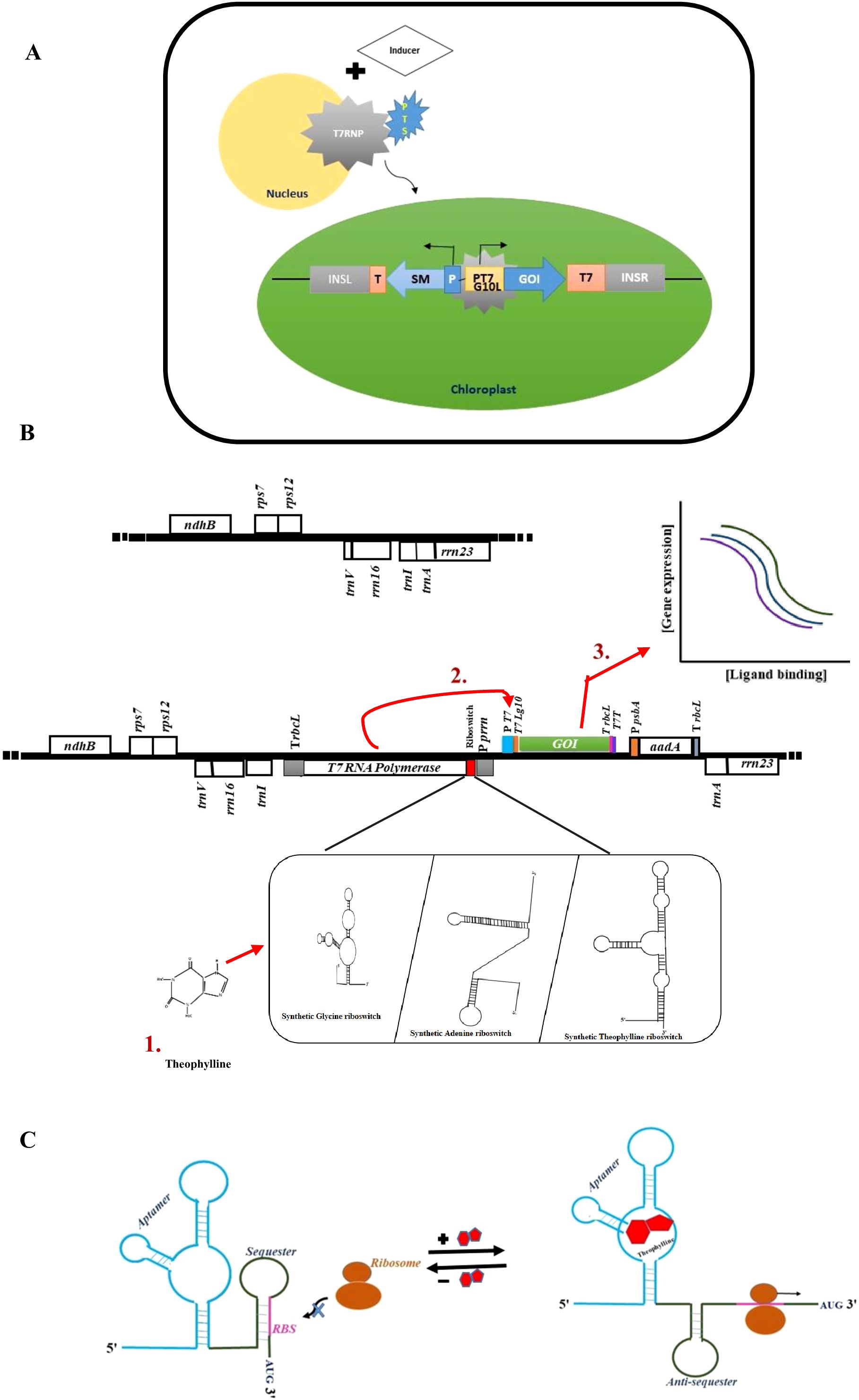
Figure 5. Different approaches for inducible transgene expression in plastids. (A) A nuclear gene- encoded T7 RNAP (RNA polymerase) fused to a plastid targeting sequence (PTS) for trans-activation in plastids expressed under control of an inducible promoter. The application of the inducer leads to the import of T7 RNAP into the chloroplasts, activating the transcription of plastid transgenes. (B) RNA amplification-enhanced riboswitch (RAmpER) mediates inducible transgene expression in the genome. A representative physical map of the plastid transformation vector showing the induction by theophylline (1,3-dimethylxanthine) (step 1), which activates the T7 RNA polymerase gene in the presence of a translational riboswitch (step 2). This is sufficient to trigger immense transcription of the gene of interest (GOI), driven by the T7 promoter (step 3). (C) In the absence of theophylline, translational control of gene expression by the synthetic theophylline-responsive riboswitch is facilitated by a folded structure that sequesters the ribosome binding site (RBS) in the mRNA transcript. In the presence of theophylline, the riboswitch adopts a conformational change at the aptamer, promoting the translation mechanism by releasing the RBS.
The development of plastid-only inducible systems is highly desirable for the direct regulation of all components from the chloroplast genome. Two such systems have been reported: an external control system for plastid gene expression that responds to chemical induction by isopropyl-β-D-thiogalactopyranoside (IPTG), which regulates transcription via a modified plastid promoter containing a LacI-binding site from E.coli (Mühlbauer and Koop, 2005), as well as the development of riboswitches, which are regulatory ribonucleic acid sequences (cis-acting mRNA elements) that bind to ligands and directly affect gene expression by forming alternative structures. Characterization of synthetic riboswitches for inducible gene expression has shown that their function can be altered by sequence modification for translational regulation, as plastids do not have effective transcription termination mechanisms. The successful design and evaluation of different synthetic chloroplast riboswitches from E.coli [e.g., thiamine pyrophosphate (TPP) and synthetic theophylline (theo) riboswitches] were reported (Verhounig et al., 2010). In addition, two other transcriptional “on” switches [the glycine (gly) and the adenine (ade) switches] from B. subtilis with modified Shine-Dalgarno (SD) or anti-Shine-Dalgarno (anti-SD) structures were developed to facilitate translational regulation (Verhounig et al., 2010). A synthetic theophylline switch (s.theo-RS) was also functionally verified for its exceptional gene regulation ability in tobacco chloroplasts (Verhounig et al., 2010). The challenge of relatively low transcription levels with riboswitches was addressed by adding a bacteriophage T7 RNA polymerase-mediated RNA amplification step, resulting in a new RNA amplification-enhanced riboswitch (RAmpER) (Figure 5B) (Emadpour et al., 2015). Riboswitches, mostly located in the 5’ UTR, regulate gene expression by either directing the switching sequence to form a short stem-loop structure that aborts transcription, or by exposing/concealing the Shine-Dalgarno sequence to control translation. In some eukaryotic mRNAs, the TPP riboswitch also regulates 3’ end splicing (Barrick et al., 2004) (Figure 5C). Improved inducible expression systems offer expanded possibilities regarding the application of transplastomic technology. Recently, RAmpER was successfully employed in the regulation of astaxanthin synthesis (Agrawal et al., 2022) and antimicrobial peptides (AMPs) (Hoelscher et al., 2022) in tobacco.
2.1.5 In vivo vector assembly
The development of a transformation vector that targets the trnfM and trnG genes of the tobacco chloroplast genome using in vivo E. coli cloning (iVEC) technology is another significant advance in chloroplast engineering. Wu et al. (2017) developed a simple and seamless method to construct a complete plastid transformation vector in a single step with five DNA fragments. PCR products comprising four insert DNA fragments and a linearized vector, all with homologous short sequences at both ends, are co-transformed into competent E. coli cells. The homologous recombination events in E. coli allow the inserts and vector backbone to recombine in vivo, generating a functional plastid transformation vector. The iVEC-assembled transformation vector has been used to generate transplastomic plants, which exhibit foreign protein accumulation levels of 9% of total soluble protein. Recently, modular cloning has taken advantage of type-IIS restriction endonucleases that cut dsDNA at a specified distance away from the recognition sequence, creating custom overhangs that are efficiently involved in the assembly of genetic elements in a one-step reaction. The Golden Braid (GB) modular cloning procedure has been described to design and assemble DNA fragments (promoters, UTRs, and multigene operons) in plastid transformation vectors. This led to the establishment of a plastid expression vector for the formation of griffithsin using a set of modules that facilitate transformation in tobacco (Vafaee et al., 2014). Occhialini et al. (2019) developed the fast and flexible chloroplast engineering toolkit “MoChlo” by assembling synthetic operons of various sizes and determined the efficiency of assembly. This library consists of 128 standardized chloroplast-specific parts, which have been used successfully to generate chloroplast transformation vectors containing up to seven transcriptional synthetic operons in a single vector (10.6-kb). The main objective of establishing the modular cloning kits is to create a repository of reusable genetic components necessary for engaging chloroplast engineering.
2.2 Chloroplast transformation methods
2.2.1 Classical gene delivery methods into plastids
The two most common methods for delivering genes into plastids are the polyethylene glycol (PEG)-mediated method and particle bombardment. The less commonly used approach for gene delivery into the plastome involves PEG-mediated transformation, which requires enzymatic removal of the cell wall to obtain protoplasts, followed by exposing the protoplasts to purified DNA in the presence of PEG (Koop et al., 1996; O'Neill et al., 1993). However, the lack of effective protoplast regeneration protocols for producing plastid-transformed commercial crops presents a challenge for this methodology, resulting in a reduced success rate. Biolistic technology has facilitated the direct delivery of foreign DNA directly into the plastome by crossing the physical barrier imposed by the double membrane of the chloroplast envelope (Sanford, 1990). Particle bombardment, the method of choice for engineering various crops, remains extremely versatile in facilitating the generation of transplastomic lines. This is accomplished by delivering plastid transformation DNA constructs into plant cells by linking the genetic material to microsized metal particles (usually gold or tungsten), followed by high-velocity deployment to explants (Klein et al., 1987; Kohli et al., 2001) (Figure 1B). A wide variety of cell types can be targeted efficiently for foreign DNA delivery, including leaf cuttings (Svab et al., 1990), calli (Wang et al., 2018), hypocotyls (Narra et al., 2018), and root explants (Ruf et al., 2019) (Table 1). The frequency with which transformed plants can be recovered after bombardment depends on the efficiency of regeneration of explants under selective antibiotic culture conditions (Altpeter et al., 2005). One of the major advantages of this method is that it does not rely on biological constraints (cellular and physiological factors that limit DNA uptake and stable integration) or host limitations (regeneration and species-specific transformation barriers) (Christou et al., 1991). Particle bombardment uses a minimal DNA expression cassette flanked by homologous chloroplast sequences to coat the metal particles and carry out the transformation (Fu et al., 2000). The major obstacle in engineering the plastid genome is the long process of selection for generating homoplasmic transplastomic plants. This is due to the presence of 10–100 plastids, each with about 100 copies of the plastid genome, in one cell, which makes it difficult to achieve a homoplastomic state in a single attempt (Ho and Cummins, 2005) (Figure 1C).
2.2.2 Chloroplast transformation by Agrobacterium tumefaciens
A few attempts have been made to optimize Agrobacterium-mediated transformation to efficiently introduce the Ti-plasmid carrying the genes into chloroplasts (Table 3). Initial attempts at Agrobacterium mediated transformation in chloroplasts resulted in chloramphenicol-resistant tobacco plants after cocultivation. Although these results were consistent with the delivery of the cat gene encoding chloramphenicol acetyltransferase contained in the plastid transformation vector, the process was hindered by difficulties in obtaining stable transgenic lines (De Block et al., 1985). This may be due to a lack of flanking chloroplast targeting sequences in the vector, which are necessary for the integration and maintenance of foreign DNA sequences. In a different study, the T-DNA segment of a binary vector comprised of 5S rRNA and a tRNA was used to generate kanamycin-resistant clones. Chloroplast transformation events were characterized by single crossover events, which resulted in unstable cointegrate structures (Venkateswarlu and Nazar, 1991). Neither of these two experiments provided a reproducible methodology for chloroplast engineering, despite the unexpected expression of marker genes. Matsuoka and Maliga (2021) made a successful attempt to re-engineer the virulence proteins VirD2 and VirE2 for targeted T-DNA delivery into tobacco chloroplasts. The authors provided the evidence for the direct export of the phiC31 phage site-specific integrase (Int) protein fused with the Rubisco small subunit transit peptide (TP) at the N-terminus and the VirF (F) T4SS signal at the C-terminus.
2.2.3 Nanoparticle-based plastid engineering
Novel options for improving chloroplast biotechnology are being opened up by developments in nanotechnology. Through the penetration of plant cell walls, cell membranes, and organellar double envelopes, nanomaterials provide a viable means of delivering transgenes (Ganesan et al., 2018; Newkirk et al., 2021) (Table 3). The general influence of nanoparticle properties such as size and coating on their uptake and transport processes has been investigated successfully (Avellan et al., 2019). The hydrophilic quantum dot (QD) with β-cyclodextrin molecular baskets conjugated with targeting peptides (RbcS: Rubisco small subunit 1A) allowed the targeted delivery of biochemical cargoes into Arabidopsis chloroplasts (Santana et al., 2020). Nanoparticles can be functionalized with biodegradable polysaccharides such as chitosan to form DNA-nanoparticle complexes that are capable of electrostatic cellular import and chloroplast delivery. A notable example of DNA-chitosan complex for chloroplast transformation was studied in Eruca sativa, Nasturtium officinale, Nicotiana tabacum, and Spinacia oleracea chloroplasts for transient expression of yellow fluorescent protein (YFP) through chitosan-wrapped single-walled carbon nanotubes (CS-SWNTs) (Kwak et al., 2019). In this study, positively charged CS-SWNTs were complexed with negatively charged plasmid DNA (pDNA), forming a net positive nanocomplex that migrates into chloroplasts. Once in the chloroplast, the pDNA is thought to disassemble from the CS-SWNTs in the weakly alkaline environment (pH ~8), resulting in a transformation frequency of approximately 88%. This success demonstrates that nanotechnology holds great promise as a platform for chloroplast transformation and should be further optimized for applying this technology to other economically important crop species.
2.2.4 Clustered cell-penetrating and chloroplast-targeting peptides mediated plastid transformation
Efficient peptide-mediated pDNA delivery to plastids can be achieved through proper linkage to cell-penetrating peptides (CPP) and chloroplast-targeting peptides (CTP) (Table 3). A transient plastid transformation technique has been established in leaves of A. thaliana and N. benthamiana and in mature green tomato fruits, using formulated pDNA/CTP/CPP complexes with similar efficiencies (Thagun et al., 2019). Electrostatic interaction at optimal amine/phosphate (N/P) ratios between pDNA and cationic CTP (KH9-OEP34) enables the condensation of pDNA and its effective delivery to plastids. Decreased transformation efficiency has been noted at higher N/P ratios, which may be due to the slow dissociation of pDNA/CTP complexes once they are delivered into plastids. In contrast, lower N/P ratios may cause destabilization and premature release of bound pDNA before reaching the target (Thagun et al., 2019). The addition of BP100 (CPP), a positively charged, membrane-binding agent, to pDNA/CTP complexes resulted in enhanced plastid transfection efficiency. Oikawa et al. (2021) developed a simple infiltration method to introduce a complex solution into intact plant cells for studying plastid-localized transgene expression. Thagun et al. (2022) introduced a high-throughput peptide-mediated foreign DNA delivery platform by spraying the leaf surface. The translocation of the CPP/CTP/pDNA complex is thought to be controlled by a stomata-dependent uptake mechanism. Odahara et al. (2022) recently studied peptide-mediated plastid transformation in tobacco, rice, and kenaf to transport foreign DNA into plastids. A fusion peptide (KH-AtOEP34) was generated by combining a polycationic DNA-binding peptide (KH) and a plastid-localizing signal of outer envelope membrane protein-OEP34/TOC34 (AtOEP34) to integrate a foreign DNA construct into the chloroplast through homologous recombination. The resultant transformants were selected for resistance to spectinomycin/streptomycin and were found to be fertile. The integrated foreign genes were successfully transmitted to the T1 generation. Another study characterized the route and tracked the movement of DNA/CTP/CPP complexes into chloroplasts of Arabidopsis thaliana (Oikawa et al., 2021). Gene delivery from the extracellular space to the chloroplast stroma was detected through fluorescent labeling and confocal laser scanning microscopy (CLSM), which occurred 6hrs after infiltration. Based on these successful attempts, peptide-mediated plastid transformation could serve as a robust transformation method in economically important agricultural plant systems.
2.2.5 Episomal plastid engineering
Over three decades of progress in plastid engineering, the effective integration of transgene constructs into the plastome has relied on the mechanism of homologous recombination (HR). Now, in the age of synthetic biology, chloroplast biotechnology provides a dynamic platform for the one-step introduction of metabolic pathways in plants. However, the ultimate goal is to create marker-free transplastomic lines by choosing an alternative to the HR mode of chloroplast engineering. Episomally replicating synthetic plasmids may provide an interesting platform, with the ability to express transgenes in plastids without integrating vector sequences into the native plastid genome (Staub and Maliga, 1994) (Table 3). Persistence across multiple generations even when the selection pressure is removed is a key determinant for the commercial application of episomal plastid engineering. In this context, the design of the mini-synplastome, a novel synthetic episomal vector engineered using NICE1 (Nicotiana plastid extrachromosomal element) (Staub and Maliga, 1994a) and dinoflagellate ori (origin of replication) (Min et al., 2015), has been pursued to explore possible methods for plastid transformation in potato plants without disturbing the native plastid genome (Occhialini et al., 2021). Shuttle vectors, developed in this study by inserting a selection and visible reporter gene cassette (aadA and smGFP) that was flanked by the vector backbone instead of homology arms, were shown to be capable of replication in E. coli and able to generate transplastomic plants. This work also demonstrated the possibility of designing an episomal construct in which the desired transgenes are incorporated through HR, while the selection process is carried out from a non-integrating region of the episome, leading to homoplasmy (Figure 6A). The selective pressure could be removed, enabling the gradual elimination of the episomal vector, resulting in marker-free transplastomic lines. A new method for transgene expression in chloroplasts has been developed using a ‘minichromosome’, a physically independent and amplifying entity. This method offers an alternate strategy for effective foreign gene expression in plastids by utilizing the replication mechanism of gemini viruses. The whole process relies on the helper protein ‘Rep’ (replication initiator protein), which mediates the amplification of transgene DNA by initiating replication via recognition of specific ‘VOR’ (viral origin of replication) sequences flanking it (Figure 6B). Further studies are needed to analyze the impact of minichromosome-induced phenotypes on progeny (Jakubiec et al., 2021).
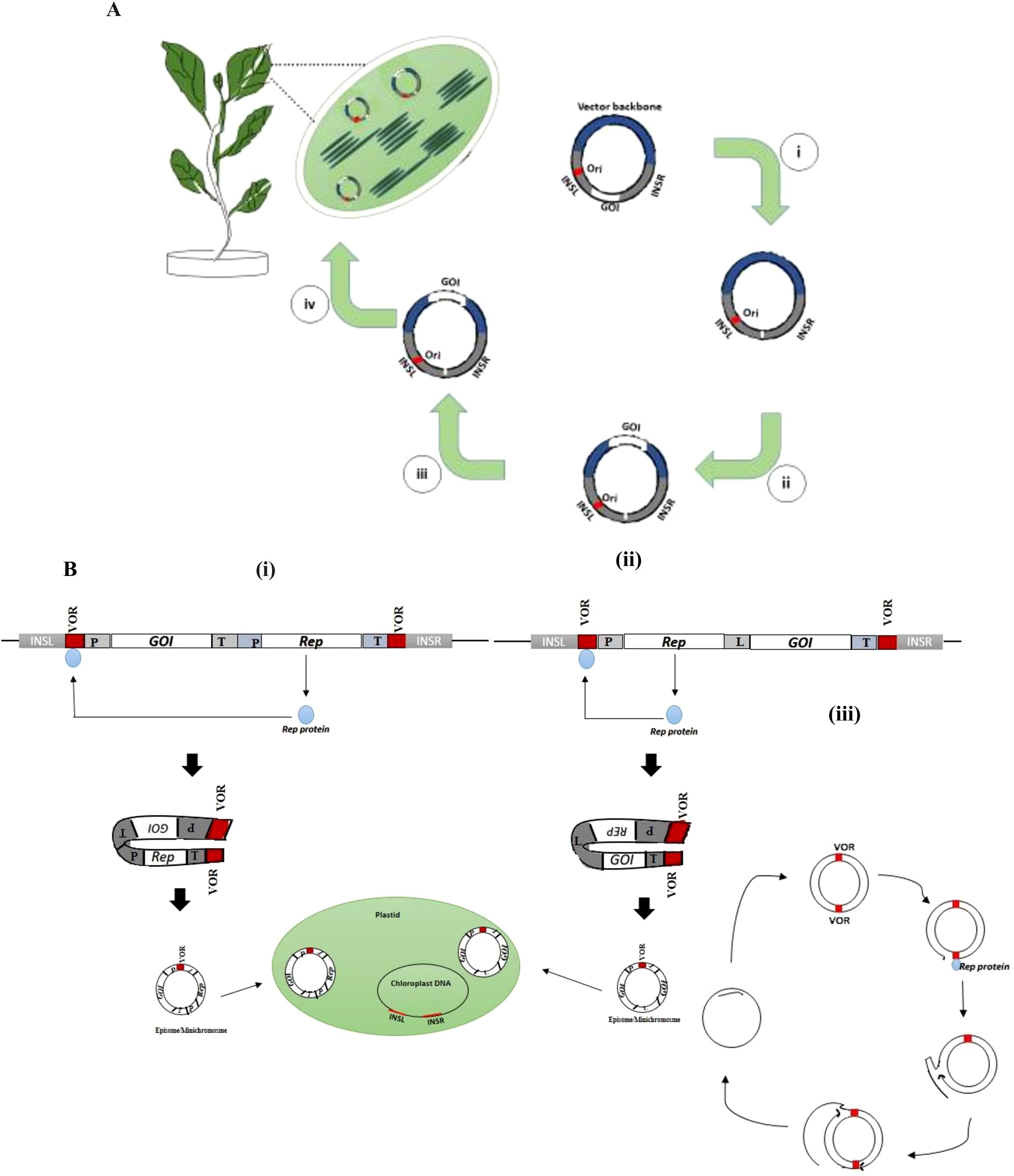
Figure 6. Episomal vector system for plastid transformation. (A) Plastid genetic engineering using a non-integrating episomal synthetic plastid vector, i.e., mini-synplastome (Occhialini et al., 2021). (i) A chloroplast-specific integration vector is used for transformation and selection of transplastomic lines without vector integration; (ii) Screening and isolation of plasmids from E. coli by back-transformation with total genomic DNA from transformed lines containing episomes; (iii) Construction of synthetic plastome with the gene of interest (GOI) for transforming chloroplasts; (iv) Mini-synplastomes as independent replicating molecule carrying the GOI. Insertional sequence left (INSL), insertional sequence right (INSR), homologous arms (Ash grey), the viral origin of replication (VOR), Ori (red), vector backbone (Blue), and the GOI (white) are indicated in the plasmids. (B) A design illustrating the episome replicating in the transplastomic plant generated using the episomal vector. (i, ii) Plastid-targeting vectors with the VOR, replication initiator protein (Rep), and GOI with flanking sequences, allows the target transgene insertion into chloroplast without integrating into native plastid genome. (iii) Mechanism of Rep-mediated recognition of a specific VOR sequence which initiates a specific nick to generate a ssDNA that can be converted to circular dsDNA.
2.2.6 Horizontal gene transfer
Horizontal gene transfer (HGT), the process of exchanging genetic material from donor to recipient by asexual means, is a plausible mechanism between sexually incompatible species. Genetic experiments have revealed that the simple method of grafting allows the introduction of entire organelles (mitochondria and chloroplasts) and/or their genomes to cross non-transformable species barriers (Stegemann et al., 2012) (Table 3). This is achieved by cell wall thinning and de novo formation of plasmodesmatal connections at the graft union of stock and scion cells. The resulting outcome of horizontal transfer is different in chloroplasts, which do not normally undergo recombination, compared to plant mitochondria, which frequently recombine to form mosaic genomes (Thyssen et al., 2012; Stegemann et al., 2012). The grafting procedure, which facilitates the horizontal transfer of the plastid genome across the graft junction between Nicotiana tabacum and nicotine-free tree species Nicotiana glauca, led to the recovery of homoplasmic transplastomic N. glauca at a higher frequency (Lu et al., 2017). Several events have been reported in which HGT occurs through the cell-to-cell transfer of entire organelles with encapsulated genomes (Hertle et al., 2021). Overall, the HGT method is effective for transforming plastids from neighboring cells, enabling chloroplast transformation in species with low efficiencies, and thereby facilitating biotechnological applications.
2.2.7 Plastid transformation with fragmented DNA
Construction of plastid transformation vectors has become cumbersome for the expression of multiple genes, despite the advances of new application tools. A low-cost, cloning-free method will be one of the best choices for chloroplast genome transformation with a single or few fragments (Table 3). One such system has been validated using multiple linear DNA fragments (Ren et al., 2022). This strategy relies on homologous recombination between DNA fragments that share homologous sequences (HS) at their ends, mediated by the endogenous plastid recombination machinery. The fragments that are delivered through particle bombardment are integrated into the plastid genome. The results have shown homologous sequences as short as 200 bp are sufficient for proper integration, and this method has been successfully employed to introduce a phage lysin-encoding gene (plyGBS) and a long operon with AtVDE (encoding violaxanthin de-epoxidase in Arabidopsis thaliana) and AtZEP (encoding zeaxanthin epoxidase in Arabidopsis thaliana) into the tobacco plastid genome. However, for the construction of synthetic pathways involving transformation and expression of multiple genes, it is important to consider that longer stretches of homologous sequences at fragment ends can have a positive effect on the efficiency of fragment insertions (Ren et al., 2022).
3 Advanced techniques in plastid genome manipulation and engineering
3.1 Plastid genome-targeted base editing
Plant nuclear genome editing has revolutionized crop improvement by enabling genetic changes without the introduction of foreign genes. However, despite notable advancements in nuclear genome editing, only a few recent studies have led to significant breakthroughs in the genome editing of plant organelles (Kang et al., 2021). Targeted base substitutions are thought to be a superior way to make desired single nucleotide polymorphisms (SNPs) without disturbing other genes in the plastid genomes of land plants. To date, methods for C-to-T and A-to-G base editing have been reported. Double-stranded DNA deaminase toxin A (DddA)-derived cytosine base editors (DdCBEs) have been developed for organellar DNA C-to-T base editing in A. thaliana (Nakazato et al., 2021, 2022), lettuce, and rapeseed (Kang et al., 2021). These CRISPR-free, protein-only base editors are fused to two halves of the DddA active domain at the C-terminus, allowing them to recognize and come together on adjacent binding sites of the target DNA. The spacer sequence separating the two TALE (Transcription activator-like effector domains)-binding sequences are edited at respective positions (Figure 7A). To target the chloroplast genome, suitable chloroplast transit peptide (CTP) sequences were added to the N-terminus of the TALE monomers. To enhance mutation frequency, uracil-DNA glycosylase is inhibited by attaching a copy of uracil DNA glycosidase inhibitor (UGI) to the C-terminus of the DdCBEs, preventing the excision of uracil from the target DNA (Tan et al., 2022) (Figure 7B). The Golden Gate assembly system was used to construct custom-designed DdCBEs encoding plasmids to target 16S rRNA, psbA, psbB, atp6, and rps14 genes in chloroplast DNA and atp6 and rps14 genes in mitochondrial DNA. This led to high frequencies of C-to-T conversions in lettuce and rapeseed protoplasts by PEG-mediated transfection. Editing events were observed in calli derived from transfected protoplasts with frequencies of up to 38% (Kang et al., 2021). Recent studies have reported the establishment of homoplasmic (100% edited) lines in the T2 generation of A. thaliana, altered with C-to-T transition after the elimination of the introduced DdCBE transgene from the nucleus by Mendelian segregation. Some off-target editing events were observed near the targeted sites or far away in the plastid genome (Nakazato et al., 2021). Nakazato et al. (2021) referred to their use of plastid-targeted DdCBE as ptpTALECD. A study was conducted to identify favored positions of substituted cytosines by ptpTALECD (Nakazato et al., 2023). In this study, ptpTALECD_v2, which employed a modified version of DddA (DddA11’), could homoplasmically edit cytosines on the 3’ side of G and C, some of which were not homoplasmically edited by DdCBEs including ptpTALECD (Nakazato et al., 2023). C-to-T base editing in the plastid genome of rice was successfully achieved by introducing stop codons into the psaA gene of photosystem I, resulting in non-photosynthetic mutants that were unable to produce seeds (Li et al., 2021) (Figure 7C). Another group used a similar strategy to introduce a premature stop codon into the rice psaA gene, achieving near-homoplasmy (Zhang et al., 2024). A variant with high base-editing activity (DddA11), developed through an in vitro evolution system (Mok et al., 2022a), was adopted instead of DddA. Wang et al., 2024 developed a monomeric C-to-T base editor for rice plastid DNA. This base editor contains CTP, TALE, a cytidine deaminase for single-stranded DNA (ssDNA) [hA3A-Y130F (Ren et al., 2021b) or TADC (Lam et al., 2023)], catalytically-inactivated DddA (DddAE1347A), and UGI in a tandem array. According to Cho et al (Cho et al., 2022), DddAE1347A likely unwinds dsDNA, enabling the deamination of target Cs by the cytidine deaminase for ssDNA. This monomeric base editor targeted multiple Cs within and adjacent to the TALE-binding sequence with frequencies of up to 81%. Both groups integrated the transgene of the base editors into the rice nucleus via Agrobacterium-mediated transformation (Li et al., 2021; Zhang et al., 2024; Wang et al., 2024). Additionally, a transiently expressed TALE-FokI nickase, a cytidine deaminase for ssDNA, and an exonuclease, each fused with CTP, were employed to preferentially edit Cs on one of two DNA strands in plastids of rice protoplasts, with frequencies of up to 1.67% (Hu et al., 2024). The dimeric TALE-FokI nickase introduced a nick in between the TALE binding sequences, and the exonuclease subsequently removed nucleotides around the nick, generating ssDNA, which was then deaminated by the ssDNA-specific cytidine deaminase.
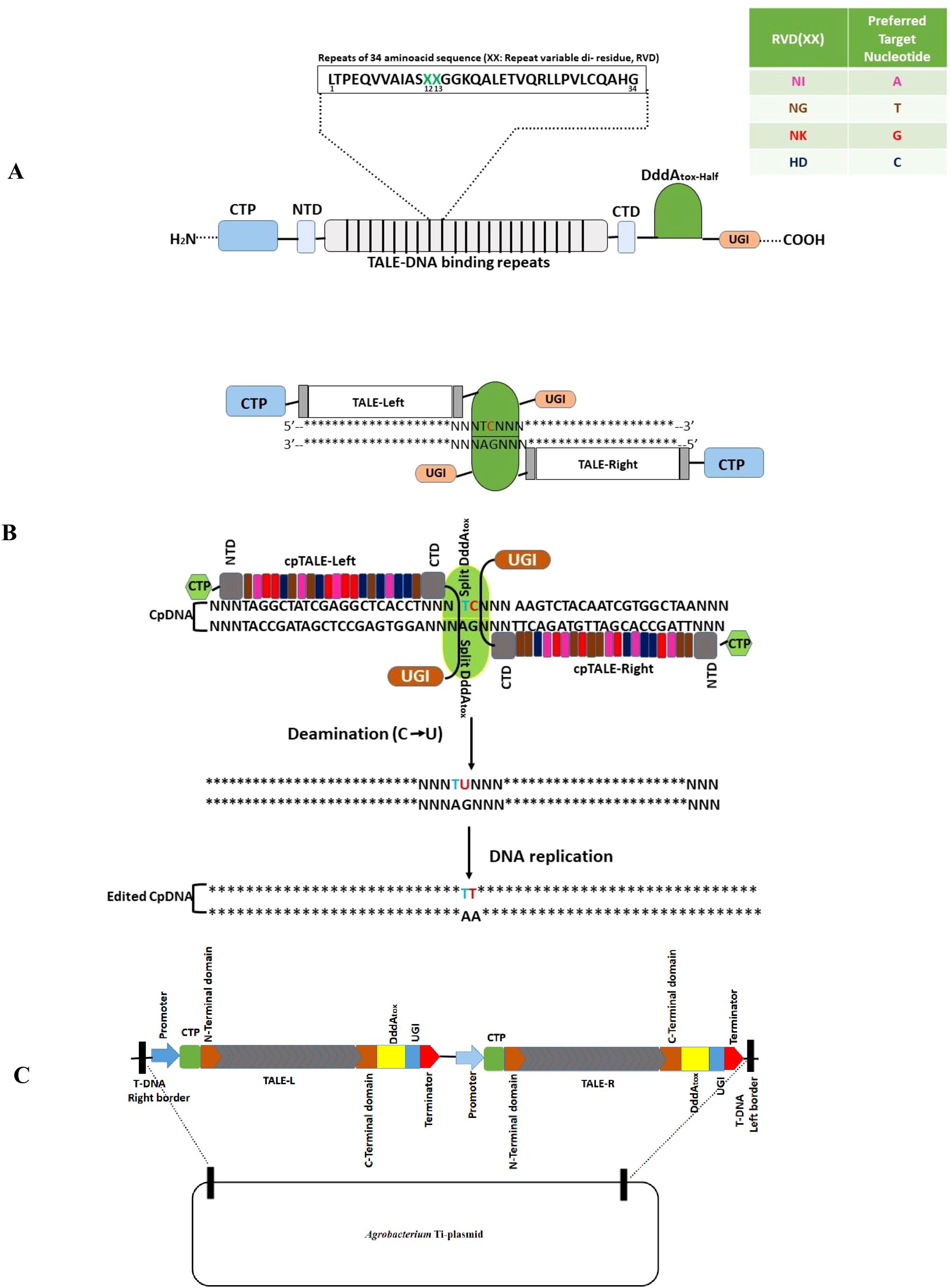
Figure 7. Mechanism of double-stranded DNA deaminase toxin A (DddA)-derived cytosine base editor (DdCBE) mediated chloroplast genome editing. (A) Structure of DNA binding repeats (TALE-array), with repeating units of 34 amino acids that each bind to one nucleotide. These units differ from one another at the 12th and 13th positions, the so-called repeat variable di-residues (RVD) which target a specific nucleotide (listed at the right) in the target DNA. The DdCBE repeating units normally vary in number up to 20. The N-terminal repeat unit of the TALE array protein binds the 5′ nucleotide of the target site (usually T will always be the first nucleotide). NTD and CTD are N-and C-terminal domains, respectively, flanking the DNA-binding TALE-array. A presequence (Chloroplast transit peptide, CTP) is added to the N-terminus for transporting the target proteins to chloroplast. The N- or the C-terminal part of DddAtox is fused to the C-terminus of the protein array. The complete complex binds to target DNA in a tail-to-tail manner, leaving a spacer region of approximately 14 nucleotides length where deamination reaction occurs, inducing a C-to-T conversion. (B) Mechanism of chloroplast DNA (CpDNA) editing involves the reconstitution of two halves of inactive DddAtox proteins in the chloroplast matrix to form active DddA cytidine deaminase base editor (DdCBE), connected with an uracil-DNA glycosylase inhibitory protein (UGI) (Asterisks* correspond to nucleotides). (C) Schematic representation of the tandem expression vector for Agrobacterium-mediated transformation.
A-to-G base editors are now available for plastid DNA in A. thaliana (Mok et al., 2022b; Zhou et al., 2024; Mok et al., 2024), lettuce (Mok et al., 2022b), and rice (Zhang and Boch, 2023; Wang et al., 2024). These editors are based on those used for human mitochondrial DNA A-to-G base editing (Cho et al., 2022). One molecule of the dimeric A-to-G base editors named TALED (Mok et al., 2022b) and pTABE_v6 (Zhang and Boch, 2023) contains CTP, TALE, and the C-terminal half of DddA or its variant (DddA6). The other molecule contains CTP, TALE, the N-terminal half of DddA or DddA6, and an adenine deaminase for ssDNA (TadA8e). Specific adenines in between TALE-binding sequences were edited (nearly) homoplasmically in A. thaliana (Mok et al., 2022b) and rice (Zhang and Boch, 2023). These homoplasmic mutations in A. thaliana were inherited by the next generation (T2 plants). In addition, some of the T2 plants did not carry the nuclear transgene (so-called null segregants) and had no off-target mutations (Mok et al., 2022b). Transiently expressed TALED edited its target adenines in lettuce protoplasts with frequencies of up to 46% (Mok et al., 2022b). Mok et al. (2024) developed UDG-TALED by fusing uracil DNA glycosylase (UDG) to DddA, which removes uracil, a product of C-to-T editing. The UDG-TALED system showed similar efficiency to TALED in editing A:T pairs in lettuce and A. thaliana, but with significantly reduced C-to-T edits (Mok et al., 2024). Monomeric A-to-G base editors named mTALEAD (Zhou et al., 2024) or mTCABE-T (Wang et al., 2024) employed CTP, TALE, TadA8e (in mTALEAD) or a TadA variant TADAC (in mTCABE-T), and DddAE1347A. Similarly to TALED, mTALEAD homoplasmically edited adenines around the TALE-binding sequence, and these homoplasmic base-edits were inherited by null-segregant T2 plants, which did not have off-target mutations (Zhou et al., 2024). The editor mTCABE targets both multiple cytosines and adenines around the TALE-binding sequence in rice with frequencies of up to 86% and 61.8%, respectively (Wang et al., 2024), due to TADAC’s ability to deaminate both cytosine and adenine (Lam et al., 2023). Plastid genome base editing recently conferred resistance to the herbicides atrazine and metribuzin in A. thaliana (Mok et al., 2024; Nakazato et al., 2024). Atrazine resistance was achieved by introducing the S264G mutation in the D1 protein using UDG-TALED, while metribuzin resistance was achieved with V219I and A251V mutations using ptpTALECD and ptpTALECD_v2. In many species, the plastid genome is maternally inherited, so herbicide-resistant mutations in the maternal line’s plastid genome are passed on to all progenies (Chung et al., 2023).
Plastid genome-targeted base editing offers a better understanding of organellar genes necessary for respiration and photosynthesis and their role in enhancing desirable traits. Additionally, in many countries, null-segregant base-edited plants can bypass strict regulations required for cultivating genetically modified crops outdoors.
3.2 CRISPR/Cas9-mediated editing in chloroplasts
Editing the chloroplast genome has remarkable potential to enhance crop modification, which in turn can help to meet the growing demands for agricultural sustainability and other future challenges. Interestingly, the RNA-guided genome editing tool CRISPR/Cas9 has been rapidly developed and widely used in plant nuclear gene editing (Li et al., 2013; Nekrasov et al., 2013). However, the application of CRISPR/Cas9-mediated editing in chloroplasts has been rarely reported, primarily due to the difficulty of simultaneously transporting and expressing both the guide RNA and cas9 protein into chloroplasts (Kim et al., 2021). Nevertheless, a few successful attempts have been made in CRISPR/Cas9-mediated chloroplast genome editing by overcoming the key obstacles to initiate the advanced chloroplast genome editing approaches. A new chloroplast genome editing technique that introduces “Edit Plasmids” in Chlamydomonas reinhardtii was successfully demonstrated. This method enabled induced donor DNA insertion at the target sites cleaved by Cas9/gRNAs in algal chloroplasts (Yoo et al., 2020) (Figure 8A). The INDEL mutations and substitutions at the target cleavage site with the “Edit Plasmids” approach were observed at a lower frequency. This suggests that homology-directed DNA repair (HDR) and replacement are the main outcomes of Cas9-induced cleavages in organelles. The Edit Plasmid can autonomously replicate and persist for an extended period in organelles to induce gene editing. Analysis of independent transgenic events for donor DNA insertion into Cas9/gRNA-driven target sites revealed an overall frequency of two positive events out of 20 independent transformants (10%). The construct employed in that study contained two guide RNAs (sgRNA1c and sgRNA2c) and Cas9 expressed under the strong psaA promoter. In another experiment, on-target mutations at one Cas9/gRNA target site were analyzed in chloroplasts transformed with Edit Plasmids containing or lacking the guide RNA (sgRNA2c) or donor DNA carrying Cas9 with different promoters (psaA, psbD, or none). SNPs resulting from Edit Plasmids (YP11 and YP31), which carry active Cas9 under the strong psaA promoter, led to the loss of an AvaII site in the chloroplast genome at a rate of 6.06%. Analysis of negative control Edit Plasmids without Cas9 (YP5) or guide RNA (YP29) showed a frequency of 7% for the loss of the AvaII site. Tang et al. successfully conducted a study involving the targeted delivery of CRISPR/Cas9 editing tools to tobacco chloroplasts using biolistics (Tang et al., 2020). The authors used editing tools to cut the specific target region between two homologous recombinant fragments to promote the integration of exogenous donor DNA. The efficiency of chloroplast transformation using this method was shown to increase six to ten-fold, demonstrating the potential to extend this technology to several other crop species (Figure 8B). However, future challenges include improving editing efficiency in different crop species, removing unmodified organellar genomes, and achieving complete homoplasmy.

Figure 8. Schematic representation of the plasmid model constructs for CRISPR/Cas9 editing in chloroplasts. (A) Structures of Edit Plasmids used in editing Chlamydomonas chloroplasts (Yoo et al., 2020), (ptP-Plastid promoter, SM-selectable marker gene, HR1 and HR2-short homologous regions of wild plastid genome). (B) Map of chloroplast transformation vector used for tobacco chloroplast genome editing (Tang et al., 2020). ptP, plastid gene promoter; ptT, plastid gene terminator; SM, selectable marker gene; HR1 and HR 2, homologous regions 1 and 2; Flank-L, left flanking sequence; Flank-R, right flanking sequence.
3.3 Plastid-mediated RNA interference
Plastid-mediated RNA interference (PM-RNAi) has emerged as a viable alternative strategy to chemical pesticides for controlling invasive pests in several crop species (Table 4). This is achieved through a core mechanism that involves the uptake of dsRNA with a sequence that matches essential insect genes into the midgut cells of the insect and leads to disruption in the expression of the targeted gene(s) (Figure 9). Stable dsRNA expression was first studied in the plastids of Nicotiana tabacum and Solanum tuberosum with three different dsRNA constructs to target the Colorado potato beetle (CPB), one of the most invasive insect pests of solanaceous crops (Zhang et al., 2015a). The accumulation levels of ACT (encoding β-actin) dsRNA were found to be ~0.4% of the total cellular RNA, which is higher than those of SHR (encoding Shrub) and ACT+SHR dsRNA. The potential effects of CPB β-actin on three other potato pests have been evaluated in another study. Growth retardation and suppression of the HvACT (Henosepilachna vigintioctopunctata actin gene) gene was observed, with 91.7% identity to ACT of CPB (Ren et al., 2021a). A different approach, “RNAi-of-RNAi”, (improving the effect of PM-RNAi in transplastomic plants by treating with in vitro-synthesized fusion dsRNA) provides conceptual proof for enhanced insect resistance in potatoes. The authors generated transplastomic potato lines expressing dsRNA corresponding to Shibire (dsSHI) (encoding a dynamin protein) of CPB, which showed a less potent RNAi response than CPB Shrub (St-dsSHR) (encoding Notch receptor) or β-actin (St-dsACT). However, an improved effect of PM-RNAi by dsSHI was achieved with a dsRNase-silencing approach, fusing dsRNAs targeting the dsRNase1/2 genes. This significantly reduced CPB larva weight, with dsSHI accumulation levels up to 0.1% of the total cellular RNA (Li et al., 2023). The silencing of cytochrome p450 monooxygenase (P450), V‐ATPase, and Chitin Synthase (Chi) genes via plastid-mediated RNAi in tobacco has been demonstrated. The disruption of target genes in the insect midgut led to stunted larval development and poor pupation in Helicoverpa armigera. The transcript abundance was found to be 3.5 million dsRNA copies per µg of total RNA (Jin et al., 2015). RNAi efficiency was assessed in another study by feeding in vitro-synthesized dsRNA of the 2222 bp v-ATPase A gene directly to larvae of M. sexta, inducing effective lethality via gene silencing. Whereas plastid transgene-derived dsRNA had not significant effect on survival, this suggests that the length of long dsRNA influences its low accumulation levels in plastids, which resulted in unproductive RNAi in M.sexta as compared to highly accumulated control short dsRNA (Burke et al., 2019). The control of Frankliniella occidentalis (Western Flower Thrip or WFT) by PM-RNAi was evaluated and published recently (Wu et al., 2022). Transplastomic tobacco plants expressing dsRNA and hairpin RNA (hpRNA) were generated to target four essential WFT genes: ACT, TUB, VAT, and SNF (Table 4). Despite using the same Prrn promotor, there was a considerable variation in RNA accumulation levels for both dsRNA and hpRNA. However, the results indicated that both acted as effective elicitors of RNAi responses and achieved better results than nuclear transgenic plants against WFT (Wu et al., 2022). An attempt to control sap-sucking insect pests by PM-RNAi was also established. The proportion of aphids feeding on transplastomic plants was lower, and these plants grew significantly faster with superior protection compared to nuclear transgenic plants (Dong et al., 2022). The PM-RNAi strategy was also validated in tomato plants expressing dsRNA of the v-ATPase A gene to control leaf-chewing and lacerate-and-flush feeding insects (Kaplanoglu et al., 2022). With the progression of research in plastid-mediated RNAi in a few crop species, there is a need to further expand the study range for economically important crops.

Figure 9. Schematic design of plastid transformation vectors for dsRNA expression. The targeted plastid genome regions with insertional sequence left (INSL) and insertional sequence right (INSR) are represented for recombination events. The strategy for cassette design to produce long dsRNA with specific promoter regions on either side of transgene, one with reverse orientation. The selectable marker gene aadA is driven by the promoter (P) with the 3’ untranslated terminator region (T).
3.4 Metabolic engineering through plastid transformation
Synthetic biology approaches in chloroplast transformation allow for achieving desired expression levels by choosing appropriate combinations of plastid gene expression elements (e.g., promoters, Shine–Dalgarno sequences, and 5’ and 3’ untranslated regions). However, this requires several trial-and-error steps to optimize the expression constructs (Herz et al., 2005). Certain factors, such as the strength of different gene expression signals and the sequence of the coding region, can influence the level of foreign protein accumulation in chloroplasts, which subsequently can be unpredictable. Recent applications of synthetic biology methods have striven to advance and extend the toolbox to incorporate different metabolic pathways (Birch-Machin et al., 2004) (Table 5). One of the best-demonstrated examples is the introduction of a complex metabolic pathway for the synthesis of biodegradable plastic, polyhydroxybutyrate (PHB). In this study, transplastomic plants accumulated significant amounts of transgene-derived protein, showed severe growth retardation, and were infertile. Application and improvements of components for inducible expression of plastid transgenes could help achieve routine high-level protein production for the development of plants as biofactories for the economic production of biopolymers (Lössl et al., 2005).
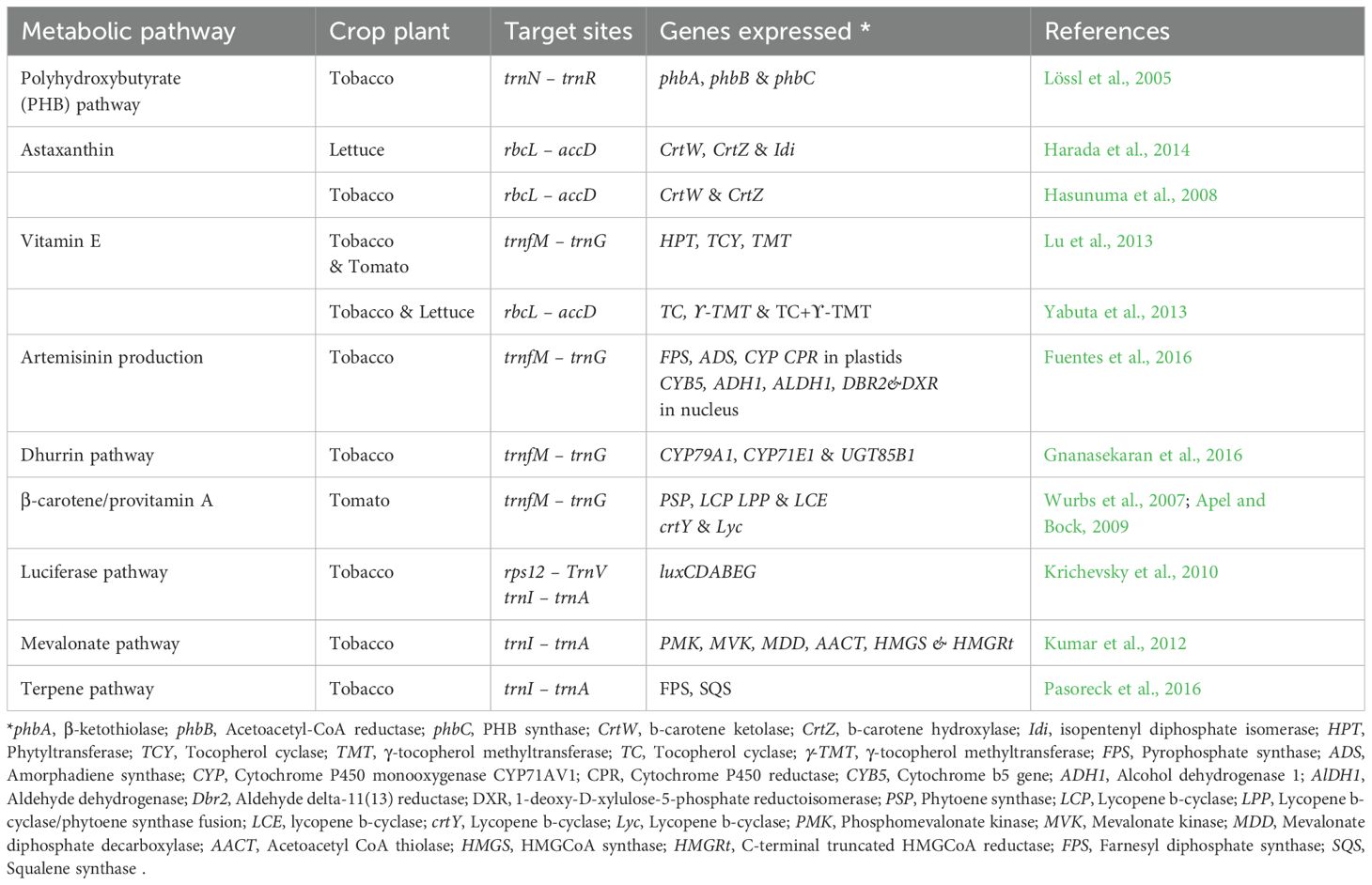
Table 5. Applications of synthetic biology in implementing metabolic engineering in crop plants via plastid transformation.
The expression of a synthetic operon consisting of genes encoding b-carotene ketolase (CrtW) and b-carotenehydroxylase (CrtZ) from the marine bacterium Brevundimona ssp. strain SD212 in tobacco chloroplasts led to a 2.1-fold higher accumulation of isoprenoid units in transplastomic plants. This was indicated by a color change in the plants from green to reddish brown (Hasunuma et al., 2008). Similar studies have also been conducted in lettuce (Harada et al., 2014). An efficient strategy has been described for enhancing the vitamin E (tocochromanol pathway) content in tomato and tobacco through stable plastid transformation (Lu et al., 2013). A series of synthetic operons were designed with homogentisate phytyltransferase (HPT), tocopherol cyclase (TCY), and γ-tocopherol methyltransferase (TMT) genes for tobacco and tomato plants, and the resulting transplastomic plants showed an overall ten-fold increase in total tocochromanol (antioxidant) accumulation in leaves. Expression of TC, ϒ-TMT, and TC+ϒ-TMT genes for vitamin-E synthesis has also been established in lettuce (Yabuta et al., 2013). Engineering of complex biochemical pathways for artemisinin biosynthesis was efficiently carried out to achieve high levels of metabolic production of the precursor compound, artemisinic acid, in tobacco. This was done using a co-transformation approach of both plastome and nuclear genomes, termed COSTREL (Combinatorial Supertransformation of Transplastomic Recipient Lines). Results from this promising strategy revealed a 77-fold increase in the accumulation of artemisinic acid and also identified the key contributor for higher product yield, i.e., aldehyde dehydrogenase (ALDH1) (Fuentes et al., 2016). A pathway from sorghum was engineered into tobacco chloroplasts for the production of dhurrin, a cyanogenic glucoside that acts as a defense metabolite (Gnanasekaran et al., 2016). Metabolic engineering in tomatoes was carried out by expressing carotenoid biosynthesis genes and analyzing the provitamin-A content, which increased four-fold (Wurbs et al., 2007). Similar studies were conducted for the expression of lycopene β-cyclase from daffodil, which resulted in a 50% increase in carotenoid accumulation in tomato fruits (Apel and Bock, 2009). A complex metabolic pathway for the expression of the lux operon with six genes was accomplished, resulting in plants capable of emitting light visible to the naked eye (Krichevsky et al., 2010). Accumulation of higher levels of mevalonate, carotenoids, squalene, sterols, and triacylglycerols was observed in transplastomic plants by inserting the entire cytosolic MEV (Mevalonate) pathway (Kumar et al., 2012). The metabolic engineering pathway for squalene biosynthesis using farnesyl diphosphate synthase (FPPS) and squalene synthase (SQS) was achieved in tobacco (Pasoreck et al., 2016). Despite several benefits, plastid-mediated metabolic engineering has certain drawbacks, including potential interference with plastid architecture, development, and metabolism due to foreign metabolites. The generation of microcompartments may also be relevant in the context of plastid metabolic engineering by protecting the plastid from toxic metabolite products. In one study, morphologically accurate carboxysomes were produced by transforming a full set of carboxysome genetic components derived from the H.neapolitanus (halophilic archaea) genome into the tobacco chloroplast or plastid genome (Chen et al., 2023).
4 Challenges to overcome in plastid transformation technology
4.1 Limited range of target plant species
Although plastid transformation has been achieved in several dicotyledonous crop plants, similar successes have not yet been achieved in monocotyledonous plants, except for rice (Lee et al., 2006; Wang et al., 2018). This may be due to the lack of efficient in vitro plant regeneration protocols among these species and their resistance to the selection agents used in chloroplast transformation (Wang et al., 2018). Another major limitation is the poor expression of transgenes in non-green plastids (Zhang et al., 2012). For instance, the expression of the fused p24-Nef operon, driven by Prrn, was shown to generate 2.5% of total soluble protein (TSP) in green tomatoes but was not observed in ripened fruit. Genome-wide analysis of green tissues of tomato fruits and potato tubers has shown that two essential genes, accD (involved in lipid metabolism) and clpP (involved in proteolytic machinery), are thought to play a key role in non-green plastids. Therefore, promoters and 5′ UTRs associated with these genes may help with high transgene accumulation in non-green plastids (Kahlau et al., 2006; Valkov et al., 2009).
4.2 Pleiotropic effects in transplastomic plants
In most reported studies, recombinant protein expression in plastids does not impact the progression of plant growth and development. However, some researchers have reported irregularities in phenotypic characteristics, such as yellowing of leaves, reduced plant growth, and infertility in transformed plants linked to expression of certain plastid transgene-derived proteins. Slower growth and a chlorotic appearance were observed, along with a reduction in protein levels by up to 50% when the HIV-1 Pr55gag polyprotein was produced in tobacco chloroplasts (Scotti et al., 2009). Similarly, 30% of transplastomic lines exhibited yellow tissues with the expression of HIV-1 proteinNef in tobacco and tomato plants (Zhou et al., 2008). The expression of the A27L immunogenic antigen from the vaccinia virus in transplastomic lines did not cause any changes in flowering or seed production, but resulted in reduced growth and a pale green appearance (Rigano et al., 2009). To date, the reasons behind these effects and their solution remain unclear, but a few clues have emerged to explain the connection between an abnormal physical appearance and the specific gene being activated (Ruiz and Daniell, 2005). Excessive production of foreign proteins may have negative impacts, such as disrupting the structure and function of thylakoids, activating new reading frames involved in cytoplasmic metabolism, or reducing ATP production levels (Pelletier and Budar, 2007). Recently, the application of protein fusions, such as SUMO (small ubiquitin-like modifier) in the expression of multiple AMPs has led to increased protein accumulation and reduced phenotypic effects (Hoelscher et al., 2022).
The potential unintended side effects on native chloroplast gene expression due to transgene insertion and subsequent high-level expression through plastid transformation have been comprehensively studied. Chloroplast gene expression within the petL-petG-psaJ-rpl33-rps18 transcription unit at three different transgene integration sites was analyzed by targeted ribosome and transcriptome profiling approaches (Ghandour et al., 2023). The data suggests that transcriptional read-through occurs frequently, with an overaccumulation of transcripts from downstream sense-oriented genes. However, there are reduced transcriptional and translational activities in downstream antisense-oriented native chloroplast genes. Together, the findings provide a deep understanding that can help in choosing insertion sites and other components for transgenic expression in plastids without influencing nearby native genes.
4.3 Lack of commercialization of plastid technology
Despite outstanding achievements at the laboratory scale, chloroplast transformation technology has not yet reached the commercial field level. Promising developments have emerged to streamline the transformation process by adopting advanced cloning methods and new selectable markers in the construction of expression vectors. The recent FDA (Food and Drug Administration) approval of IND (Investigational New Drug) CTB-ACE2 (cholera toxin B fused angiotensin-converting enzyme 2) chewing gum (Ganesan et al., 2023) paved the way for a Phase I/II placebo-controlled, randomized double blind clinical trial to advance CTB proinsulin to clinics and develop affordable oral insulin (Daniell et al., 2023). A few studies have demonstrated downstream technologies for protein purification using temporary immersion bioreactors (TIBs) for transgenic plant biomass propagation (Michoux et al., 2013). More recently, Wu et al. (2025) outlined the experimental procedures for designing transformation constructs for PEP purification from transplastomic tobacco plants (Wu et al., 2025). Synthetic elements are emerging as key tools for the advancement of chloroplast engineering and transformation methods, driving this technology towards field level applications (Scharff and Bock, 2014).
5 Concluding remarks
Chloroplast engineering provides a promising opportunity for expressing various industrial and agricultural traits to meet the growing food demand from an increasing world population. Advances in chloroplast transformation have demonstrated its potential in the fields of agriculture (Harada et al., 2014; Lin et al., 2014), phytoremediation (Ruiz et al., 2011), and biopharmaceuticals (Boyhan and Daniell, 2011). Progress in transplastomic technology has highlighted its importance as an ideal bio-factory for the production of antigenic vaccines (Su et al., 2015). Synthetic biology approaches are being explored for vector design, DNA delivery, and gene regulation to advance plastid engineering research. However, plastid transformation in non-green tissues remains elusive (Bock, 2015). Recent advancements in plastid engineering and transformation methods have created new opportunities for exploring precise genome-level editing. Despite numerous challenges, plastid transformation holds the potential to enable cost-effective production of recombinant proteins and eliminate socio-environmental issues associated with nuclear genome-modified crops.
Author contributions
MN: Conceptualization, Writing – original draft, Writing – review & editing. IN: Writing – review & editing. BP: Writing – review & editing. SA: Writing – review & editing. GW: Writing – review & editing. PB: Investigation, Supervision, Writing – review & editing.
Funding
The author(s) declare that financial support was received for the research and/or publication of this article. Publication fees were covered by the National Research Council of Canada.
Acknowledgments
MKN would like to thank Prof. A. Sadanandam, Kakatiya University, India for his support and valuable suggestions.
Conflict of interest
Author GW was employed by company Relica Genomics.
The remaining authors declare that the research was conducted in the absence of any commercial or financial relationships that could be construed as a potential conflict of interest.
Generative AI statement
The author(s) declare that no Generative AI was used in the creation of this manuscript.
Publisher’s note
All claims expressed in this article are solely those of the authors and do not necessarily represent those of their affiliated organizations, or those of the publisher, the editors and the reviewers. Any product that may be evaluated in this article, or claim that may be made by its manufacturer, is not guaranteed or endorsed by the publisher.
References
Agrawal, S., Karcher, D., Ruf, S., Erban, A., Hertle, A. P., Kopka, J., et al. (2022). Riboswitch-mediated inducible expression of an astaxanthin biosynthetic operon in plastids. Plant Physiol. 188, 637–652. doi: 10.1093/plphys/kiab428
Allison, L. A., Maliga, P. (1995). Light-responsive and transcription enhancing elements regulate the plastid psbD core promoter. EMBO J. 14, 3721–3730. doi: 10.1002/j.1460-2075.1995.tb00042.x
Altpeter, F., Baisakh, N., Beachy, R., Bock, R., Capell, T., Christou, P., et al. (2005). Particle bombardment and the genetic enhancement of crops: myths and realities. Mol. Breed. 15, 305–327. doi: 10.1007/s11032-004-8001-y
Apel, W., Bock, R. (2009). Enhancement of carotenoid biosynthesis in transplastomic tomatoes by induced lycopene-to-provitamin A conversion. Plant Physiol. 151, 59–66. doi: 10.1104/pp.109.140533
Avellan, A., Yun, J., Zhang, Y., Spielman-Sun, E., Unrine, J. M., Thieme, J., et al. (2019). Nanoparticle size and coating chemistry control foliar uptake pathways, translocation, and leaf-to-rhizosphere transport in wheat. ACS Nano 13, 5291–5305. doi: 10.1021/acsnano.8b09781
Baeza, L., Bertrand, A., Mache, R., Lerbs-Mache, S. (1991). Characterization of a protein binding sequence in the promoter region of the 16S rRNA gene of the spinach chloroplast genome. Nucleic Acids Res. 19, 3577–3581. doi: 10.1093/nar/19.13.3577
Barkan, A., Goldschmidt-Clermont, M. (2000). Participation of nuclear genes in chloroplast gene expression. Biochimie 82, 559–572. doi: 10.1016/s0300-9084(00)00602-7
Barkan, A., Rojas, M., Fujii, S., Yap, A., Chong, Y. S., Bond, C. S., et al. (2012). A combinatorial amino acid code for RNA recognition by pentatricopeptide repeat proteins. PloS Genet. 8, e1002910. doi: 10.1371/journal.pgen.1002910
Barrick, J. E., Corbino, K. A., Winkler, W. C., Nahvi, A., Mandal, M., Collins, J., et al. (2004). New RNA motifs suggest an expanded scope for riboswitches in bacterial genetic control. Proc. Natl. Acad. Sci. U. S. A. 101, 6421–6426. doi: 10.1073/pnas.0308014101
Bellucci, M., De Marchis, F., Ferradini, N., Pompa, A., Veronesi, F., Rosellini, D. (2015). A mutant Synechococcus gene encoding glutamate 1-semialdehyde aminotransferase confers gabaculine resistance when expressed in tobacco plastids. Plant Cell Rep. 34, 2127–2136. doi: 10.1007/s00299-015-1856-z
Birch-Machin, I., Newell, C. A., Hibberd, J. M., Gray, J. C. (2004). Accumulation of rotavirus VP6 protein in chloroplasts of transplastomic tobacco is limited by protein stability. Plant Biotechnol. J. 2, 261–270. doi: 10.1111/j.1467-7652.2004.00072.x
Bock, R. (2007). Plastid biotechnology: prospects for herbicide and insect resistance, metabolic engineering and molecular farming. Curr. Opin. Biotechnol. 18, 100–106. doi: 10.1016/j.copbio.2006.12.001
Bock, R. (2014). Engineering chloroplasts for high-level foreign protein expression. Methods Mol. Biol. (Clifton N.J.) 1132, 93–106. doi: 10.1007/978-1-62703-995-6_5
Bock, R. (2015). Engineering plastid genomes: methods, tools, and applications in basic research and biotechnology. Annu. Rev. Plant Biol. 66, 211–241. doi: 10.1146/annurev-arplant-050213-040212
Boyhan, D., Daniell, H. (2011). Low-cost production of proinsulin in tobacco and lettuce chloroplasts for injectable or oral delivery of functional insulin and C-peptide. Plant Biotechnol. J. 9, 585–598. doi: 10.1111/j.1467-7652.2010.00582.x
Buhot, L., Horvàth, E., Medgyesy, P., Lerbs-Mache, S. (2006). Hybrid transcription system for controlled plastid transgene expression. Plant J. 46, 700–707. doi: 10.1111/j.1365-313X.2006.02718.x
Burke, W. G., Kaplanoglu, E., Kolotilin, I., Menassa, R., Donly, C. (2019). RNA interference in the tobacco hornworm, Manduca sexta, using plastid-encoded long double-stranded RNA. Front. Plant Sci. 10. doi: 10.3389/fpls.2019.00313
Carrer, H., Hockenberry, T. N., Svab, Z., Maliga, P. (1993). Kanamycin resistance as a selectable marker for plastid transformation in tobacco. Mol. Gen. Genetics: MGG 241, 49–56. doi: 10.1007/BF00280200
Carrillo, N., Seyer, P., Tyagi, A., Herrmann, R. G. (1986). Cytochrome b-559 genes from Oenothera hookeri and Nicotiana tabacum show a remarkably high degree of conservation as compared to spinach. The enigma of cytochrome b-559: highly conserved genes and proteins but no known function. Curr. Genet. 10, 619–624. doi: 10.1007/BF00418129
Chen, T., Hojka, M., Davey, P., Sun, Y., Dykes, G. F., Zhou, F., et al. (2023). Engineering α-carboxysomes into plant chloroplasts to support autotrophic photosynthesis. Nat. Commun. 14, 2118. doi: 10.1038/s41467-023-37490-0
Chen, Q., Wu, Y., Wang, Y., Zhang, J., Li, S. (2024). Stable plastid transformation in kiwifruit (Actinidia chinensis). aBIOTECH 6, 72–80. doi: 10.1007/s42994-024-00186-0
Cheng, L., Li, H. P., Qu, B., Huang, T., Tu, J. X., Fu, T. D., et al. (2010). Chloroplast transformation of rapeseed (Brassica napus) by particle bombardment of cotyledons. Plant Cell Rep. 29, 371–381. doi: 10.1007/s00299-010-0828-6
Cho, S. I., Lee, S., Mok, Y. G., Lim, K., Lee, J., Lee, J. M., et al. (2022). Targeted A-to-G base editing in human mitochondrial DNA with programmable deaminases. Cell 185, 1764–1776. doi: 10.1016/j.cell.2022.03.039
Christou, P., Ford, T., Kofron, M. (1991). Production of transgenic rice (Oryza Sativa L.) plants from agronomically important indica and japonica varieties via electric discharge particle acceleration of exogenous DNA into immature zygotic embryos. Nat. Biotechnol. 9, 957–962. doi: 10.1038/nbt1091-957
Chung, K. P., Gonzalez-Duran, E., Ruf, S., Endries, P., Bock, R. (2023). Control of plastid inheritance by environmental and genetic factors. Nat. Plants. 9, 68–80. doi: 10.1038/s41477-022-01323-7
Craig, W., Lenzi, P., Scotti, N., De Palma, M., Saggese, P., Carbone, V., et al. (2008). Transplastomic tobacco plants expressing a fatty acid desaturase gene exhibit altered fatty acid profiles and improved cold tolerance. Transgenic Res. 17, 769–782. doi: 10.1007/s11248-008-9164-9
Daniell, H., Datta, R., Varma, S., Gray, S., Lee, S. B. (1998). Containment of herbicide resistance through genetic engineering of the chloroplast genome. Nat. Biotechnol. 16, 345–348. doi: 10.1038/nbt0498-345
Daniell, H., Lin, C. S., Yu, M., Chang, W. J. (2016). Chloroplast genomes: diversity, evolution, and applications in genetic engineering. Genome Biol. 17, 134. doi: 10.1186/s13059-016-1004-2
Daniell, H., Singh, R., Mangu, V., Nair, S. K., Wakade, G., Balashova, N. (2023). Affordable oral proinsulin bioencapsulated in plant cells regulates blood sugar levels similar to natural insulin. Biomaterials 298, 122142. doi: 10.1016/j.biomaterials.2023.122142
Davoodi-Semiromi, A., Schreiber, M., Nalapalli, S., Verma, D., Singh, N. D., Banks, R. K., et al. (2010). Chloroplast-derived vaccine antigens confer dual immunity against cholera and malaria by oral or injectable delivery. Plant Biotechnol. J. 8, 223–242. doi: 10.1111/j.1467-7652.2009.00479.x
De Block, M., Schell, J., Van Montagu, M. (1985). Chloroplast transformation by Agrobacterium tumefaciens. EMBO J. 4, 1367–1372. doi: 10.1002/j.1460-2075.1985.tb03788.x
De Cosa, B., Moar, W., Lee, S. B., Miller, M., Daniell, H. (2001). Overexpression of the Bt cry2Aa2 operon in chloroplasts leads to formation of insecticidal crystals. Nat. Biotechnol. 19, 71–74. doi: 10.1038/83559
De Marchis, F., Wang, Y., Stevanato, P., Arcioni, S., Bellucci, M. (2009). Genetic transformation of the sugar beet plastome. Transgenic Res. 18, 17–30. doi: 10.1007/s11248-008-9193-4
Dong, Y., Wu, M., Zhang, Q., Fu, J., Loiacono, F. V., Yang, Y., et al. (2022). Control of a sap-sucking insect pest by plastid-mediated RNA interference. Mol. Plant 15, 1176–1191. doi: 10.1016/j.molp.2022.05.008
Drechsel, O., Bock, R. (2011). Selection of Shine-Dalgarno sequences in plastids. Nucleic Acids Res. 39, 1427–1438. doi: 10.1093/nar/gkq978
Dufourmantel, N., Pelissier, B., Garcon, F., Gilles Peltier, G., Ferullo, J. M., Tissot, G. (2004). Generation of fertile transplastomic soybean. Plant Mol. Biol. 55, 479–489. doi: 10.1007/s11103-004-0192-4
Eibl, C., Zou, Z., Beck, a., Kim, M., Mullet, J., Koop, H. U. (1999). In vivo analysis of plastid psbA, rbcL and rpl32 UTR elements by chloroplast transformation: tobacco plastid gene expression is controlled by modulation of transcript levels and translation efficiency. Plant J. 19, 333–345. doi: 10.1046/j.1365-313x.1999.00543.x
Eisermann, A., Tiller, K., Link, G. (1990). In vitro transcription and DNA binding characteristics of chloroplast and etioplast extracts from mustard (Sinapis alba) indicate differential usage of the psbA promoter. EMBO J. 9, 3981–3987. doi: 10.1002/j.1460-2075.1990.tb07619.x
Emadpour, M., Karcher, D., Bock, R. (2015). Boosting riboswitch efficiency by RNA amplification. Nucleic Acids Res. 43, e66. doi: 10.1093/nar/gkv165
Fu, X., Duc, L. T., Fontana, S., Bong, B. B., Tinjuangjun, P., Sudhakar, D., et al. (2000). Linear transgene constructs lacking vector backbone sequences generate low-copy-number transgenic plants with simple integration patterns. Transgenic Res. 9, 11–19. doi: 10.1023/a:1008993730505
Fuentes, P., Zhou, F., Erban, A., Karcher, D., Kopka, J., Bock, R. (2016). A new synthetic biology approach allows transfer of an entire metabolic pathway from a medicinal plant to a biomass crop. eLife 5, e13664. doi: 10.7554/eLife.13664
Ganesan, P. K., Kulchar, R. J., Kaznica, P., Montoya-Lopez, R., Green, B. J., Streatfield, S. J., et al. (2023). Optimization of biomass and target protein yield for Phase III clinical trial to evaluate angiotensin converting enzyme 2 expressed in lettuce chloroplasts to reduce SARS-CoV-2 infection and transmission. Plant Biotechnol. J. 21, 244–246. doi: 10.1111/pbi.13954
Ganesan, I., Shi, L.-X., Labs, M., Theg, S. M. (2018). Evaluating the functional pore size of chloroplast TOC and TIC protein translocons: import of folded proteins. Plant Cell 30, 2161–2173. doi: 10.1105/tpc.18.00427
Ghandour, R., Gao, Y., Laskowski, J., Barahimipour, R., Ruf, S., Bock, R., et al. (2023). Transgene insertion into the plastid genome alters expression of adjacent native chloroplast genes at the transcriptional and translational levels. Plant Biotechnol. J. 21, 711–725. doi: 10.1111/pbi.13985
Gnanasekaran, T., Karcher, D., Nielsen, A. Z., Martens, H. J., Ruf, S., Kroop, X., et al. (2016). Transfer of the cytochrome P450-dependent dhurrin pathway from Sorghum bicolor into Nicotiana tabacum chloroplasts for light-driven synthesis. J. Exp. Bot. 67, 2495–2506. doi: 10.1093/jxb/erw067
Golds, T., Maliga, P., Koop, H. U. (1993). Stable plastid transformation in PEG-treated protoplasts of Nicotiana tabacum. Nat. Biotechnol. 11, 95–97. doi: 10.1038/nbt0193-95
Gosal, S. S., Wani, S. H., Kang, M. S. (2009). Biotechnology and drought tolerance. J. Crop Improv. 23, 19–54. doi: 10.1080/15427520802418251
Harada, H., Maoka, T., Osawa, A., Hattan, J., Kanamoto, H., Shindo, K., et al. (2014). Construction of transplastomic lettuce (Lactuca sativa) dominantly producing astaxanthin fatty acid esters and detailed chemical analysis of generated carotenoids. Transgenic Res. 23, 303–315. doi: 10.1007/s11248-013-9750-3
Hasunuma, T., Miyazawa, S., Yoshimura, S., Shinzaki, Y., Tomizawa, K., Shindo, K., et al. (2008). Biosynthesis of astaxanthin in tobacco leaves by transplastomic engineering. Plant J. Cell Mol.Biol. 55, 857–868. doi: 10.1111/j.1365-313X.2008.03559.x
Hayashi, K., Shiina, T., Ishii, N., Iwai, K., Ishizaki, Y., Morikawa, K., et al. (2003). A role of the – 35 element in the initiation of transcription at psbA promoter in tobacco plastids. Plant Cell Physiol. 44, 334–341. doi: 10.1093/pcp/pcg041
Hayes, R., Kudla, J., Schuster, G., Gabay, L., Maliga, P., Gruissem, W. (1996). Chloroplast mRNA 3'-end processing by a high molecular weight protein complex is regulated by nuclear encoded RNA binding proteins. EMBO J. 15, 1132–1141.
Hennig, A., Bonfig, K., Roitsch, T., Warzecha, H. (2007). Expression of the recombinant bacterial outer surface protein A in tobacco chloroplasts leads to thylakoid localization and loss of photosynthesis. FEBS J. 274, 5749–5758. doi: 10.1111/j.1742-4658.2007.06095.x
Hertle, A. P., Haberl, B., Bock, R. (2021). Horizontal genome transfer by cell-to-cell travel of whole organelles. Sci. Adv. 7, eabd8215. doi: 10.1126/sciadv.abd8215
Herz, S., Füssl, M., Steiger, S., Koop, H. U. (2005). Development of novel types of plastid transformation vectors and evaluation of factors controlling expression. Transgenic Res. 14, 969–982. doi: 10.1007/s11248-005-2542-7
Hirata, N., Yonekura, D., Yanagisawa, S., Iba, K. (2004). Possible involvement of the 5′-flanking region and the 5′ UTR of plastid accD gene in NEP-dependent transcription. Plant Cell Physiol. 45, 176–186. doi: 10.1093/pcp/pch021
Hirose, T., Sugiura, M. (2004). Multiple elements required for translation of plastid atpB mRNA lacking the Shine-Dalgarno sequence. Nucleic Acids Res. 32, 3503–3510. doi: 10.1093/nar/gkh682
Ho, M. W., Cummins, J. (2005). Molecular pharming by chloroplast transformation. (Institute of Science in Society). Available online at: https://www.isis.org.uk/MPBCT.php (Accessed July 20, 2005).
Hoelscher, M. P., Forner, J., Calderone, S., Krämer, C., Taylor, Z., Loiacono, F. V., et al. (2022). Expression strategies for the efficient synthesis of antimicrobial peptides in plastids. Nat. Commun. 13, 5856. doi: 10.1038/s41467-022-33516-1
Hou, B. K., Zhou, Y. H., Wan, L. H., Zhang, Z. L., Shen, G. F., Chen, Z. H., et al. (2003). Chloroplast transformation in oilseed rape. Transgenic Res. 12, 111–114. doi: 10.1023/a:1022180315462
Hu, J., Sun, Y., Li, B., Liu, Z., Wang, Z., Gao, Q., et al. (2024). Strand-preferred base editing of organellar and nuclear genomes using CyDENT. Nat. Biotechnol. 42, 936–945. doi: 10.1038/s41587-023-01910-9
Huang, F. C., Klaus, S. M., Herz, S., Zou, Z., Koop, H. U., Golds, T. J. (2002). Efficient plastid transformation in tobacco using the aphA-6 gene and kanamycin selection. Mol. Genet. Genomics: MGG 268, 19–27. doi: 10.1007/s00438-002-0738-6
Iamtham, S., Day, A. (2000). Removal of antibiotic resistance genes from transgenic tobacco plastids. Nat. Biotechnol. 18, 1172–1176. doi: 10.1038/81161
Jakubiec, A., Sarokina, A., Choinard, S., Vlad, F., Malcuit, I., Sorokin, A. P. (2021). Replicating minichromosomes as a new tool for plastid genome engineering. Nat. Plants. 7, 932–941. doi: 10.1038/s41477-021-00940-y
Jin, S. X., Singh, N. D., Li, L. B., Zhang, X. L., Daniell, H. (2015). Engineered chloroplast dsRNA silences cytochrome p450 monooxygenase, V-ATPase and chitin synthase genes in the insect gut and disrupts Helicoverpa armigera larval development and pupation. Plant Biotechnol. J. 13, 435–446. doi: 10.1111/pbi.12355
Kahlau, S., Aspinall, S., Gray, J. C., Bock, R. (2006). Sequence of the tomato chloroplast DNA and evolutionary comparison of solanaceous plastid genomes. J. Mol. Evol. 63, 194–207. doi: 10.1007/s00239-005-0254-5
Kahlau, S., Bock, R. (2008). Plastid transcriptomics and translatomics of tomato fruit development and chloroplast-to-chromoplast differentiation: chromoplast gene expression largely serves the production of a single protein. Plant Cell 20, 856–874. doi: 10.1105/tpc.107.055202
Kanamoto, H., Yamashita, A., Asao, H., Okumura, S., Takase, H., Hattori, M., et al. (2006). Efficient and stable transformation of Lactuca sativa L. cv. Cisco (lettuce) plastids. Transgenic Res. 15, 205–217. doi: 10.1007/s11248-005-3997-2
Kang, B. C., Bae, S. J., Lee, S., Lee, J. S., Kim, A., Lee, H., et al. (2021). Chloroplast and mitochondrial DNA editing in plants. Nat. Plants. 7, 899–905. doi: 10.1038/s41477-021-00943-9
Kang, T. J., Loc, N. H., Jang, M. O., Jang, Y. S., Kim, Y. S., Seo, J. E., et al. (2003). Expression of the B subunit of E. coli heat-labile enterotoxin in the chloroplasts of plants and its characterization. Transgenic Res. 12, 683–691. doi: 10.1023/b:trag.0000005114.23991.bc
Kaplanoglu, E., Kolotilin, I., Menassa, R., Donly, C. (2022). Plastid transformation of micro-tom tomato with a hemipteran double-stranded RNA results in RNA interference in multiple insect species. Int. J. Mol. Sci. 23, 3918. doi: 10.3390/ijms23073918
Kapoor, S., Sugiura, M. (1999). Identification of two essential sequence elements in the nonconsensus type II PatpB-290 plastid promoter by using plastid transcription extracts from cultured tobacco BY-2 cells. Plant Cell 11, 1799–1810. doi: 10.1105/tpc.11.9.1799
Kaushal, C., Abdin, M. Z., Kumar, S. (2020). Chloroplast genome transformation of medicinal plant Artemisia annua. Plant Biotechnol. J. 18, 2155–2157. doi: 10.1111/pbi.13379
Kavanagh, T. A., Thanh, N. D., Lao, N. T., McGrath, N., Peter, S. O., Horváth, E. M., et al. (1999). Homeologous plastid DNA transformation in tobacco is mediated by multiple recombination events. Genetics 152, 1111–1122. doi: 10.1093/genetics/152.3.1111
Khan, M. S., Kanwal, B., Nazir, S. (2015). Metabolic engineering of the chloroplast genome reveals that the yeast ArDH gene confers enhanced tolerance to salinity and drought in plants. Front. Plant Sci. 6. doi: 10.3389/fpls.2015.00725
Kim, J. S., Kang, B. C., Bae, S. J., Lee, S., Kim, J. (2021). Chloroplast and mitochondrial DNA editing in plants. Nat. Plants. 7, 899–905. doi: 10.1038/s41477-021-00943-9
Kittiwongwattana, C., Lutz, K., Clark, M., Maliga, P. (2007). Plastid marker gene excision by the phiC31 phage site-specific recombinase. Plant Mol. Bio. 64, 137–143. doi: 10.1007/s11103-007-9140-4
Klaus, S. M., Huang, F. C., Golds, T. J., Koop, H. U. (2004). Generation of marker-free plastid transformants using a transiently cointegrated selection gene. Nat. Biotechnol. 22, 225–229. doi: 10.1038/nbt933
Klein, T., Wolf, E., Wu, R., Sanford, J. C. (1987). High-velocity microprojectiles for delivering nucleic acids into living cells. Nature 327, 70–73. doi: 10.1038/327070a0
Kohli, A., Xiong, J., Greco, R., Christou, P., Pereira, A. (2001). Tagged transcriptome display (TTD) in indica rice using Ac transposition. Mol. Genet. Genomics: MGG 266, 1–11. doi: 10.1007/s004380100528
Koop, H. U., Steinmüller, K., Wagner, H., Rössler, C., Eibl, C., Sacher, L. (1996). Integration of foreign sequences into the tobacco plastome via polyethylene glycol-mediated protoplast transformation. Planta 199, 193–201. doi: 10.1007/BF00196559
Kota, S., Lakkam, R., Kasula, K., Narra, M., Qiang, H., Rao Allini, V., et al. (2019). Construction of a species-specific vector for improved plastid transformation efficiency in Capsicum annuum L. 3 Biotech. 9, 226. doi: 10.1007/s13205-019-1747-z
Krichevsky, A., Meyers, B., Vainstein, A., Maliga, P., Citovsky, V. (2010). Autoluminescent plants. PLoS One 5, e15461. doi: 10.1371/journal.pone.0015461
Kumar, S., Dhingra, A., Daniell, H. (2004). Stable transformation of the cotton plastid genome and maternal inheritance of transgenes. Plant Mol. Bio. 56, 203–216. doi: 10.1007/s11103-004-2907-y
Kumar, S., Dhingra, A., Daniell, H. (2004a). Plastid-expressed betaine aldehyde dehydrogenase gene in carrot cultured cells, roots, and leaves confers enhanced salt tolerance. Plant Physiol. 136, 2843–2854. doi: 10.1104/pp.104.045187
Kumar, S., Hahn, F. M., Baidoo, E., Kahlon, T. S., Wood, D. F., McMahan, C. M., et al. (2012). Remodeling the isoprenoid pathway in tobacco by expressing the cytoplasmic mevalonate pathway in chloroplasts. Metab. Eng. 14, 19–28. doi: 10.1016/j.ymben.2011.11.005
Kuroda, H., Maliga, P. (2001a). Complementarity of the 16S rRNA penultimate stem with sequences downstream of the AUG destabilizes the plastid mRNAs. Nucleic Acids Res. 29, 970–975. doi: 10.1093/nar/29.4.970
Kuroda, H., Maliga, P. (2001b). Sequences downstream of the translation initiation codon are important determinants of translation efficiency in chloroplasts. Plant Physiol. 125, 430–436. doi: 10.1104/pp.125.1.430
Kuroda, H., Sugiura, M. (2014). Processing of the 5′-UTR and existence of protein factors that regulate translation of tobacco chloroplast psbN mRNA. Plant Mol. Biol. 86, 585–593. doi: 10.1007/s11103-014-0248-z
Kwak, S.-Y., Lew, T. T. S., Sweeney, C. J., Koman, V. B., Wong, M. H., Bohmert-Tatarev, K., et al. (2019). Chloroplast-selective gene delivery and expression in planta using chitosan-complexed single-walled carbon nanotube carriers. Nat. Nanotechnol. 14, 447–455. doi: 10.1038/s41565-019-0375-4
Lam, D. K., Feliciano, P. R., Arif, A., Bohnuud, T., Fernandez, T. P., Gehrke, J. M., et al. (2023). Improved cytosine base editors generated from TadA variants. Nat. Biotechnol. 41, 686–697. doi: 10.1038/s41587-022-01611-9
Lee, S. M., Kang, K., Chung, H., Yoo, S. H., Xu, X. M., Lee, S. B., et al. (2006). Plastid transformation in the monocotyledonous cereal crop, rice (Oryza sativa) and transmission of transgenes to their progeny. Mol. Cells 21, 401–410. doi: 10.1007/s11248-005-3997-2
Legen, J., Dühnen, S., Gauert, A., Götz, M., Schmitz-Linneweber, C. A. (2023). CRR2-dependent sRNA sequence supports papillomavirus vaccine expression in tobacco chloroplasts. Metabolites 21, 315. doi: 10.3390/metabo13030315
Lelivelt, C. L. C., McCabe, M. S., Newell, C. A., Bastiaan deSnoo, C., van Dun, M. P. K., et al. (2005). Stable plastid transformation in lettuce (Lactuca sativa L.). Plant Mol. Biol. 58, 763–774. doi: 10.1007/s11103-005-7704-8
Li, R., Char, S. N., Liu, B., Liu, H., Li, X., Yang, B. (2021). High-efficiency plastome base editing in rice with TAL cytosine deaminase. Mol. Plant 14, 1412–1414. doi: 10.1016/j.molp.2021.07.007
Li, J., Norville, J. E., Aach, J., McCormack, M., Zhang, D., Bush, J., et al. (2013). Multiplex and homologous recombination–mediated genome editing in Arabidopsis and Nicotiana benthamiana using guide RNA and Cas9. Nat. Biotechnol. 31, 688–691. doi: 10.1038/nbt.2654
Li, W., Ruf, S., Bock, R. (2011). Chloramphenicol acetyltransferase as selectable marker for plastid transformation. Plant Mol. Biol. 76, 443–451. doi: 10.1007/s11103-010-9678-4
Li, Y., Xu, H., He, W., Rong, H., Li, S., Sung Kim, D., et al. (2023). Silencing of insect dsRNase genes enhances the plastid-mediated RNAi effect on the Colorado potato beetle. Entomol. Gen. 43, 69–77. doi: 10.1127/entomologia/2023/1739
Liere, K., Maliga, P. (1999a). In vitro characterization of the tobacco rpoB promoter reveals a core sequence motif conserved between phage-type plastid and plant mitochondrial promoters. EMBO J. 18, 249–257. doi: 10.1093/emboj/18.1.249
Liere, K., Maliga, P. (1999b). “Novel in Vitro transcription assay indicates that the ACCD Nep promoter is contained in a 19 BP fragment,” in The chloroplast: from molecular biology to Biotechnology, NATO science series vol. 64 . Eds. argyroudi-akoyunoglou, J. H., senger, H. (Springer, dordrecht). doi: 10.1007/978-94-011-4788-0_11
Lin, M. T., Occhialini, A., Andralojc, P. J., Parry, M. A., Hanson, M. R. (2014). A faster rubisco with potential to increase photosynthesis in crops. Nature 513, 547–550. doi: 10.1038/nature13776
Liu, C. W., Lin, C. C., Chen, J. J., Tseng, M. J. (2007). Stable chloroplast transformation in cabbage (Brassica oleracea L. var. capitata L.) by particle bombardment. Plant Cell Rep. 26, 1733–1744. doi: 10.1007/s00299-007-0374-z
Lössl, A., Bohmert, K., Harloff, H., Eibl, C., Mühlbauer, S., Koop, H. U. (2005). Inducible trans-activation of plastid transgenes: expression of the R. eutropha phb operon in transplastomic tobacco. Plant Cell Physiol. 46, 1462–1471. doi: 10.1093/pcp/pci157
Lössl, A., Eibl, C., Harloff, H. J., Jung, C., Koop, H. U. (2003). Polyester synthesis in transplastomic tobacco (Nicotiana tabacum L): Significant contents of polyhydroxybutyrate are associated with growth reduction. Plant Cell Rep. 21, 891–899. doi: 10.1007/s00299-003-0610-0
Lu, Y., Rijzaani, H., Karcher, D., Ruf, S., Bock, R. (2013). Efficient metabolic pathway engineering in transgenic tobacco and tomato plastids with synthetic multigene operons. Proc. Natl. Acad. Sci. U.S.A. 19, 23–32. doi: 10.1073/pnas.1216898110
Lu, Y., Stegemann, S., Agrawal, S., Karcher, D., Ruf, S., Bock, R. (2017). Horizontal transfer of a synthetic metabolic pathway between plant species. Curr. Biol. 27, 3034–3041.e3. doi: 10.1016/j.cub.2017.08.044
Lutz, K. A., Knapp, J. E., Maliga, P. (2001). Expression of bar in the plastid genome confers herbicide resistance. Plant Physiol. 125, 1585–1590. doi: 10.1104/pp.125.4.1585
Macedo-Osorio, K. S., Pérez-España, V. H., Garibay-Orijel, C., Guzmán-Zapata, D., Durán-Figueroa, N. V., Badillo-Corona, J. A. (2018). Intercistronic expression elements (IEE) from the chloroplast of Chlamydomonas reinhardtii can be used for the expression of foreign genes in synthetic operons. Plant Mol. Biol. 98, 303–317. doi: 10.1007/s11103-018-0776-z
Maliga, P. (2003). Progress towards commercialization of plastid transformation technology. Trends Biotechnol. 21, 20–28. doi: 10.1016/s0167-7799(02)00007-0
Maliga, P. (2004). Plastid transformation in higher plants. Annu. Rev. Plant Biol. 55, 289–313. doi: 10.1146/annurev.arplant.55.031903.141633
Matsuoka, A., Maliga, P. (2021). Prospects for reengineering Agrobacterium tumefaciens for T-DNA delivery to chloroplasts. Plant Physiol. 186, 215–220. doi: 10.1093/plphys/kiab081
Michoux, F., Ahmad, N., Hennig, A., Nixon, P. J., Warzecha, H. (2013). Production of leafy biomass using temporary immersion bioreactors: an alternative platform to express proteins in transplastomic plants with drastic phenotypes. Planta 237, 903–908. doi: 10.1007/s00425-012-1829-1
Min, S. R., Davarpanah, S. J., Jung, S. H., Park, Y., Liu, J. R., Jeong, W. J. (2015). An episomal vector system for plastid transformation in higher plants. Plant Biotechnol. Rep. 9, 443–449. doi: 10.1007/s11816-015-0381-4
Mok, Y. G., Hong, S., Bae, S.-J., Cho, S.-I., Kim, J.-S. (2022b). Targeted A-to-G base editing of chloroplast DNA in plants. Nat. Plants 8, 1378–1384. doi: 10.1038/s41477-022-01279-8
Mok, Y. G., Hong, S., Seo, D. I., Choi, S., Kim, H. K., Jin, D. M., et al. (2024). Herbicide-resistant plants produced by precision adenine base editing in plastid DNA. Nat. Plants 10, 1652–1658. doi: 10.1038/s41477-024-01808-7
Mok, B. Y., Kotrys, A. V., Raguram, A., Huang, T. P., K., M., Liu, D. R. (2022a). CRISPR-free base editors with enhanced activity and expanded targeting scope in mitochondrial and nuclear DNA. Nat. Biotechnol. 40, 1378–1387. doi: 10.1038/s41587-022-01256-8
Monde, R. A., Greene, J. C., Stern, D. B. (2000). The sequence and secondary structure of the 3'-UTR affect 3'-end maturation, RNA accumulation, and translation in tobacco chloroplasts. Plant Mol. Biol. 44, 529–542. doi: 10.1023/a:1026540310934
Mühlbauer, S. K., Koop, H. U. (2005). External control of transgene expression in tobacco plastids using the bacterial lac repressor. Plant J. 43, 941–946. doi: 10.1111/j.1365-313X.2005.02495.x
Muralikrishna, N., Srinivas, K., Kumar, K. B., Sadanandam, A. (2016). Stable plastid transformation in Scoparia dulcis L. Physiol. Mol. Bio. Plants. 22, 575–581. doi: 10.1007/s12298-016-0386-7
Mustafa, G., Khan, M. S. (2020). Transmission of engineered plastids in sugarcane, a C4 monocotyledonous plant, reveals that sorting of preprogrammed progenitor cells produce heteroplasmy. Plants 10, 26. doi: 10.3390/plants10010026
Nakazato, I., Okuno, M., Itoh, T., Tsutsumi, N., Arimura, S. I. (2023). Characterization and development of a plastid genome base editor, ptpTALECD. Plant J. 115, 1151–1162. doi: 10.1111/tpj.16311
Nakazato, I., Okuno, M., Yamamoto, H., Tamura, Y., Itoh, T., Shikanai, T., et al. (2021). Targeted base editing in the plastid genome of Arabidopsis thaliana. Nat. Plants. 7, 906–913. doi: 10.1038/s41477-021-00954-6
Nakazato, I., Okuno, M., Zhou, C., Itoh, T., Tsutsumi, N., Takenaka, M., et al. (2022). Targeted base editing in the mitochondrial genome of Arabidopsis thaliana. Proc. Natl. Acad. Sci. U.S.A. 119, e2121177119. doi: 10.1073/pnas.212117711
Nakazato, I., Yamori, W., Matsumura, H., Qu, Y., Okuno, M., Tsutsumi, N., et al. (2024). Resistance to the herbicide metribuzin conferred to Arabidopsis thaliana by targeted base editing of the chloroplast genome. Plant Biotechnol. J. 6, 14490. doi: 10.1111/pbi.14490
Narra, M., Kota, S., Velivela, Y., Ellendula, R., Allini, V. R., Abbagani, S. (2018). Construction of chloroplast transformation vector and its functional evaluation in Momordica charantia L. 3 Biotech. 8, 140. doi: 10.1007/s13205-018-1160-z
Nekrasov, V., Staskawicz, B., Weigel, D., Jones, J. D. G., Kamoun, S. (2013). Targeted mutagenesis in the model plant Nicotiana benthamiana using Cas9 RNA guided endonuclease. Nat. Biotechnol. 31, 691–693. doi: 10.1038/nbt.2655
Newkirk, G. M., de Allende, P., Jinkerson, R. E., Giraldo, J. P. (2021). Nanotechnology approaches for chloroplast Biotechnology advancements. Front. Plant Sci. 12. doi: 10.3389/fpls.2021.691295
Nugent, G. D., Coyne, S., Nguyen, T. T., Kavanaghb, T. A., Dix, P. J. (2006). Nuclear and plastid transformation of Brassica oleracea var. botrytis (cauliflower) using PEG-mediated uptake of DNA into protoplasts. Plant Sci. 170, 135–142. doi: 10.1016/j.plantsci.2005.08.020
Nugent, G. D., Ten Have, M., van der Gulik, A., Dix, P. J., Uijtewaal, B. A., Mordhorst, A. P. (2005). Plastid transformants of tomato selected using mutations affecting ribosome structure. Plant Cell Rep. 24, 341–349. doi: 10.1007/s00299-005-0930-3
O'Neill, C., Horváth, G. V., Horváth, E., Dix, P. J., Medgyesy, P. (1993). Chloroplast transformation in plants: polyethylene glycol (PEG) treatment of protoplasts is an alternative to biolistic delivery systems. Plant J. Cell Mol.Biol. 3, 729–738. doi: 10.1111/j.1365-313X.1993.00729.x
Occhialini, A., Pfotenhauer, A. C., Li, L., Harbison, S. A., Lail, A. J., Burris, J. N., et al. (2021). Mini-synplastomes for plastid genetic engineering. Plant Biotechnol. J. 20, 360–373. doi: 10.1111/pbi.13717
Occhialini, A., Piatek, A. A., Pfotenhauer, A. C., Frazier, T. P., Stewart, C. N., Lenaghan, S. C. (2019). MoChlo: A versatile modular cloning toolbox for chloroplast biotechnology. Plant Physiol. 179, 943–957. doi: 10.1104/pp.18.01220
Odahara, M., Horii, Y., Itami, J., Watanabe, K., Numata, K. (2022). Functional peptide-mediated plastid transformation in tobacco, rice, and kenaf. Front. Plant Sci. 13. doi: 10.3389/fpls.2022.989310
Oikawa, K., Tateishi, A., Odahara, M., Kodama, Y., Numata, K. (2021). Imaging of the entry pathway of a cell-penetrating peptide–DNA complex from the extracellular space to chloroplast nucleoids across multiple membranes in Arabidopsis leaves. Front. Plant Sci. 12. doi: 10.3389/fpls.2021.759871
Okumura, S., Sawada, M., Park, Y. W., Hayashi, T., Shimamura, M., Takase, H., et al. (2006). Transformation of poplar (Populus alba) plastids and expression of foreign proteins in tree chloroplasts. Transgenic Res. 15, 637–646. doi: 10.1007/s11248-006-9009-3
Okuzaki, A., Tsuda, M., Konagaya, K. I., Tabei, Y. (2020). A novel strategy for promoting homoplasmic plastid transformant production using the barnase-barstar system. Plant Biotechnol. 37, 223–232. doi: 10.5511/plantbiotechnology.20.0503a
Pasoreck, E. K., Su, J., Silverman, I. M., Gosai, S. J., Gregory, B. D., Yuan, J. S., et al. (2016). Terpene metabolic engineering via nuclear or chloroplast genomes profoundly and globally impacts off-target pathways through metabolite signalling. Plant Biotechnol. J. 14, 1862–1875. doi: 10.1111/pbi.12548
Pelletier, G., Budar, F. (2007). The molecular biology of cytoplasmically inherited male sterility and prospects for its engineering. Curr. Opin. Biotechnol. 18, 121–125. doi: 10.1016/j.copbio.2006.12.002
Plader, W., Sugiura, M. (2003). The Shine-Dalgarno-like sequence is a negative regulatory element for translation of tobacco chloroplast rps2 mRNA: an additional mechanism for translational control in chloroplasts. Plant J. 34, 377–382. doi: 10.1046/j.1365-313x.2003.01732.x
Ren, B. L., Cao, J. N., He, Y. Q., Yang, S., Zhang, J. (2021a). Assessment on effects of transplastomic potato plants expressing colorado potato beetle β-actin double-stranded RNAs for three non-target pests. Pestic. Biochem. Physiol. 178, 104909. doi: 10.1016/j.pestbp.2021.104909
Ren, Q., Sretenovic, S., Liu, G., Zhong, Z., Wang, J., Huang, L., et al. (2021b). Improved plant cytosine base editors with high editing activity, purity, and specificity. Plant Biotechnol. J. 19, 2052–2068. doi: 10.1111/pbi.13635
Ren, K., Xu, W., Ren, B., Fu, J., Jiang, C., Zhang, J. (2022). A simple technology for plastid transformation with fragmented DNA. J. Exp. Bot. 73, 6078–6088. doi: 10.1093/jxb/erac256
Rigano, M. M., Manna, C., Giulini, A., Pedrazzini, E., Capobianchi, M., Castilletti, C., et al. (2009). Transgenic chloroplasts are efficient sites for high-yield production of the vaccinia virus envelope protein A27L in plant cellsdagger. Plant Biotechnol. J. 7, 577–591. doi: 10.1111/j.1467-7652.2009.00425.x
Rojas, M., Yu, Q., Williams-Carrier, R., Maliga, P., Barkan, A. (2019). Engineered PPR proteins as inducible switches to activate the expression of chloroplast transgenes. Nat. Plants. 5, 505–511. doi: 10.1038/s41477-019-0412-1
Ruf, S., Forner, J., Hasse, C., Kroop, X., Seeger, S., Schollbach, L., et al. (2019). High-efficiency generation of fertile transplastomic Arabidopsis plants. Nat. Plants. 5, 282–289. doi: 10.1038/s41477-019-0359-2
Ruf, S., Hermann, M., Berger, I. J., Carrer, H., Bock, R. (2001). Stable genetic transformation of tomato plastids and expression of a foreign protein in fruit. Nat. Biotechnol. 19, 870–875. doi: 10.1038/nbt0901-870
Ruiz, O. N., Alvarez, D., Torres, C., Roman, L., Daniell, H. (2011). Metallothionein expression in chloroplasts enhances mercury accumulation and phytoremediation capability. Plant Biotechnol. J. 9, 609–617. doi: 10.1111/j.1467-7652.2011.00616.x
Ruiz, O. N., Daniell, H. (2005). Engineering cytoplasmic male sterility via the chloroplast genome by expression of {beta}-ketothiolase. Plant Physiol. 138, 1232–1246. doi: 10.1104/pp.104.057729
Sakamoto, W., Sturm, N. R., Kindle, K. L., Stern, D. B. (1994). petD mRNA maturation in Chlamydomonas reinhardtii chloroplasts: role of 5' endonucleolytic processing. Mol. Cell. Biol. 14, 6180–6186. doi: 10.1128/mcb.14.9.6180-6186.1994
Sanford, J. C. (1990). Biolistic plant transformation. Physiol. Plant 79, 206–209. doi: 10.1111/j.1399-3054.1990.tb05888.x
Santana, I., Wu, H., Hu, P., Giraldo, J. P. (2020). Targeted delivery of nanomaterials with chemical cargoes in plants enabled by a biorecognition motif. Nat. Commun. 11, 2045. doi: 10.1038/s41467-020-15731-w
Satoh, J., Baba, K., Nakahira, Y., Tsunoyama, Y., Shiina, T., Toyoshima, Y. (1999). Developmental stage-specific multi-subunit plastid RNA polymerases (PEP) in wheat. Plant J. 18, 407–415. doi: 10.1046/j.1365-313X.1999.00465.x
Scharff, L. B., Bock, R. (2014). Synthetic biology in plastids. Plant Mol. Biol. 78, 783–798. doi: 10.1111/tpj.12356
Scotti, N., Alagna, F., Ferraiolo, E., Formisano, G., Sannino, L., Buonaguro, L., et al. (2009). High-level expression of the HIV-1 Pr55gag polyprotein in transgenic tobacco chloroplasts. Planta 229, 1109–1122. doi: 10.1007/s00425-009-0898-2
Shiina, T., Allison, L., Maliga, P. (1998). rbcL transcript levels in tobacco plastids are independent of light: reduced dark transcription rate is compensated by increased mRNA stability. Plant Cell 10, 1713–1722. doi: 10.1105/tpc.10.10.1713
Shiina, T., Tsunoyama, Y., Nakahira, Y., Khan, M. S. (2005). Plastid RNA polymerases, promoters, and transcription regulators in higher plants. Int. Rev. Cytol. 244, 1–68. doi: 10.1016/S0074-7696(05)44001-2
Sidorov, V. A., Kasten, D., Pang, S. Z., Hajdukiewicz, P. T., Staub, J. M., Nehra, N. S. (1999). Technical Advance: Stable chloroplast transformation in potato: use of green fluorescent protein as a plastid marker. Plant J. Cell Mol. Biol. 19, 209–216. doi: 10.1046/j.1365-313x.1999.00508.x
Sikdar, S., Serino, G., Chaudhuri, S., Maliga, P. (1998). Plastid transformation in Arabidopsis thaliana. Plant Cell Rep. 18, 20–24. doi: 10.1007/s002990050525
Singh, A. K., Verma, S. S., Bansal, K. C. (2010). Plastid transformation in eggplant (Solanum melongena L.). Transgenic Res. 19, 113–119. doi: 10.1007/s11248-009-9290-z
Skarjinskaia, M., Svab, Z., Maliga, P. (2003). Plastid transformation in Lesquerella fendleri, an oilseed Brassicacea. Transgenic Res. 12, 115–122. doi: 10.1023/A:1022110402302
Sriraman, P., Silhavy, D., Maliga, P. (1998). The phage-type PclpP-53 plastid promoter comprises sequences downstream of the transcription initiation site. Nucleic Acids Res. 26, 4874–4879. doi: 10.1093/nar/26.21.4874
Staub, J. M., Garcia, B., Graves, J., Hajdukiewicz, P. T., Hunter, P., Nehra, N., et al. (2000). High-yield production of a human therapeutic protein in tobacco chloroplasts. Nat. Biotechnol. 18, 333–338. doi: 10.1038/73796
Staub, J. M., Maliga, P. (1994a). Extrachromosomal elements in tobacco plastids. Proc. Natl. Acad. Sci. U.S.A. 91, 7468–7472. doi: 10.1073/pnas.91.16.7468
Staub, J. M., Maliga, P. (1994b). Translation of psbA mRNA is regulated by light via the 5′-untranslated region in tobacco plastids. Plant J. 6, 547–553. doi: 10.1046/j.1365-313x.1994.6040547.x
Staub, J. M., Maliga, P. (1995). Expression of a chimeric uidA gene indicates that polycistronic mRNAs are efficiently translated in tobacco plastids. Plant J. Cell Mol. Biol. 7, 845–848. doi: 10.1046/j.1365-313x.1995.07050845.x
Stegemann, S., Keuthe, M., Greiner, S., Bock, R. (2012). Horizontal transfer of chloroplast genomes between plant species. Proc. Natl. Acad. Sci. U.S.A. 109, 2434–2438. doi: 10.1073/pnas.1114076109
Stern, D. B., Gruissem, W. (1987). Control of plastid gene expression: 3' inverted repeats act as mRNA processing and stabilizing elements, but do not terminate transcription. Cell 24, 1145–1157. doi: 10.1016/0092-8674(87)90600-3
Stern, D. B., Higgs, D. C., ang, J. (1997). Transcription and translation in chloroplasts. Trends Plant Sci. 2, 308–315. doi: 10.1016/S1360-1385(97)89953-0
Su, J., Sherman, A., Doerfler, P. A., Byrne, B. J., Herzog, R. W., Daniell, H. (2015). Oral delivery of acid alpha glucosidase epitopes expressed in plant chloroplasts suppresses antibody formation in treatment of Pompe mice. Plant Biotechnol. J. 13, 1023–1032. doi: 10.1111/pbi.12413
Suzuki, J. Y., Sriraman, P., Svab, Z., Maliga, P. (2003). Unique architecture of the plastid ribosomal RNA operon promoter recognized by the multisubunit RNA polymerase in tobacco and other higher plants. Plant Cell 15, 195–205. doi: 10.1105/tpc.007914
Svab, Z., Hajdukiewicz, P., Maliga, P. (1990). Stable transformation of plastids in higher plants. Proc. Natl. Acad. Sci. U. S. A. 87, 8526–8530. doi: 10.1073/pnas.87.21.8526
Svab, Z., Maliga, P. (1993). High-frequency plastid transformation in tobacco by selection for a chimeric aadA gene. Proc. Natl. Acad. Sci. U.S.A. 90, 913–917. doi: 10.1073/pnas.90.3.913
Tabatabaei, I., Ruf, S., Bock, R. (2017). A bifunctional aminoglycoside acetyltransferase/phosphotransferase conferring tobramycin resistance provides an efficient selectable marker for plastid transformation. Plant Mol. Bio. 93, 269–281. doi: 10.1007/s11103-016-0560-x
Tamburino, R., Castiglia, D., Marcolongo, L., Sannino, L., Ionata, E., Scotti, N. (2023). Tobacco plastid transformation as production platform of lytic polysaccharide monoOxygenase auxiliary enzymes. Int. J. Mol. Sci. 24, 309. doi: 10.3390/ijms24010309
Tan, J., Forner, J., Karcher, D., Bock, R. (2022). DNA base editing in nuclear and organellar genomes. Trends Genet. 38, 1147–1169. doi: 10.1016/j.tig.2022.06.015
Tang, N., Xia, Y., Zhan, Y., Dan, J., Yu, M., Bu, X., et al. (2020). Improvement of chloroplast transformation using CRISPR/Cas9. J. Biobased Mater. Bio. 14, 401. doi: 10.1166/jbmb.2020.1970
Tangphatsornruang, S., Birch-Machin, I., Newell, C. A., Gray, J. C. (2011). The effect of different 3′ untranslated regions on the accumulation and stability of transcripts of a gfp transgene in chloroplasts of transplastomic tobacco. Plant Mol. Biol. 76, 385–396. doi: 10.1007/s11103-010-9689-1
Thagun, C., Chuah, J. A., Numata, K. (2019). Targeted gene delivery into various plastids mediated by clustered cell-penetrating and chloroplast-targeting peptides. Adv. Sci. (Weinheim Baden-Wurttemberg Germany) 6, 1902064. doi: 10.1002/advs.201902064
Thagun, C., Horii, Y., Mori, M., Fujita, S., Ohtani, M., Tsuchiya, K., et al. (2022). Non-transgenic gene modulation via spray delivery of nucleic acid/peptide complexes into plant nuclei and chloroplasts. ACS Nano 16, 3506–3521. doi: 10.1021/acsnano.1c07723
Thum, K. E., Kim, M., Morishige, D. T., Eibl, C., Koop, H.-U., Mullet, J. E. (2001). Analysis of barley chloroplast psbD light-responsive promoter elements in transplastomic tobacco. Plant Mol. Biol. 47, 353–366. doi: 10.1023/a:1011616400264
Thyssen, G., Svab, Z., Maliga, P. (2012). Cell-to-cell movement of plastids in plants. Proc. Natl. Acad. Sci. U. S. A. 109, 2439–2443. doi: 10.1073/pnas.1114297109
Tungsuchat, T., Kuroda, H., Narangajavana, J., Maliga, P. (2006). Gene activation in plastids by the CRE site-specific recombinase. Plant Mol. Biol. 61, 711–718. doi: 10.1007/s11103-006-0044-5
Vafaee, Y., Staniek, A., Mancheno-Solano, M., Warzecha, H. (2014). A modular cloning toolbox for the generation of chloroplast transformation vectors. PloS One 9, e110222. doi: 10.1371/journal.pone.0110222
Valkov, V. T., Gargano, D., Manna, C., Formisano, G., Dix, P. J., Gray, J. C., et al. (2011). High efficiency plastid transformation in potato and regulation of transgene expression in leaves and tubers by alternative 5' and 3' regulatory sequences. Transgenic Res. 20, 137–151. doi: 10.1007/s11248-010-9402-9
Valkov, V. T., Scotti, N., Kahlau, S., Maclean, D., Grillo, S., Gray, J. C., et al. (2009). Genome-wide analysis of plastid gene expression in potato leaf chloroplasts and tuber amyloplasts: transcriptional and posttranscriptional control. Plant Physiol. 150, 2030–2044. doi: 10.1104/pp.109.140483
Venkateswarlu, K., Nazar, R. (1991). Evidence for T–DNA mediated gene targeting to tobacco chloroplasts. Nat. Biotechnol. 9, 1103–1105. doi: 10.1038/nbt1191-1103
Verhounig, A., Karcher, D., Bock, R. (2010). Inducible gene expression from the plastid genome by a synthetic riboswitch. Proc. Nat. Acad. Sci. U.S.A. 107, 6204–6209. doi: 10.1073/pnas.0914423107
Verma, D., Kanagaraj, A., Jin, S., Singh, N. D., Kolattukudy, P. E., Daniell, H. (2010). Chloroplast-derived enzyme cocktails hydrolyse lignocellulosic biomass and release fermentable sugars. Plant Biotechnol. J. 8, 332–350. doi: 10.1111/j.1467-7652.2009.00486.x
Wang, Y., Fan, J., Wei, Z., Xing, S. (2023). Efficient expression of fusion human epidermal growth factor in tobacco chloroplasts. BMC Biotechnol. 23, 1. doi: 10.1186/s12896-022-00771-5
Wang, X., Fang, T., Lu, J., Tripathi, L., Qi, Y. (2024). Broad range plastid genome editing with monomeric TALE-linked cytosine and dual base editors. Plant Biotechnol. J. 22, 2441–2443. doi: 10.1111/pbi.14358
Wang, Y., Wei, Z., Xing, S. (2018). Stable plastid transformation of rice, a monocot cereal crop. Biochem. Biophys. Res. Commun. 503, 2376–2379. doi: 10.1016/j.bbrc.2018.06.164
Wang, Y. P., Wei, Z. Y., Zhong, X. F., Lin, C. J., Cai, Y. H., Ma, J., et al. (2015). Stable expression of basic fibroblast growth factor in chloroplasts of tobacco. Int. J. Mol. Sci. 17, 19. doi: 10.3390/ijms17010019
Willey, D. L., Gray, J. C. (1990). An open reading frame encoding a putative haem-binding polypeptide is contranscribed with the pea chloroplast gene for apocytochrome f. Plant Mol. Biol. 15, 347–356. doi: 10.1007/BF00036920
Wu, M. T., Dong, Y., Zhang, Q., Li, S. C., Chang, L., Loiacono, F. V., et al. (2022). Efficient control of western flower thrips by plastid-mediated RNA interference. Proc. Natl. Acad. Sci. U. S. A. 119, e2120081119. doi: 10.1073/pnas.2120081119
Wu, X. X., Li, F., Zhu, C., Sun, S. Y., Mu, W. H., Ruf, S., et al. (2025). Protocol for the purification of the plastid-encoded RNA polymerase from transplastomic tobacco plants. STAR Prot. 6, 103528. doi: 10.1016/j.xpro.2024.103528
Wu, Y., You, L., Li, S., Ma, M., Wu, M., Ma, L., et al. (2017). In vivo assembly in Escherichia coli of transformation vectors for plastid genome engineering. Front. Plant Sci. 8. doi: 10.3389/fpls.2017.01454
Wurbs, D., Ruf, S., Bock, R. (2007). Contained metabolic engineering in tomatoes by expression of carotenoid biosynthesis genes from the plastid genome. Plant J. Cell Mol. Biol. 49, 276–288. doi: 10.1111/j.1365-313X.2006.02960.x
Yabuta, Y., Tanaka, H., Yoshimura, S., Suzuki, A., Tamoi, M., Maruta, T., et al. (2013). Improvement of vitamin E quality and quantity in tobacco and lettuce by chloroplast genetic engineering. Transgenic Res. 22, 391–402. doi: 10.1007/s11248-012-9656-5
Yan, J., Yao, Y., Hong, S., Yang, Y., Shen, C., Zhang, Q., et al. (2019). Delineation of pentatricopeptide repeat codes for target RNA prediction. Nucleic Acids Res. 47, 3728–3738. doi: 10.1093/nar/gkz075
Ye, G. N., Colburn, S. M., Xu, C. W., Hajdukiewicz, P. T., Staub, J. M. (2003). Persistence of unselected transgenic DNA during a plastid transformation and segregation approach to herbicide resistance. Plant Physiol. 133, 402–410. doi: 10.1104/pp.103.021949
Ye, G. N., Hajdukiewicz, P. T., Broyles, D., Rodriguez, D., Xu, C. W., Nehra, N., et al. (2001). Plastid-expressed 5-enolpyruvylshikimate-3-phosphate synthase genes provide high level glyphosate tolerance in tobacco. Plant J. Cell Mol. Biol. 25, 261–270. doi: 10.1046/j.1365-313x.2001.00958.x
Yoo, B. C., Yadav, N. S., Orozco, E. M., Jr., Sakai, H. (2020). Cas9/gRNA-mediated genome editing of yeast mitochondria and Chlamydomonas chloroplasts. Peer J. 8, e8362. doi: 10.7717/peerj.8362
Yu, Q., Barkan, A., Maliga, P. (2019). Engineered RNA-binding protein for transgene activation in non-green plastids. Nat. Plants. 5, 486–490. doi: 10.1038/s41477-019-0413-0
Yu, Q., Lutz, K. A., Maliga, P. (2017). Efficient plastid transformation in Arabidopsis. Plant Physiol. 175, 186–193. doi: 10.1104/pp.17.00857
Zhang, D., Boch, J. (2023). Development of TALE-adenine base editors in plants. Plant Biotechnol. J. 22, 1067–1077. doi: 10.1111/pbi.14246
Zhang, J., Khan, S. A., Hasse, C., Ruf, S., Heckel, D. G., Bock, R., et al. (2015a). Full crop protection from an insect pest by expression of long double-stranded RNAs in plastids. Science 347, 991–994. doi: 10.1126/science.1261680
Zhang, D., Pries, V., Boch, J. (2024). Targeted C•G-to-T•A base editing with TALE-cytosine deaminases in plants. BMC Biol. 22, 99. doi: 10.1186/s12915-024-01895-0
Zhang, J., Ruf, S., Hasse, C., Childs, L., Scharff, L. B., Bock, R. (2012). Identification of cis-elements conferring high levels of gene expression in non-green plastids. Plant J. 72, 115–128. doi: 10.1111/j.1365-313X.2012.05065.x
Zhou, F., Badillo-Corona, J. A., Karcher, D., Gonzalez-Rabade, N., Piepenburg, K., Borchers, A. M., et al. (2008). High-level expression of human immunodeficiency virus antigens from the tobacco and tomato plastid genomes. Plant Biotechnol. J. 6, 897–913. doi: 10.1111/j.1467-7652.2008.00356.x
Zhou, F., Karcher, D., Bock, R. (2007). Identification of a plastid intercistronic expression element (IEE) facilitating the expression of stable translatable monocistronic mRNAs from operons. Plant J. 52, 961–972. doi: 10.1111/j.1365-313X.2007.03261.x
Zhou, W., Lu, Q., Li, Q., Wang, L., Ding, S., Zhang, A., et al. (2017). PPR-SMR protein SOT1 has RNA endonuclease activity. Proc. Natl. Acad. Sci. U.S.A. 114, E1554–E1563. doi: 10.1073/pnas.1612460114
Zhou, C., Okuno, M., Nakazato, I., Tsutsumi, N., Arimura, S. (2024). Targeted A-to-G base editing in the organellar genomes of Arabidopsis with monomeric programmable deaminases. Plant Physiol. 194, 2278–2287. doi: 10.1093/plphys/kiad678
Keywords: chloroplast transformation, SWNTs, CRISPR, nanotechnology, RNAi, biolistics, synthetic biology, metabolic engineering
Citation: Narra M, Nakazato I, Polley B, Arimura S-i, Woronuk GN and Bhowmik PK (2025) Recent trends and advances in chloroplast engineering and transformation methods. Front. Plant Sci. 16:1526578. doi: 10.3389/fpls.2025.1526578
Received: 12 November 2024; Accepted: 17 March 2025;
Published: 17 April 2025.
Edited by:
Andrew D. L. Nelson, Boyce Thompson Institute (BTI), United StatesReviewed by:
Gireesha Mohannath, Birla Institute of Technology and Science, IndiaJon Y. Suzuki, United States Department of Agriculture (USDA), United States
Copyright © 2025 His Majesty the King in Right of Canada, as represented by the National Research Council Canada. This is an open-access article distributed under the terms of the Creative Commons Attribution License (CC BY). The use, distribution or reproduction in other forums is permitted, provided the original author(s) and the copyright owner(s) are credited and that the original publication in this journal is cited, in accordance with accepted academic practice. No use, distribution or reproduction is permitted which does not comply with these terms.
*Correspondence: Pankaj K. Bhowmik, UGFua2FqLkJob3dtaWtAbnJjLWNucmMuZ2MuY2E=