- 1Department of Environment and Biodiversity, University of Salzburg, Salzburg, Austria
- 2Department of Functional and Evolutionary Ecology, Faculty of Life Sciences, University of Vienna, Vienna, Austria
The cytoplasm of characean internodal cells is characterized by a stationary layer of cortical chloroplast files and a mobile endoplasm moving along subcortical actin bundles. Occasionally, chloroplasts detach from the cortex and are passively carried along with the endoplasmic flow. Previous studies revealed that local irradiation with intense light causes chloroplast bleaching followed by a release into the endoplasm (“window formation”). We found that endoplasmic chloroplasts of Chara australis resettle at the window and align parallel to the streaming direction. The process takes several weeks with neither chloroplast division nor growth of proplastids being involved. Both release and re-attachment are actin-dependent. Resettled chloroplasts showed slightly, but significantly lower maximum quantum efficiency (Fv/Fm) values as compared with control regions. Extremely low Fv/Fm values were measured in chloroplasts at the border of the window even after three months indicating longevity, although with serious damage. In higher plants, a protein complex is responsible for the motility and anchorage of chloroplasts, with CHUP1 (CHLOROPLAST UNUSUAL POSITIONING 1) being an essential part. We discovered a homologous form CaCHUP1, encoding a polypeptide of 1201 amino acids with a calculated molecular mass of about 130 kDa. When transiently expressed in epidermal cells of Nicotiana benthamiana leaves, fluorescently tagged CaCHUP1 localizes to chloroplasts. We assume that CaCHUP1 is involved in the anchorage of chloroplasts and in the polymerization of actin filaments, but not in active movement. Our study revealed that endoplasmic chloroplasts can re-anchor at the cell cortex thereby refilling chloroplast-free regions, which we interpret as a repair mechanism after various kinds of damage. It confirms that chloroplasts use different strategies for repositioning, either via polymerization of cp-actin or via cytoplasmic streaming.
1 Introduction
In higher plants, ferns, mosses and sessile algae, chloroplasts relocate towards illuminated areas within the cell (accumulation response), or to shaded regions if exposed to excess light (avoidance response; e. g. Wada and Kong, 2018). Chloroplast movement thereby ensures efficient photosynthesis under various light conditions and prevents photodamage (e.g. Kasahara et al., 2002). With few exceptions, both accumulation and avoidance responses depend on the actin cytoskeleton and associated proteins (Kong et al., 2024; Oikawa et al., 2003, 2008; Suetsugu and Wada, 2016; Suetsugu et al., 2010, 2017; Wada and Kong, 2018, 2019; Yamamoto-Negi et al., 2024). Actin-dependent chloroplast movements have also been described for cold-treated (Kimura and Kodama, 2016) and for injured cells (Gamalei et al., 1994).
The chloroplasts of characean algae share properties with those of vascular plants to which they are closely related (Delwiche and Cooper, 2015; Domozych and Bagdan, 2022; McCourt et al., 2017; Turmel et al., 2013 for review). However, they are immobilized at the cell periphery and are unable to relocate actively in response to changing light or temperature via polymerization of actin filaments. Stromules (Natesan et al., 2009) have also not yet been described in characean algae.
We investigated the repair of the cortical chloroplast layer in the Chara australis R.Brown after strong local irradiation. The internodal cells of C. australis have a cylindrical shape and are up to several cm long (Figures 1A, B). The cells have been proved as a valuable experimental system to study various aspects of cell biology, including ion transport and electrophysiology (reviewed by e.g. Beilby and Bisson, 2012; Beilby, 2016; Beilby and Casanova, 2014), cell organization and organelle movements (reviewed by Foissner and Wasteneys, 2012, 2014). The immobilization of chloroplasts in the cortex allows to study interactions between chloroplasts, and between chloroplasts and the plasma membrane (e. g. Bulychev and Komarova, 2014; Bulychev, 2020). Although the genome of Chara braunii has been published (Nishiyama et al., 2018) and the subcellular proteome of Chara australis internodal cells has been analyzed (Pertl-Obermeyer et al., 2018), the molecular biology of the Characeae is still at its infancy (Domozych and Bagdan, 2022; Holzhausen et al., 2022; Kurtović et al., 2024).
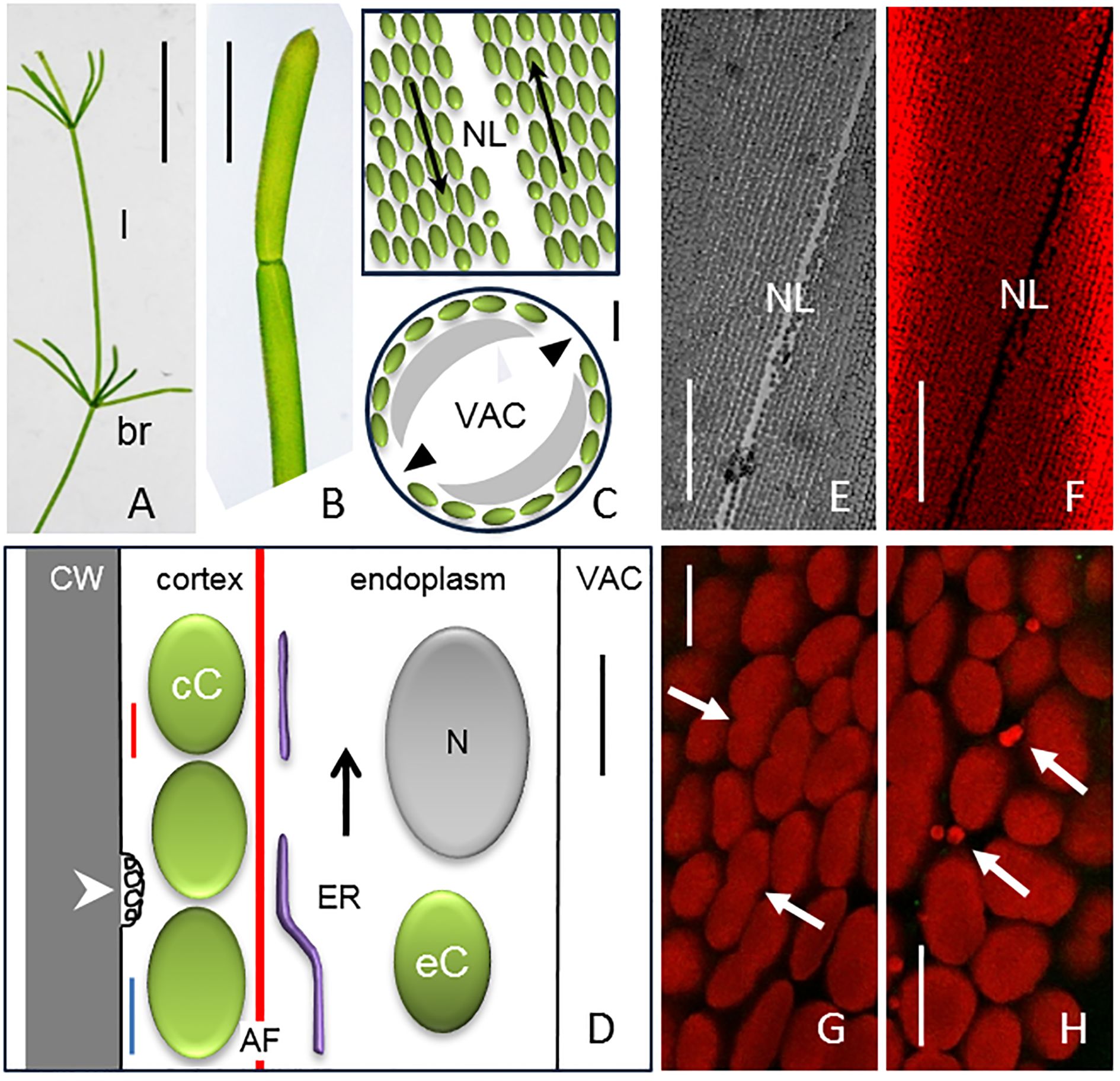
Figure 1. Thallus, organization of internodal cell and cortical chloroplasts. (A) Thallus of Chara australis (I = internodal cell of main axis, br = branchlet). (B) Distal and part of proximal branchlet internodal cell. (C, D) Schematic drawings of internodal cell organization (compare Foissner and Wasteneys, 2012). (C) Stationary chloroplasts (green) are arranged in helical files (tangential view, upper image). Opposite streams of endoplasm (arrows) are separated by the neutral line (NL and arrow heads in the cross-section; lower image). The grey areas in the cross section represent the endoplasm; VAC = central vacuole. (D) Fine structure of internodal cell (longitudinal section). The cortex mainly consists of stationary (cortical) chloroplasts (cC). Actin filaments (thin red line) and microtubules (blue line) are located at the plasma membrane which may by convoluted to charasomes (arrow head). Subcortical actin bundles (AF; thick red line) are attached to the inner side of the chloroplasts and generate endoplasmic streaming by interaction with myosin coated endoplasmic reticulum (ER). The endoplasm contains nuclei (N), endoplasmic chloroplasts (eC) and various other organelles (not shown). VAC = central vacuole. (E–H) Bright field image (E) and fluorescence images [(F–H); single optical sections] of chloroplasts in a mature, non-elongating branchlet cell. Note dumbbell-shaped chloroplasts [arrows in (G)] and tiny organelles [arrows in (H)] in the higher magnifications. Bar = 1.5 cm (A), 300 µm (B), 5 µm (C, D), 100 µm (E, F), 10 µm (G, H). Schematic images are only approximately drawn to scale.
The cytoplasm of Chara internodal cells is partitioned into a stationary cortex and a mobile endoplasm, which performs rotational streaming (Figures 1C, D; for references see Foissner and Wasteneys, 2014). The tangential (upper image) and the cross-section (lower image) in Figure 1C illustrate that chloroplasts are organized as files which have been shown to follow the helical strain pattern of the cell wall (Green, 1964). Up- and down-streaming endoplasm is separated by the neutral line (NL and arrow heads in Figure 1C). The cortex consists of files of immobile (cortical) chloroplasts (cC in Figure 1D); cortical actin filaments and microtubules are located at the plasma membrane which contains convoluted domains (“charasomes”) that play a role in ion transport (arrow head in Figure 1D; for references see Absolonova et al., 2018). Subcortical actin bundles consisting of up to 100 filaments are attached to the inner side of the cortical chloroplast and generate endoplasmic streaming by interaction with myosin-coated cisternae of the endoplasmic reticulum (ER; Figure 1D). The endoplasm also contains numerous nuclei (N), mitochondria, Golgi bodies, multivesicular bodies and other organelles. Cortical chloroplasts occasionally detach from the cortex and are then carried passively with endoplasmic flow (eC in Figure 1D). They may, however, perform active, myosin-dependent rotational movements, if actin bundles (rings) are attached to their surface (Jarosch, 1956; Wasteneys et al., 1996). Actin rings are occasionally present at chloroplasts anchored at the neutral line (Wasteneys et al., 1996). A huge vacuole (VAC) occupies the center of the cells.
Chloroplasts can be removed by various treatments, e. g. mechanical damage (Chen, 1983), chemicals which interfere with the Ca2+ metabolism (Foissner, 1988; 1990) and by local irradiation with strong light (Kamitsubo, 1972). The latter method was successfully used to study the regeneration of the actin and microtubule cytoskeleton in these chloroplast-free areas called “windows” (Williamson and Hurley, 1986; Williamson et al., 1984; Foissner and Wasteneys, 2012). The sequence of events that have been described so far during and after local irradiation are (1) the bleaching and rounding up of cortical chloroplasts, (2) the retardation or even arrest of endoplasmic streaming, (3) the dissolution of cortical actin filaments, microtubules and (possibly) subcortical actin bundles, (4) the detachment of bleached chloroplasts and their release into the streaming endoplasm (“window formation”). The recovery process includes (5) the regeneration of the subcortical actin bundles including recovery of active cytoplasmic streaming, and (6) the reappearance of microtubules except those of cortical actin filaments (see review by Foissner and Wasteneys, 2012). Longer irradiation induces the secretion of a wound wall, which requires a three-dimensional actin meshwork for the transport of wall-forming vesicles (Foissner and Wasteneys, 1997).
In the current study, we aimed to discover potential repair mechanisms in windows. We assumed either a refilling through dividing cortical chloroplasts or via a resettlement of endoplasmic chloroplasts. We also searched for an explanation at the molecular level, which could be e.g. the CHUP1 protein, which is required for photo-relocation of chloroplasts in vascular plants (Kong et al., 2024). The outcome of this study is also of relevance for plants living in their natural habitat. The organisms face various challenges in the environment; repair mechanisms of mechanical and radiation damage are indispensable for their survival.
2 Materials and methods
2.1 Algal material and culture conditions
Male thalli of Chara australis R.Brown were grown at room temperature in 10-50 L containers in a mixed substrate of soil, peat and sand covered by distilled water. Fluorescent lamps provided a photon flux density of 5 μmol·m−2·s−1 photosynthetically active radiation (PAR) at the water surface for 14 hours per day. The maximum age of cultures used for experiments was two months. Internodal cells of lateral branchlets (axis with limited growth) were isolated from the 2nd or 3rd node of the thallus and stored in artificial fresh water (AFW, 0.1 mM NaCl, 0.1 mM KCl, 1 mM CaCl2; unbuffered pH 5.6) until use.
2.2 Inhibitor treatments and staining
The involvement of the cytoskeleton in chloroplast detachment and resettlement was investigated using specific inhibitors. The actin inhibitor cytochalasin D reversibly arrests endoplasmic streaming in characean internodal cells and was used at concentrations of 10 and 20 µM. Oryzalin depolymerizes microtubules and paclitaxel inhibits the dynamics of microtubules and stabilizes microtubules. Both oryzalin and paclitaxel were applied at concentrations of 8 and 10 µM, respectively. Stock solutions of cytochalasin D (Sigma, 10 mM in DMSO), oryzalin (Riedel-de Haen, 10 mM in DMSO) and paclitaxel (Molecular probes, 2 mM in DMSO) were diluted with AFW; controls contained the equivalent concentration of DMSO. Inhibitor and control solutions were prepared freshly for short time experiments or were replaced every week for long term experiments.
Actin was labelled by perfusion of cells with Alexa Fluor 488 phalloidin (Invitrogen) as described in Foissner and Wasteneys (2007).The 6.6 µM stock solution in methanol was dissolved in perfusion solution (200 mM sucrose, 70 mM KCl, 4.49 mM MgCl2, 5 mM EGTA, 1.48 mM CaCl2, 10 mM Pipes, pH 7). Images were taken 5-20 min after dye loading.
2.3 Light microscopy and window formation
Chloroplast resettlement at windows depended on the presence of endoplasmic chloroplasts (compare Figures 2A–C). We therefore counted the relative number of these chloroplasts before irradiation using consecutive, non-overlapping images of the endoplasm and assuming an endoplasm thickness of 5 µm. Windows were produced in cells with at least 1 chloroplast per 50 000 µm3 endoplasm.
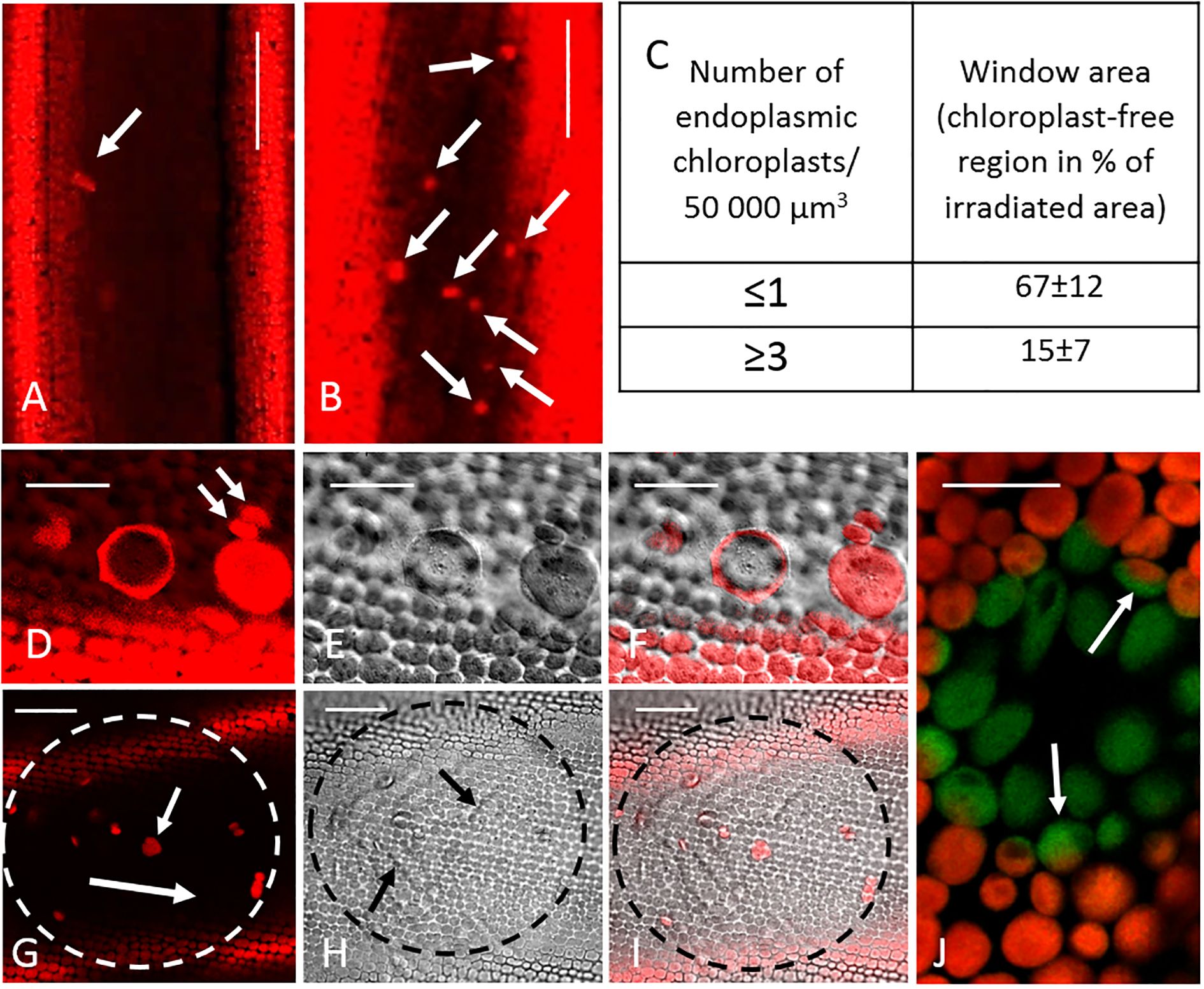
Figure 2. Endoplasmic chloroplasts and window formation. (A, B) Endoplasmic chloroplasts (arrows) in two cells. Note different abundance (0.3 and 3 chloroplasts/50000 µm3 endoplasm, respectively, for these images). (C) Recovery of the cortical chloroplast layer depends on the presence of endoplasmic chloroplasts. Cells were examined 4 weeks after irradiation and the remaining (non-healed) window area was calculated. Data are means ± SD, and are significantly different (t-test; P ≤ 0.005; 14 windows (cells) from 3 different thalli were investigated). (D–F) Endoplasmic chloroplasts at the neutral line. The left giant chloroplast is slightly cup-shaped and has a polygonal outline; the right giant chloroplast is disc-shaped. Endoplasmic chloroplasts with normal size are indicated by arrows. Cortical chloroplasts are seen at the lower area of the images. (G–I) Local irradiation (circled area) with sufficient energy and duration causes bleaching of cortical chloroplasts and disturbance of endoplasmic streaming. Images were taken 1 hour after irradiation. Note bright fluorescence of cortical chloroplasts outside the window and of moving endoplasmic chloroplasts located in the inner layer). The long arrow in G indicates the streaming direction, the short arrow points at an endoplasmic chloroplast. Arrows in H indicate bulges (uneven distribution of endoplasm beneath the window). (J) Bleached chloroplasts in a small window. Note partially bleached chloroplasts (arrows) at the border of the window. (A, B, D, G, J) fluorescence of chloroplasts); (E, H) bright field images, (F, I) merged images. All images are single optical sections. Bar = 100 µm (A, B) 40 µm (G–I), 20 µm (D–F) and 10 µm (J).
Windows with a roundish or rectangular shape and a size between 10 000 to 40 000 µm2 were produced by local irradiation with a 50 or 100 W mercury lamp or a halide lamp (highest intensity) guided through blue light emitting fluorescence filter cubes (emission 450-490 nm) and using 40x lenses (numerical apertures between 0.7 and 0.85) of various fluorescence microscopes. The light intensity at the surface of the cell was about 4000 μmol.m−2.s−1 PAR (MC-MQS/OVV Microscopy Quantum Sensor, Walz, Effeltrich, Germany). The irradiation time required to retard streaming and to induce the detachment of chloroplasts mainly depended on the light intensity of the respective lamp and on the growth status of cells. At the provided conditions and with the cells used, irradiation for 1.5-6.0 min was sufficient for release of cortical chloroplasts within one day.
Bright field and fluorescent images were obtained using either a Leica TCS SP5 confocal laser scanning microscope (CLSM) coupled to a DMI 6000B inverted microscope (Mannheim, Germany), or a Zeiss LSM 510 coupled to an Axiovert inverted microscope (Jena, Germany). The red chlorophyll fluorescence was detected between 637 and 750 nm (Leica; tunable detection) or beyond 585 nm using a long pass filter (Zeiss). The fluorescence of Alexa Fluor 488 phalloidin was recorded between 500 and 573 nm. Bright field images of chloroplasts were also taken using a digital color camera (Canon EOS) attached to a Leitz Dialux 20 compound microscope.
CLSM images shown in this study are either single optical sections or projections of Z-stacks processed using Leica or Zeiss software and GIMP (GNU Image Manipulation Program; https://www.gimp.org).
2.4 Electron microscopy
Branchlet internodal cells were chemically fixed with 1% glutaraldehyde for 20 min at room temperature, followed by post-fixation with 2% OsO4 for 12 h at 4°C. Samples were dehydrated with ethanol, then transferred to propylene oxide and embedded in Agar low viscosity resin (Agar Scientific, Rotherham, UK) (Foissner et al., 2016). Part of the samples were also high-pressure frozen and cryo-substituted as described in Lütz-Meindl and Aichinger (2004), followed by dehydration with ethanol, transfer to propylene oxide and embedding in LR Gold (London Resin, Reading, UK).
2.5 Pulse amplitude modulation fluorescence
To get insight into the photosynthetic performance of PSII in resettled chloroplasts and in control regions for a comparison, fluorescence yields were measured and visualized by means of an imaging PAM fluorescence device (Imaging PAM M‐Series, Microscopy version, Walz company, Effeltrich, Germany). We measured the maximum efficiency of PSII during low light of 20 µmol photons m-2. s-1 (Fv’/Fm’ LL) and high light of 60 µmol photons m-2. s-1 (Fv’/Fm’ HL). Additionally, maximum quantum efficiency of PSII (Fv/Fm) was measured after 5 min of dark acclimation of cells. Fv/Fm is defined as Fv/Fm =(Fm − F0)/Fm (Maxwell and Johnson, 2000) and provides information about the overall photosynthetic performance; a reduced Fv/Fm indicates different types of plant stress. Fm represents the maximum dark fluorescence after a strong flash of light (all PSII reduced), Fm’ is the maximum fluorescence in ambient light. F0 is the minimum fluorescence in the dark (all PSII oxidized), F0’ the minimum fluorescence immediately after switching off ambient light; Fv is called variable fluorescence during darkness, Fv’ variable fluorescence during light exposure. We compared the development of photosynthetic performance of various regions over several weeks: upstream and downstream regions of the window (controls), borders of the window and the window itself.
2.6 Statistical analysis
Chloroplast numbers and areas were calculated using ImageJ (https://imagej.nih.gov). Diagrams were produced using Sigma Plot V14.5 (https://systatsoftware.com). Mann-Whitney-U tests were calculated to test significant effects of inhibitors oryzalin and paclitaxel. For cytochalasin D, a general linear mixed model (GLMM) was applied with window area taken as dependent variable, time and treatment set as fixed factors, and the replicates as fixed random factors. T-test was used after the normal distribution was checked with Shapiro-Wilk test. Data were obtained from different cells collected from at least 3 thalli.
PAM fluorescence data were analyzed with GLMM. Yield was taken as dependent variable, time and area were set as fixed factors, and the replicates as fixed random factors. For each region, 16 cells (replicates) from whorls of 5 thalli were repeatedly measured at the start of the experiment, after 1, 2, 3, 7, 10, 17, 29 and 79 days (end of the experiment). Statistics were performed with the jamovi package V2.3.28.0 (https://www.jamovi.org), jmw and GAMLj suite (https://gamlj.github.io/) installed.
2.7 Cloning and protein analyses
Arabidopsis CHUP1 protein (GenBank accession number: OAP01771) was used as a template to screen for homologous sequences in the transcriptomic database of Chara australis described in Pertl-Obermeyer et al. (2018). To verify the obtained CHUP1-like open reading frame from CL5265.Contig1_All, fresh total RNA from C. australis thalli was extracted using TRI-Reagent according to the manufacturer instructions (Sigma-Aldrich - Merck, Darmstadt, Germany). Residual genomic DNA was digested by RNase-free DNase (EN0521, Thermo Fischer Scientific, Waltham, MA, USA) and first-strand cDNA was synthesized from 1 μg total RNA by M-MuLV Reverse Transcriptase (RevertAid; EP0441, Thermo Fischer Scientific) using an anchored oligo(d)T primer-mix according to the supplier’s protocol. The obtained cDNA was used as a template for PCR amplification. All PCRs were performed with Phusion High-Fidelity DNA polymerase (F530S, Thermo Fischer Scientific) according to the manufacturer’s instructions and verified by sequencing. CaCHUP1 was cloned using the primers CL5265_fwd (5’-ATGTGGGTTCGATTCGCCCTCA-3’) and CL5265_rev (5’-CTACTGCTTGGCGGCGGGAGATT-3’). The amplicon (3606 base pairs) was sub-cloned into pJet1.2 cloning vector (Thermo Fischer Scientific, #K1231). The sequence vas verified by sequencing and named Chara australis CHLOROPLAST UNUSUAL POSITIONING 1 (CaCHUP1). The expression vector pGI-AtUBQ10p::CaCHUP1::GFP was created using the Hot-Fusion protocol as described in (Fu et al., 2014). This process involved our pGI-AtUBQ10p::GFP vector (described in Hoepflinger et al., 2013), the primers AtUBQ10p_CaCHUP1_HF_F (5’-AGGTCGACGGTATCGATAATGTGGGTTCGATTCGCCCTCA-3’) and CaCHUP1_GFP_HF_R (5’-TTCTCCTTTACTCATCCCCTGCTTGGCGGCGGGAGATT-3’) as well as the restriction enzyme sites SmaI and HindIII. The resulting expression vector pGI-AtUBQ10p::CaCHUP1::GFP contained an A. thaliana ubiquitin-10 promoter (AtUBQ10p; At4g05320), CaCHUP1 and a C-terminally fused GFP. This vector was transformed into Agrobacterium tumefaciens strain GV3101 (harboring the pSoup helper plasmid) and transiently expressed in Nicotiana benthamiana leaves as described in detail in Hoepflinger et al. (2013). Briefly, four-weeks old N. benthamiana plants, grown on standard fertilized soil (type ED73) in a growth chamber (16/8 h light/dark cycle at 22°C to 23°C, and a relative humidity of 60%), were used for Agrobacterium-mediated transient protein expression. A. tumefaciens carrying either CaCHUP1::GFP or a P19 repressor were grown to late-exponential phase (about 16 h) in liquid YEB medium. Cultures were harvested by centrifugation at room temperature (6000g, 5 min) and the resulting pellets were resuspended in infiltration buffer [10 mM MES/KOH (pH 5.6), 10 mM MgCl2, 150 μM acetosyringone]. Bacterial concentration was determined photometrically and both suspensions were combined to a final concentration of OD600 = 0.5 for A. tumefaciens carrying CaCHUP1::GFP, and OD600 = 0.25 for A. tumefaciens carrying the P19 repressor. After 2 h of incubation at room temperature, N. benthamiana leaves were infiltrated with the described suspension using a 1 ml syringe without needle. Tobacco plants were replaced to the growth chamber and examined after 3 to 4 days of expression. All obtained sequences and constructs were verified by sequencing. CaCHUP1 GenBank accession number PQ306591.
Clustal Omega (EMBL-EBI) was used for protein sequence alignments and conserved domains were detected using InterPro (Mitchell et al., 2014). The following sequences were compared: Charophytes: Chara australis CHUP1 (this paper), Chara braunii hypothetical protein CBR_g4598 (GenBank: GBG69767), Klebsormidium nitens CHUP1B (GenBank: GAQ78600); liverwort: Marchantia paleacea hypothetical protein Mapa_014704 (GenBank: KAG6543864); moss: Physcomitrium patens CHUP1 isoform X1 (NCBI Reference Sequence: XP_024379808); fern: Adiantum capillus-veneris CHUP1A (GenBank: BAG54845); eudicot: Arabidopsis thaliana CHUP1 (GenBank: OAP01771); Amino acid sequence identities were calculated with the bioinformatic software CloneManager (http://www.scied.com/).
For western blot analysis, thalli of C. australis were homogenized in liquid nitrogen using mortar and pestle. 2.5 volumes of ice cooled extraction buffer (100 mM NaH2PO4 (pH 7.8), 100 mM KCl, 1 mM DTT, 0.1% Tween) were added and the suspension was shaken for 15 min on ice followed by centrifugation (15 min, 7500 × g, 4°C). The supernatant was separated on a 10% acrylamide separation gel. Proteins were blotted onto a polyvinylidene difluoride membrane (PVDF; Merck, Darmstadt, Germany) for 70 min at 100 V. The membrane was blocked for 1.5 h in TBST-BSA (1% BSA, w/v) at room temperature followed by 1.25 h in TBST-BSA with 1:1000 dilution of α-CaCHUP1 antibody (affinity purified antiserum derived from rabbit, 0.5 µg/ml from Davids Biotechnology GmbH, Regensburg, Germany). The PVDF membrane was washed three times for 5 min each in TBST. The secondary antibody, a monoclonal anti-rabbit IgG coupled to alkaline phosphatase (Sigma-Aldrich), was used at a concentration of 1:20,000 in TBST for 1 h. After another wash step (3 × 5 min in TBST), protein labelling was detected using Tropix® CDP-Star (T2146, Thermo Fischer Scientific) and the LAS 3000 mini-imaging system (Fujifilm, Tokyo, Japan).
3 Results
3.1 Cortical and endoplasmic chloroplasts
Figure 1 shows a thallus of Chara australis (Figure 1A), two adjacent internodal cells of a branchlet (Figure 1B), and schematic images of the organization of internodal cells (Figures 1C, D; see introduction for details).
Bright field and fluorescence images of cortical chloroplast files in a fully grown branchlet cell are shown in Figures 1E, F. Most chloroplasts had an ovate to elongate shape with lengths ranging between 5 and 11 µm, but dumbbell-shaped chloroplasts or chloroplasts with division furrows smaller roundish organelles were also present (Figure 1G, arrows). Dot-like organelles (diameter up to 2.4 µm) with typical chlorophyll autofluorescence, are also seen (Figure 1H, arrows).
Chloroplasts were occasionally released from the cortex and moved passively along with endoplasmic flow, which was up to 100 µm s-1 in our experiments (Supplementary Video 1). Their abundance varied considerably (Figures 2A, B). Our previous observations indicated that the presence of endoplasmic chloroplasts is a prerequisite for the resettlement of the chloroplast layer at windows (Figure 2C). Most endoplasmic chloroplasts were comparable in size and shape to those found in the cortex, but significantly larger, disk-like or broadly ellipsoidal giant chloroplasts with diameters up to 32 µm (Figures 2D–F) and chloroplasts with division furrows could also be observed. Within the streaming endoplasm, many chloroplasts additionally performed active rotational movement (Supplementary Video 2) due to the presence of actin rings at their surface (see below).
3.2 Release of chloroplasts after local irradiation with intense blue light
Cells were irradiated with intense blue light until the endoplasm became unevenly distributed (Figures 2G–I) due to local retardation; sometimes active streaming even stopped. The endoplasm then moved passively and with slower velocities through the irradiated area due to delivery from undisturbed regions (Supplementary Video 3; Supplementary Figures 1A-C). The irradiated, bleached cortical chloroplasts (Figure 2J) became roundish, performed wiggling or trembling motions (Supplementary Video 4), detached from the cell periphery, and were removed with the endoplasm during the following hours (Supplementary Figures 2A, B). They were mostly released in groups, which remained visible up to several weeks after window formation, without signs of further degradation. Chloroplasts at the border of the window were sometimes partly bleached (arrows in Figure 2J). During irradiation, the red chlorophyll fluorescence decreased, and the green fluorescence of chloroplasts became distinct.
Electron micrographs show chloroplasts in the control regions (Figures 3A-C) and at the border of a window (Figure 3D). In control regions, the chloroplast surface is closely attached to the plasma membrane (Figure 3A), but not always along its whole length. Occasionally, the putative attachment or at least the contact sites are zipped out (arrows in Figure 3B). Close contact is also observed between chloroplasts and charasomes (convoluted domains of the plasma membrane; asterisks in Figures 3B, C), and between chloroplasts and cisternae of the cortical ER (arrow in Figure 3C). The regions between the contact sites are occupied by mitochondria and other organelles (Figures 3A–C). The inset in Figure 3A shows a proplastid which probably corresponds to the small autofluorescent organelles visible in the CLSM (compare Figure 1H). A subcortical actin filament bundle is illustrated in Figure 3D (arrow). Its interaction with ER cisternae generates streaming. Figure 3E shows part of a newly formed window one hour after local irradiation. A swollen, detaching chloroplast (sC) is seen at the border of the window.
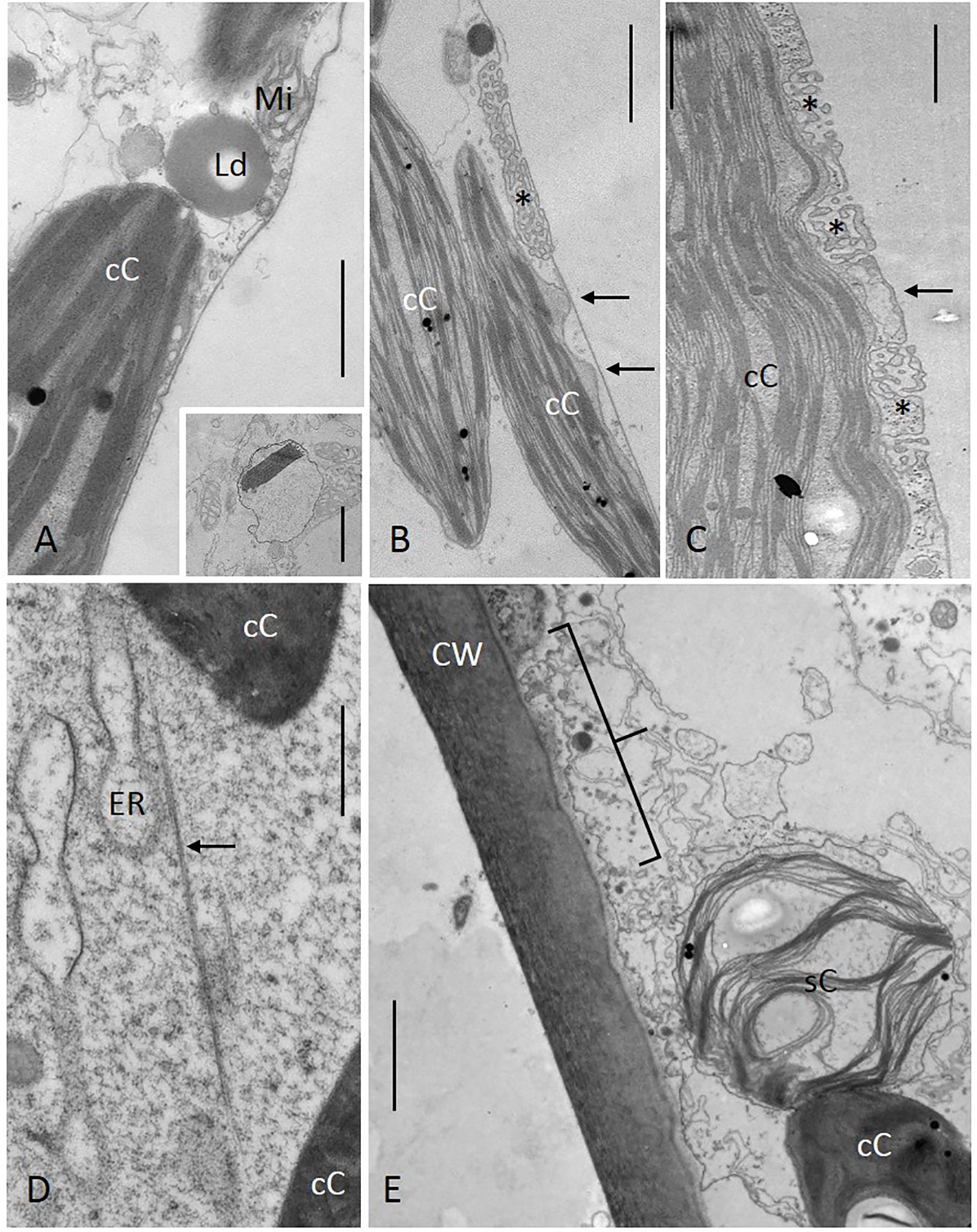
Figure 3. Fine structure of branchlet internodal cells. (A–D) Control regions, (E) window. The outer membrane of the cortical chloroplast (cC) is located adjacent to the plasma membrane (A, B), to the inner surface of charasomes [asterisks in (B, C)] and to cisternae of the endoplasmic reticulum [arrow in (C)]. (B) is a tangential section of cortical chloroplasts with lobes contacting the plasma membrane (arrows). The inset in (A) is a section through a proplastid. (D) shows a subcortical actin bundle (arrow) spanning the region between two chloroplasts. Note cisternae of the endoplasmic reticulum (ER). (E) Cross-section through the border of a window. The swollen chloroplast (sC) was probably about to detach (bracket in E indicates part of the window). Detachment of the plasma membrane from the cell wall is a fixation artefact. (A-B), (E) Chemical fixation, (D) cryofixation. CW cell wall, Ld lipid droplet, Mi mitochondrium. Bar = 2 µm (E), 1 µm [(A–C), inset in A] and 500 nm (D).
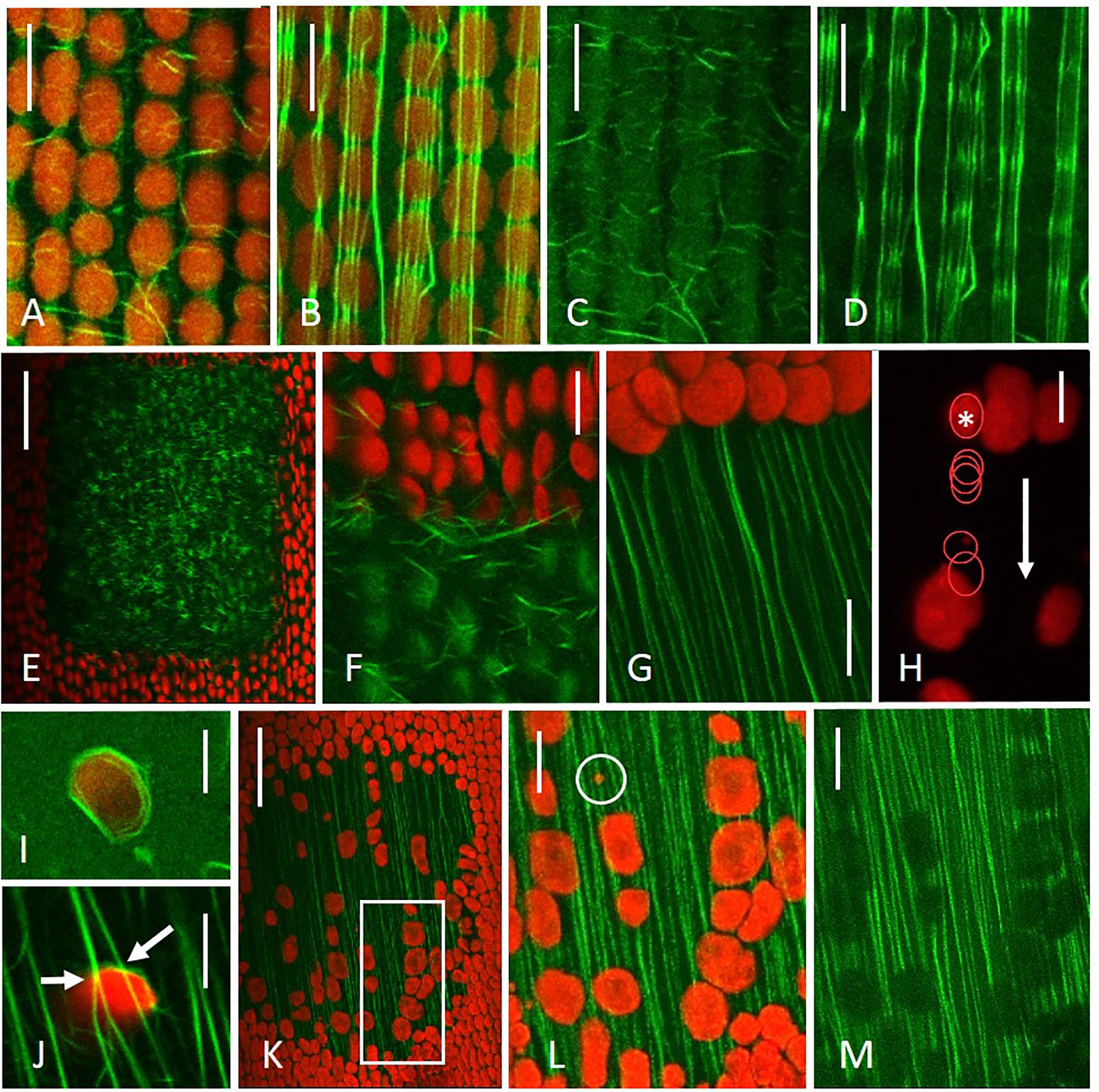
Figure 4. Actin cytoskeleton and chloroplasts in control regions and in windows. (A–D) Cortical actin strands (A, C) and subcortical actin bundles (B, D) in a control region. Note that the signal of the subcortical bundles is attenuated beneath chloroplasts (D). (E, F) Short, randomly oriented actin rods are present between the plasma membrane and bleached chloroplasts. (G) After the detachment of bleached chloroplasts, subcortical actin bundles become clearly visible at the upstream end of the window. (H) At the cortex of a window, a chloroplast (asterisk and subsequent outlined stages) moves slowly and with irregular velocity parallel to the streaming direction (arrow). Time interval between stages is 5 s. The other chloroplasts were immobile during the observation period. (I) Actin ring around an endoplasmic chloroplast. (J) Contacts (arrows) between chloroplast-associated actin ring and subcortical actin bundles. Note that the signal of the subcortical bundles is not attenuated indicating that the chloroplast is still located in the endoplasm. (K–M) Actin filament bundles at the inner, endoplasmic side of resettled chloroplasts; chloroplasts are aligned parallel to the actin bundles. Inset in K corresponds to higher magnifications shown in (L, M). A proplastid is seen in the encircled area (L). Images were taken 2 hours (E-F), 1 day (H, J), 2 days (G) and 7 days (K–M) after irradiation. Images (A–D, I) were taken from control cells. Actin was visualized by perfusion of cells with green fluorescent phalloidin, red = chlorophyll fluorescence; (A,B, E-G, I–L) are merged images. Images are single optical sections, except (G, J, K-M) which are maximum projections of 27, 20, 100 and 75 optical sections with a thickness of 0.9 µm, respectively. Bars are 50 (E, K) and 10 µm (all other images).
3.3 Actin cytoskeleton and resettlement of chloroplasts
Figures 4A–D show the actin cytoskeleton in a control cell, stained by perfusion with fluorescent phalloidin. Delicate actin strands are present at the plasma membrane (Figures 4A, C); subcortical, continuous actin bundles extend along the inner, endoplasmic side of chloroplasts (Figures 4B, D). The fluorescence of these bundles is attenuated by the autofluorescence of the chloroplasts (Figure 4D).
Two hours after irradiation, numerous short actin strands and rods were present between the plasma membrane and bleached chloroplasts; they were distinct (mostly thicker and straighter) from the delicate actin strands in nearby control regions (Figures 4E, F). Subcortical actin filament bundles either persisted or regenerated within a few hours from the upstream (endoplasm-delivering) border of the window, bleached chloroplasts detached and cortical actin strands or rods disappeared (Figure 4G). After one day active endoplasmic streaming recovered to near-control rates over the whole window (Supplementary Video 5).
Most endoplasmic chloroplasts were passively and continuously transported within the flowing endoplasm with many of them actively rotating as previously described (Supplementary Video 2). At the cortex of windows, slow and discontinuous straight movement was occasionally observed (Figure 4H), which was never found in control regions. Figures 4I, J show actin filament bundles (rings) attached to the surface of endoplasmic chloroplasts. The actin ring in Figure 4J contacts two subcortical actin bundles in a window (arrows). A contact between an endoplasmic chloroplast without actin ring is shown in Supplementary Figures 2C, D. Such an orientation (plasma membrane – subcortical actin bundle – chloroplast) was, however, observed only rarely.
Figures 4K–M show resettled chloroplasts in a 7-days old window. Their bright chlorophyll fluorescence indicates that they are not remnants of the original chloroplast layer, but newly attached organelles. Chloroplasts were squeezed between plasma membrane and actin bundles just as in control regions (plasma membrane – chloroplast - subcortical actin bundle; Figures 4A–D), and chloroplast-associated actin rings were absent. Most subcortical bundles appeared to be continuous. However, since their fluorescence signal was attenuated by the chlorophyll fluorescence (Figure 4M), we cannot exclude the possibility that some bundles were interrupted. Subcortical bundles between resettled chloroplasts were close to the plasma membrane (see Supplementary Figures 2E-F). In regions with abundant chloroplasts and in fully replenished windows, fine actin strands were again present near the plasma membrane (Supplementary Figure 2G).
Chloroplasts with division furrows were likewise able to resettle at windows and their shape remained unchanged for days (Figures 5A–E). Figures 5C–E (arrows) show that the size of a presumed proplastid did not increase during the observation period. Bleached chloroplasts were never observed to resettle at windows.
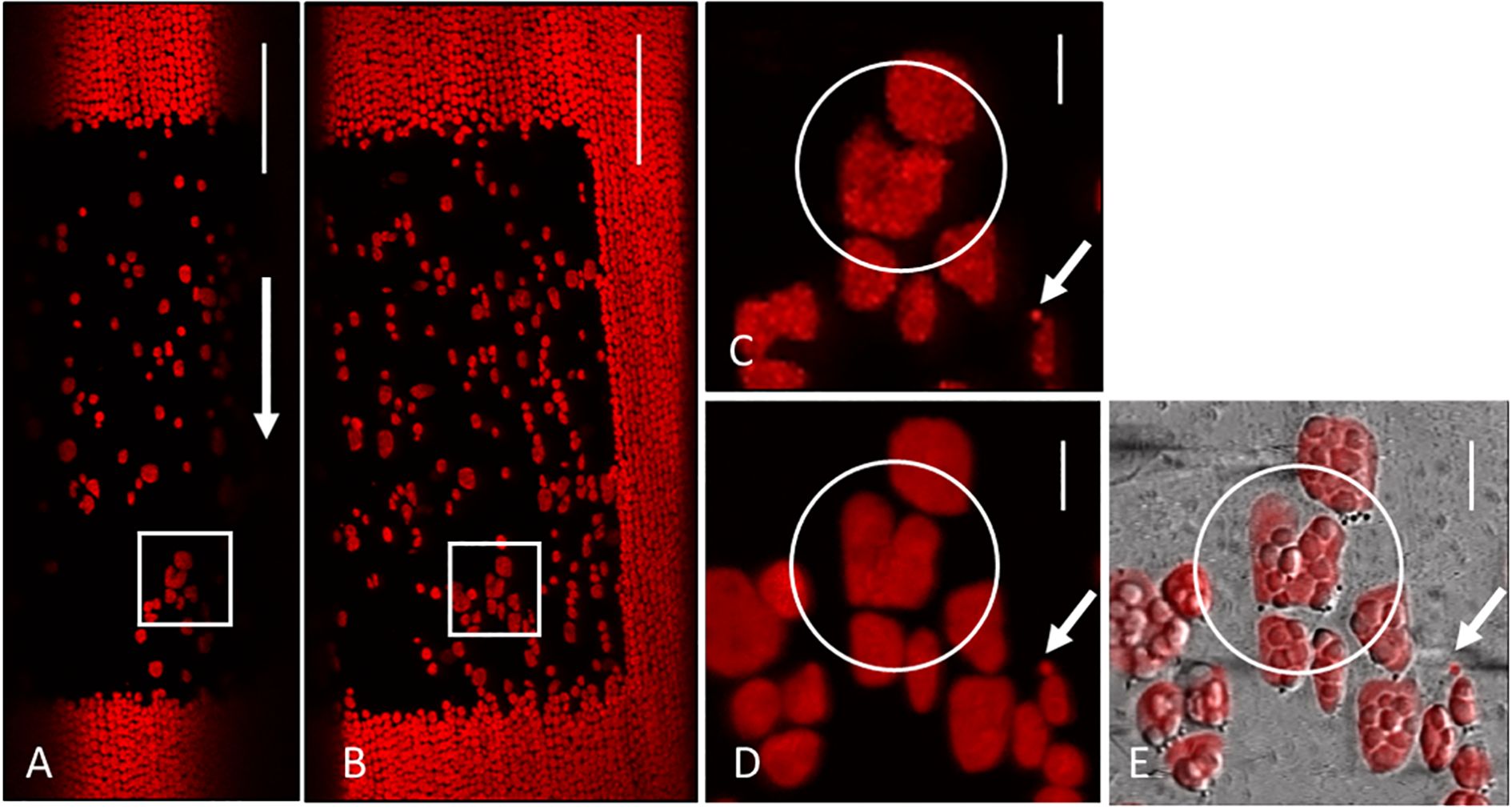
Figure 5. Giant chloroplasts resettled in a 5 (A, C) and 10 day old window (B, D, E). The arrow in (A) indicates the streaming direction. The rectangles in (A, B) correspond to the enlarged images (C–E). A chloroplast with a stable division furrow is encircled in (C–E). The arrows in (C–E) mark the position of a proplastid. Chlorophyll autofluorescence (A–D), merged with bright field (E). Images (A, B) are maximum projections of 21 optical sections with a thickness of 0.9 µm, (C–E) are single sections. Bar = 50 µm (A, B) and 10 µm (C, D, E).
3.4 Preferred attachment of chloroplasts at the downstream end of windows
A typical time series illustrating the changes in chloroplast number and position over a period of 5 weeks is shown in Figure 6. The first image was taken one day after window formation with several scattered chloroplasts visible in the window. During the following days and weeks, some of them slightly changed their position, whereas others disappeared completely (Figures 6A–D and pairwise overlays in E-G). Chloroplasts often reattached in files parallel to the direction of actin bundles and cytoplasmic streaming, respectively (red arrow in A). The comparison of the first (A) and the last image of this series (D; see overlay H) reveals that endoplasmic chloroplasts resettled preferentially at the downstream end of the window. The preferred downstream attachment was clearly visible in windows containing the neutral line (Figure 7; note that this cell was also treated with microtubule depolymerizing oryzalin). Such windows show two opposing up- and downstream regions, respectively (arrows in Figure 7A indicate the streaming directions), with chloroplasts preferentially resettling at the corresponding downstream ends (compare Figure 7F which is the overlay of A and E). Figure 7 also shows that not only the downstream ends are preferred sites for resettlement of chloroplasts, but also the neutral line itself. Observations in windows containing the neutral line also confirm the alignment of resettled chloroplasts along actin bundles. Inside windows or in their direct vicinity, the passive endoplasmic flow during irradiation became occasionally deviated across the neutral line which induces a U-shaped regeneration of the actin bundles and corresponding active endoplasmic flow. These U-turns are mirrored by the orientation of chloroplast files (Supplementary Figure 3).
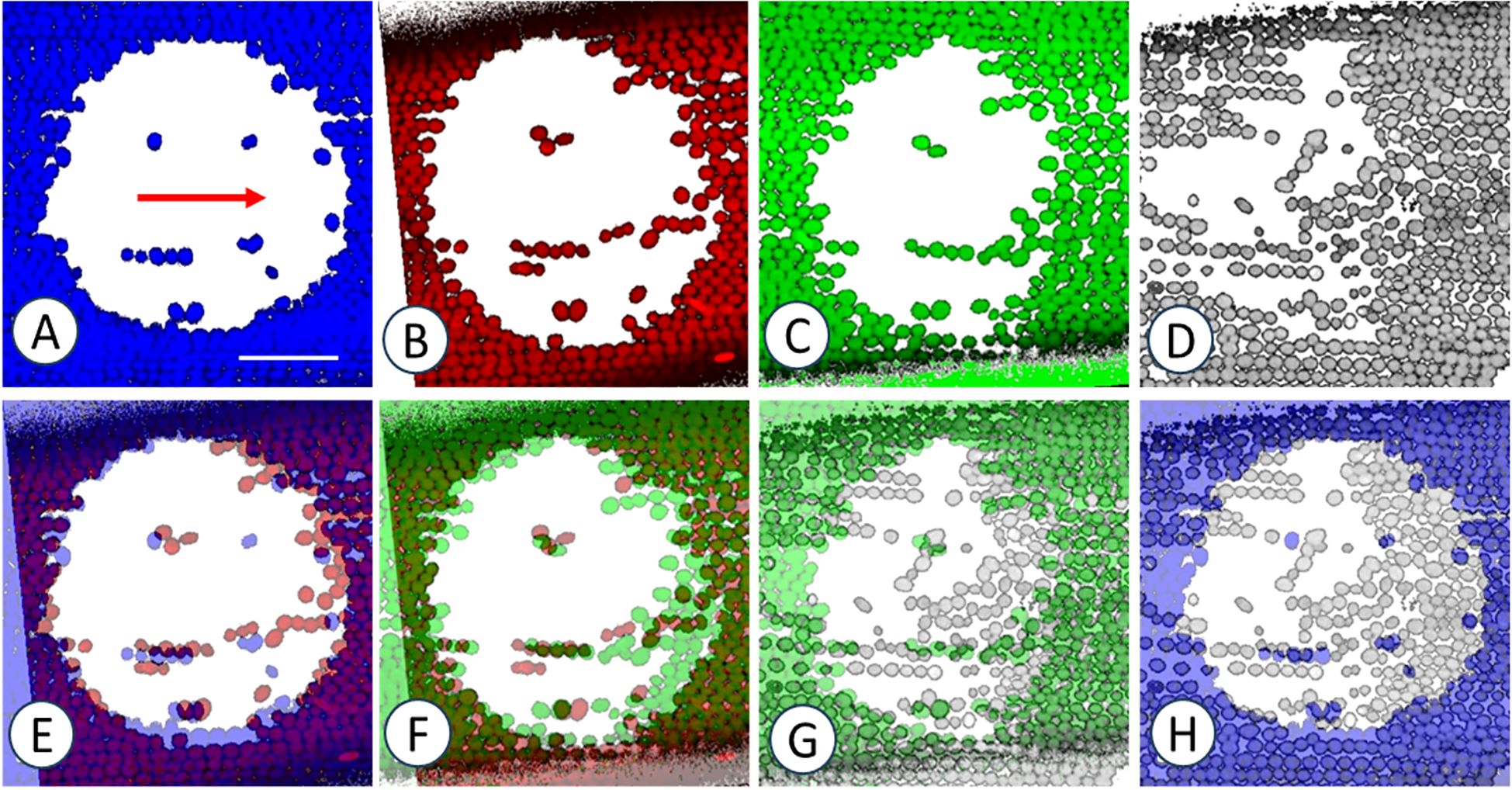
Figure 6. Preferred resettlement of chloroplasts at the downstream end of windows. Images were taken 1 day (A), 1 week (B), 3 weeks (C) and 5 weeks (D) after irradiation. Arrow in (A) indicates streaming direction. (E–G) Pairwise overlays of consecutive images (A/B, B/C, C/D). (H) Comparison between (A, D) False coloured images are maximum projections of 7 optical sections with a thickness of 1.3 µm. Bar in (A) = 50 µm for all images.
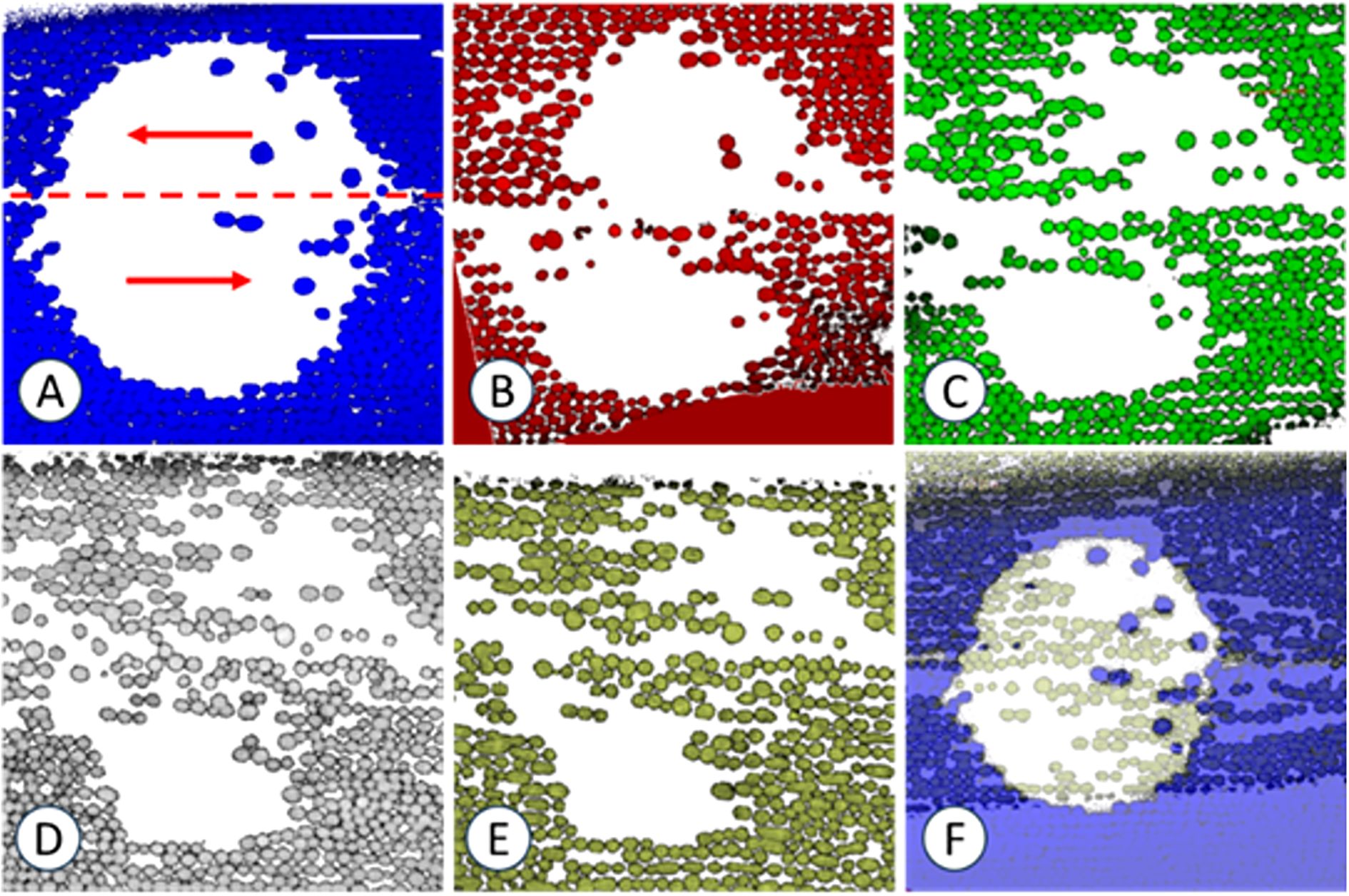
Figure 7. Resettlement of chloroplasts in a window located at the neutral line and in the presence of oryzalin. Images were taken 1 day (A), 1 week (B), 3 weeks (C), 6 weeks (D) and 7 weeks (E) after irradiation. The cell was treated with 8 µm oryzalin before and after irradiation. Red arrows in (C) indicate streaming direction. (F) shows the overlay of (A, E) Note preferred resettlement of chloroplasts at the neutral line (dashed line in A) and at the two opposing downstream ends. False coloured images are maximum projections of 8 optical sections with a thickness of 1.3 µm. Bar in (A) = 50 µm for all images.
Provided that the number of endoplasmic chloroplasts is sufficient, the entire window area will be covered by chloroplasts. These “closed” windows have parallel rows of chloroplasts and are almost indistinguishable from non-irradiated control areas (Figures 8A, B). Often, a narrow, sickle-shaped region at the upstream region remains chloroplast-free (small arrow in Figure 8B). The resettled chloroplasts accumulate starch grains, just as the control chloroplasts. We occasionally observed starch-free chloroplasts at the border of the window with more intense chlorophyll fluorescence signals compared to other chloroplasts (Figures 8C, D). We assume that these chloroplasts correspond to partly irradiated chloroplasts which did not detach (compare Figure 2D) and were partially damaged, which is in accordance with PAM fluorescence data (see below).
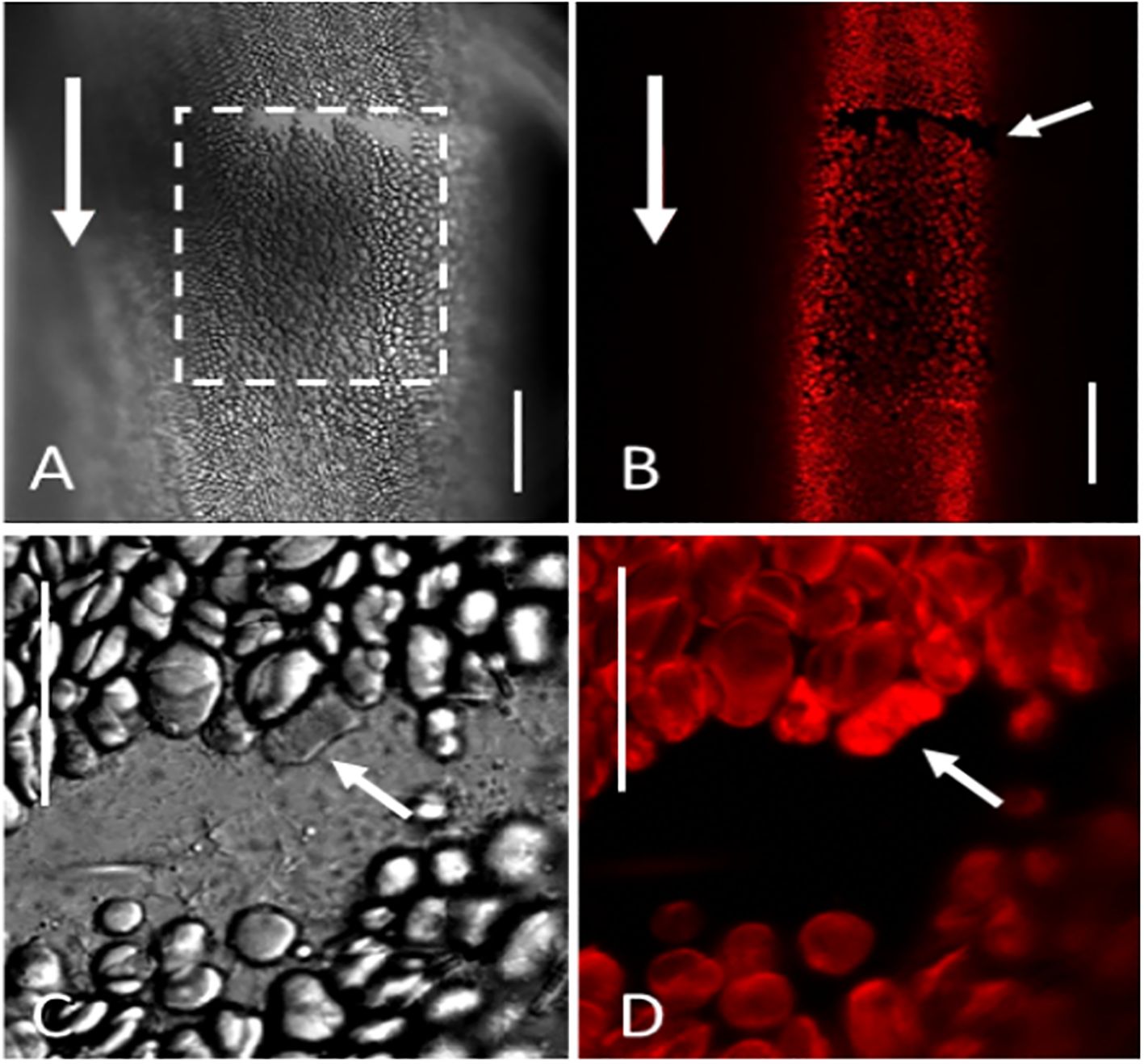
Figure 8. Healed (closed) window. The cell was irradiated 8 weeks before the image was taken. (A, B) Most of the irradiated area (rectangle in A) has been replenished by chloroplasts apart from a thin region at the upstream end of the window (small white arrow in (B); large arrows indicate streaming direction). (C, D) Note starchless and stronger fluorescent chloroplast at the border of the window. (A, C) bright field, (B, D) chlorophyll fluorescence. All images are single sections. Bar = 100 µm (A, B) and 20 µm (C, D).
3.5 Actin-dependent cytoplasmic streaming, but not microtubules are required for release and resettlement of chloroplasts
To study the effect of cytoplasmic streaming we applied cytochalasin D. Concentrations of 10 and 20 µM cytochalasin D inhibited cytoplasmic streaming within 30 min (Supplementary Video 6) and, when applied before or immediately after bleaching, prevented the release of damaged chloroplasts (Supplementary Figures 4A–C; note inhibition of cytoplasmic streaming in Supplementary Video 7 which is a z-stack of bright field images). The effect of cytochalasin D was reversible; chloroplast-free windows were obtained several hours after cytochalasin was washed out with AFW (Supplementary Figures 4D–F; note recovery of cytoplasmic streaming in Supplementary Video 8 which is a z-stack of bright field images). When cytochalasin D was applied one day after irradiation, i. e. after the detachment of bleached chloroplasts, the resettlement of chloroplasts at windows was significantly impaired compared to the control (GLMM - groups p = 0.003, time p < 0.001, groups x time p < 0001; Figure 9A; Supplementary Figures 4G–L, Supplementary Data Sheet 1).
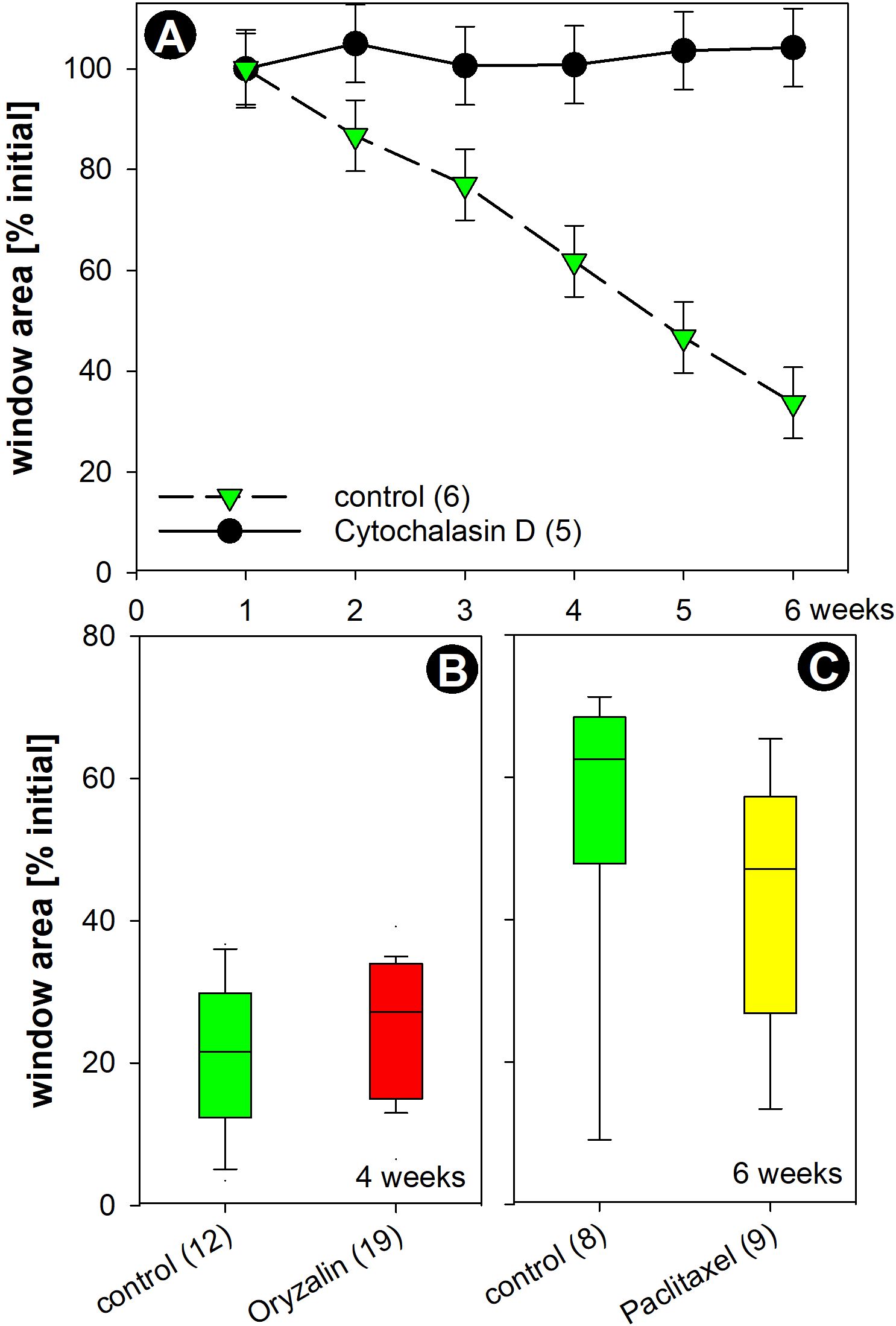
Figure 9. Effect of cytoskeleton inhibitors on the resettlement of chloroplasts. (A-C) Relative size of windows (chloroplast-free areas) in control cells (green symbols and green bars) and in cells treated with cytoskeleton inhibitors [black symbols in A, red and yellow bars in (B, C)]. Cells were irradiated and left in AFW for 1 day to allow the release of bleached chloroplasts. They were then treated with 20 µM cytochalasin D (A), 8 µM oryzalin (B) or 10 µM paclitaxel (C). Data are means ± SD, given as % of initial window size for (A) with GLMM resulting in significant differences (p< 0.001). For oryzalin (p = 0.54) and paclitaxel (p = 0.11), no significant differences were found (Mann-Whitney-test). Bars in (B, C) represent median boxes with 90-75-50-25-5 percentiles. Numbers in brackets indicate number of replicates.
Depolymerization of microtubules by oryzalin (8 µM; Figures 7A–F, 9B) or their stabilization by paclitaxel (10 µM; Figure 9C) had no significant effect on the recovery of the chloroplast layer (Mann-Whitney-U test p = 0.535 for oryzalin, p = 0.114 for paclitaxel, Supplementary Data Sheet 1).
3.6 Photosynthetic performance of chloroplasts
We determined photosynthetic performance in resettled and control chloroplasts using PAM fluorescence. Fluorescence yields were measured over a period of up to 78 days after irradiation took place. Measuring points included control regions about 100 µm (close) and 200 µm (far) apart from the window, resettled chloroplasts and individual chloroplasts directly at the border of the windows (“border chloroplasts”) (Figure 10).
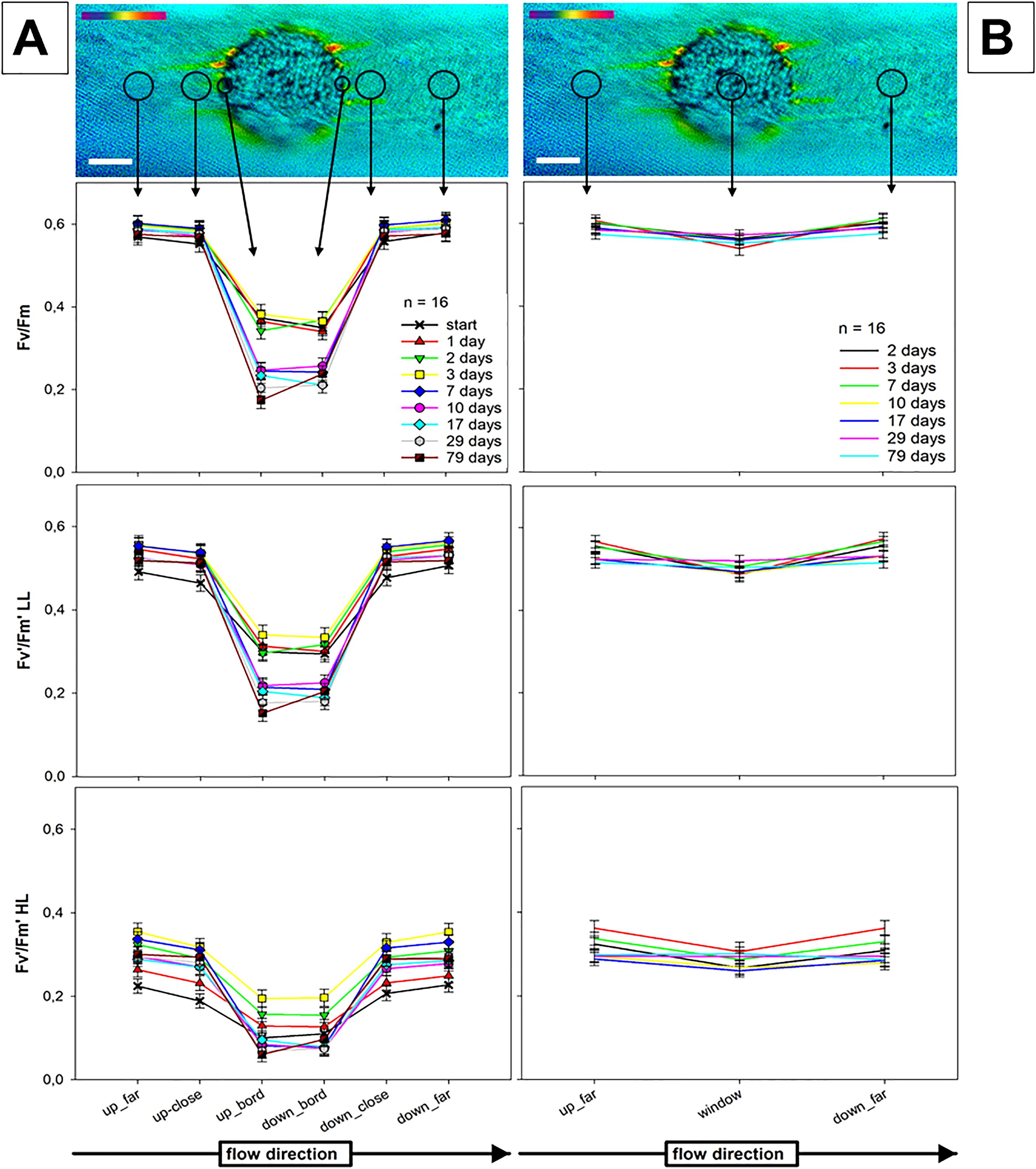
Figure 10. Photosynthetic parameters of chloroplasts in branchlet internodal cells of C. australis. Fluorescence yields measured after dark adaptation (Fv/Fm) and after exposure to low light (20 µmol photons m-2.s-1; Fv’/Fm’ LL) and high light (60 µmol photons m-2.s-1; Fv’/FM’ HL), respectively. The top image shows a false colour image of maximum dark yield (Fv/Fm) of resettled chloroplasts, damaged chloroplasts at the border of the window and chloroplasts in undamaged control regions. Arrows indicate measuring spots for the graphs placed below. (A) Comparison between chloroplasts located at the border of the window and chloroplasts in control regions. (B) Comparison of chloroplasts resettled at windows and chloroplasts in remote regions. Means and standard errors. Bar in (A) = 50 µm.
Figures 10A, B show Fv/Fm, Fv’/Fm’ LL and Fv’/FM’ HL. Figure 10A displays the comparison between control chloroplasts outside the window and border chloroplasts. Both Fv/Fm and Fv’/Fm’ of border chloroplasts were always significantly lower than those of control chloroplasts and further decreased with time (GLMM, Supplementary Data Sheet 2). No significant differences were found between control regions located close or far away from the window or located at the upstream or downstream end of windows. Figure 10B compares the properties of resettled chloroplasts and chloroplasts in control regions. The data obtained for resettled chloroplasts were slightly, but significantly lower than those measured in control chloroplast, independent of light provided (GLMM, Supplementary Data Sheet 3). Fv/Fm and Fv’/Fm’ values of resettled chloroplasts remained stable over time, but values of control chloroplasts slightly decreased.
3.7 Detection of CaCHUP1 and analyses of its protein sequence
CHUP1 is involved in chloroplast movement in vascular plants (see Kong et al., 2024 and references therein). We screened for this protein in Chara databases and found homologous forms in C. australis as well as in C. braunii. To examine the sequence more closely, we cloned and sequenced the CHUP homolog of C. australis. CaCHUP1 comprises 1201 amino acids and has a calculated MW of 133 kDa. Both Characean sequences were aligned with representative proteins of Klebsormidium nitens (KnCHUP1B, Charophyta), Marchantia paleacea (MpCHUP, liverworth), Physcomitrium patens (PpCHUP, moss), Adiantum capillus-veneris (AcvCHUP1A, fern), and the eudicot Arabidopsis thaliana (AtCHUP1); conserved domains and regions were labelled (see Supplementary Figure 5). While the two proteins of Chara (CaCHUP1 and the hypothetical protein CBR_g4598 of C. braunii (named CbCHUP in the alignment) showed 92% sequence homology when compared to each other, CaCHUP1 shared only 26-29% identity when compared to all other CHUP sequences mentioned above.
An InterPro search revealed domains and regions all sharing an N-terminal signal peptide sequence that directs them to the outer membrane of chloroplasts (Supplementary Figure 5 illustrates their arrangement along the proteins. They all share an N-terminal signal peptide sequence that directs them to the outer membrane of chloroplasts. Moreover, all sequences show a coiled-coil region followed by an F-actin-binding region at the beginning of CHUP1/IPGA1-like domain (IPR040265, CHLOROPLAST UNUSUAL POSITIONING 1/INCREASED PETAL GROWTH ANISOTROPY 1). Interestingly, all three Charophyta protein sequences show slightly longer coiled-coil and CHUP-domains.
Native CaCHUP1 protein was visualized by western blot analyses using C. australis protein extracts and an antibody created against the protein sequence of CaCHUP1 (see Figure 11A). A prominent band was visible at about 130 kDa, as expected from the calculated molecular mass of the protein.
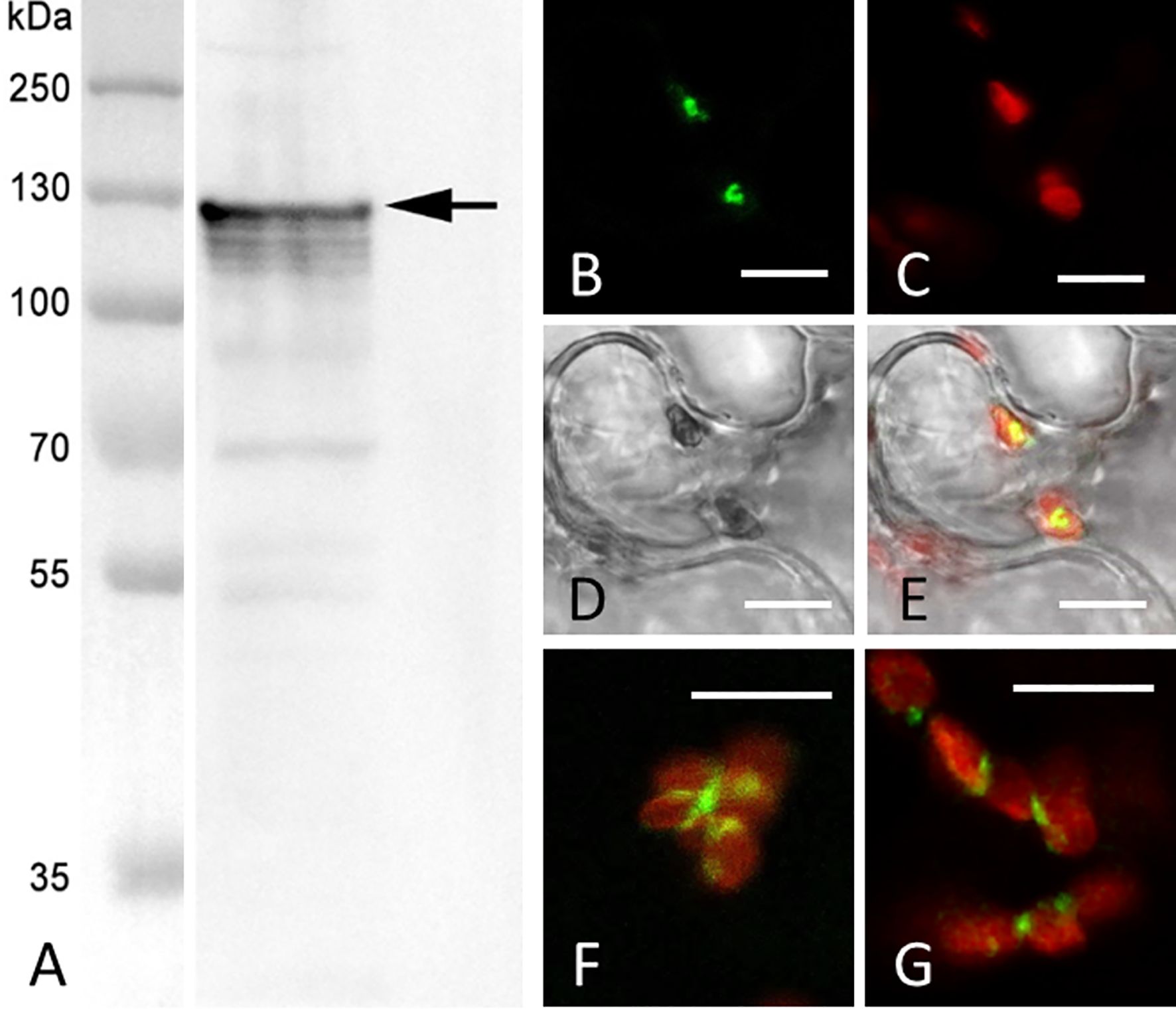
Figure 11. Visualization of native CaCHUP1 in C. australis protein extract and localization of transiently expressed CaCHUP1-GFP in tobacco leaves. (A) Western blot of C. australis protein extract. The prominent CaCHUP1 band (arrow) was visualized using an antibody created against the protein sequence of CaCHUP1 (marker = molecular mass in kDa) (B–G) Transient expression of CaCHUP1-GFP in epidermal cells of tobacco leaves. (B, C) CaCHUP1-GFP is shown in green, while chloroplasts are shown in red (chlorophyll autofluorescence); (D) is the corresponding DIC image which is merged with the fluorescent images in (E) In (F, G) the GFP fluorescence is merged with chlorophyll fluorescence. All images are single sections. Bars are 10 µm.
At present, we are not able to transform Chara directly. Thus, we expressed CaCHUP1 in tobacco leaves to gain insights into its potential function. As shown in Figures 11B–G, CLSM revealed a CaCHUP1-GFP that localizes in the form of small, punctate spots on the surface of chloroplasts.
4 Discussion
4.1 Chloroplasts of Chara internodal cells
In contrast to most vascular plants and non-motile algae (Suetsugu et al., 2017; Wada, 2013), the chloroplasts of characean internodal cells are firmly anchored at the cell periphery. They are embedded in a fibrous meshwork (presumably cortical actin and associated filaments) between the plasma membrane and the subcortical actin bundles which run parallel to the chloroplast files (Wasteneys et al., 1996; Williamson, 1985; Williamson et al., 1985). Dissolution of the presumed actin meshwork by perfusion of cells with low-ionic strength (low salt) solution leads to a loss of the subcortical actin bundles and releases the chloroplasts. These observations suggest that the close association of actin with chloroplasts reflects a dependence of chloroplast alignment on actin bundle organization (e.g. Wasteneys et al., 1996). Chara chloroplasts must therefore be equipped with proteins responsible for anchorage at the plasma membrane, with actin-binding and, possibly, actin-polymerizing proteins. In addition, the cortical ER was also shown to participate in the immobilization and relocation of chloroplasts (Andersson et al., 2007a, b; Lichtscheidl and Url, 1990).
Chloroplasts may detach from the cortex and are then transported along with endoplasmic flow; reasons for their release are manifold. From time to time, long-living giant chloroplasts in the endoplasm are present, which probably reflects an imbalance between cell elongation and chloroplast growth and division (compare Green, 1964). Chloroplasts may be released after mechanical damage, centrifugation, chemical treatment (references in Foissner and Wasteneys, 2012; 2014), and local illumination with excess light (“window” formation; Kamitsubo, 1972). In the current study, strong blue light was provided for window formation, but also UV (Foissner and Wasteneys, 2012), green, yellow and red light (laser lines 514, 561 and 633, respectively) of adequate duration and intensity can be used (unpublished observations), which indicates that detachment is not linked to a photoreceptor (Łabuz et al., 2022). It still remains an enigma, how irradiation causes a loosening of chloroplast anchoring. One possible explanation suggests a role of Ca2+ binding proteins, because Foissner and Wasteneys (2012) reported that chemicals causing an influx of Ca2+ into the cell induce the release of chloroplasts.
We were able to show that non-damaged endoplasmic chloroplasts resettle in windows, leading to an almost complete structural and physiological repair of the cortical layer (see Figure 12 for a schematic summary). Long-term observations of individual resettled chloroplasts, including presumed proplastids (Figure 5; Choi et al., 2021), and the fact that the repair of the cortical chloroplast layer depends on the presence of endoplasmic chloroplasts (Figures 2A–C) exclude the possibility that chloroplast division is involved. Bleached chloroplasts released from irradiated areas were not able to recover and to re-attach at windows.
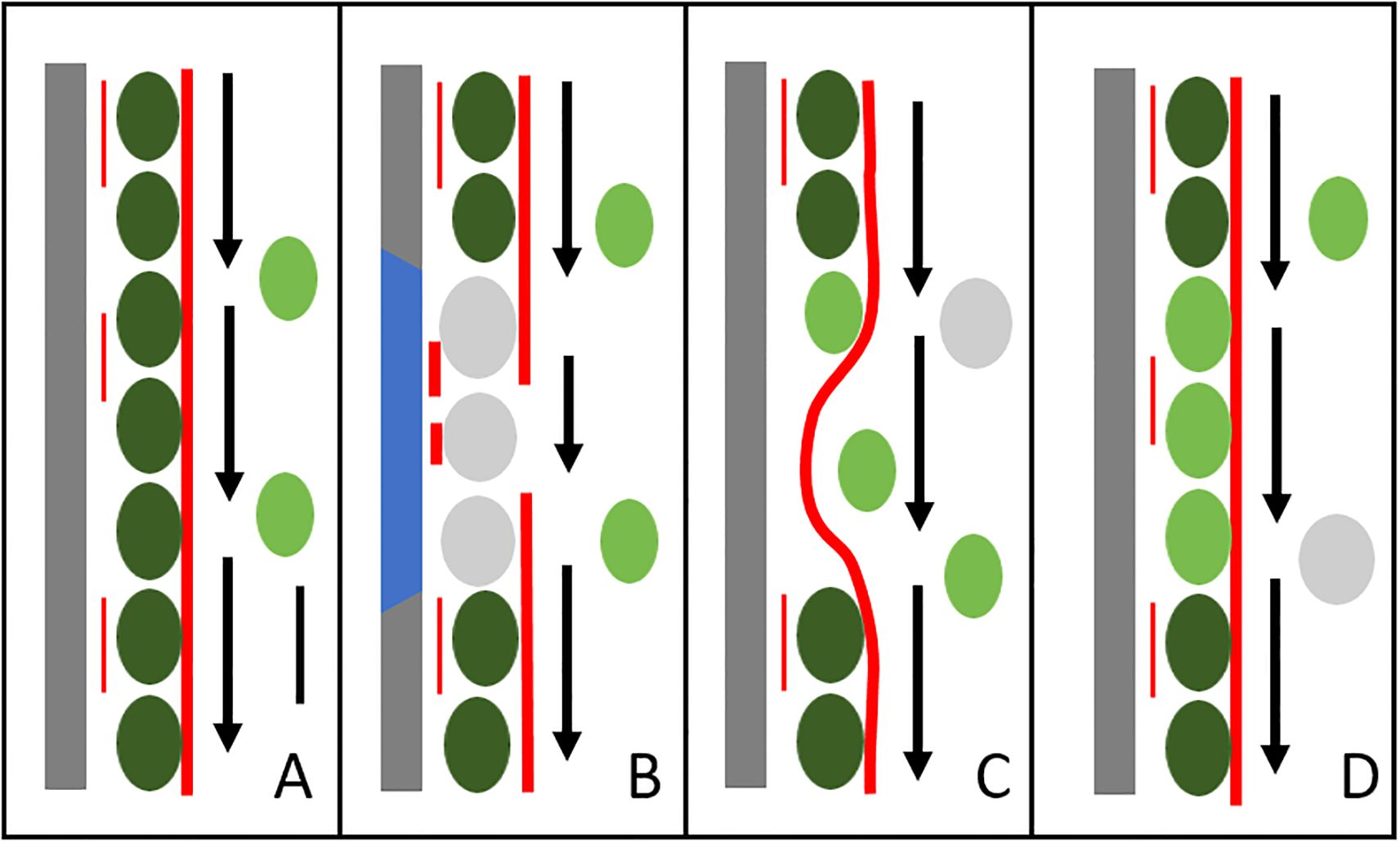
Figure 12. Schematic illustration of actin-dependent chloroplast resettlement at windows created in Chara internodal cells (A) Intact cell. Stationary, cortical chloroplasts (dark green) are located next to the cell wall (grey bar), thin red lines are cortical actin filaments near the plasma membrane, thick red line is a subcortical actin bundle. Endoplasmic chloroplasts (light green) are passively transported in the endoplasm (arrows indicate direction and velocity). (B) Irradiation with strong light (blue bar) causes bleaching and swelling of cortical chloroplasts (grey) and the transient formation of short cortical actin bundles. Endoplasmic streaming is locally disturbed and, possibly, subcortical bundles are disrupted. (C) Recovery of subcortical actin bundles and endoplasmic streaming after detachment of bleached chloroplasts. Note that subcortical bundles are closer to the plasma membrane. (D) Replenished window. Only organelles and structures relevant for this process are drawn. Bar in (A) = approximately 10 µm.
4.2 Actin cytoskeleton is involved in the release and resettlement of chloroplasts
The actin cytoskeleton, especially cytoplasmic streaming, plays a pivotal role both in the removal of bleached chloroplasts and in the resettlement of intact chloroplasts. After treatment with inhibitory concentrations of cytochalasin D, bleached chloroplasts remained in place and, if applied after removal of bleached chloroplasts, windows remained empty (Supplementary Figures 4A–C; Figure 9A). Both effects were reversible (Supplementary Figures 4D-F). Depolymerization of microtubules by oryzalin or stabilization by paclitaxel had no significant effect on the recovery of the cortical chloroplast layer (Figures 9B, C). Previous studies have shown that microtubules are also not required for the deposition of a wound wall after mechanical or chemical damage (Foissner and Wasteneys, 2012).
The recovery of the actin cytoskeleton in windows was already described earlier (e. g. Williamson et al., 1984) and is summarized in Figure 12 which includes the findings obtained in this study. Actin filament bundles regenerate from the upstream region of the window which corresponds to the growing (barbed) end of actin filaments (Williamson et al., 1984). In contrast, chloroplast resettlement preferably occurs at the downstream border of windows. This is possibly due to the delayed recovery of actin bundles which retards cytoplasmic bulk streaming (just as at the neutral line; Figure 7) and thereby facilitates the physical contact of the chloroplasts with the cortex. Furthermore, chloroplasts arriving at the downstream end encounter an “obstacle” in form of the cortical chloroplasts at the edge of the window. This will also lead to a disturbance and retardation of laminar streaming, accumulation of chloroplasts and probably closer contact with the cortex.
Once a chloroplast is settled, the probability that other chloroplasts attach near or at the upstream end of this chloroplast is high. This leads to the formation of chloroplast files parallel to the direction of mass streaming or subcortical actin bundles, respectively. In principle, chloroplasts may attach not only at the downstream end but anywhere in the window and serve as a starting point for the formation of a file by consecutive alignment of individual plastids.
In the absence of resettled chloroplasts, the cortex at windows is shielded from the endoplasm by a dense layer of subcortical actin bundles which are located close to the plasma membrane (Figure 4G; Supplementary Figures 2E-G); compare Foissner and Wasteneys, 1999). Saltatory, probably active movements of chloroplasts at the window as well as contact between chloroplast-associated actin rings with subcortical bundles have been observed (Figures 4H, I). We assume that they are due to actin-actin interactions either via bundling proteins (e.g. formin; Thomas et al., 2009), motor proteins (myosin; Buchnik et al., 2015; Ueda et al., 2015), or proteins involved in actin polymerization (e. g. CHUP1; see below). The chloroplasts have then to cross the actin layer to get their final position near the plasma membrane. Whether this involves a slipping between actin bundles or, more probably, dynamic actin remodeling (de- and repolymerization of actin bundles) remains to be investigated.
Chen (1983) mentioned that chloroplasts align along (abnormal) pathways of streaming after mechanical removal of cortical chloroplasts by means of centrifugation. We identified the same phenomenon by providing excess light. In addition, we studied chloroplast resettlement in detail, which has not been published before.
4.3 Photosynthetic performance of chloroplasts
Chlorophyll fluorescence measurements showed that the photosynthetic properties of resettled chloroplasts are within the range of those in non-damaged regions. Thus, the cortex at windows recovered not only in the typical morphology, also the photosynthetic performance was comparable to the control regions. Chloroplasts with extremely low Fm/Fv and Fm’/Fv’ values, which decreased further with time, were occasionally found at the border of the windows (Figure 11). They probably corresponded to partly bleached chloroplasts which were neither able to detach nor able to recover, consistent with the absence of starch grains (Figures 8C, D). These impacted chloroplasts, however, survived the whole experiment for around three months.
Bulychev and coworkers (Bulychev, 2019, 2020; Dodonova and Bulychev, 2011) found that chloroplast performance measured at some distance of a local light spot (which did not cause chloroplast release), varied according to the direction of cytoplasmic streaming, with lower fluorescence values downstream the irradiated area. They concluded that ions and metabolites released during photosynthesis affect the performance of chloroplasts in shaded regions. Based on these studies, we also considered the possibility of a cyclosis-driven asymmetry along the illuminated measuring area: upstream endoplasm might have a different chemical composition than the downstream endoplasm just before leaving the illuminated area. In this study, however, we did not find any significant differences in fluorescent yields, neither between the reference spots, nor between up- and downstream window margins. Our results indirectly confirm the experiments of Bulychev (2019, 2020), because photosynthetic metabolites are not released in the absence of chloroplasts (window area). In addition, distances between chloroplast-bearing control spots might have been too small for measuring differences, but this assumption calls for further investigation.
4.4 Chloroplast anchorage and actin binding proteins
Characean chloroplasts have characteristics comparable to those of vascular plants, to which they are closely related (e.g. McCourt et al., 2011; Turmel et al., 2013). In vascular plants (Wada and Kong, 2018) and probably also in ferns, mosses, and certain algae (e.g. Mineyuki et al., 1995), a protein complex is involved in both motility and anchorage of chloroplasts. Among these proteins, CHUP1 is required for chloroplast photo-relocation. CHUP1 homologs were found in Nitella mirabilis (Suetsugu and Wada, 2016) and also in the current study in C. australis. Recently, Yamamoto-Negi et al. (2024) characterized a CHUP1 in Marchantia polymorpha and pointed out the relevance of this protein in chloroplast positioning. Very recently, CHUP1 was also shown to restrict movement of chloroplasts in tobacco and Arabidopsis (Nedo et al., 2024). CaCHUP1, like other known CHUP1 proteins, is composed of several functional domains and regions (compare Supplementary Figure 5) including the N-terminal hydrophobic signal peptide that targets the protein to the outer membrane of chloroplasts (compare Figures 11B-G). The signal peptide is followed by a region of several coiled-coil domains that is described to function in anchoring chloroplasts to the plasma membrane. On the C-terminus, the highly conserved CHUP1/IPGA1-like domain with its actin-binding region is located. Both, N-terminal hydrophobic signal peptide and CHUP1/IPGA1-like domain, are described to be involved in movement and proper positioning of chloroplasts in plants (Oikawa et al., 2003; 2008). The presence of orthologs of KAC in the Charophyceae (Suetsugu and Wada, 2016) suggests that CaCHUP1 is also involved in actin polymerization. A role of CaCHUP1 in chloroplast motility can be excluded since Chara chloroplasts are immobilized in the cortex under normal conditions. When released from the cortex, the organelles are passively transported by bulk endoplasmic flow. Actin rings or polygons at the surface of chloroplasts support active rotation (Figures 4I, J; Wasteneys et al., 1996), but clusters of short actin filaments – typical for cp-actin (Kong et al., 2024) – have never been observed in characean internodal cells (compare Wasteneys et al., 1996; Williamson and Hurley, 1986; Williamson et al., 1987). These observations, along with the fact that Chara chloroplasts cannot perform active photo-relocation, makes movement via polymerization of actin unlikely. It is more plausible that rotation and possible short-range movements of chloroplasts at windows occur via actin-myosin interaction, similar to endoplasmic streaming (Grolig et al., 1988). Even for vascular plants, active movement of plastids along actin bundles with the help of myosin motors is still a topic of discussion (e.g. Erickson and Schattat, 2018; Krzeszowiec and Gabryś, 2007; Natesan et al., 2009; Paves and Truve, 2007; Suetsugu et al., 2010) and furthermore, the exact mechanism of myosin-dependent motility in plants remains unclear (Buchnik et al., 2015).
Recent work indicates that chloroplasts in Nicotiana epidermal cells are transported via cytoplasmic streaming and that NtCHUP1 is involved in chloroplast anchorage (Nedo et al., 2024). We assume that CaCHUP1 plays a comparable role in the polymerization of actin filaments and bundles (but not of cp-actin) and in the anchorage of chloroplasts together with other proteins. Our study revealed that chloroplasts use different strategies for re-localization, either via polymerization of specialized cp-filaments or via cytoplasmic streaming. More information about the function of CaCHUP1 will be gained once a transformation method for characean internodes has been developed.
Data availability statement
The datasets presented in this study can be found in online repositories. The names of the repository/repositories and accession number(s) can be found in the article/Supplementary Material.
Author contributions
MH: Formal analysis, Investigation, Methodology, Validation, Visualization, Writing – original draft, Writing – review & editing. MH: Investigation, Methodology, Visualization, Writing – original draft, Writing – review & editing. AS: Investigation, Methodology, Validation, Visualization, Writing – original draft, Writing – review & editing. FH: Investigation, Visualization, Writing – review & editing. MS: Conceptualization, Investigation, Methodology, Supervision, Validation, Visualization, Writing – original draft, Writing – review & editing. IF: Conceptualization, Investigation, Methodology, Project administration, Supervision, Validation, Visualization, Writing – original draft, Writing – review & editing.
Funding
The author(s) declare that financial support was received for the research and/or publication of this article. This research was funded in part by the Austrian Science Fund (FWF) (grant DOI 10.55776/P 27536-B16 to IF).
Acknowledgments
We thank Alexander Bulychev (Moscow State University) and Mary Beilby for advice and stimulating discussion.
Conflict of interest
The authors declare that the research was conducted in the absence of any commercial or financial relationships that could be construed as a potential conflict of interest.
Generative AI statement
The author(s) declare that no Generative AI was used in the creation of this manuscript.
Publisher’s note
All claims expressed in this article are solely those of the authors and do not necessarily represent those of their affiliated organizations, or those of the publisher, the editors and the reviewers. Any product that may be evaluated in this article, or claim that may be made by its manufacturer, is not guaranteed or endorsed by the publisher.
Supplementary material
The Supplementary Material for this article can be found online at: https://www.frontiersin.org/articles/10.3389/fpls.2025.1544999/full#supplementary-material
Supplementary Figure 1 | Organelle motility in window and control region. (A, B) Bright field image and fluorescence image of a window (indicated by dashed line) two hours after irradiation. (C) Trajectories of endoplasmic (non-bleached) chloroplasts in the window and in the adjacent control region. Arrows indicate direction and distance travelled within 1.3 seconds. The short arrows within the window reflect lower velocities. Bars are 100 µm
Supplementary Figure 2 | Detached chloroplasts, actin cytoskeleton and resettled chloroplasts. (A, B) Clump of bleached, detached chloroplasts (arrows) in the endoplasm of a cell 1 day after local irradiation. A is the bright field image and B the corresponding fluorescence image. (C, D) Actin cytoskeleton and resettled chloroplasts in a 4 day old window. The arrow points at an actin bundle located between an endoplasmic chloroplast and the cell periphery. The fluorescence signal of the other actin bundles is attenuated beneath chloroplasts (e. g. asterisk) indicating that they are located along the inner, endoplasmic side of chloroplasts. (E) Subcortical actin bundles at a 1-week old window. (F) Depth coding image of the area indicated by an arrow in the z stack E. Purple-coloured actin bundles are close to the plasma membrane, yellow-coloured actin bundles are located at the inner, endoplasm-facing side of a resettled chloroplast (arrow). (G) Actin filaments in the cortex of a replenished window (image was taken 4 weeks after irradiation). Actin was visualized by green fluorescent phalloidin (C, H) and merged with red chlorophyll fluorescence in D and E. Images are single sections (A, B, G) and maximum projections of 20 (C, D) and 12 (E) optical sections with a thickness of 0.9 µm (C, D). Bars are 50 µm (A, B), 20 µm (E), 5 µm (C, D, F, G).
Supplementary Figure 3 | Orientation of resettled chloroplasts determined by passive endoplasmic flow. In windows located near the neutral line (NL) subcortical actin bundles and chloroplast files occasionally regenerate into the opposite streaming direction (lower arrow) along the passive cytoplasmic flow prevailing after irradiation. Image is a maximum projection of 15 optical sections with a thickness of 1.3 µm. Bar = 100 µm
Supplementary Figure 4 | Inhibition of endoplasmic streaming by cytochalasin reversibly delays the detachment of bleached chloroplasts and prevents the resettlement of endoplasmic chloroplasts. (A-E) Cell was treated with 10 µM cytochalasin D during and after irradiation; images A-C were taken 1 week after irradiation. Images D and E show the same window 1 day after recovery in AFW. (F) Area covered by bleached chloroplasts in % of irradiated area (window) in cells treated as described above. Data are means±SD, Asterisk indicates significant differences (P≤0.05; t-test; 22 cells from 6 different thalli were investigated). (G-L) Cell was irradiated and remained in AFW to allow the detachment of irradiated chloroplasts. After 1 d the cell was incubated in 10 µM cytochalasin D over a period of 7 weeks (compare Figure 9). Images A and D are maximum projections of 5 and 7 optical sections with a thickness of 1.3 µm; images B,C and E are single sections. Images G-L are false coloured projections of chlorophyll fluorescence (22 optical sections with a thickness of 1.36 µm) and were taken 1 day (G), 1 week (H), 3 weeks (I), 5 weeks (J) and 7 weeks (K) after irradiation. (L) is the comparison between the first (D) and the last picture (H) of this series. Bars are 50 µm.
Supplementary Figure 5 | Protein sequence alignment of different plant CHUPs. The alignment was performed with Clustal Omega (EMBL-EBI). Identical residues are highlighted in black, and numbering of amino acid residues begins at the first methionine. The following sequences were compared: Charophytes: Chara australis CHUP1 (CaCHUP1, this paper), Chara braunii hypothetical protein CBR_g4598 (CbCHUP, GenBank: GBG69767), Klebsormidium nitens CHUP1B (KnCHUP1B, GenBank: GAQ78600); liverworth: Marchantia paleacea hypothetical protein Mapa_014704 (MpCHUP, GenBank: KAG6543864); moss: Physcomitrium patens CHUP1 isoform X1 (PpCHUP, NCBI Reference Sequence: XP_024379808); fern: Adiantum capillus-veneris CHUP1A (AcvCHUP1A, GenBank: BAG54845); eudicot: Arabidopsis thaliana CHUP1 (AtCHUP1, GenBank: OAP01771); conserved domains and regions are displayed in different colours as indicated.
Supplementary Data Sheet 1 | Statistics results of inhibitor experiments with cytochalasin, oryzalin, paclitaxel.
Supplementary Data Sheet 2 | Statistics results of PAM fluorescence measurements control regions and border chloroplasts.
Supplementary Data Sheet 3 | Statistics results of PAM fluorescence measurements control regions and resettled chloroplasts.
Supplementary Video 1 | Rotational streaming in an internodal cell of Chara australis (chlorophyll fluorescence and bright field images). Note neutral line with opposite flow directions of the endoplasm.
Supplementary Video 2 | Giant rotating chloroplasts in the stagnant endoplasm at the neutral line (compare Figures 2D-F). Red chlorophyll fluorescence is merged with bright field image.
Supplementary Video 3 | Slowly streaming endoplasm 1 hour after local irradiation (Red chlorophyll fluorescence, bright field and merged images; compare Figures 2G-I).
Supplementary Video 4 | Wiggling motion of bleached chloroplasts 5 hours after irradiation.
Supplementary Video 5 | Recovery of continuous endoplasmic streaming at a window 1 day after local irradiation.
Supplementary Video 6 | Reversible inhibition of endoplasmic streaming by cytochalasin D (bright field images). Combined time series of an internodal cell taken before (left), 30 minutes after treatment with 20 cytochalasin D (middle) and after 1 hour recovery in artificial fresh water (right).
Supplementary Video 7 | Cytochalasin D inhibits the detachment of bleached chloroplasts. Cell was treated with 10 µM cytochalasin D during and after irradiation, images were taken 1 week after bleaching. Note stagnant endoplasm in the Z-stack of bright field images corresponding to Supplementary Figures 4A-C.
Supplementary Video 8 | Detachment of bleached chloroplasts after 1-day recovery from treatment with cytochalasin D in AFW (see Supplementary Video 7). Note recovery of endoplasmic streaming in the Z-stack of bright field images corresponding to Supplementary Figures 4D, E.
Abbreviations
ER, endoplasmic reticulum; cp-actin, chloroplast actin; CaCHUP1, Chara australis CHLOROPLAST UNUSUAL POSITIONING 1; GLMM, general linear mixed model; PAM, pulse amplitude modulation; Fv/Fm, maximum quantum efficiency of PSII; PAR, photosynthetically active radiation; AFW, artificial fresh water; CLSM, confocal laser scanning microscope.
References
Absolonova, M., Foissner, I., Sommer, A. (2018). Cubic plasma membrane domains stabilize and restrict zones for pH band formation in Chara internodal cells. Bot. Lett. 166, 283–293. doi: 10.1080/23818107.2018.1544508
Andersson, M. X., Goksör, M., Sandelius, A. S. (2007a). Membrane contact sites: Physical attachment between chloroplasts and endoplasmic reticulum revealed by optical manipulation. Plant Signal. Behav. 2, 185–187. doi: 10.4161/psb.2.3.3973
Andersson, M. X., Goksör, M., Sandelius, A. S. (2007b). Optical manipulation reveals strong attracting forces at membrane contact sites between endoplasmic reticulum and chloroplasts. J. Biol. Chem. 282, 1170–1174. doi: 10.1074/jbc.M608124200
Beilby, M. J. (2016). Multi-scale characean experimental system: From electrophysiology of membrane transporters to cell-to-cell connectivity, cytoplasmic streaming and auxin metabolism. Front. Plant Sci. 7. doi: 10.3389/fpls.2016.01052
Beilby, M. J., Bisson, M. A. (2012). “pH banding in charophyte algae,” in Plant Electrophysiology. Ed. Volkov, A. G. (Berlin, Heidelberg: Springer), 247–271. doi: 10.1007/978-3-642-29119-7_11
Beilby, M. J., Casanova, M. T. (2014). The physiology of characean cells. (Berlin, Heidelberg: Springer).
Buchnik, L., Abu-Abied, M., Sadot, E. (2015). Role of plant myosins in motile organelles: Is a direct interaction required? J. Integr. Plant Biol. 57, 23–30. doi: 10.1111/jipb.12282
Bulychev, A. A. (2019). Cyclosis-mediated intercellular transmission of photosynthetic metabolites in Chara revealed with chlorophyll microfluorometry. Protoplasma 256, 815–826. doi: 10.1007/s00709-018-01344-0
Bulychev, A. A. (2020). Transient depletion of transported metabolites in the streaming cytoplasm of Chara upon shading the long-distance transmission pathway. Biochim. Biophys. Acta 1861, 148257. doi: 10.1016/j.bbabio.2020.148257
Bulychev, A. A., Komarova, A. V. (2014). Long-distance signal transmission and regulation of photosynthesis in characean cells. Biochemistry (Moscow) 79, 273–281.
Chen, J. (1983). Effects of elevated centrifugal field on the Nitella cell and postcentrifugation patterns of its cytoplasmic streaming and chloroplast files. Cell Struct. Funct. 8, 109–118. doi: 10.1247/csf.8.109
Choi, H., Yi, T., Ha, S. H. (2021). Diversity of plastid types and their Interconversions. Front. Plant Sci. 12. doi: 10.3389/fpls.2021.692024
Delwiche, C. F., Cooper, E. D. (2015). The evolutionary origin of a terrestrial flora. Curr. Biol. 25, R899–R910. doi: 10.1016/j.cub.2015.08.029
Dodonova, S. O., Bulychev, A. A. (2011). Cyclosis-related asymmetry of chloroplast-plasma membrane interactions at the margins of illuminated area in Chara corallina cells. Protoplasma 248, 737–749. doi: 10.1007/s00709-010-0241-6
Domozych, D. S., Bagdan, K. (2022). The cell biology of charophytes: exploring the past and models for the future. Plant Physiol. 190, 1588–1608. doi: 10.1093/plphys/kiac390
Erickson, J. L., Schattat, M. H. (2018). Shaping plastid stromules-principles of in vitro membrane tubulation applied in planta. Curr. Opin. Plant Biol. 46, 48–54. doi: 10.1016/j.pbi.2018.07.003
Foissner, I. (1988). Chlortetracycline-induced formation of wall appositions (callose plugs) in internodal cells of Nitella flexilis (Characeae). J. Phycol. 24, 458–467. doi: 10.1111/j.1529-8817.1988.tb04248.x
Foissner, I. (1990). Wall appositions induced by ionophore A 23187, CaCl2, LaCl3, and nifedipine in characean cells. Protoplasma 154, 80–90. doi: 10.1007/BF01539835
Foissner, I., Sommer, A., Hoeftberger, M., Hoepflinger, M. C., Absolonova, M. (2016). Is wortmannin-induced reorganization of the trans-Golgi network the key to explain charasome formation? Front. Plant Sci. 7. doi: 10.3389/fpls.2016.00756
Foissner, I., Wasteneys, G. O. (1997). A cytochalasin-sensitive actin filament meshwork is a prerequisite for local wound wall deposition in Nitella internodal cells. Protoplasma 200, 17–30. doi: 10.1007/BF01280731
Foissner, I., Wasteneys, G. O. (1999). Microtubules at wound sites of Nitella internodal cells passively co-align with actin bundles when exposed to hydrodynamic forces generated by cytoplasmic streaming. Planta 208, 480–490. doi: 10.1007/s004250050585
Foissner, I., Wasteneys, G. O. (2007). Wide-ranging effects of eight cytochalasins and latrunculin A and B on intracellular motility and actin filament reorganization in characean internodal cells. Plant Cell Physiol. 48, 585–597. doi: 10.1093/pcp/pcm030
Foissner, I., Wasteneys, G. O. (2012). The characean internodal cell as a model system for studying wound healing. J. Microsc. 247, 10–22. doi: 10.1111/j.1365-2818.2011.03572.x
Foissner, I., Wasteneys, G. O. (2014). Characean internodal cells as a model system for the study of cell organization. Int. Rev. Cell Mol. Biol. 311, 307–364. doi: 10.1016/B978-0-12-800179-0.00006-4
Fu, C., Donovan, W. P., Shikapwashya-Hasser, O., Ye, X., Cole, R. H. (2014). Hot Fusion: an efficient method to clone multiple DNA fragments as well as inverted repeats without ligase. PloS One 9, e115318. doi: 10.1371/journal.pone.0115318
Gamalei, Y. V., Fromm, J., Krabel, D., Eschrich, W. (1994). Chloroplast movement as response to wounding in Elodea canadensis. J. Plant Physiol. 144, 518–524. doi: 10.1016/S0176-1617(11)82132-2
Green, P. B. (1964). Cinematic observations on the growth and division of chloroplasts in Nitella. Am. J. Bot. 51, 334–342. doi: 10.1002/j.1537-2197.1964.tb06640.x
Grolig, F., Williamson, R. E., Parke, J., Miller, C., Anderton, B. H. (1988). Myosin and Ca2+-sensitive streaming in the alga Chara: detection of two polypeptides reacting with a monoclonal anti-myosin and their localization in the streaming endoplasm. Eur. J. Cell Biol. 47, 22–31.
Hoepflinger, M. C., Geretschlaeger, A., Sommer, A., Hoeftberger, M., Nishiyama, T., Sakayama, H., et al. (2013). Molecular and biochemical analysis of the first ARA6 homologue, a RAB5 GTPase, from green algae. J. Exp. Bot. 64, 5553–5568. doi: 10.1093/jxb/ert322
Holzhausen, A., Stingl, N., Rieth, S., Kühn, C., Schubert, H., Rensing, S. A. (2022). Establishment and optimization of a new model organism to study early land plant evolution: Germination, cultivation and oospore variation of Chara braunii Gmeli. Front. Plant Sci. 13. doi: 10.3389/fpls.2022.987741
Jarosch, R. (1956). Plasmaströmung und Chloroplastenrotation bei Characeen. Phyton (Arg.) 6, 87–107.
Kamitsubo, E. (1972). A “window technique” for detailed observation of characean cytoplasmic streaming. Exp. Cell Res. 74, 613–616. doi: 10.1016/0014-4827(72)90430-2
Kasahara, M., Kagawa, T., Oikawa, K., Suetsugu, N., Miyao, M., Wada, M. (2002). Chloroplast avoidance movement reduces photodamage in plants. Nature 420, 829–832. doi: 10.1038/nature01213
Kimura, S., Kodama, Y. (2016). Actin-dependence of the chloroplast cold positioning response in the liverwort Marchantia polymorpha L. PeerJ 4, e2513. doi: 10.7717/peerj.2513
Kong, S.-G., Yamazaki, Y., Shimada, A., Kijima, S. T., Hirose, K., Katoh, K., et al. (2024). CHLOROPLAST UNUSUAL POSITIONING 1 is a plant-specific actin polymerization factor regulating chloroplast movement. Plant Cell 36, 1159–1181. doi: 10.1093/plcell/koad320
Krzeszowiec, W., Gabryś, H. (2007). Phototropin mediated relocation of myosins in Arabidopsis thaliana. Plant Signal. Behav. 2, 333–336. doi: 10.4161/psb.2.5.4509
Kurtović, K., Schmidt, V., Nehasilová, M., Vosolsobě, S., Petrášek, J. (2024). Rediscovering Chara as a model organism for molecular and evo-devo studies. Protoplasma 261, 183–196. doi: 10.1007/s00709-023-01900-3
Łabuz, J., Sztatelman, O., Hermanowicz, P. (2022). Molecular insights into the phototropin control of chloroplast movements. J. Exp. Bot. 73, 6034–6051. doi: 10.1093/jxb/erac271
Lichtscheidl, I. K., Url, W. G. (1990). Organization and dynamics of cortical endoplasmic reticulum in inner epidermal cells of onion bulb scales. Protoplasma 157, 203–215. doi: 10.1007/BF01322653
Lütz-Meindl, U., Aichinger, N. (2004). Use of energy-filtering transmission electron microscopy for routine ultrastructural analysis of high-pressure-frozen or chemically fixed plant cells. Protoplasma 223, 155–162. doi: 10.1007/s00709-003-0033-3
Maxwell, K., Johnson, G. N. (2000). Chlorophyll fluorescence - a practical guide. J. Exp. Bot. 51, 659–668. doi: 10.1093/jexbot/51.345.659
McCourt, R. M., Casanova, M. T., Perez, W., Proctor, V. W., Karol, K. G. (2011). Phylogeny of the Chareae (Charophyta) based on analysis of two plastid genes (Atpb, Rbcl) and implications for conventional taxonomy. J. Phycol. 47, 18–18. doi: 10.3390/plants10061157
McCourt, R. M., Karol, K. G., Hall, J. D., Casanova, T., Grant, M. C. (2017). “Charophyceae (Charales),” in Handbook of the Protists. Eds. Archibald, J. M., Simpson, A. G. B., Slamovits, C. H. (Springer International Publishing, Cham), 165–183.
Mineyuki, Y., Kataoka, H., Masuda, Y., Nagai, R. (1995). Dynamic changes in the actin cytoskeleton during the high- fluence rate response of the Mougeotia chloroplast. Protoplasma 185, 222–229. doi: 10.1007/BF01272863
Mitchell, A., Chang, H.-Y., Daugherty, L., Fraser, M., Hunter, S., Lopez, R., et al. (2014). The InterPro protein families database: the classification resource after 15 years. Nucleic Acids Res. 43, D213–D221. doi: 10.1093/nar/gku1243
Natesan, S. K. A., Sullivan, J. A., Gray, J. C. (2009). Myosin XI is required for actin-associated movement of plastid stromules. Mol. Plant 2, 1262–1272. doi: 10.1093/mp/ssp078
Nedo, A. O., Liang, H., Sriram, J., Razzak, M. A., Lee, J. Y., Kambhamettu, C., et al. (2024). CHUP1 restricts chloroplast movement and effector-triggered immunity in epidermal cells. New Phytol. 244, 1864–1881. doi: 10.1111/nph.20147
Nishiyama, T., Sakayama, H., de Vries, J., Buschmann, H., Saint-Marcoux, D., Ullrich, K. K., et al. (2018). The Chara genome: Secondary complexity and implications for plant terrestrialization. Cell 174, 448–464 e424. doi: 10.1016/j.cell.2018.06.033
Oikawa, K., Kasahara, M., Kiyosue, T., Kagawa, T., Suetsugu, N., Takahashi, F., et al. (2003). CHLOROPLAST UNUSUAL POSITIONING1 is essential for proper chloroplast positioning. Plant Cell 15, 2805–2815. doi: 10.1105/tpc.016428
Oikawa, K., Yamasato, A., Kong, S. G., Kasahara, M., Nakai, M., Takahashi, F., et al. (2008). Chloroplast outer envelope protein CHUP1 is essential for chloroplast anchorage to the plasma membrane and chloroplast movement. Plant Physiol. 148, 829–842. doi: 10.1104/pp.108.123075
Paves, H., Truve, E. (2007). Myosin inhibitors block accumulation movement of chloroplasts in Arabidopsis thaliana leaf cells. Protoplasma 230, 165–169. doi: 10.1007/s00709-006-0230-y
Pertl-Obermeyer, H., Lackner, P., Schulze, W. X., Hoepflinger, M. C., Hoeftberger, M., Foissner, I., et al. (2018). Dissecting the subcellular membrane proteome reveals enrichment of H+ (co-)transporters and vesicle trafficking proteins in acidic zones of Chara internodal cells. PloS One 13, e0201480. doi: 10.1371/journal.pone.0201480
Suetsugu, N., Dolja, V. V., Wada, M. (2010). Why have chloroplasts developed a unique motility system? Plant Signal. Behav. 5, 1190–1196. doi: 10.4161/psb.5.10.12802
Suetsugu, N., Higa, T., Wada, M. (2017). Ferns, mosses and liverworts as model systems for light-mediated chloroplast movements. Plant Cell Environ. 40, 2447–2456. doi: 10.1111/pce.12867
Suetsugu, N., Wada, M. (2016). Evolution of the cp-actin-based motility system of chloroplasts in green plants. Front. Plant Sci. 7. doi: 10.3389/fpls.2016.00561
Thomas, C., Tholl, S., Moes, D., Dieterle, M., Papuga, J., Moreau, F., et al. (2009). Actin bundling in plants. Cell Motil. Cytoskel. 66, 940–957. doi: 10.1002/cm.20389
Turmel, M., Otis, C., Lemieux, C. (2013). Tracing the evolution of streptophyte algae and their mitochondrial genome. Genome Biol. Evol. 5, 1817–1835. doi: 10.1093/gbe/evt135
Ueda, H., Tamura, K., Hara-Nishimura, I. (2015). Functions of plant-specific myosin XI: from intracellular motility to plant postures. Curr. Opin. Plant Biol. 28, 30–38. doi: 10.1016/j.pbi.2015.08.006
Wada, M., Kong, S. G. (2018). Actin-mediated movement of chloroplasts. J. Cell Sci. 131, 1–8. doi: 10.1242/jcs.210310
Wada, M., Kong, S.-G. (2019). “Chloroplast actin filaments involved in chloroplast photorelocation movements,” in The Cytoskeleton: Diverse Roles in a Plant’s Life. Eds. Sahi, V. P., Baluška, F. (Springer International Publishing, Cham), 37–48.
Wasteneys, G. O., Collings, D. A., Gunning, B. E. S., Hepler, P. K., Menzel, D. (1996). Actin in living and fixed characean internodal cells: Identification of a cortical array of fine actin strands and chloroplast actin rings. Protoplasma 190, 25–38. doi: 10.1007/BF01281192
Williamson, R. E. (1985). Immobilisation of organelles and actin bundles in the cortical cytoplasm of the alga Chara corallina Klein ex Wild. Planta 163, 1–8. doi: 10.1007/BF00395890
Williamson, R. E., Hurley, U. A. (1986). Growth and regrowth of actin bundles in Chara - bundle assembly by mechanisms differing in sensitivity to cytochalasin. J. Cell Sci. 85, 21–32. doi: 10.1242/jcs.85.1.21
Williamson, R. E., Hurley, U. A., Perkin, J. L. (1984). Regeneration of actin bundles in Chara: polarized growth and orientation by endoplasmic flow. Eur. J. Cell Biol. 34, 221–228.
Williamson, R. E., Mccurdy, D. W., Hurley, U. A., Perkin, J. L. (1987). Actin of Chara giant internodal cells - a single isoform in the subcortical filament bundles and a larger, immunologically related protein in the chloroplasts. Plant Physiol. 85, 268–272. doi: 10.1104/pp.85.1.268
Williamson, R. E., Perkin, J. L., Hurley, U. A. (1985). Selective extraction of Chara actin bundles: Identification of actin and two coextracting proteins. Cell Biol. Int. Rep. 9, 547–554. doi: 10.1016/0309-1651(85)90019-0
Keywords: actin cytoskeleton, characean internodal cells, chloroplast anchorage, CaCHUP1 (Chara australis chloroplast unusual positioning 1), confocal laser scanning microscopy, cortex regeneration, PAM fluorescence
Citation: Hoepflinger MC, Höftberger M, Sommer A, Hohenberger F, Schagerl M and Foissner I (2025) Recovery of the cortical chloroplast layer in the green alga Chara after local irradiation. Front. Plant Sci. 16:1544999. doi: 10.3389/fpls.2025.1544999
Received: 13 December 2024; Accepted: 25 March 2025;
Published: 05 May 2025.
Edited by:
Tomokazu Kawashima, University of Kentucky, United StatesReviewed by:
Justyna Maria Labuz, Jagiellonian University, PolandSam-Geun Kong, Kongju National University, Republic of Korea
Copyright © 2025 Hoepflinger, Höftberger, Sommer, Hohenberger, Schagerl and Foissner. This is an open-access article distributed under the terms of the Creative Commons Attribution License (CC BY). The use, distribution or reproduction in other forums is permitted, provided the original author(s) and the copyright owner(s) are credited and that the original publication in this journal is cited, in accordance with accepted academic practice. No use, distribution or reproduction is permitted which does not comply with these terms.
*Correspondence: Ilse Foissner, aWxzZS5mb2lzc25lckBwbHVzLmFjLmF0
†These authors have contributed equally to this work and share last authorship