- 1Department of Biology, Sultan Qaboos University, Muscat, Oman
- 2School of Life Sciences, University of Essex, Colchester, United Kingdom
Blue light exerts a profound influence on plant physiology by tightly regulating photosynthetic efficiency, developmental processes, and stress signaling networks. Within the photosynthetically active radiation range, blue wavelengths uniquely activate cryptochromes and phototropins, which in turn regulate processes such as chloroplast repositioning, phototropism, and transcriptional adjustments linked to stress mitigation. Under high intensity blue irradiation, photosynthetic electron transport chains and apoplastic NADPH oxidases generate reactive oxygen species (ROS), acting as key signaling intermediates yet posing oxidative challenges. Plants deploy intricate antioxidant defenses including superoxide dismutase, ascorbate peroxidase, catalase, and non-enzymatic scavengers like ascorbate, glutathione, and anthocyanins to maintain redox homeostasis and mitigate ROS damage. Emerging evidence indicates that the balance between beneficial and detrimental blue light effects is modulated by intensity, photoreceptor abundance, species-specific traits, and developmental context. This minireview explores the molecular and physiological responses to blue light, focusing on its role in stress signaling, reactive oxygen species (ROS) regulation, and antioxidant activity in plants.
1 Introduction
Sunlight, the fundamental energy source driving plant life and photosynthesis, extends beyond its role in photosynthesis to serve as a critical environmental cue that governs numerous aspects of plant physiology. It is composed of a broad electromagnetic spectrum, including ultraviolet (UV), infrared (IR), and visible light (VL) (Ahres et al., 2020; Pech et al., 2024). Within the visible light, blue light (400–500 nm), red light (600–700 nm) and green light (500-600nm) (Liu and van Iersel, 2021). Plants exhibit a unique interaction with light as specific wavelengths play key roles in driving their photosynthetic machinery. Historically, the photosynthetically active radiation (PAR) — the spectrum of light that facilitates photosynthesis — was delineated to span from 400 to 700 nm (McCree, 1972). Whitin this range, blue light (400–500 nm) plays an important role in photosynthesis, photomorphogenesis and plant development and nutrition (Trivellini et al., 2023). Blue light enhances photosynthetic efficiency by improving light absorption and triggering repairs mechanisms that protect against photodamage. It also plays a critical role in regulating plant growth regulation by activating specialized photoreceptors, notably cryptochromes (CRYPs) and phototropins (PHOTs), which mediate essential processes such as phototropism, stomatal opening, and chloroplast movement. (Cashmore et al., 1999; Briggs and Christie, 2002; Roeber et al., 2021). Additionally, blue light exposure provides enhances plant tolerance to frost and low temperatures by modulating the levels of key hormones, gibberellic acid (GA), salicylic acid (SA), and jasmonic acid (JA) (Ahres et al., 2023, 2025; Gulyás et al., 2025). A particular fascinating aspect of blue light signaling its dual role in the generation of reactive oxygen species (ROS). ROS function as a signaling molecules and potential sources of oxidative stress in plant cells (Jourdan et al., 2015). In plants, ROS generation is an inevitable consequence of light absorption and photosynthetic activity (Huang et al., 2019). While ROS, such as hydrogen peroxide (H2O2), superoxide anions (O2-), and singlet oxygen (¹O2), are normal byproducts of aerobic metabolism, blue light can enhance their production in chloroplasts, mitochondria, and peroxisomes (Banaś et al., 2012). This is particularly significant under high-intensity light, where the balance between energy capture and dissipation becomes strained, leading to overexcitation of the photosynthetic machinery (Consentino et al., 2015; Jourdan et al., 2015).
Under strong blue light irradiation, cryptochromes are activated, influencing the generation and accumulation of H2O2 at the at the plasma membrane and at the chloroplast surface (Müller and Ahmad, 2011; Consentino et al., 2015; El-Esawi et al., 2017). This response can be inhibited by ROS scavengers such as ascorbic acid, glutathione, mannitol or secondary metabolite (anthocyanin) when its added before illumination (Trivellini et al., 2023). Beside these non-enzymatic antioxidants, enzymatic antioxidants such as the peroxidase (POD), catalase, ascorbate peroxidase (APX), and superoxide dismutase (SOD) are able to attenuate the ROS generated under an excess of blue light illumination (Mastropasqua et al., 2012; Yu et al., 2017). Despite the growing body of literature on the impacts of blue light on plant physiology, many aspects remain insufficiently understood. Key questions persist: How do photoreceptors fine-tune ROS production and antioxidant defenses under varying environmental and developmental contexts? To what extent do species specific differences and genotypic variations modify blue light induced oxidative stress responses and antioxidant pathways? Therefore, in this minireview, we synthesize current knowledge regarding blue light perception, ROS generation, and antioxidant regulation in plants. Specifically, it is seeks to elucidate the relationship between blue light, receptors, and the specific antioxidant mechanisms that plants employ to cope with stress.
2 Blue light stress responses through photoreceptors
Plants use several classes of blue light receptors to modulate a range of physiological responses including both biotic and abiotic stresses (Roeber et al., 2021). Changes in light intensity and quality affect the plant redox machinery through ROS formation and antioxidant activity. Upon blue light exposure (390 to 500 nm), three classes of receptors are mainly modulated: cryptochromes (CRYP), phototropins (PHOT), and the members of the Zeiltupe (ZLT) family (D’Amico-Damião and Carvalho, 2018; Lin, 2000). Zeiltupe receptors are involved in setting up the circadian clock (Kim et al., 2007), whereas phototropins are plasma membrane photoreceptors that mediate phototropism, chloroplast movement, and oxidative stress responses (Demarsy et al., 2018; Rusaczonek et al., 2021).
Phototropins (PHOT1 and PHOT2) serve as sophisticated blue light sensors in plants. These cytosolic and plasma membrane-associated photoreceptors regulate key physiological responses, such as phototropism, chloroplast movement, and adaptation to oxidative stress. Each receptors contain two flavin mononucleotides (FMN) binding domains located within two conserved N-terminal sequences knows as LOV1 and LOV2 domains, as well as a C-terminal serine/threonine kinase (STK) domain responsible for initiating downstream signaling (Figure 1) (Xin et al., 2022). Light Oxygen Voltage (LOV) domains, functioning as blue light sensors, undergo autophosphorylation upon blue light photoexcitation (Christie et al., 1999; Christie 2007; Flores-Ibarra et al., 2023). These LOVs domains regulate stress responses through flavin mononucleotide-binding redox active cysteine (Yee et al., 2015). Flavin-binding proteins serve as signal receptors for redox potential, partial oxygen pressure (pO2), and blue light (Christie et al., 1999; Briggs and Christie, 2002; Oide et al., 2016). PHOT1 and PHOT2 proteins are structurally the same and under blue light irradiation, a portion of the PHOT1 protein is detached from the cell membrane and disperses in the cytoplasm. whereas, portion of the PHOT2 proteins can be couple to the Golgi apparatus (Wan et al., 2008; Kong and Wada, 2016). In addition, a fraction of PHOT2 has been detected on the chloroplast outer membrane (Kong et al., 2013). PHOT1 and PHOT2 are functionally distinct. While PHOT1 is activated only by low blue light intensity (< 1 μmol m−2 s−1), PHOT2 responds more effectively to high blue light intensities, ensuring efficient adaptation to varying light conditions (Sakai et al., 2001; Briggs and Christie, 2002). In Arabidopsis, PHOT1 and PHOT2 have partially overlapping functions and influence UV-C induced photooxidative stress responses, cell death, and photosynthesis (Rusaczonek et al., 2021). PHOT receptors induce chloroplast movement in response to blue light (Briggs et al., 2001; Wen et al., 2008). Blue light perceived by the PHOT1/PHOT2 receptors represses enhances drought tolerance by repressing the inhibitory function of the multifunctional E3 ubiquitin ligase constitutive photomorphogenic 1 (COP1) on open stomata resulting in closed stomata (Roeber et al., 2021). Arabidopsis phot1 and phot2 single and double mutants showed a reduced accumulation of H2O2 and more efficient photosynthetic electron transport compared to the wild type after an oxidative stress caused by UV-C treatment, whereas very little information is known about the blue light effect (Rusaczonek et al., 2021). PHOT2 functions under high blue light intensity by preventing the damage of the chloroplast photosynthetic apparatus and contribute to high light tolerance (Kagawa et al., 2001; Roeber et al., 2021).
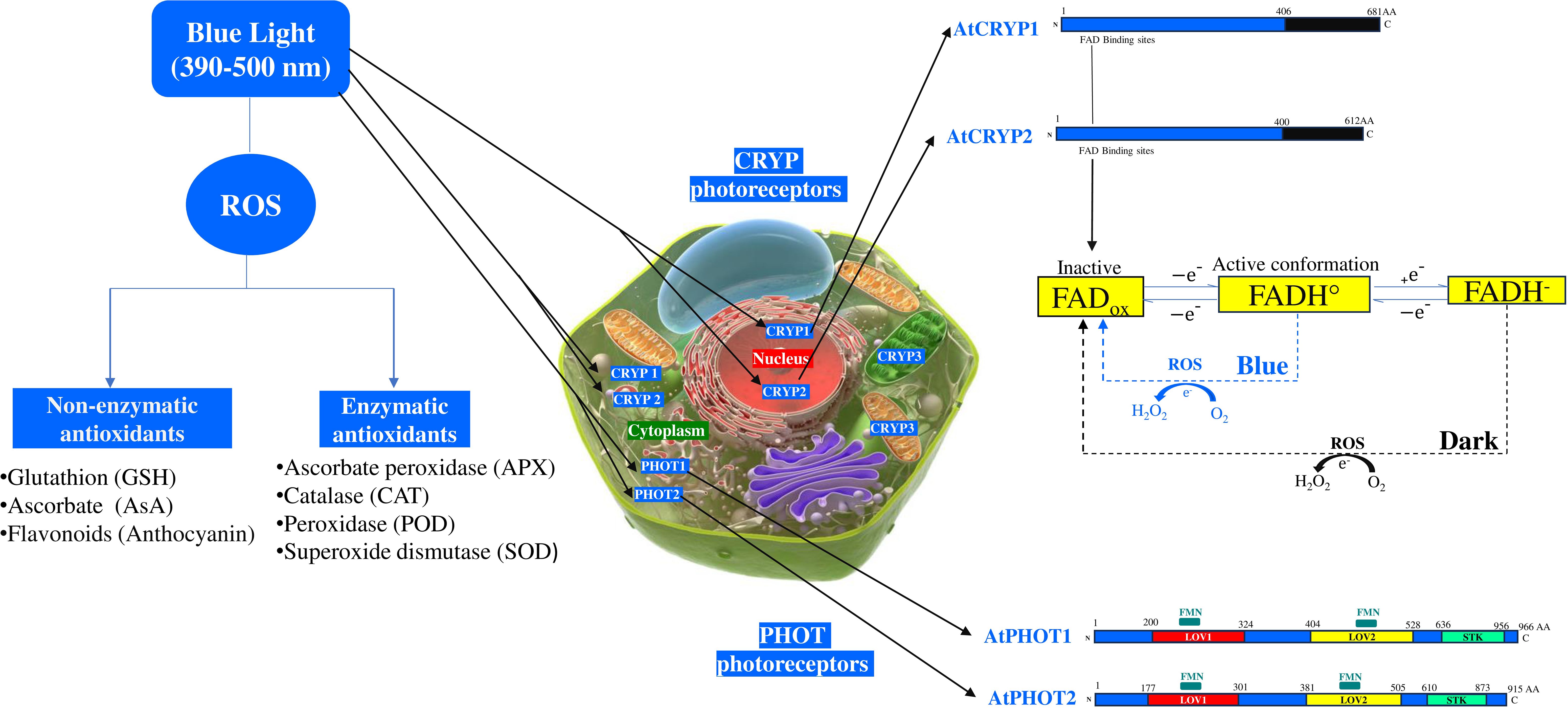
Figure 1. Effects of blue light on cryptochrome photoreceptors and enzymatic, non-enzymatic antioxidants. AtCRYP, Arabidopsis thaliana cryptochrome; AtPHOT, Arabidopsis thaliana phototropin; FADox, Flavin adenine dinucleotide oxidized; FADH°, Radical form of flavin adenine dinucleotide; FADH-, Reduced form of flavin adenine dinucleotide; FMN, Flavin mononucleotide binding domain; LOV, Light-Oxygen-Voltage-sensing domain; ROS, Reactive oxygen species; STK, Serine/Threonine Kinase domain.
Cryptochromes are flavin binding blue light receptors which are involved in the growth and development of plants, photoperiodic control of flowering, and reactive oxygen species (ROS) generation (Liu et al., 2011; El-Esawi et al., 2017). These receptors, primarily absorb in the blue light region and act as key regulators of a range of biotic stress responses, such as drought, salinity, heat, and high radiation (D’Amico-Damião and Carvalho, 2018). The Arabidopsis genome possess three cryptochrome genes, CRYP1, CRYP2, and CRYP3 which encodes their respective proteins. CRYP1 and CRYP2 are nuclear/cytoplasmic localized proteins, whereas CRYP3 is chloroplastic/mitochondrial (Figure 1) (Liu et al., 2011). Interestingly, nuclear CRYP2 can translocate to the cytoplasm, upon blue light exposure, suggesting dynamic spectral regulation (Jourdan et al., 2015). Whilst CRYP1 and CRYP2 are structurally similar, each containing a flavin adenine dinucleotide (FAD), but there are different in stability (Figure 1). The C-terminal part of the CRYP2 is shorter than CRYP1 and bears little homology (Ahmad et al., 1998). Unlike CRYP1 protein, CRYP2 is highly susceptible to degradation under various light fluences (green, UV, blue). CRY3 function as a photoreceptor in mitochondria and chloroplasts and it is part of the CRY-DASH clade of the photolyase/cryptochrome superfamily, which works to repair UV-damaged DNA in a light-dependent manner, however no study has yet confirmed their role under blue light fluence (Selby and Sancar, 2006; Mishra and Khurana, 2017). Upon blue light fluence, CRYP1 and CRYP2 are activated through interconversion of flavin redox states resulting in the production of ROS in the nucleus and potentially the cytoplasm (Bouly et al., 2007; Ahmad, 2016). The FAD, is a two-electron carrier that can be represented under different redox states or protonated forms: oxidized (FADox), semireduced (anion radical semiquinone FAD•— or neutral radical FADH•), and fully reduced flavin (FADH— or FADH2). Among the different redox forms, only FADox and FAD•— absorb significant amounts of blue light (~400–500nm) (Ahmad, 2016). This photon absorption is followed by an electron transfer via a tryptophan (W) pathway to excited state flavin from a proximal tryptophane residue (W) at position 397 (W397) in CRYP2 or at position 400 (W400) (in CRYP1) with the formation of FADH. Besides, the flavin-proximal W radical is turned reduced by a chain of intraprotein electron transfer through two further Trp residues W377 to W324 (CRYP1) or W374 to W321 (CRYP2) to a surface-exposed Tyr residue (Ahmed et al., 2016). Under dark condition, the flavin in the CRYP1 and CRYP2 are in inactive state (FADox), whereas under blue light illumination, it is reduced to FADH• (Liu et al., 2011; Fantini and Facella, 2020). Arabidopsis CRYP1 activation by blue light results in the conversion of molecular oxygen (O2) to ROS (Consentino et al., 2015). Overexpressing the CRYP1 induce H2O2 formation and cell death (Müller and Ahmad, 2011; Jourdan et al., 2015).
3 Roles of blue light on ROS synthesis and antioxidants activity
Blue light is an enhancer of ROS formation under both normal and high light intensity and the most represented ROS are: hydrogen peroxide (H2O2), superoxide radical (O2•¯), hydroxyl radical (HO•), and singlet oxygen (1O2) (Borbély et al., 2022; Rahman et al., 2025). ROS are produced in almost nearly every subcellular compartment and can diffuse across the membranes. The diffusion of ROS and the types produced vary according to the light intensity/quality, plant species and the environmental conditions (Wan et al., 2008; Borbély et al., 2022). High blue light (HBL) exposure leads to ROS production through multiple mechanisms. In the chloroplast thylakoid, electron spillover from the photosynthetic electron transport chain (ETC) generates ROS (El-Esawi et al., 2017; Borbély et al., 2022). These ROS can then be converted into H2O2, a harmful molecule that can diffuse into cytosol through chloroplast membranes (El-Esawi et al., 2017; Borbély et al., 2022). Additionally, the chlorophyll molecule itself contributes to ROS generation. During photosynthesis, if there is an excess of light energy that cannot be fully utilized or dissipated by the plant, chlorophyll can transfer excitation energy directly to oxygen, forming singlet oxygen (1O2) (Foyer and Hanke, 2022). Moreover, ROS accumulate in the cytosol after being converted to the damaging oxidant H2O2 and diffusing through chloroplast membranes. On the other hand, HBL activates the apoplastic NADPH oxidase (NOX) and the plasma membrane H+-ATPase enzymes, which increase the rate of O2•¯ generation and H+ transport across the plasma membrane (Wen et al., 2008; Xin et al., 2022). The O2•¯ generated is then converted to H2O2 by the superoxide dismutase (SOD) enzyme, which utilizes the H+ available in the apoplast due to H+-ATPase activity (Kinoshita and Shimazaki, 1999; Murakami et al., 2022). De novo H2O2 formed diffuses through the plasma membrane, forming a cytosolic ROS pool and alongside chloroplast-derived ROS (Borbély et al., 2022; Murakami et al., 2022). The accumulated cytosol ROS pool synthesized and the threshold of the cytosolic Ca2+ influences actin polymerization either directly by changing actin amino acids or indirectly by influencing the activities of actin-binding proteins (ABPs) (Rentel and Knight, 2004; Görlach et al., 2015). Actin polymerization/depolymerization enables chloroplasts to migrate along the plasma membrane to limit excessive light absorption (Xing et al., 2022).
Under blue light fluence, ROS can drive changes in the plant redox homeostasis. To cope with this harmful ROS generated under blue fluence, which leads to increased levels of singlet oxygen and hydrogen peroxide, plants increase the antioxidants biosynthesis such as the glutathione (GSH), ascorbic acid (AsA), anthocyanin and the detoxifying enzymes levels (Heyneke et al., 2013; Fantini and Facella, 2020; Rahman et al., 2025). In general, under high light intensity, the ROS generated such as the H2O2 can be scavenged in the cytosol and peroxisomes by AsA, and peroxidase (POD), in chloroplasts by AsA, GSH, and ascorbate peroxidase (APX) activity, and in vacuoles by AsA and POD (Figure 1) (Borbély et al., 2022). Under blue light illumination, the leaves and roots of Rehmannia glutinosa increased total antioxidant capacity and the activity of several antioxidant enzymes such as superoxide dismutase, catalase, ascorbate peroxidase, glutathione peroxidase (Manivannan et al., 2015). In leaves of Camptotheca acuminata seedlings, blue light increased H2O2, O2•¯ and stimulate the SOD and POD activities (Yu et al., 2017; Nam et al., 2018). In Boehmeria nivea (ramie), blue light stimulates the activities of SOD and POD (Rehman et al., 2020). Under blue light, the plant growth of Camptotheca acuminata seedlings was reduced, whereas SOD, POD, and catalase activities increased significantly (Yu et al., 2017). In oat leaves, enhanced APX activity was reported under blue light fluence (Mastropasqua et al., 2012). The accumulation of ROS under blue light fluence can be significantly decreased by applying green light treatment which, concurrently confers drought resistance to Cucumis melo seedlings (Li et al., 2024). Moreover, a combination of blue and red light significantly reduces the ROS (O2•-, H2O2) intensity in alfalfa seedlings by regulating the activity of the antioxidant enzymes such as ascorbate peroxidase, catalase, superoxide dismutase, and glutathione reductase, as well as the expression of genes related to the ascorbate-glutathione (AsA-GSH) pathway (Rahman et al., 2025) The ascorbate (AsA)/Glutathione (GSH) pathway is a major antioxidant defense mechanism that detoxifies ROS in plant cells during biotic and abiotic stress (Hasanuzzaman et al., 2019). GSH, AsA, and flavonoid (anthocyanin) are the major known non-enzymatic antioxidants triggered under blue light fluence (Toldi et al., 2019; Pech et al., 2024). H2O2 degradation is facilitated by the accumulation of the reduced forms of antioxidants such as ascorbate and glutathione in chloroplasts, peroxisomes and cytosol in Arabidopsis leaves (Heyneke et al., 2013). Under high light intensity, after the accumulation of ROS, the reduced GSH is oxidized to GSSG (oxidized glutathione) and AsA is oxidized to DHA (dehydroascorbic acid). The regeneration of the GSSG and DHA is insured, respectively, by glutathione reductase (GR) and dehydroascorbate reductase (DHAR) (Borbély et al., 2022; Zhou et al., 2023). Accumulation of the glutathione redox state varies depending on light intensity, type of species and the development stage of the plant. In wheat, the pool of GSSG is 2-fold higher in high and blue light compared to white light (Toldi et al., 2019). In young and flag wheat leaves, blue light exposure leads to a decrease in GSH content (Monostori et al., 2018). Conversely, Asghar et al. (2022) demonstrated that light intensity, especially under-far-red light, significantly enhances GSH content and freezing tolerance in two wheat genotypes. Blue light is effective in increasing AsA production in several plant species, including Lactuca sativa, Spinacea oleracea, Brassica compestris, and Avena Sativa leaves (Ohashi-Kaneko et al., 2007; Mastropasqua et al., 2012). The effectiveness of this increase depends on exposure time and light intensity (Mastropasqua et al., 2012). Under excessive blue light, ROS oxidize the AsA into DHA in lettuce leaves due to the elevated level of H2O2 (Zhou et al., 2023). The accelerated recycling or regeneration of the AsA pool can not only improve AsA levels but also decrease in the same time the ROS content in lettuce leaves (Raja et al., 2017; Zhou et al., 2023). ROS decomposition is accompanied by enhanced activity of APX, L-galactono-1,4-lactone dehydrogenase (GLDH) (AsA metabolism enzyme), and the recycling AsA enzymes such as: the dehydroascorbate reductase (DHAR) and the monodehydroascorbate reductase (MDHR) (Zhou et al., 2023). The AsA/GSH cycle and flavonoids, particularly anthocyanins, are knowns as complementary non-enzymatic antioxidant pathways involved in mitigating light induced oxidative stress (Liu et al., 2023; Foyer and Kunert, 2024). In several plant species, anthocyanins represent a crucial defense component against blue light induced (Sharma et al., 2021; Cai et al., 2023). This major class of flavonoids accumulates in the cell vacuoles and is triggered by ROS under blue light. Anthocyanins are photoprotective pigments that absorb the yellow, green and blue parts of the visible spectrum (Karageorgou and Manetas, 2006; Hughes and Smith, 2007). Under high light, anthocyanins can scavenge free radicals including DPPH· (1,1-diphenyl-2-picrylhy-drazyl, C18H13N5O6), superoxide anions and hydroxyl radicals (Zeng et al., 2009). The cross-regulation between ROS and anthocyanin production under blue light were analyzed through cryptochrome (cryp1/cryp2) knock-out genotypes of Arabidopsis, tomato and rapeseed seedlings mutants. It has been demonstrated that anthocyanins are accumulated in the cryp1 mutant, whereas no reduction in anthocyanins accumulation has been reported for cryp2 mutant (Lin, 2002; Chatterjee et al., 2006; Vandenbussche et al., 2007). Overexpressing tomato CRYP2 leads to high levels of anthocyanins in all seedling organs (Giliberto et al., 2005; Fantini and Facella, 2020), Moreover, it has been reported that under blue light, the CRY1Pa tomato mutant enhances the expression of bZIP transcription factor HY5 transcription factor, which acts downstream of cryptochromes and promotes anthocyanin biosynthesis (Liu et al., 2018). Blue light regulates the expression of PHOT genes, which encode PHOT1 and PHOT2 that play a central role in plant and algal adaptation to light excess (Sharma et al., 2021). These photoreceptors mediate photoprotective responses such as chloroplast avoidance movement, non-photochemical quenching, and carotenoid biosynthesis to avoid photooxidative damage (Li et al., 2009). While PHOT1 and PHOT2 function under low light, PHOT2 predominantly governs the chloroplast avoidance response under high blue light fluence in Arabidopsis, algae and mosses (Cazzaniga et al., 2013). PHOT genes contribute to photoprotection by modulating gene expression and interacting with other photoreceptors to maintain redox homeostasis and limit ROS accumulation. On other hands, Overexpressing PHOT2 protein in Fragaria ananassa fruits and leaves leads to an increase in anthocyanins pools and enhanced blue light stress resistance (Sharma et al., 2001).
4 Conclusion and future prospects
Blue light plays an important role in the growth regulation and plant development, and also participates in stress response. Understanding the complex interplay between blue light, ROS signaling, and antioxidant systems is crucial for developing strategies to improve plant stress tolerance and photosynthetic efficiency under harmful blue light conditions. One promising avenue lies in developing tailored lighting regimes that optimize blue light intensity, duration, and spectral composition to improve crop yield while minimizing excessive ROS production. Coupling these lighting solutions with a sensor-based system for real-time monitoring of ROS levels in real time could enable precision environmental management. In parallel, exploring the application of synthetic plant growth regulators or hormones that work synergistically with blue light to enhance stress resistance and yield could be a sustainable approach. Finally, alternating blue and green light, or combining red and blue light treatments can be a strategic alternative to reduce ROS formation and stimulate antioxidant production, thereby enhancing stress resistance, photosynthesis and increasing crop production. However, several key questions remain that warrant further investigation: (1) How do photoreceptors precisely calibrate ROS production and antioxidant defenses across different environmental contexts and developmental stages? (2) What molecular mechanisms underlie species-specific and genotypic variations in blue light responses? (3) How can we effectively translate our understanding of blue light signaling into practical applications for agriculture and horticulture? Further research is needed to elucidate the molecular mechanisms and genetic pathways that govern the crosstalk between blue light signaling, ROS generation, and antioxidant capacity in plants. Such insights will inform not only horticultural lighting strategies but also breeding projects and biotechnological interventions aimed at refining photoprotection and improving crop performance.
Author contributions
KC: Conceptualization, Investigation, Project administration, Resources, Supervision, Validation, Visualization, Writing – original draft, Writing – review & editing. HG: Validation, Visualization, Writing – original draft, Writing – review & editing. MF: Writing – original draft, Visualization, Validation, Writing – review & editing.
Funding
The author(s) declare that no financial support was received for the research and/or publication of this article.
Conflict of interest
The authors declare that the research was conducted in the absence of any commercial or financial relationships that could be construed as a potential conflict of interest.
Generative AI statement
The author(s) declare that no Generative AI was used in the creation of this manuscript.
Publisher’s note
All claims expressed in this article are solely those of the authors and do not necessarily represent those of their affiliated organizations, or those of the publisher, the editors and the reviewers. Any product that may be evaluated in this article, or claim that may be made by its manufacturer, is not guaranteed or endorsed by the publisher.
References
Ahmad, M. (2016). Photocycle and signaling mechanisms of plant cryptochromes. Curr. Opin. Plant Biol. 33, 108–115. doi: 10.1016/j.pbi.2016.06.013
Ahmad, M., Jarillo, J. A., and Cashmore, A. R. (1998). Chimeric proteins between cry1 and cry2 Arabidopsis blue light photoreceptors indicate overlapping functions and varying protein stability. Plant Cell. 10, 197–207. doi: 10.1105/tpc.10.2.197
Ahres, M., Gierczik, K., Boldizsár, Á., Vítámvás, P., and Galiba, G. (2020). Temperature and light-quality-dependent regulation of freezing tolerance in barley. Plants (Basel). 9, 83. doi: 10.3390/plants9010083
Ahres, M., Pálmai, T., Farkas, Z., Gulyás, Z., Soltész, A., Borbély, P., et al. (2025). Investigating the impact of spring (Vrn-A1) and winter (vrn-A1) vernalization alleles on frost tolerance induced by light spectrum and low temperatures in different wheat backgrounds. Env. Exp. Bot. 229, 106079. doi: 10.1016/j.envexpbot.2024.106079
Ahres, M., Pálmai, T., Kovács, T., Kovács, L., Lacek, J., Vanková, R., et al. (2023). The effect of white light spectrum modifications by excess of blue light on the frost tolerance, lipid- and hormone composition of barley in the early pre-hardening phase. Front. Plant Sci. 12, 40. doi: 10.3390/plants12010040
Asghar, M. A., Balogh, E., Szalai, G., Galiba, G., and Kocsy, G. (2022). Differences in the light-dependent changes of the glutathione metabolism during cold acclimation in wheat varieties with different freezing tolerance. J. Agron. Crop Sci. 208, 65–75. doi: 10.1111/jac.12566
Banaś, A. K., Aggarwal, C., Łabuz, J., Sztatelman, O., and Gabryś, H. (2012). Blue light signalling in chloroplast movements. J. Exp. Bot. 63, 1559–1574. doi: 10.1093/jxb/err429
Borbély, P., Gasperl, A., Pálmai, T., Ahres, M., Asghar, M. A., Galiba, G., et al. (2022). Light intensity- and spectrum-dependent redox regulation of plant metabolism. Antioxid. (Basel). 11, 1311. doi: 10.3390/antiox11071311
Bouly, J. P., Schleicher, E., Dionisio-Sese, M., Vandenbussche, F., Van Der Straeten, D., Bakrim, N., et al (2007). Cryptochrome blue light photoreceptors are activated through interconversion of flavin redox states. J. Biol. Chem. 282, 9383–9391. doi:10.1074/jbc.M609842200
Briggs, W. R., Beck, C. F., Cashmore, A. R., Christie, J. M., Hughes, J., Jarillo, J, et al. (2001). The phototropin family of photoreceptors. Plant Cell 13, 993–997. doi: 10.1105/tpc.13.5.993
Briggs, W. R. and Christie, J. M. (2002). Phototropins 1 and 2: versatile plant blue-light receptors. Trends Plant Sci. 7, 204–210. doi: 10.1016/s1360-1385(02)02245-8
Cai, C., Shem, J., and Chen, L. (2023). Content, composition, and biosynthesis of anthocyanin in fragaria species: A review. HortScience 58, 988–995. doi: 10.21273/HORTSCI17207-23
Cashmore, A. R., Jarillo, J. A., Wu, Y. J., and Liu, D. (1999). Cryptochromes: blue light receptors for plants and animals. Science 284, 760–765. doi:10.1126/science.284.5415.760
Cazzaniga, S., Dall’ Osto, L., Kong, S. G., Wada, M., and Bassi, R. (2013). Interaction between avoidance of photon absorption, excess energy dissipation and zeaxanthin synthesis against photooxidative stress in Arabidopsis. Plant J. 76, 568–579. doi: 10.1111/tpj.12314
Chatterjee, M., Sharma, P., and Khurana, J. P. (2006). Cryptochrome 1 from Brassica napus is up-regulated by blue light and controls hypocotyl/stem growth and anthocyanin accumulation. Plant Physiol. 141, 61–74. doi: 10.1104/pp.105.076323
Consentino, L., Lambert, S., Martino, C., Jourdan, N., Bouchet, P. E., Witczak, J., et al. (2015). Blue-light dependent reactive oxygen species formation by Arabidopsis cryptochrome may define a novel evolutionarily conserved signaling mechanism. New Phytol. 206, 1450–1462. doi: 10.1111/nph.13341
Christie, J. M. (2007). Phototropin blue-light receptors. Annu. Rev. Plant Biol. 58, 21–45. doi:10.1146/annurev.arplant.58.032806.103951
Christie, J. M., Salomon, M., Nozue, K., Wada, M., and Briggs, W. R. (1999). LOV (light, oxygen, or voltage) domains of the blue-light photoreceptor phototropin (nph1): binding sites for the chromophore flavin mononucleotide. Proc. Natl. Acad. Sci. U S A 96, 8779–8783. doi:10.1073/pnas.96.15.8779
D’Amico-Damião, V. and Carvalho, R. F. (2018). Cryptochrome-related abiotic stress responses in plants. Front. Plant Sci. 9. doi: 10.3389/fpls.2018.01897
Demarsy, E., Goldschmidt-Clermont, M., and Ulm, R. (2018). Coping with ‘Dark sides of the sun’ through photoreceptor. Signaling Trends Plant Sci. 23, 260–271. doi: 10.1016/j.tplants.2017.11.007
El-Esawi, M., Arthaut, L. D., Jourdan, N., d’Harlingue, A., Link, J., Martino, C. F., et al. (2017). Blue-light induced biosynthesis of ROS contributes to the signaling mechanism of Arabidopsis cryptochrome. Sci. Rep. 7, 13875. doi: 10.1038/s41598-017-13832-z
Fantini, E. and Facella, P. (2020). Cryptochromes: how blue light perception influences. Plant Physiol. eLS., 1–10. doi: 10.1002/9780470015902.a0028355
Flores-Ibarra, A., Maia, R. N. A., Olasz, B., Church, J. R., Gotthard, G., Schapiro, I., et al. (2023). Light-oxygen-voltage (LOV)-sensing domains: activation mechanism and optogenetic stimulation. J. Mol. Biol. 7, 168356. doi: 10.1016/j.jmb.2023.168356
Foyer, C. H. and Hanke, G. (2022). ROS production and signalling in chloroplasts: cornerstones and evolving concepts. Plant J. 111, 642–661. doi: 10.1111/tpj.15856
Foyer, C. H. and Kunert, K. (2024). The ascorbate-glutathione cycle coming of age. J. Exp. Bot. 75, 2682–2699. doi: 10.1093/jxb/erae023
Giliberto, L., Perrotta, G., Pallara, P., Weller, J. L., Fraser, P. D., Bramley, P. M., et al. (2005). Manipulation of the blue light photoreceptor cryptochrome 2 in tomato affects vegetative development, flowering time, and fruit antioxidant content. Plant Physiol. 137, 199–208. doi: 10.1104/pp.104.051987
Görlach, A., Bertram, K., Hudecova, S., and Krizanova, O. (2015). Calcium and ROS: A mutual interplay. Redox Biol. 6, 260–271. doi: 10.1016/j.redox.2015.08.010
Gulyás, Z., Ahres, M., Pálmai, T., Kulman, K., Tahmasebi, Z., Singh, K., et al. (2025). Blue or far-red light supplementation induced pre-hardening in the leaves of the Rht12 wheat dwarfing line: hormonal changes and freezing tolerance. Physiol. Plant 177, 70112. doi: 10.1111/ppl.70112
Hasanuzzaman, M., Bhuyan, M. H. M. B., Anee, T. I., Parvin, K., Nahar, K., Mahmud, J. A., et al. (2019). Regulation of ascorbate-glutathione pathway in mitigating oxidative damage in plants under abiotic stress. Antioxid. (Basel). 9, 384. doi: 10.3390/antiox8090384
Heyneke, E., Luschin-Ebengreuth, N., Krajcer, I., Wolkinger, V., Müller, M., and Zechmann, B. (2013). Dynamic compartment specific changes in glutathione and ascorbate levels in Arabidopsis plants exposed to different light intensities. BMC Plant Biol. 13, 104. doi: 10.1186/1471-2229-13-104
Huang, H., Ullah, F., Zhou, D. X., Yi, M., and Zhao, Y. (2019). Mechanisms of ROS regulation of plant development and stress responses. Front. Plant Sci. 10. doi: 10.3389/fpls.2019.00800
Hughes, N. M. and Smith, W. K. (2007). Attenuation of incident light in Galax urceolata (Diapensiaceae): concerted influence of adaxial and abaxial anthocyanic layers on photoprotection. Am. J. Bot. 94, 784–790. doi: 10.3732/ajb.94.5.784
Jourdan, N., Martino, C. F., El-Esawi, M., Witczak, J., Bouchet, P. E., d’Harlingue, A., et al. (2015). Blue-light dependent ROS formation by Arabidopsis cryptochrome-2 may contribute toward its signaling role. Plant Signal Behav. 10, e1042647. doi: 10.1080/15592324.2015.1042647
Kagawa, T., Sakai, T., Suetsugu, N., Oikawa, K., Ishiguro, S., Kato, T., et al. (2001). Arabidopsis NPL1: a phototropin homolog controlling the chloroplast high-light avoidance response. Science 291, 2138–2141. doi: 10.1126/science.291.5511.2138
Karageorgou, P. and Manetas, Y. (2006). The importance of being red when young: anthocyanins and the protection of young leaves of Quercus coccifera from insect herbivory and excess light. Tree Physiol. 26, 613–621. doi: 10.1093/treephys/26.5.613
Kim, W. Y., Fujiwara, S., Suh, S. S., Kim, J., Kim, Y., Han, L., et al. (2007). ZEITLUPE is a circadian photoreceptor stabilized by GIGANTEA in blue light. Nature 449, 356–360. doi: 10.1038/nature06132
Kinoshita, T. and Shimazaki, K. (1999). Blue light activates the plasma membrane H(+)-ATPase by phosphorylation of the C-terminus in stomatal guard cells. EMBO J. 18, 5548–5558. doi: 10.1093/emboj/18.20.5548
Kong, S. G., Suetsugu, N., Kikuchi, S., Nakai, M., Nagatani, A., and Wada, M. (2013). Both phototropin 1 and 2 localize on the chloroplast outer membrane with distinct localization activity. Plant Cell Physiol. 54, 80–92. doi: 10.1093/pcp/pcs151
Kong, S. G. and Wada, M. (2016). Molecular basis of chloroplast photorelocation movement. J. Plant Res. 129, 159–166. doi: 10.1007/s10265-016-0788-1
Li, Z., Wakao, S., Fischer, B. B., and Niyogi, K. K. (2009). Sensing and responding to excess light. Annu. Rev. Plant Biol. 60, 239–260. doi: 10.1146/annurev.arplant.58.032806.103844
Li, X., Zhao, S., Cao, Q., Qiu, C., Yang, Y., Zhang, G., et al. (2024). Effect of Green Light Replacing Some Red and Blue Light on Cucumis melo under Drought Stress. Int. J. Mol. Sci. 10, 25:7561. doi: 10.3390/ijms25147561
Lin, C. (2000). Plant blue-light receptors. Trends Plant Sci. 5, 337–342. doi: 10.1016/s1360-1385(00)01687-3
Lin, C. (2002). Blue light receptors and signal transduction. Plant Cell 14 Suppl, S207–S225. doi:10.1105/tpc.000646
Liu, C. C., Chi, C., Jin, L. J., Zhu, J., Yu, J. Q., and Zhou, Y. H. (2018). The bZip transcription factor HY5 mediates CRY1a-induced anthocyanin biosynthesis in tomato. Plant Cell Environ. 41, 1762–1775. doi: 10.1111/pce.13171
Liu, H., Liu, B., Zhao, C., Pepper, M., and Lin, C. (2011). The action mechanisms of plant cryptochromes. Trends Plant Sci. 16, 684–691. doi: 10.1016/j.tplants.2011.09.002
Liu, J. and van Iersel, M. W. (2021). Photosynthetic physiology of blue, green, and red light: light intensity effects and underlying mechanisms. Front Plant Sci. 12, 619987. doi: 10.3389/fpls.2021.619987
Liu, Y., Tang, L., Wang, Y., Zhang, L., Xu, S., Wang, X., et al. (2023). The blue light signal transduction module FaCRY1-FaCOP1-FaHY5 regulates anthocyanin accumulation in cultivated strawberry. Front. Plant Sci. 14. doi: 10.3389/fpls.2023.1144273
Manivannan, A., Soundararajan, P., Halimah, N., Ko, C. H., and Jeong, B. R. (2015). Blue LED light enhances growth, phytochemical contents, and antioxidant enzyme activities of Rehmannia glutinosa cultured in vitro. Hortic. Environ. Biotechnol. 56, 105–113. doi: 10.1007/s13580-015-0114-1
Mastropasqua, L., Borraccino, G., Bianco, L., and Paciolla, C. (2012). Light qualities and dose influence ascorbate pool size in detached oat leaves. Plant Sci. 183, 57–64. doi: 10.1016/j.plantsci.2011.11.009
McCree, K. J. (1972). Test of current definitions of photosynthetically active radiation against leaf photosynthesis data. Agric. Meteorol. 10, 443–453. doi: 10.1016/0002-1571(72)90045-3
Mishra, S. and Khurana, J. P. (2017). Emerging roles and new paradigms in signaling mechanisms of plant cryptochromes. Crit. Rev. Plant Sci. 36, 89–115. doi: 10.1080/07352689.2017.1348725
Monostori, I., Heilmann, M., Kocsy, G., Rakszegi, M., Ahres, M., Altenbach, S. B., et al. (2018). LED lighting - modification of growth, metabolism, yield and flour composition in wheat by spectral quality and intensity. Front. Plant Sci. 4. doi: 10.3389/fpls.2018.00605
Müller, P. and Ahmad, M. (2011). Light-activated cryptochrome reacts with molecular oxygen to form a flavin-superoxide radical pair consistent with magnetoreception. J. Biol. Chem. 286, 21033–21040. doi: 10.1074/jbc.M111.228940
Murakami, N., Fuji, S., Yamauchi, S., Hosotani, S., Mano, J., and Takemiya, A. (2022). Reactive carbonyl species inhibit blue-light-dependent activation of the plasma membrane H+-ATPase and stomatal opening. Plant Cell Physiol. 17, 631168–631176. doi: 10.1093/pcp/pcac094
Nam, T. G., Kim, D. O., and Eom, S. H. (2018). Effects of light sources on major flavonoids and antioxidant activity in common buckwheat sprouts. Food Sci. Biotechnol. 27, 169–176. doi: 10.1007/s10068-017-0204-1
Ohashi-Kaneko, K., Takase, M., Kon, N., Fujiwara, K., and Kurata, K. (2007). Effect of light quality on growth and vegetable quality in leaf lettuce, spinach and komatsuna. Environ. Control. Biol. 45, 189–198. doi: 10.2525/ecb.45.189
Oide, M., Okajima, K., Kashojiya, S., Takayama, Y., Oroguchi, T., Hikima, T., et al. (2016). Blue light-excited light-oxygen-voltage-sensing domain 2 (LOV2) triggers a rearrangement of the kinase domain to induce phosphorylation activity in arabidopsis phototropin1. J. Biol. Chem. 291, 19975–19984. doi: 10.1074/jbc.M116.735787
Pech, R., Volná, A., Špunda, V., and Nezval, J. (2024). Blue light as an important factor increasing plant tolerance to acute photooxidative stress. Environ. Exp. Bot. 226, 105923. doi: 10.1016/j.envexpbot.2024.105923
Rahman, M. A., Lee, S. H., Park, H. S., Min, C. W., Woo, J. H., Choi, B. R., et al. (2025). Light quality plays a crucial role in regulating germination, photosynthetic efficiency, plant development, reactive oxygen species production, antioxidant enzyme activity, and nutrient acquisition in alfalfa. Int. J. Mol. Sci. 3, 26:360. doi: 10.3390/ijms26010360
Raja, V., Majeed, U., Kang, H., Andrabi, K. I., and John, R. (2017). Abiotic stress: Interplay between ROS, hormones and MAPKs. Environ. Exp. Bot. 137, 142–157. doi: 10.1016/j.envexpbot.2017.02.010
Rehman, M., Fahad, S., Saleem, M. H., Hafeez, M., Ur Rahman, M. H., Liu, F., et al. (2020). Red light optimized physiological traits and enhanced the growth of ramie (Boehmeria nivea L.). Photosynthetica 58, 922–931. doi: 10.32615/ps.2020.040
Rentel, M. C. and Knight, M. R. (2004). Oxidative stress-induced calcium signaling in Arabidopsis. Plant Physiol. 135, 1471–1479. doi: 10.1104/pp.104.042663
Roeber, V. M., Bajaj, I., Rohde, M., Schmülling, T., and Cortleven, A. (2021). Light acts as a stressor and influences abiotic and biotic stress responses in plants. Plant Cell Environ. 44, 645–664. doi: 10.1111/pce.13948
Rusaczonek, A., Czarnocka, W., Willems, P., Sujkowska-Rybkowska, M., Van Breusegem, F., and Karpiński, S. (2021). Phototropin 1 and 2 influence photosynthesis, UV-C induced photooxidative stress responses, and cell death. Cells 10, 200. doi: 10.3390/cells10020200
Sakai, T., Kagawa, T., Kasahara, M., Swartz, T. E., Christie, J. M., Briggs, W. R., et al. (2001). Arabidopsis nph1 and npl1: blue light receptors that mediate both phototropism and chloroplast relocation. Proc. Natl. Acad. Sci. USA. 98, 6969–6974. doi: 10.1073/pnas.101137598
Selby, C. P. and Sancar, A. (2006). A cryptochrome/photolyase class of enzymes with single-stranded DNA-specific photolyase activity. Proc. Natl. Acad. Sci. USA. 103, 17696–17700. doi: 10.1073/pnas.0607993103
Sharma, S., Sanyal, S. K., Sushmita, K., Chauhan, M., Sharma, A., Anirudhan, G., et al. (2021). Modulation of phototropin signalosome with artificial illumination holds great potential in the development of climate-smart crops. Curr. Genomics 18, 181–213. doi: 10.2174/1389202922666210412104817
Toldi, D., Gyugos, M., Darkó, É., Szalai, G., Gulyás, Z., Gierczik, K., et al. (2019). Light intensity and spectrum affect metabolism of glutathione and amino acids at transcriptional level. PloS One 31, 14:e0227271. doi: 10.1371/journal.pone.0227271
Trivellini, A., Toscano, S., Romano, D., and Ferrante, A. (2023). The role of blue and red light in the orchestration of secondary metabolites, nutrient transport and plant quality. Plants (Basel). 18, 2026. doi: 10.3390/plants12102026
Vandenbussche, F., Habricot, Y., Condiff, A. S., Maldiney, R., van der Straeten, D., and Ahmad, M. (2007). HY5 is a point of convergence between cryptochrome and cytokinin signalling pathways in Arabidopsis thaliana. Plant J. 49, 428–441. doi: 10.1111/j.1365-313X.2006.02973.x
Wan, Y. L., Eisinger, W., Ehrhardt, D., Kubitscheck, U., Baluska, F., and Briggs, W. (2008). The subcellular localization and blue-light-induced movement of phototropin 1-GFP in etiolated seedlings of Arabidopsis thaliana. Mol. Plant 1, 103–117. doi: 10.1093/mp/ssm011
Wen, F., Xing, D., and Zhang, L. (2008). Hydrogen peroxide is involved in high blue light-induced chloroplast avoidance movements in Arabidopsis. J. Exp. Bot. 59, 2891–2901. doi: 10.1093/jxb/ern147
Xin, G. Y., Li, L. P., Wang, P. T., Li, X. Y., Han, Y. J., and Zhao, X. (2022). The action of enhancing weak light capture via phototropic growth and chloroplast movement in plants. Stress Biol. 1, 50. doi: 10.1007/s44154-022-00066-x
Yee, E. F., Diensthuber, R. P., Vaidya, A. T., Borbat, P. P., Engelhard, C., Freed, J. H., et al. (2015). Signal transduction in light-oxygen-voltage receptors lacking the adduct-forming cysteine residue. Nat. Commun. 6, 10079. doi: 10.1038/ncomms10079
Yu, W., Liu, Y., Song, L., Jacobs, D. F., Du, X., Ying, Y., et al. (2017). Effect of differential light quality on morphology, photosynthesis, and antioxidant enzyme activity in camptotheca acuminata seedlings. J. Plant Growth Regul. 36, 148–160. doi: 10.1007/s00344-016-9625-y
Zeng, X. Q., Chow, W. S., Su, L. J., Peng, X. X., and Peng, C. L. (2009). Protective effect of supplemental anthocyanins on Arabidopsis leaves under high light. Physiol. Plant 138, 215–225. doi: 10.1111/j.1399-3054.2009.01316.x
Keywords: antioxidants, blue light, photoreceptors, ROS, stress response
Citation: Chibani K, Gherli H and Fan M (2025) The role of blue light in plant stress responses: modulation through photoreceptors and antioxidant mechanisms. Front. Plant Sci. 16:1554281. doi: 10.3389/fpls.2025.1554281
Received: 01 January 2025; Accepted: 23 April 2025;
Published: 16 May 2025.
Edited by:
Baris Uzilday, Ege University, TürkiyeReviewed by:
Chunhong Yang, Chinese Academy of Sciences (CAS), ChinaKlára Kosová, Crop Research Institute (CRI), Czechia
Alfonso Ortega Garrido, Universidad de Extremadura, Spain
Copyright © 2025 Chibani, Gherli and Fan. This is an open-access article distributed under the terms of the Creative Commons Attribution License (CC BY). The use, distribution or reproduction in other forums is permitted, provided the original author(s) and the copyright owner(s) are credited and that the original publication in this journal is cited, in accordance with accepted academic practice. No use, distribution or reproduction is permitted which does not comply with these terms.
*Correspondence: Kamel Chibani, ay5jaGliYW5pQHNxdS5lZHUub20=
†ORCID: Kamel Chibani, orcid.org/0009-0000-5754-0284
Hussein Gherli, orcid.org/0000-0002-9714-4179
Mengjie Fan, orcid.org/0009-0001-8909-8651