- 1School of Agriculture, Forestry and Medicine, The Open University of China, Beijing, China
- 2State Key Laboratory of Plateau Ecology and Agriculture, Qinghai University, Xining, China
- 3Ministry of Education Key Laboratory of Bioinformatics, Center for Plant Biology, School of Life Sciences, Tsinghua University, Beijing, China
- 4National Key Laboratory of Tropical Crop Breeding, Institute of Tropical Bioscience and Biotechnology and Sanya Research Institute, Chinese Academy of Tropical Agricultural Sciences, Hainan, China
Plants cope with Pi deficiency by triggering an array of adaptive responses, including the remodeling of root system architecture (RSA). Arabidopsis thaliana grown on a Pi-deficient (-Pi) medium in transparent Petri dishes exhibits an inhibition of primary root (PR) growth. Previous work has shown that direct illumination on roots by blue light is both required and sufficient for the Pi deficiency-induced inhibition of PR growth. However, whether light illumination on shoots of seedlings contributes to the inhibition of PR growth under -Pi condition and whether light signaling pathway is involved in this process remain largely unknown. In addition to Pi deficiency-induced inhibition of PR growth, how light affects the transcriptomic changes under -Pi also remains elusive. Here, we found that the inhibition of PR growth under -Pi condition is determined by light illumination on roots instead of shoots. Further experiments revealed that blue light receptors CRY1/CRY2 and key regulator in blue light signaling pathway HY5 play minor roles in this process. Finally, we evaluated the light effects on the transcriptomic changes during the inhibition of PR growth under -Pi condition. We found that light promotes the expression of many genes involved in stress and phytohormones-related processes and has both upregulated and downregulated effects on the expression of typical phosphate starvation-induced (PSI) genes. Taken together, our work further demonstrates our previous hypothesis that the inhibition of PR growth under -Pi condition is caused by blue light-triggered chemical reactions, rather than blue light signaling pathways. Apart from the inhibition of PR growth under -Pi, light exposure also results in substantial alterations of transcriptome under -Pi condition, encouraging us to carefully evaluate the phenotype under illuminated, transparent Petri dishes.
Introduction
Phosphorus (P) is an essential macronutrient for plant growth, development, and metabolism. Plants uptake P from soil in the form of inorganic phosphate (Pi), which is quite limited in soils due to its low diffusion rate, fixation with metals, and conversion to organophosphates by microorganisms (Nussaume et al., 2011; Cong et al., 2020). To cope with Pi deficiency, plants have evolved sophisticated adaptive responses called Pi starvation responses (PSR) to sustain their growth, development, and reproduction (López-Arredondo et al., 2014). The major PSR of several plant species is the remodeling of root system architecture (RSA), including the inhibition of primary root (PR) growth, the increase of lateral roots and root hairs (Liu, 2021).
In the past decades, most studies of Arabidopsis root biology have used illuminated, transparent Petri dishes because it is simply easier to control the environment and collect data with experiments in Petri dishes than those in soil. When grown in Petri dishes under -Pi condition, Arabidopsis exhibits an inhibition of PR growth (Liu, 2021). Previous studies demonstrated that iron (Fe) accumulation in root apoplasts and secretion of malate into the rhizosphere are critical for Pi deficiency-induced inhibition of PR growth in Arabidopsis. Functional disruption of LPR1 (a ferroxidase that oxidizes Fe2+ to Fe3+) and its close homolog LPR2, ALMT1 (an aluminum-activated malate transporter), and STOP1 (a transcription factor that directly regulates the transcription of ALMT1) reduced the accumulation of Fe3+ in root apoplasts and make plants insensitive to Pi deficiency-induced inhibition of PR growth (Svistoonoff et al., 2007; Müller et al., 2015; Balzergue et al., 2017; Mora-Macías et al., 2017; Wang et al., 2019). In contrast, mutation of ALS3 (a transmembrane domain of a putative ABC transporter complex), STAR1 (a nucleotide binding domain of a putative ABC transporter complex), two of the uncharacterized cytochrome b561 and DOMON domain (CYBDOM) protein family members, CRR and HYP1, over-accumulates Fe3+ in the root apoplasts and make plants hypersensitive to Pi deficiency-induced inhibition of PR growth (Dong et al., 2017; Clúa et al., 2024; Maniero et al., 2024).
Our laboratory previously reported that Pi deficiency-induced inhibition of PR growth is caused by a blue light-mediated Photo-Fenton reaction that couples with a canonical Fenton reaction to form a Fe redox cycle. The resultant Fe redox cycle generates hydroxyl radicals (·OH, a strong reactive oxygen species), thus inhibiting PR growth (Zheng et al., 2019). Although the strong inhibition of PR growth under -Pi condition is largely rescued by shielding of roots from light, which was supported by other research groups (Silva-Navas et al., 2019; Yeh et al., 2020; Gao H, et al., 2021; Tian et al., 2021), whether Pi deficiency-induced accumulations of Fe, callose, lignin, and ·OH are influenced by light illumination and the effects of light on transcriptomic changes under -Pi condition in roots have not been investigated. In contrast, Gao Y. Q. et al. (2021) claimed that inhibition of root elongation by -Pi requires blue light signal perception at the shoot and transduction to the root. In this process, after light perception by blue light receptor CRY1/CRY2, a crucial downstream component, HY5, migrates from shoot to root. The shoot-derived HY5 autoactivates root HY5 and regulates PR growth by directly activating the expression of LPR1. Another study revealed that the insensitive phenotype to PR growth inhibition of hy5 mutant might be due to blockage of blue light responses (Yeh et al., 2020). Therefore, light exposure on which part (shoots or roots) is vital to trigger Pi deficiency-induced inhibition of PR growth and whether blue light signaling pathway contributes to this process remain to be investigated.
In the current study, we demonstrate that the inhibition of PR growth under -Pi condition is determined by light illumination on roots but not shoots. Further results revealed that blue light signaling pathway plays a minor role in this process. Through transcriptomic analyses, we found that light promotes the expression of a group of stress and phytohormone-related genes and has both upregulation and downregulation of the expression of typical PSI genes. These results support our previous hypothesis that the inhibition of PR growth under -Pi condition is caused by blue light-triggered chemical reactions. We therefore suggest that re-evaluation is needed of the many published studies that used illuminated Petri dishes to investigate root responses to environmental conditions.
Materials and methods
Plant materials and growth conditions
All Arabidopsis (Arabidopsis thaliana) plants used in this study were in the Columbia-0 background. The cry1-104cry2-1 mutant (Mao et al., 2005) was kindly provided by Dr. Hongquan Yang (Shanghai Normal University, China). The hy5-215 (Oyama et al., 1997), HY5 OX-1, and HY5 OX-2 lines were kind gifts from Dr. Haodong Chen (Tsinghua University, China). The lpr1lpr2 mutant (Svistoonoff et al., 2007) was kindly provided by Dr. Thierry Desnos (CEA Cadarache, France). The LPR1 OX-1 and LPR1 OX-2 lines were generated in our laboratory (Wang et al., 2019).
The Arabidopsis seeds were surface sterilized for 10 min with 20% (v/v) bleach and then washed three times with sterile-distilled water (ddH2O). After stratification at 4°C for 2 days, the seeds were sown on Petri dishes containing Pi-sufficient (+Pi) medium or Pi-deficient (-Pi) medium. The standard +Pi medium was half-strength Murashige and Skoog medium (Caisson Labs, MSP01-01190008) with 1% (w/v) sucrose, 0.1% (w/v) MES, and 0.8% (w/v) agarose (Biowest Regular Agarose G-10). For the -Pi medium, half-strength Murashige & Skoog without Pi (Caisson Labs, MSP11-05160009) was used to replace Murashige & Skoog basal salts. The pH was adjusted to 5.8 for both +Pi and -Pi media. The Petri dishes with Arabidopsis seeds were placed vertically in a phytotron with a photoperiod of 16 h of white light and 8 h of dark at 22-24°C. The intensity of white light was 100 µmol m–2 s–1.
To keep the shoots and roots in darkness, respectively, in light shielding experiment, we used autoclaved aluminum foil that was placed above the medium to cover the shoots or roots of 4-day-old seedlings. Moreover, we employed black plastic film in the rear of the plates to prevent the light from passing through the back (Zheng et al., 2019). The light was shone on the seedlings from above. Despite we did not insert the aluminum foil into the medium, as the transplanted 4-day-old seedlings were grown at a distance from the edges of the plates, the influence of light leakage from the sides that were not covered with aluminum foil on the root phenotypes might be negligible.
Perls and Perls/DAB staining assays
The Perls staining method was performed as described with minor modifications (Roschzttardtz et al., 2009). Briefly, roots were stained with a Perls stain kit (Solarbio) for 30 min and rinsed with ddH2O twice. Then the stained roots were stored in 0.1 M Na-Phosphate buffer (pH 7.4).
The Perls/DAB staining was performed as described with small modifications (Balzergue et al., 2017). The final concentration of DAB solution was 0.025%. The stained roots were stored in 0.1 M Na-Phosphate buffer (pH 7.4). All stained samples were cleared on glass slides by 80% HCG clearing solution (diluted with 0.1 M Na-Phosphate buffer, pH 7.4). The Fe staining patterns in roots were examined using a 20x objective with a differential interference contrast (DIC) microscope (Olympus BX51) equipped with a camera (Olympus DP71).
Callose staining assay
The callose staining was performed as described with minor modifications (Müller et al., 2015). Callose was stained with 0.1% (w/v) aniline blue (AppliChem) in 100 mM Na-phosphate buffer (pH 9.0) for 1.5 h. The callose staining patterns in roots were examined with a LSM710 confocal microscope (Zeiss).
Lignin staining assay
The lignin staining was conducted as described with minor modifications (Ziegler et al., 2016). The roots were totally covered with 5% phloroglucinol solution and inoculated at room temperature for 3 min. The same volume of HCl solution was then added for 3 min in room temperature. Approximate 40% glycerol was added to the stained roots before observation with the microscope (Olympus BX51).
Histochemical detection of ·OH in roots
The ·OH staining was conducted as described before (Setsukinai et al., 2003; Zheng et al., 2019). Roots were excised from 5-day-old seedlings and were pre-incubated in a 100 mM phosphate buffer (pH 6.1) for 20 min. The samples were then transferred to the same buffer containing 10 µM HPF (hydroxyphenyl fluorescein), and incubated for another 20 min. The fluorescent signals representing ·OH were observed with an LSM710 confocal microscope (Zeiss).
Analysis of root-associated APase activity
For histochemical staining of APase activity on the root surface of Arabidopsis seedlings, an agar solution (0.5%, w/v) containing 0.01% (w/v) BCIP was evenly overlaid on the roots grown on agar plates (Wang et al., 2011). After 12 h of color development, the roots were photographed with a camera attached to a stereomicroscope (Olympus SZ61).
Reverse transcription quantitative PCR analyses of PSI gene expression
Four-day-old +Pi seedlings were transferred to +Pi or -Pi medium with roots under light or in darkness for another four days. Then total RNA was extracted from roots using the Highpure Total RNA Mini kit (Magen). M-MLV reverse transcriptase (Takara) was used to reversely transcribe RNAs into cDNAs. SYRB Fast qPCR Master Mix (KAPA) was used for RT-qPCR analyses on a Bio-Rad CFX96 real-time PCR system. ACTIN2 was used as an internal control, and the relative expression level of each gene was calculated by the 2-ΔΔCt method (Livak and Schmittgen, 2001). The primers used for RT-qPCR analyses are listed in Supplementary Table S1.
Transcriptomic analysis
Four-day-old +Pi seedlings were transferred to +Pi or -Pi medium with roots under light or in darkness for another four days. The total RNA was extracted from roots and 3 μg of RNA for each sample was used for library construction and subsequent RNA-deep sequencing on the Illumina Hiseq 2500 platform. Three biological replicates were used in this experiment. The adaptor sequences and low quality sequences were removed. Approximately 6.0 GB of clean reads were generated from each sample. The clean reads were mapped to the Arabidopsis reference genome (TAIR10) using TOPHAT v.2.1.0 with TAIR10 gene annotation as the transcript index. The minimum and maximum intron lengths were set to 40 and 5000 separately. CUFFLINKS v.2.2.1 was used to assemble the new transcripts. HTSEQ v.0.6.0 was used to calculate the raw read counts for each gene. Gene expression normalization among samples was performed by using DESEQ2. The different gene expression data were collected from the comparison with a fold change ≥ 2 and a false discovery rate (FDR, Benjamini-Hochberg adjusted P value) ≤ 0.01. Venn diagram was drawn by Venny 2.1.0 (https://bioinfogp.cnb.csic.es/tools/venny/). GO enrichment was performed using Metascape (https://metascape.org/gp/index.html). Heatmap analyses and hierarchical clustering were conducted by Morpheus (https://software.broadinstitute.org/morpheus/).
Results
Light illumination on roots, but not shoots, is both required and sufficient for Pi deficiency-induced inhibition of PR growth
Previous studies have revealed that Pi deficiency-induced inhibition of PR growth was largely rescued by shielding of roots from light (Silva-Navas et al., 2019; Zheng et al., 2019; Gao H, et al., 2021; Tian et al., 2021). To further assess the contributions of shoots and roots in Pi deficiency-induced inhibition of PR growth, we conducted a detailed light shielding experiment. The wild-type (WT) Arabidopsis seeds were directly germinated on Pi-sufficient (+Pi) medium. Four days after germination (DAG), the seedlings were transferred to +Pi or Pi-deficient (-Pi) medium under white light illumination with different shielding of shoots and roots (Figure 1A). At four days after transfer (DAT), the seedlings grown on -Pi medium with both shoots and roots exposed to white light (SL+RL) displayed the inhibition of PR growth compared to those grown on +Pi medium (Figures 1A, B). The root tips of these seedlings exhibited typical Pi deficiency-induced morphological changes, including the reduced meristem size, the increased root hair density and length, and the thickened cell walls (Figures 1C, D). For the seedlings with both shoots and roots grown in darkness (SD+RD), their PR growth was substantially reduced on both +Pi and -Pi media compared to SL+RL seedlings grown under +Pi condition (Figures 1A, B). Although the SD+RD seedlings showed reduced PR growth under -Pi condition, their root tips did not exhibit the Pi deficiency-induced morphological changes (Figures 1C, D).
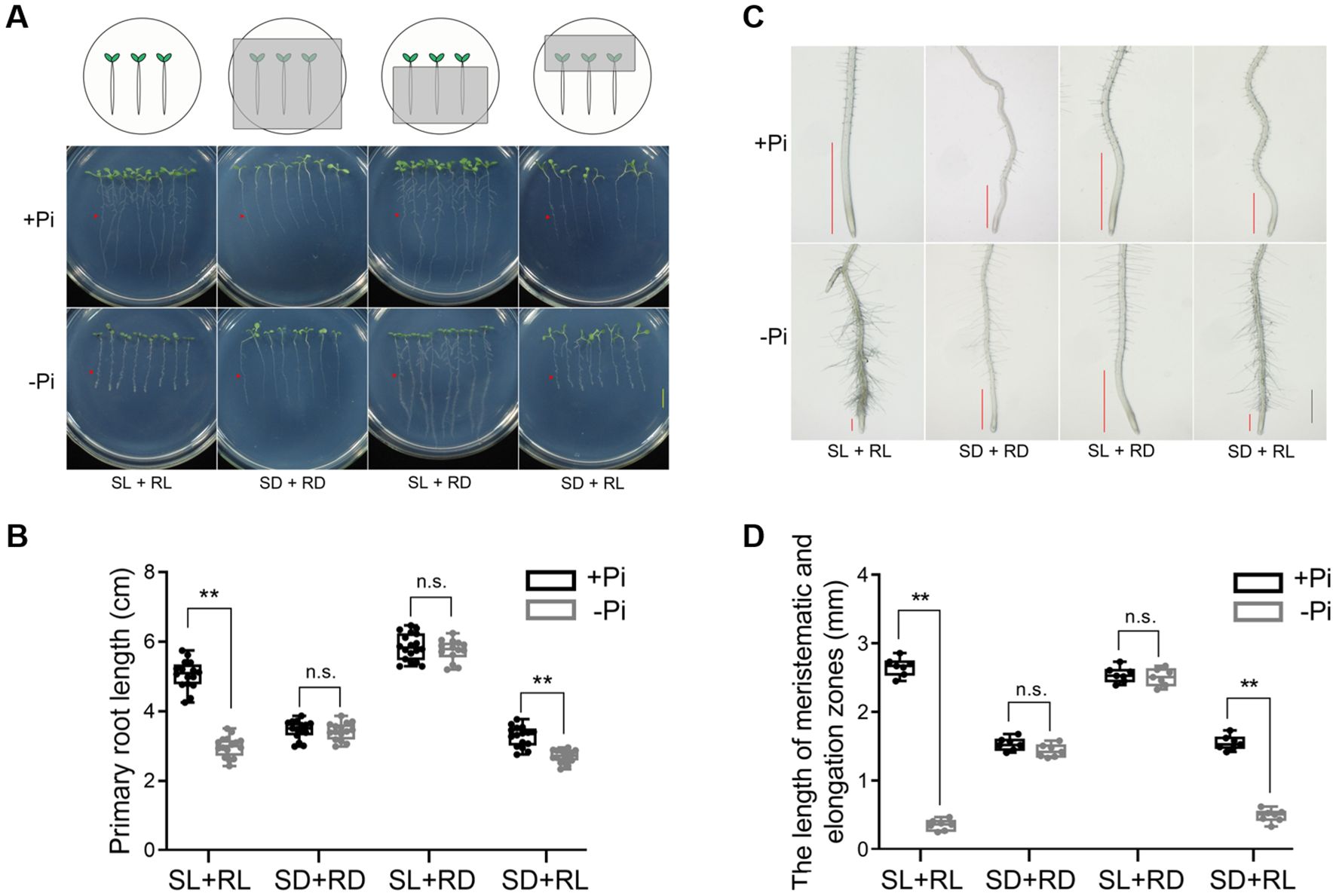
Figure 1. Light illumination on roots but not shoots is both required and sufficient for Pi deficiency-induced inhibition of PR growth. (A) Four-day-old +Pi WT seedlings were transferred to +Pi or –Pi medium and grown for another four days with shoots and roots exposed to different light conditions. SL: shoot under light; RL: root under light; SD: shoot in darkness. RD: root in darkness. The schematic diagrams on the top indicate how shoots and roots of the seedling were shielded from light by aluminum foil. The red triangles indicate the position of the root tips before the seedlings were transferred. The scale bar = 1 cm. (B) Quantification of the primary root length of the seedlings in (A). The experiments were repeated three times, and the representative results are shown (median ± interquartile; Tukey whiskers; n = more than 10 seedlings per condition; **p < 0.01 in Student’s t-tests, n.s. represents no significant difference). (C) Morphologies of the root tips of the seedlings shown in (A). The red lines indicate the size of meristematic and elongation zones. The scale bar = 1 mm. (D) Quantification of the length of meristematic and elongation zones in (C). The experiments were repeated three times, and the representative results are shown (median ± interquartile; Tukey whiskers; n = 7 seedlings per condition; **p < 0.01 in Student’s t-tests, n.s. represents no significant difference).
To further distinguish the functions of shoots and roots in Pi deficiency-induced inhibition of PR growth, we then excluded shoots and roots from light, respectively, by shielding them with aluminum foil. Four-day-old seedlings grown +Pi medium were transferred to +Pi or -Pi medium with either shoots or roots shielded from light. At 4 DAT, the seedlings whose shoots were under light and roots were in darkness (SL+RD) no longer showed the inhibition of PR growth on -Pi medium (Figures 1A, B). The morphological changes in the root tips of these seedlings largely resembled those of SL+RL seedlings grown on +Pi medium (Figures 1C, D). In contrast, seedlings whose shoots were in the dark and roots were under light (SD+RL) exhibited retarded PR growth on +Pi medium compared with +Pi seedlings under SL+RL condition, and displayed further reduction on -Pi medium (Figures 1A, B). Unlike SD+RD seedlings, their root tips exhibited typical Pi deficiency-induced morphological changes, especially the reduced meristem size, on -Pi medium (Figures 1C, D). These results clearly demonstrated that the direct illumination on roots, instead of shoots, was both required and sufficient to mediate Pi deficiency-induced inhibition of PR growth.
The Pi deficiency-induced accumulations of Fe, callose, lignin, and ·OH are attenuated in dark-grown roots
Previously, accumulation of Fe in root apoplasts has been shown to be crucial for Pi deficiency-induced inhibition of PR growth (Svistoonoff et al., 2007; Müller et al., 2015). We wondered whether the Fe accumulation under -Pi condition decreased when roots of seedlings were grown in darkness. We then examined the accumulation of Fe3+ and total Fe (Fe2+ and Fe3+ included) in roots by Perls and Perls/DAB staining methods. The seeds were directly germinated on +Pi medium and grown for four days before they were transferred to +Pi or -Pi medium with shoots grown under light (SL) and root grown under light (RL) or in darkness (RD). We then used the Perls and Perls/DAB staining to assess Fe accumulations in roots at 1, 2, and 3 DAT. Previously, by conducting a time course analysis of the Fe accumulation patterns in roots, Wang et al. (2019) found that the degree of the inhibition of PR growth by Pi deficiency is not linked to the level of Fe in the stem cell niche and elongation zone, but the maturation zone. Therefore, we next mainly focused on the Fe accumulation patterns in maturation zone (The upper row of each treatment in Figure 2). Under RL conditions, both the Fe3+ (Figure 2A) and total Fe (Figure 2B) were obviously accumulated in the maturation zone of the Pi-deficient roots at 2 and 3 DAT, which was consistent with the previous report (Wang et al., 2019). Under RD conditions, however, the accumulations of Fe3+ (Figure 2A) and total Fe (Figure 2B) in maturation zone were apparently abolished in Pi-deficient roots compared with those of the seedlings grown in RL conditions.
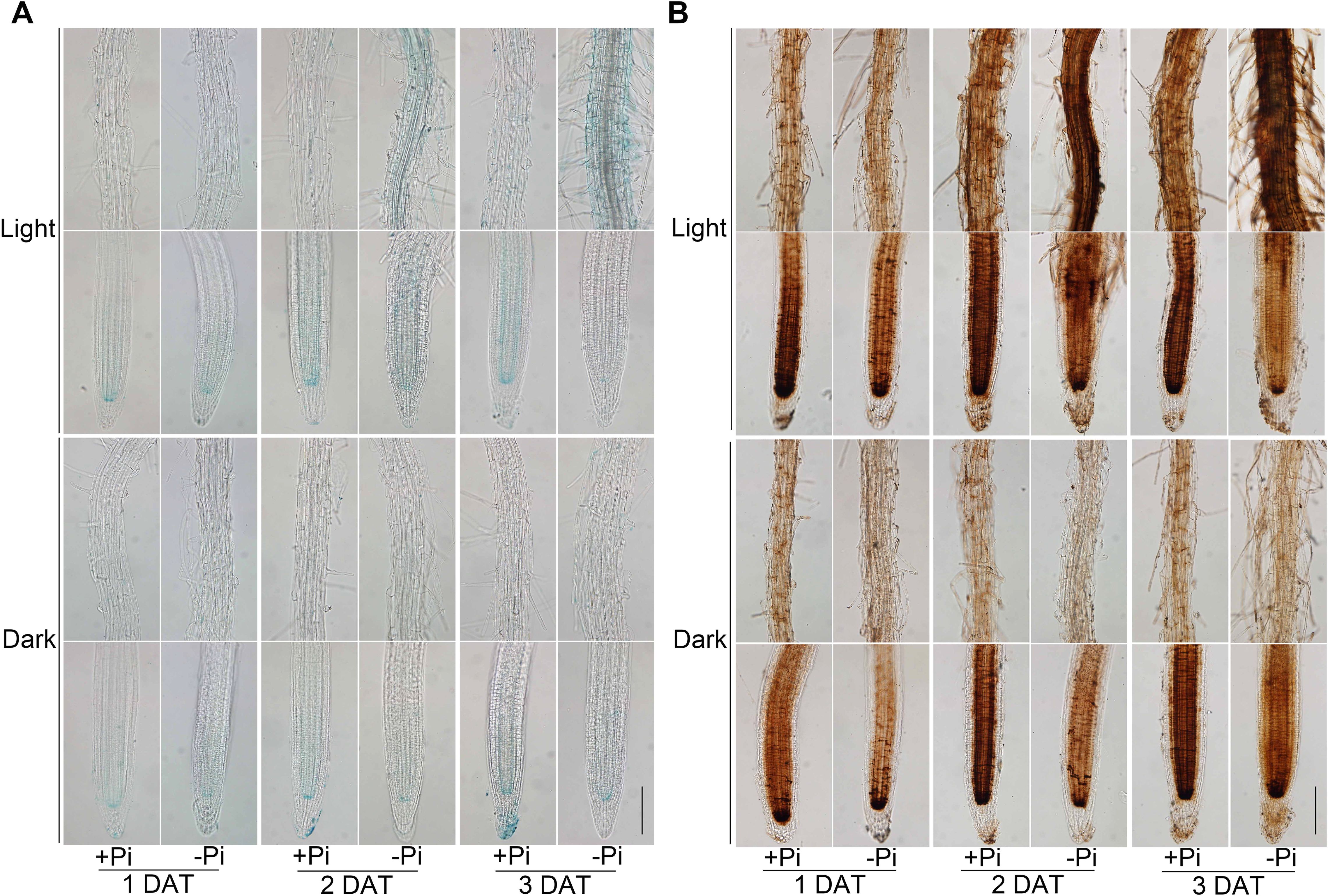
Figure 2. Iron staining patterns of the roots on +Pi and –Pi media under light or in darkness. The seeds were directly germinated on +Pi medium and grown for four days before they were transferred to +Pi and -Pi media under light or in darkness. Perls staining (A) and Perls/DAB staining (B) assays were performed at 1, 2, and 3 days after transfer (DAT). The upper and lower rows in each treatment are photographs of a part of the maturation zone and the root tip, respectively. The scale bar = 100 μm.
It was previously reported that in light-grown seedlings, LPR1-dependent accumulations of callose, lignin, and ·OH in root tips contribute to the inhibition of PR growth under -Pi condition (Williamson et al., 2001; Sanchez-Calderon et al., 2005; Müller et al., 2015; Ziegler et al., 2016; Zheng et al., 2019; Khandal et al., 2020). To further evaluate the impact of light exposure on the inhibition of PR growth under -Pi condition, we examined the accumulations of callose, lignin, and ·OH in the roots on +Pi and –Pi media under light or in darkness. The seeds were directly germinated on +Pi medium and grown for four days before they were transferred to +Pi or -Pi medium under SL+RL or SL+RD conditions. Callose and lignin staining assays were conducted at 4 DAT. Under RL conditions, the callose (Figure 3A) and lignin (Figure 3B) obviously deposited in Pi-deficient roots compared with those in Pi-sufficient roots, whereas the callose and lignin staining signals almost disappeared in the dark-grown roots. For ·OH detection, the seeds were directly germinated under +Pi condition for four days and then transferred to +Pi or -Pi medium with roots exposed to light or in the dark for another two days. The ·OH accumulation in roots were detected by HPF staining. We found that the light illumination-induced ·OH accumulation in roots under -Pi condition was reduced in darkness (Figure 3C). The above results together indicated that the accumulations of Fe, callose, lignin, and ·OH largely rely on light exposure on roots under Pi deficiency, which further confirmed the importance of root illumination in Pi deficiency-induced inhibition of PR growth.
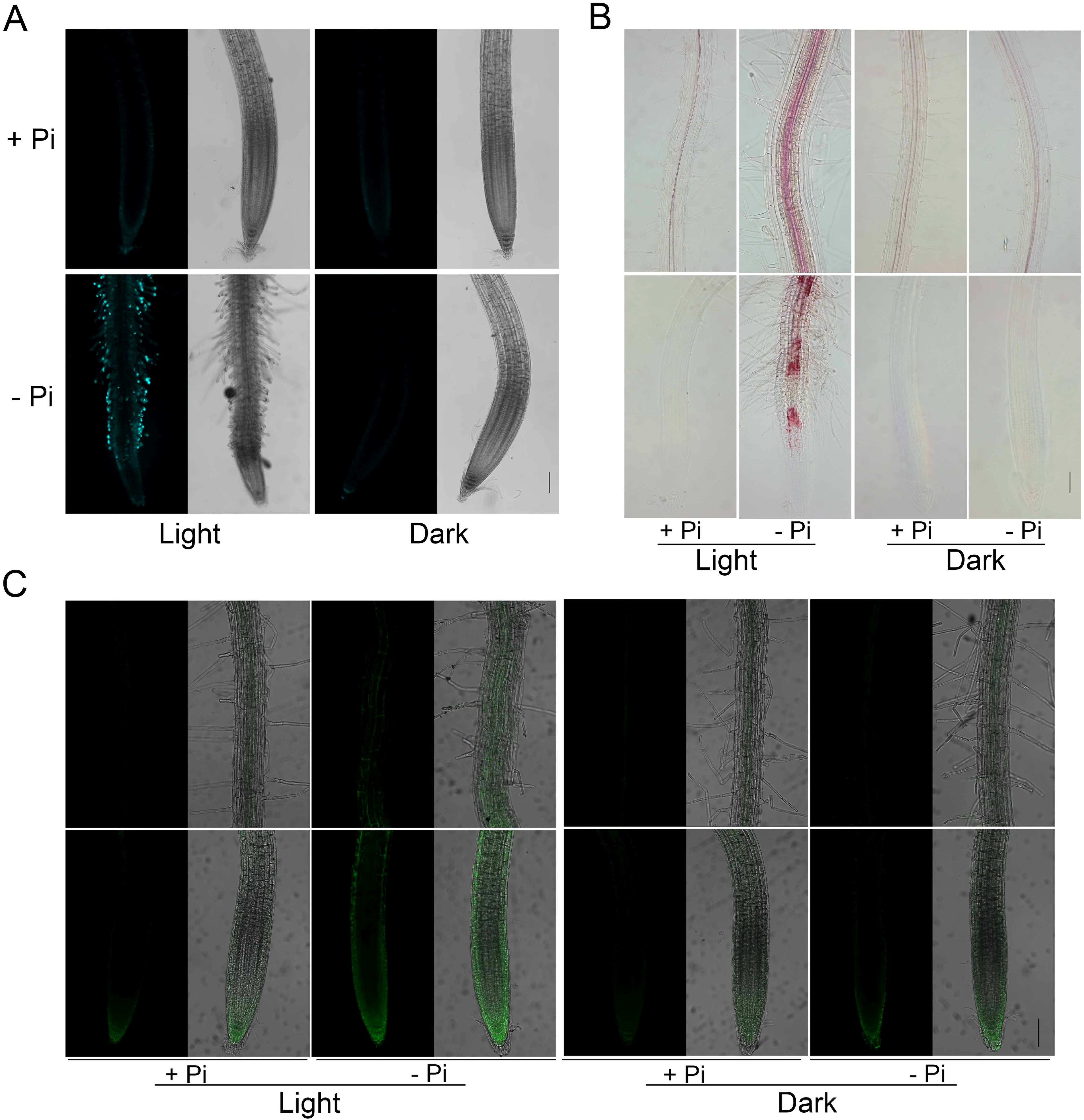
Figure 3. Callose, lignin, and ·OH staining in the roots on +Pi and –Pi media under light or in darkness. The seeds were directly germinated on +Pi medium and grown for four days before they were transferred to +Pi and -Pi medium under light or in darkness. Callose (A) and lignin (B) in roots were stained at 4 DAT. (C)·OH in the roots was assessed by HPF staining at 2 DAT. The upper and lower rows in each treatment of (B, C) are photographs of a part of the maturation zone and the root tip, respectively. The scale bar = 100 μm.
Blue light signaling pathway plays a minor role in regulating the inhibition of PR growth under -Pi condition
Blue light has been implicated in regulating Pi starvation-induced inhibition of PR growth (Zheng et al., 2019; Yeh et al., 2020), and long-distance blue light signaling components, CRY1/CRY2 and HY5, contribute to regulating this process by directly activating the expression of LPR1 (Gao Y. Q. et al., 2021). We next investigated the function of CRY1/CRY2 and HY5 in the Pi deficiency-induced inhibition of PR growth. The seeds of the WT, cry1cry2, hy5, and lpr1lpr2 were directly germinated on +Pi and -Pi media and PR lengths were evaluated at 8 DAG. On +Pi medium, the PR lengths of cry1cry2 and hy5 were significantly decreased compared to that of the WT and lpr1lpr2 (Supplementary Figures S1A, B). The morphologies of the root tips of cry1cry2, hy5, and lpr1lpr2 were comparable to that of the WT (Supplementary Figure S1C). Under -Pi condition, the PR lengths of cry1cry2 and hy5 were slightly longer than that of the WT, while lpr1lpr2 showed a much longer PR length that was similar to itself grown on +Pi medium (Supplementary Figures S1A, B). The relative root lengths (average PR length of –Pi vs. +Pi) of cry1cry2 (51%) and hy5 (42%) were higher than that of the WT (17%), but lower than that of the lpr1lpr2 (95%) (Supplementary Figure S1B), indicating that the cry1cry2 and hy5 were partially insensitive to Pi deficiency in terms of PR length. However, the morphologies of the root tips of cry1cry2 and hy5 were comparable to that of the WT, whereas the lpr1lpr2 showed the similar root tip morphology with itself grown under +Pi condition (Supplementary Figures S1C, D).
To avoid the influence of extremely low Pi environment on the root development of seedlings, we further performed the transferring experiment. The seeds of the WT, cry1cry2, hy5, and lpr1lpr2 were directly germinated on +Pi medium and grown for four days before they were transferred to +Pi or -Pi medium and grown for another four days under SL+RL or SL+RD conditions. Under RL conditions, the PR length of cry1cry2 and hy5 were reduced to around half of the WT under +Pi condition. On –Pi medium, cry1cry2 displayed similar PR lengths with WT, while hy5 showed slightly reduced PR length compared to the WT (Figure 4A; Supplementary Figure S2A). The relative root lengths of cry1cry2 (76%) and hy5 (54%) were higher than that of the WT (46%), but lower than that of the lpr1lpr2 (100%) (Supplementary Figure S2A), indicating that consistent with the direct germination assay (Supplementary Figure S1), the PR length of cry1cry2 and hy5 were partially insensitive to Pi deficiency. The Pi deficiency-induced morphological changes of the root tips of cry1cry2 and hy5 were more comparable to that of the WT but not lpr1lpr2 (Figure 4B; Supplementary Figure S2C). Under RD conditions, the Pi deficiency-induced inhibition of PR growth and the morphological changes of the root tips of the WT, cry1cry2, and hy5 were completely abolished (Figures 4A, B; Supplementary Figures S2B, D).
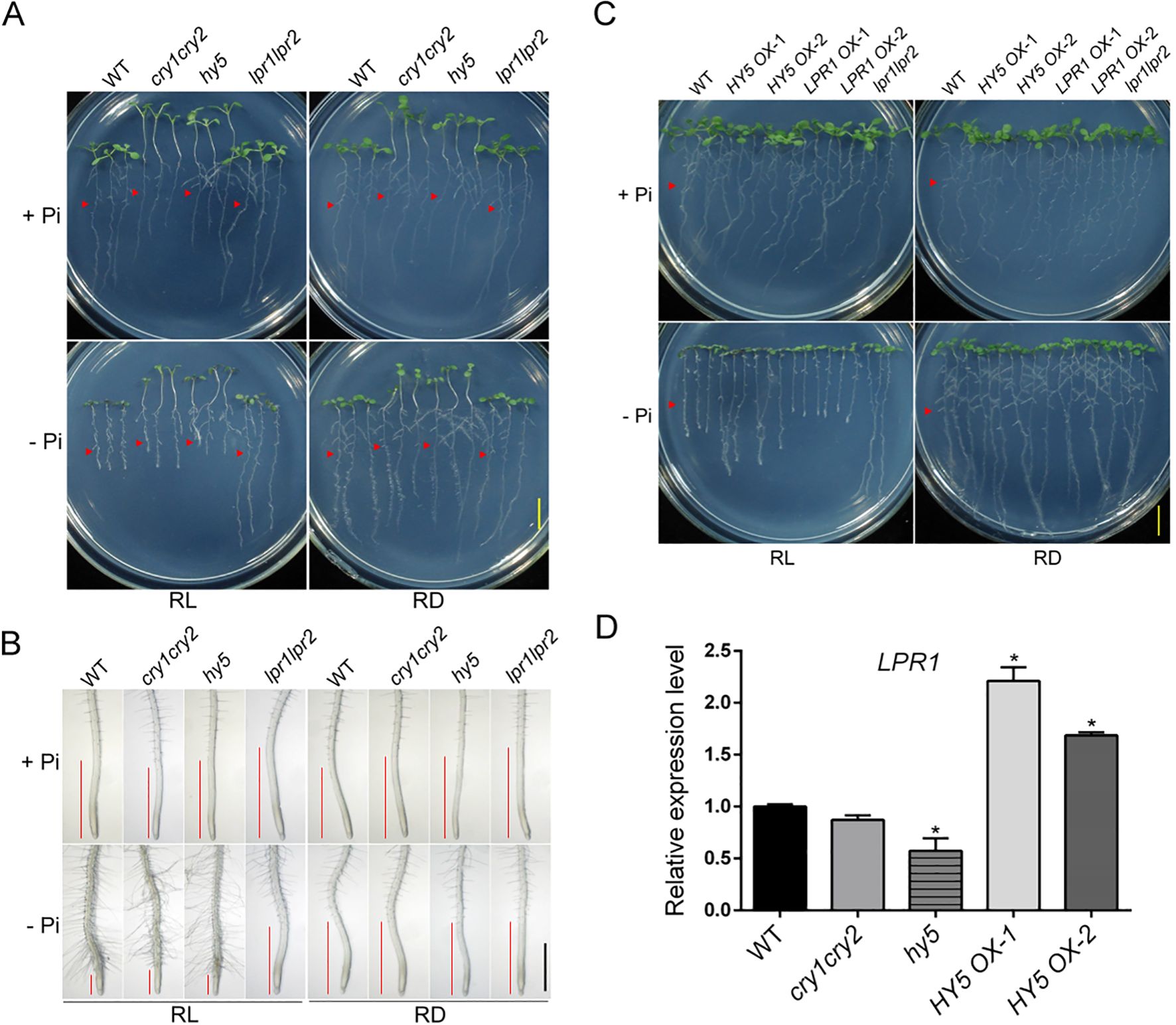
Figure 4. The role of light signaling pathway in Pi deficiency-induced inhibition of PR growth. (A) The 4-day-old +Pi seedlings of the WT, cry1cry2, hy5, and lpr1lpr2 were transferred to +Pi or –Pi medium and grown for another four days with the shoots grown under light and roots grown under light or in darkness. RL: root under light; RD: root in dark. The red triangles indicate the position of the root tips before transfer. The scale bar = 1 cm. (B) The morphologies of the root tips of the seedlings shown in (A). The red lines indicate the meristematic and elongation zones. The scale bar = 1 mm. (C) The 4-day-old +Pi seedlings of the WT, HY5 OX-1, HY5 OX-2, LPR1 OX-1, LPR1 OX-2, and lpr1lpr2 were transferred to +Pi or –Pi medium and grown for another four days with the shoots grown under light and roots grown under light or in darkness. The red triangles indicate the root length before transfer. The scale bar = 1 cm. (D) RT-qPCR analyses of the expression of the LPR1 gene in the seedlings in (C) under RL condition. ACTIN2 was used as an internal control. The experiments were repeated three times, and representative results are shown. The values are means ± SD of three samples each with three technical replicates. Asterisks indicate significant differences from the WT (Student’s t test, *p < 0.01).
We next determined the Pi deficiency-induced accumulations of Fe, callose, lignin, and ·OH in the WT, cry1cry2, hy5, and lpr1pr2. The seeds were germinated on +Pi medium and grown for four days before they were transferred to +Pi and –Pi media with SL+RL or SL+RD. At 4 DAT, under RL condition, a large quantity of Fe2+ (Supplementary Figure S3) and total Fe (Supplementary Figure S4) accumulated in the maturation zone of the roots of the WT, cry1cry2, and hy5, but not lpr1lpr2, on -Pi medium. These accumulations almost disappeared when seedlings were grown in the dark. The same results could also be applied to the accumulations of callose, lignin, and ·OH (Supplementary Figures S5-S7). Overall, the Pi deficiency-induced accumulations of Fe, callose, lignin, and ·OH in cry1cry2 and hy5 were similar with that in the WT, but not that in lpr1lpr2.
Using protoplast assays, Gao Y. Q. et al. (2021) indicated that the overexpression of a single HY5 gene was sufficient to upregulate LPR1 expression. Based on this result, one would predict that the HY5-overexpressing (HY5 OX) line would have enhanced expression of LPR1 and have a hypersensitive PR phenotype similar to that of the LPR1-overexpressing line (LPR1 OX) lines on –Pi medium as previously reported (Müller et al., 2015; Wang et al., 2019; Zheng et al., 2019). We then examined the phenotypes of two HY5 OX lines that had short hypocotyls, indicating that the overexpressed HY5 proteins were functional (Supplementary Figure S8). The 4-day-old Pi-sufficient seedlings of the WT, HY5 OX-1, HY5 OX-2, LPR1 OX-1, LPR1 OX-2, and lpr1lpr2 were transferred to +Pi or –Pi medium and grown for another four days under SL+RL or SL+RD conditions. Under RL condition, we found that the HY5-OX lines had only a slightly enhanced expression of LPR1 (a two-fold increase) (Figure 4D) and did not display the hypersensitive PR phenotype to Pi deficiency as LPR1 OX lines (Figure 4C; Supplementary Figure S9A). Interestingly, the HY5 OX lines were even more insensitive than the WT to Pi deficiency. Like that of the WT, the Pi deficiency-induced inhibition of PR growth of HY5 OX lines and LPR1 OX lines was largely suppressed in the dark (Figure 4C, Supplementary Figure S9B).
Taken together, these results indicated that although the functional disruption of blue light signaling components, CRY1/CRY2 and HY5, result in partial insensitivity of the inhibition of PR growth in response to Pi deficiency, this phenotype cannot be properly explained by their regulation of the expression of LPR1 and by LPR1-dependent accumulations of callose, lignin, and ·OH in root tips under our experimental conditions.
Genome-wide identification of light- and dark-affected phosphate starvation responsive genes
Light affects phosphate starvation responses not only reflected in regulating Pi deficiency-induced inhibition of PR growth under blue light, but also in regulating the transcriptomic changes, for example, red/far-red light regulates the expression of Pi starvation-induced (PSI) genes by activation of PHR1 (Liu et al., 2017), whereas simulated shade conditions compromised Pi starvation responses via repress PHR1 activity by JAZ proteins (Sun et al., 2023). On the other hand, photosynthate sucrose is a global regulator of plant responses to Pi starvation and 73% of the genes that are induced by Pi starvation in WT plants can be induced by elevated levels of sucrose in hps1 mutants (Lei et al., 2011). To further elucidate the impact of light on the transcriptional changes under -Pi, we conducted transcriptomic analyses of the roots grown on +Pi and -Pi media under light or in darkness. The seeds were directly germinated under +Pi condition for four days and transferred to +Pi or -Pi medium with roots exposed to light or in darkness for another four days and then the roots were collected for total RNA extraction (Figure 5A). These batches of RNAs were then subjected to sequencing using next generation sequencing technology. Three biological replicates were used for all genotypes.
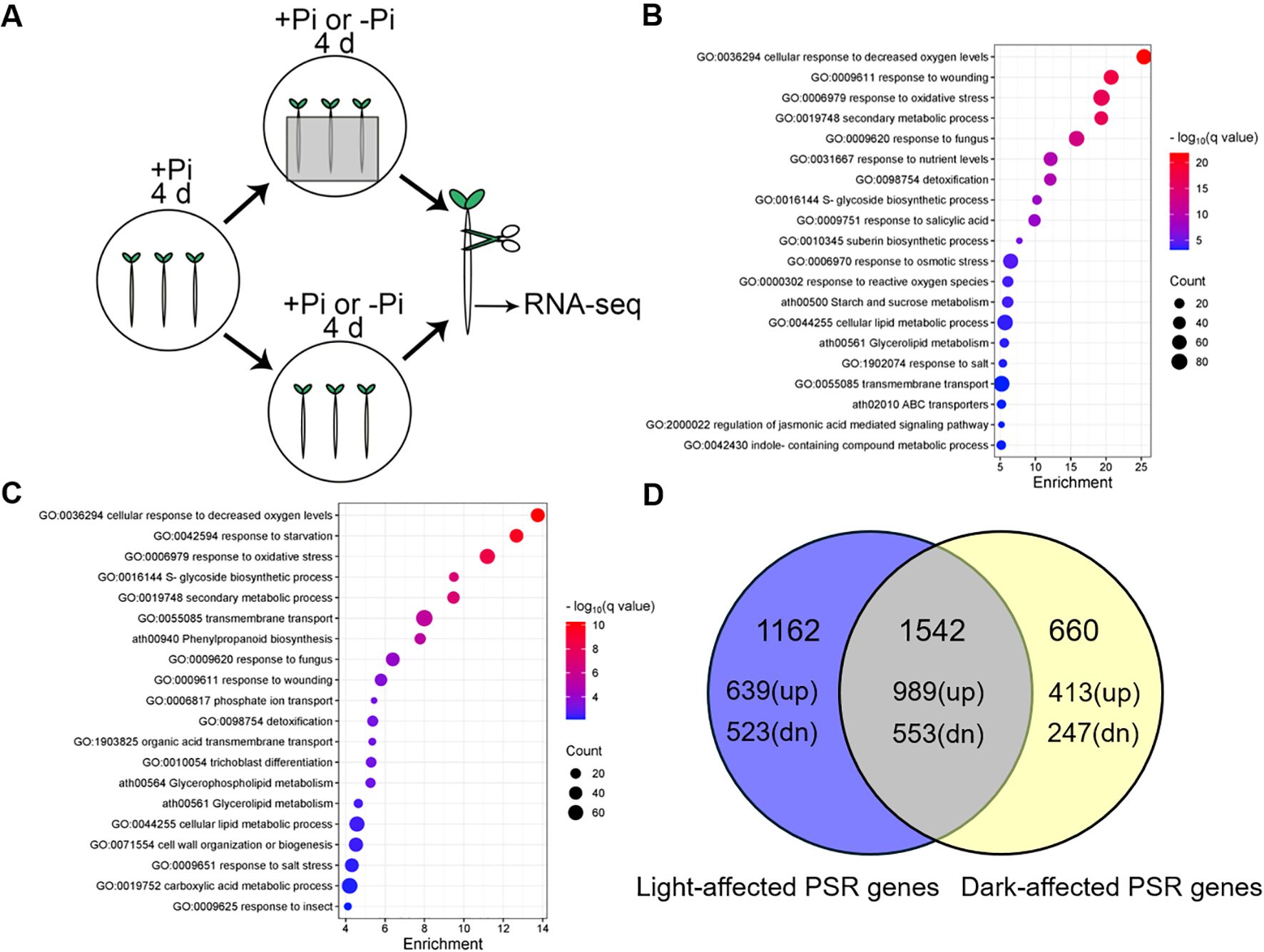
Figure 5. Genome-wide identification of light- and dark-affected phosphate starvation responsive (PSR) genes. (A) The schematic diagram depicts the experimental procedure in this study. The 4-day-old +Pi seedlings were transferred to +Pi or –Pi medium with their shoots grown under light and roots grown under light or in darkness for another four days. Then the roots were collected for RNA extraction and RNA-seq analyses. (B) The GO analysis of the light-affected PSI genes. (C) The GO analysis of the dark-affected PSI genes. (D) The common and specific light- and dark-affected PSR genes. Blue circle represents the light-affected PSR genes, while yellow circle represents the dark-affected PSR genes. “up” in the brackets represents number of the light or dark-affected PSI genes, while “dn” in the brackets represents the number of the light or dark-affected PSS genes.
We first analyzed the effects of light on the expression of genes in roots grown on +Pi medium. Using |Log2FC| ≥ 1 (differential expression level greater than 2 folds) and FDR (false discovery rate) ≤ 0.01 as cutoff, we identified 86 differentially expressed genes (DEG) that were upregulated and 186 DEGs that were downregulated in the roots grown in the dark compared with that grown under light (Supplementary Figure S10A; Supplementary Table S2A). Gene Ontology (GO) analysis showed that most of these DEGs were involved in photosynthesis and response to light stimulus which is consistent with former results (Supplementary Figure S10B) (Silva-Navas et al., 2015; Qu et al., 2017; Zhang et al., 2019).
We then determined the effects of light on the expression of PSR genes. When roots were exposed to light, we identified 2704 PSR genes by comparison of the gene expression levels between roots grown under –Pi-light and +Pi-light, including 1628 PSI genes and 1076 Pi starvation-suppressed (PSS) genes (Supplementary Figure S11A, Supplementary Table S2B). These PSR genes were hereafter referred to as light-affected PSR genes. GO analyses showed that most light-affected PSI genes were enriched in cellular response to decreased oxygen levels, response to wounding, response to oxidative stress, and response to nutrient levels (Figure 5B), while most light-affected PSS genes were enriched in photosynthesis, response to light intensity, cellular response to sucrose starvation, intracellular iron ion homeostasis (Supplementary Figure S11B). When roots were grown in the dark, we identified 2202 PSR genes by comparison of the gene expression levels between roots grown under –Pi-dark and +Pi-dark, which included 1399 PSI genes and 803 PSS genes (Supplementary Figure S12A, Supplementary Table S2C). These PSR genes were defined as dark-affected PSR genes. GO analyses indicated that most dark-affected PSI genes were enriched in cellular response to decreased oxygen levels, response to starvation, phosphate ion transport, cellular lipid metabolic process, trichoblast differentiation (Figure 5C), while most dark-affected PSS genes were enriched in photosynthesis, response to absence of light, nitrogen metabolism, intracellular iron ion homeostasis (Supplementary Figure S12B).
The common and specific light- and dark-affected PSR genes
To identify the common and specific light- and dark-affected PSR genes, a Venn diagram was drawn by comparison of the expression levels between light- and dark-affected PSR genes. We obtained 1542 common PSR genes between light- and dark-affected PSR genes, 1162 PSR genes that were specifically affected by light conditions and 660 PSR genes that were specifically affected by dark conditions (Figure 5D).
For the 1542 common PSR genes that were both affected by light and dark conditions, there were 989 PSI genes and 553 PSS genes (Figure 5D). The GO analysis indicated that most common PSI genes were enriched in cellular response to decreased oxygen levels, response to nutrient levels, S-glycoside biosynthetic process, phosphate ion transport (Supplementary Figure S13A; Supplementary Tables S2B, C), and most common PSS genes were enriched in photosynthesis, response to light intensity, photosystem II assembly (Supplementary Figure S13B; Supplementary Tables S2B, C). Next, we analyzed the PSR genes that were specifically affected by light and dark conditions. We identified 639 specific light-affected PSI genes and 523 specific light-affected PSS genes (Figure 5D). The GO analysis of 639 specific light-affected PSI genes showed that most of these genes were enriched in response to wounding, response to fungus, defense response to other organism, response to salicylic acid, indole-containing compound metabolic process (Supplementary Figure S13C; Supplementary Table S2B). Most specific light-affected PSS genes were enriched in photosynthesis and water transport (Supplementary Figure S13D; Supplementary Table S2B). Finally, we identified 413 specific dark-affected PSI genes and 247 specific dark-affected PSS genes (Figure 5D). GO analysis suggested that most specific dark-affected PSI genes were enriched in root hair elongation, wax biosynthetic process, flavonoid biosynthesis (Supplementary Figure S13E; Supplementary Table S2C), and most specific dark-affected PSS genes were enriched in nitrogen metabolism, cellular response to hypoxia and so on (Supplementary Figure S13F; Supplementary Table S2C).
Expression profile of the light- and dark-affected PSR genes
To give a global view of how light and dark conditions affect the transcriptomic changes under +Pi and –Pi media, we drew a heatmap using the expression levels of all PSR genes (obtained by comparisons of roots grown under -Pi-light vs. +Pi-light, -Pi-dark vs. +Pi-dark, -Pi-dark vs. +Pi-light) among four types of treatments (Supplementary Tables S2B-D).
Hierarchical clustering was conducted and presented in the heatmap (Figure 6; Supplementary Table S3). By hierarchical clustering analysis, we obtained three clusters, among which cluster 1 and 2 represented PSI genes while cluster 3 represented PSS genes. We found that under -Pi condition, light had more impact on the expression of PSI genes than PSS genes. For 1102 PSI genes in cluster 1, the expression of most genes was upregulated by Pi starvation in the dark and was further increased when roots were exposed to light illumination. GO analysis suggests that these genes were highly enriched in response to wounding and fungus, cellular response to decreased oxygen levels, glucosinolate biosynthesis from methionine, response to salt, and hormone-related processes, such as salicylic acid, auxin, abscisic acid, and jasmonic acid (Figure 6; Supplementary Figure S14A). For 1004 PSI genes in cluster 2, the expression of the genes was upregulated in the dark on -Pi medium, whereas the upregulation was partially decreased when roots were exposed to light. GO analysis suggested that these genes were highly enriched in cellular response to phosphate starvation, glycerophospholipid metabolism, trichoblast differentiation, inositol phosphate metabolism, sulfolipid metabolic process, cell wall organization or biogenesis (Figure 6; Supplementary Figure S14B). For 1346 PSS genes in cluster 3, light has more effect on the expression of these genes on +Pi medium than –Pi medium. GO analysis suggested that these genes were enriched in photosynthesis, iron ion transport, cellular response to sucrose starvation and so on (Figure 6; Supplementary Figure S14C).
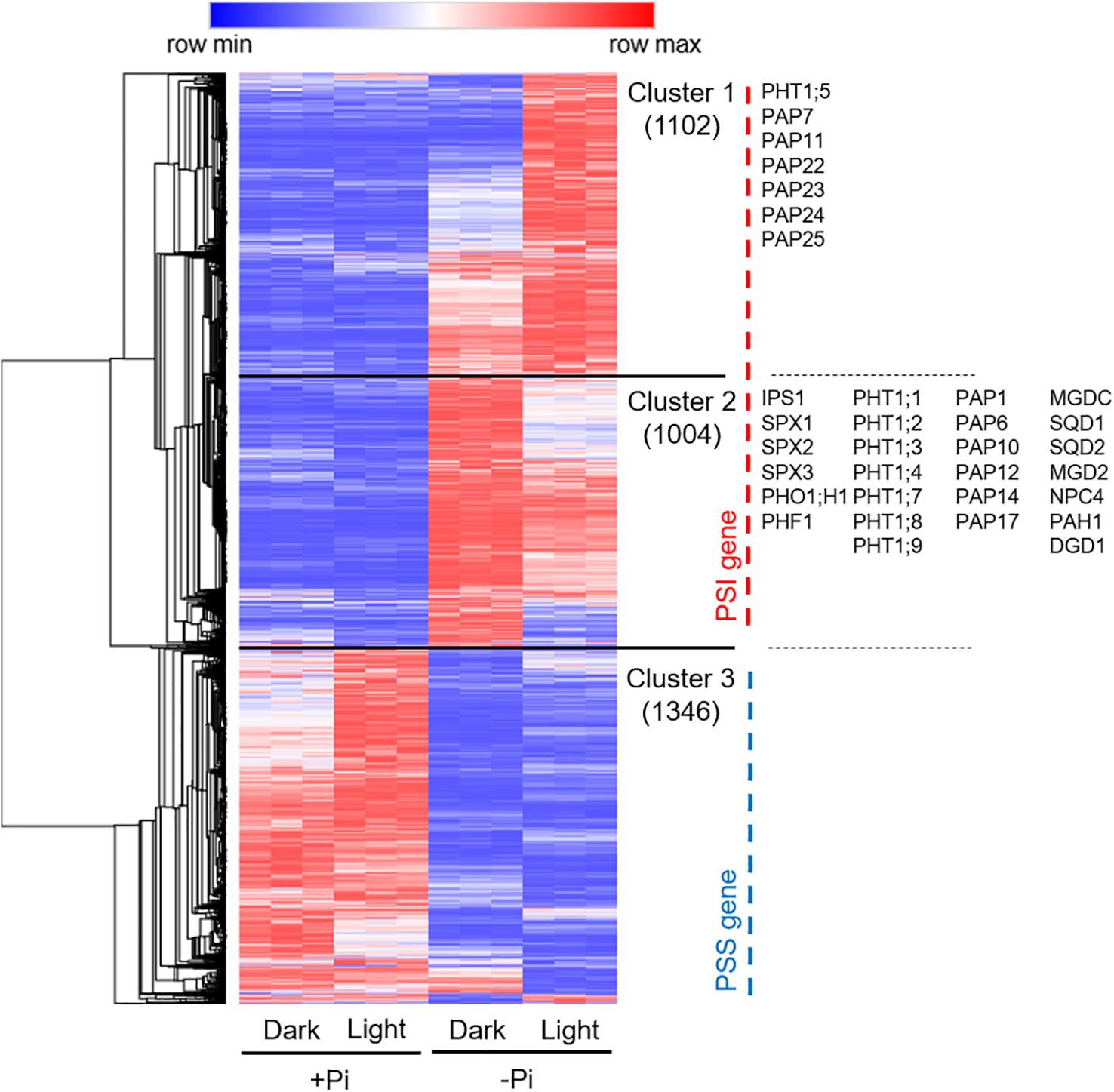
Figure 6. Expression profile of the light- and dark-affected PSR genes. The expression levels of all PSR genes were used to draw the heatmap. Three clusters were obtained by hierarchical clustering, among which genes in cluster 1 and 2 belong to PSI genes while genes in cluster 3 belong to PSS genes. The TPM (transcripts per million) values used in the heatmaps have been standardized by min-max standardization. The gene number of each cluster were shown in brackets. The positions of the known or putative PSI genes were indicated at the right side of the heatmap.
To elucidate the impact of light exposure on the expression of typical PSI genes (Bustos et al., 2010; Wang et al., 2024), we selected a group of PSI genes that are known or putative to be involved in various Pi starvation responses, such as Pi signaling (IPS1, SPX1, SPX2, SPX3, PHF1, PHO1;H1), Pi transport (PHT1;1, PHT1;2, PHT1;3, PHT1;4, PHT1;5, PHT1;7, PHT1;8, PHT1;9), Pi remobilization (PAP1, PAP6, PAP7, PAP10, PAP11, PAP12, PAP14, PAP17, PAP22, PAP23, PAP24, PAP25), and phospholipid remodeling (MGDC, SQD1, SQD2, MGD2, NPC4, PAH1, DGD1). We found that most of these genes belong to cluster 2, while a few of them belong to cluster 1 (Figure 6; Supplementary Table S3).
Taken together, these results indicated that light has a substantially promotion on Pi deficiency-induced cellular response to stress (biotic and abiotic) and phytohormone-related processes (SA, auxin, ABA, JA), while has a suppression of genes involved in cellular response to Pi starvation, such as Pi signaling, Pi transport, Pi remobilization, and phospholipid remodeling.
Light has both positive and negative effects on the expression of known or putative PSI genes under -Pi condition
To further reveal the light effects on the expression of above-mentioned typical PSI genes, we drew a heatmap of these genes by their expression levels (Figure 7A). In this heatmap, we found that the effects of light on the expression of the typical PSI genes can be summarized into three categories: (1) light exposure promotes the expression of the PSI genes, such as PHT1;5, PAP7/11/23; (2) light exposure inhibits the expression of the PSI genes, such as IPS1, PHT1;1, SPX3; (3) light exposure has no obvious effect on the expression of the PSI genes, such as SPX1, SPX2, and PHF1 (Figure 7A). To confirm this, we conducted RT-qPCR analysis of seven hallmark PSI genes, including two non-coding transcripts, IPS1 and AT4 (Burleigh and Harrison, 1999); a microRNA, miR399d (Fujii et al., 2005); two high-affinity phosphate transporters, AtPT1 (Pht1;1) and AtPT2 (Phtl;4) (Muchhal et al., 1996); a ribonuclease, RNS1 (Bariola et al., 1994); and an acid phosphatase, ACP5 (AtPAP17) (del Pozo et al., 1999). We found that light has positive effects on the expression of ACP5, AtPT2, and RNS1, whereas has negative effects on the expression of miR399d and IPS1 (Figure 7B). These results demonstrated that light has both positive and negative effects on the expression of known or putative PSI genes under -Pi condition.
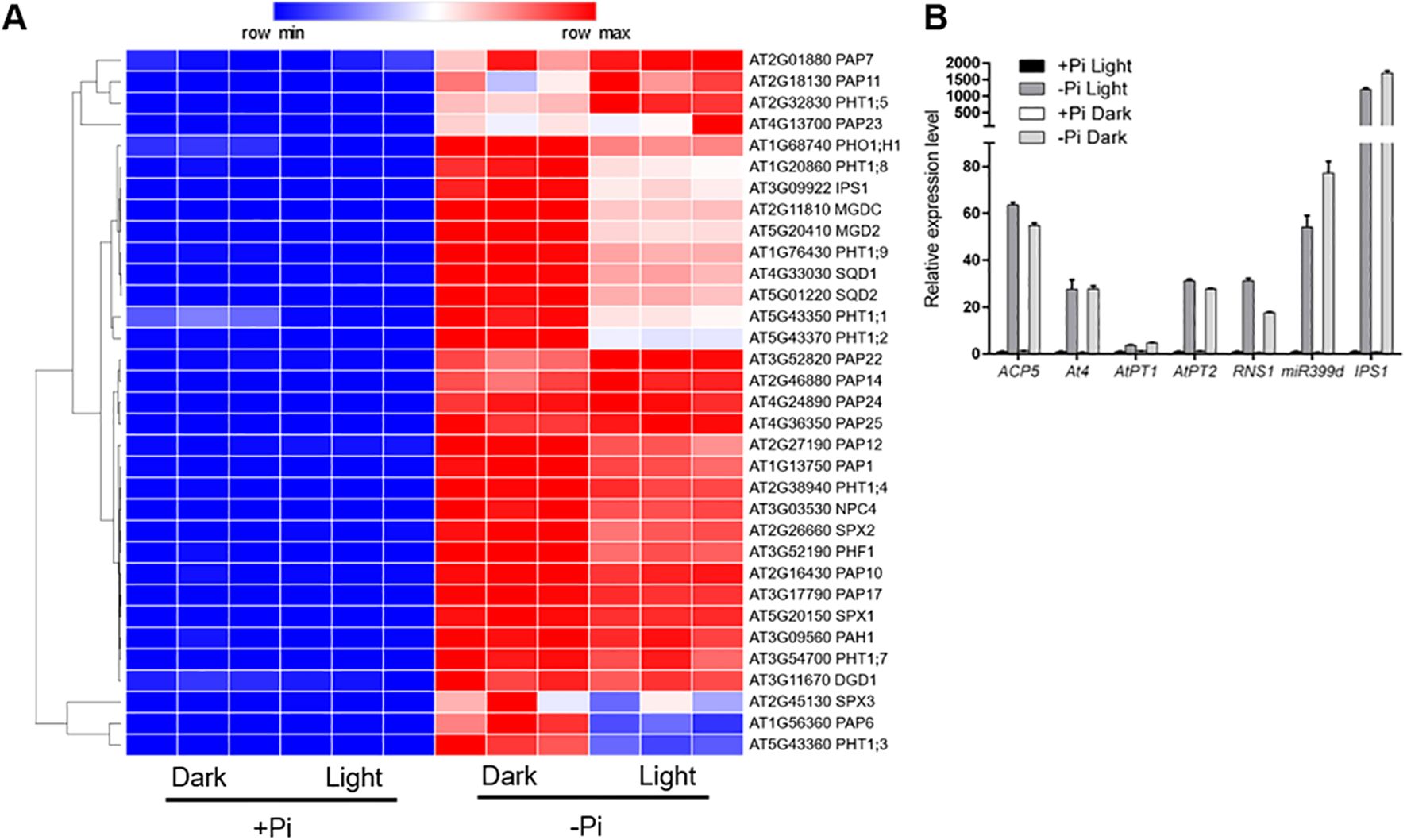
Figure 7. Light has both positive and negative effects on the expression of known or putative PSI genes under -Pi condition. (A) The heatmap of 33 known or putative PSI genes under light or in darkness. A relative color scheme uses the minimum and maximum values in each row to convert values to colors. (B) RT-qPCR analyses of the expression level of seven hallmark PSI genes under different conditions in roots. ACTIN2 was used as an internal control. The experiments were repeated three times, and representative results are shown. The values are means ± SD of three samples each with three technical replicates.
Light enhances local and systemic phosphate starvation responses
Given light has a profound influence on the transcriptomic changes under –Pi condition, we wondered whether it affects other Pi starvation responses. Induction and secretion of APases on the root surface is one of the hallmark responses of plants to Pi starvation. Among multiple purple acid phosphatases in Arabidopsis, PAP10, is predominantly associated with the root surface after its secretion (Wang et al., 2011). Root surface-associated APase activity can be detected by histochemical staining using a substrate of APase, BCIP (5-bromo-4-chloro-3-indolyl phosphate). The product of the enzyme reaction forms a blue precipitate. We next evaluated the APase activity in the root surface by BCIP staining. We found that acid phosphatase activity on the root surface was highly induced on -Pi medium in the dark and this induction was apparently further increased when roots were exposed to light condition (Figure 8A).
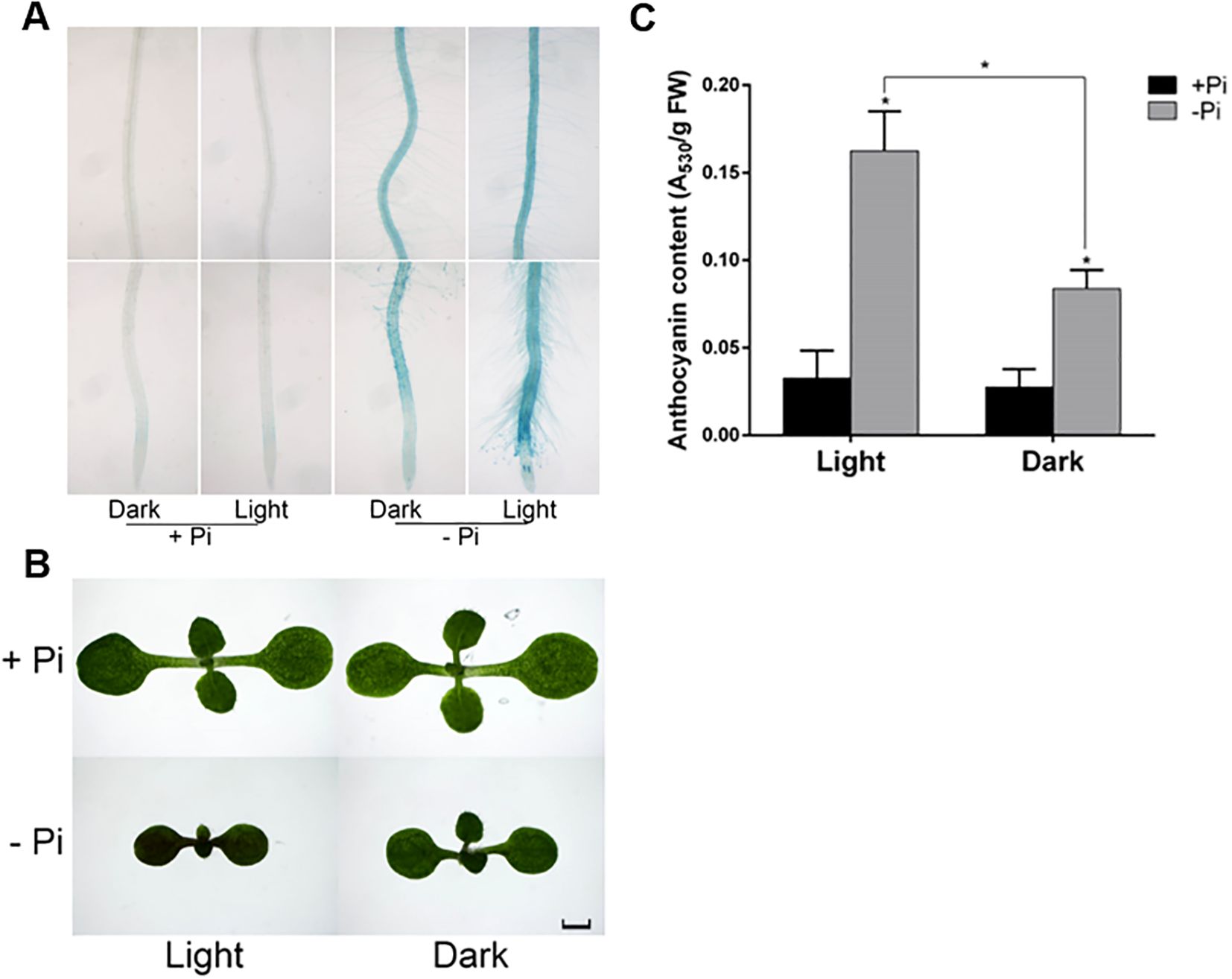
Figure 8. The root-associated APase activity and anthocyanin accumulation of the WT on +Pi and –Pi media under light or in darkness. The WT seedlings grown on +Pi for four days and then transferred into +Pi or -Pi medium for another four days with shoots grown under light and roots grown under light or in the dark. (A) Root-associated APase activity were detected by BCIP staining. Anthocyanin accumulation were detected by microscopy (B) and A530 absorption (C). In (C), The experiments were repeated three times, and representative results are shown. The values are means ± SD of three samples each with three technical replicates. Asterisks indicate significant differences from the WT (Student’s t-test, *p <0.05). The scale bar = 1 mm.
As light enhanced the root surface-associated APase activity in roots, we next wondered whether light illumination on roots could stimulate systemic responses in shoots. Anthocyanin accumulation is acknowledged as a phenotypic indicator of Pi starvation. We then determined the anthocyanin accumulation in shoots when roots were grown on +Pi and -Pi media under light or in darkness. The seeds were grown on +Pi medium for four days and transferred into +Pi and –Pi media for another four days under SL+RL or SL+RD conditions before anthocyanin accumulation was detected. We found that a large quantity of anthocyanins accumulated in shoots on -Pi medium under light and the accumulation was largely decreased when roots were grown in darkness (Figures 8B, C).
Taken together, these results suggested that light exposure on roots results in increased Pi starvation responses, such as root surface-associated APase activity; it also triggers systemic stress responses in shoots of seedlings, such as anthocyanin accumulation, by an as yet undetermined mechanism.
Discussion
The inhibition of PR growth is a major response of Arabidopsis to Pi deficiency. In the past decades, by identifying a group of mutants that are insensitive to Pi deficiency-induced inhibition of PR growth, there is a common view that Fe accumulation and malate in roots is critical to this process (Svistoonoff et al., 2007; Müller et al., 2015; Balzergue et al., 2017; Mora-Macías et al., 2017; Wang et al., 2019). Our laboratory further showed that direct blue light illumination on roots is both required and sufficient for the Pi deficiency-induced inhibition of PR growth (Zheng et al., 2019). Another study revealed that the inhibition of root elongation by -Pi requires blue light signal perception at the shoot and transduction to the root (Gao Y. Q. et al., 2021). The discrepancy between this work and our study raised questions that which part (shoots or roots) contributes to Pi deficiency-induced inhibition of PR growth, and that whether blue light signaling pathway is involved in this process.
After analyses of the experimental data provided in Gao Y. Q. et al. (2021), we considered that the experimental results did not disprove the notion that light illumination on roots is required to trigger the Pi deficiency-induced inhibition of PR growth. Meanwhile, light shielding of shoots did not abolish this responsiveness. In this work, to address whether shoots or roots are critical for Pi deficiency-induced inhibition of PR growth, we conducted detailed light shielding assays to separate the role of shoots and roots in the inhibition of PR growth under -Pi. We found that compared to the inhibition of PR growth in Pi-deficient SL+RL seedlings, the Pi deficiency-induced inhibition of PR growth were abolished in SL+RD seedlings, suggesting that the light illumination on roots is required for this process; on the other hand, compared to the SD+RD seedlings, the Pi deficiency-induced PR growth inhibition and typical morphological changes of root tips, especially the reduced meristem size, was observed for SD+RL seedlings under -Pi condition, indicating that the light illumination on roots is sufficient for this process (Figure 1). Notably, when shoots were placed in darkness (SD+RD and SD+RL), the PR lengths of these seedlings were decreased to about one half under +Pi condition in contrast to that of SL+RL seedlings under +Pi (Figure 1B). These could be attributed to two possible reasons: (1) Fewer photosynthates are delivered to roots to support their growth due to the lack of photosynthesis. By addition of sucrose into medium, this hypothesis can be easily tested in future; (2) Light illumination on shoots promotes PR elongation by long-distance shoot-root communication, which was repressed by shielding shoots from light. Previous studies have shown that COP1 and HY5 are involved in light-mediated PR growth by regulation of auxin transporters (Sassi et al., 2012) and the balance of C/N (Chen et al., 2016). Last but not least, the contrasting results obtained by Gao Y. Q. et al. (2021) and our laboratory (Zheng et al., 2019) might be resulted from different experimental designs (direct germination assay or transferring experiment), medium formulas (Hoagland medium or 1/2 MS medium), Pi contents (+Pi: 250μM, -Pi: 50μM or +Pi: 625μM, -Pi: 0μM), and environmental conditions (light intensity of 100 or 200 μmol m-2 s-1).
The initial evidence for the working model proposed by Gao Y. Q. et al. (2021) was that the blue light signaling mutants cry1cry2 and hy5 had a reduced expression of LPR1 (the mutants still retained 20-30% of expression) and were completely insensitive to Pi deficiency-induced inhibition of PR growth. In our work, we found that the PR growth of cry1cry2 and hy5 was still partially inhibited by Pi deficiency and that the degree of inhibition depended on the growth conditions (seeds were germinated on +Pi medium and grown for four days before they were transferred to –Pi medium for another four days, or seeds were germinated on –Pi medium and grown for eight days). Consistently, the partial inhibition of PR growth of cry1cry2 and hy5 on -Pi medium has also been reported by Yeh et al. (2020). We also noticed that the morphological changes of the root tips of cry1cry2 and hy5 did not differ from those of the WT under Pi deficiency (Figure 4B, Supplementary Figure S2C). Furthermore, like WT root tips, the root tips of cry1cry2 and hy5 had enhanced Fe accumulation, callose deposition, lignin formation, and ROS accumulation under Pi deficiency (Supplementary Figures S3-7). These responses were largely suppressed when roots were grown in the dark (Figure 4B, Supplementary Figures S2D, S3-S7).
Although Gao Y. Q. et al. (2021) showed that hy5 had reduced expression of LPR1 in roots, there was no direct evidence supporting the inference that the insensitivity of hy5 to Pi deficiency was caused by the reduced expression of LPR1. In our work, we found that the HY5 OX lines had about two-fold increased expression of LPR1 (Figure 4D) and did not display the hypersensitive PR phenotype to Pi deficiency as LPR1 OX lines (Figure 4C; Supplementary Figure S9A). Interestingly, the HY5 OX lines were even more insensitive than the WT to Pi deficiency. The above results indicated that despite the PR growth of cry1cry2 and hy5 displayed partial insensitivity to Pi deficiency, these phenotypes cannot be explained by their regulation of the expression of LPR1 and by LPR1-dependent Fe redox cycle in root tips under our experimental conditions. Instead, one could only conclude that the accumulation of HY5 in roots plays a role in maintaining the basal expression of LPR1 under both Pi sufficiency and Pi deficiency (Gao Y. Q. et al., 2021).
Over the last 20 years, many Arabidopsis mutants with altered sensitivity of PR growth to Pi deficiency have been identified. Except for lpr1, stop1, almt1, als3, star1, crr, and hyp1 (Svistoonoff et al., 2007; Balzergue et al., 2017; Dong et al., 2017; Mora-Macías et al., 2017; Clúa et al., 2024; Maniero et al., 2024), most of them, however, exhibited developmental abnormality under +Pi (Ma et al., 2003; Miura et al., 2005; Nacry et al., 2005; Jiang et al., 2007; Mayzlish-Gati et al., 2012; Singh et al., 2014). HY5 acts as a master regulator of light-mediated transcriptional regulatory hub that directly or indirectly controls the transcription of approximately one-third of genes at the whole genome level (Xiao et al., 2022). It was reported that HY5 may regulate the PR growth by directly controlling the expression of auxin and BR pathway related genes (Li et al., 2020), and by regulating the light-responsive transcription of miRNA163 (Li et al., 2021). Besides, hy5 has an insensitive PR growth phenotype in thermomorphogenesis process (Lee et al., 2021). Considering the short PR lengths of cry1cry2 and hy5 under normal conditions, we assumed that CRY1/CRY2 and HY5 possess pleotropic effects in regulating PR growth and development, and that the partial insensitivity of PR growth of cry1cry2 and hy5 in response to Pi deficiency was independent of their regulation of the expression of LPR1.
In fact, the photo-Fenton reaction not only occurs in Pi deficiency-induced inhibition of PR growth as a source of damage in transparent Petri dishes, it also takes place in the shoots of seedlings under normal condition. For example, the LPR1-mediated Fe2+ oxidation and blue light-triggered photo-Fenton reaction were observed in the translocation of Fe in the xylem for Fe distribution in plants. A recent study revealed that LPR1 and LPR2 are required to oxidize Fe2+ and maintain Fe3+-citrate stability and mobility during xylem translocation against photoreduction (Xu et al., 2022). Therefore, we speculated that when we place Pi-deficient roots in light exposure, it triggers an existing mechanism of Fe distribution of shoots. Unlike the xylem with cell wall lignification and protoplast lysis in shoots, the root apical meristem is more fragile in response to damages, thereby resulting in Pi deficiency-induced inhibition of PR growth under light.
Light is one of the most important environmental signals and regulates many biological processes in plants. Previous studies reported that both light signaling pathway and photosynthate sucrose have a significant impact on transcriptional changes under –Pi (Lei et al., 2011; Liu et al., 2017). To further illustrate the light effects on transcriptional changes under –Pi, we analyzed the transcriptomic changes in roots on +Pi and -Pi media under light or in darkness (Figure 5A). We identified 1628 light-affected PSI genes and 1076 light-affected PSS genes, 1399 dark-affected PSI genes and 803 dark-affected PSS genes (Supplementary Tables S2B, C). By comparison of common and specific light- and dark-affected PSR genes, we found that among 1542 common PSR genes, most common PSI genes were enriched in cellular response to decreased oxygen levels, response to nutrient levels, S-glycoside biosynthetic process, phosphate ion transport (Supplementary Figure S13A), most common PSS genes were enriched in photosynthesis, response to light intensity, photosystem II assembly (Supplementary Figure S13B), which were consistent with previous transcriptomic studies under Pi starvation (Wu et al., 2003; Misson et al., 2005; Morcuende et al., 2007; Bustos et al., 2010; Osorio et al., 2019; Hani et al., 2021; Wang et al., 2023). For specific light-affected PSI genes, most of them were enriched in response to wounding, response to fungus, defense response to other organism, response to salicylic acid, indole-containing compound metabolic process (Supplementary Figure S13C); most specific light-affected PSS genes were enriched in photosynthesis and water transport (Supplementary Figure S13D). For specific dark-affected PSI genes, most of them were enriched in root hair elongation, wax biosynthetic process, flavonoid biosynthesis (Supplementary Figure S13E); most specific dark-affected PSS genes were enriched in nitrogen metabolism, cellular response to hypoxia (Supplementary Figure S13F). These results indicated that the transcriptomic changes of an array of PSR genes (specific light- and dark-affected PSR genes, including genes involved in stress and phytohormone-related processes, root hair elongation, nitrogen metabolism) are due to the light illumination on roots in transparent Petri dishes instead of Pi deficiency per se.
By drawing a heatmap and conducting hierarchical clustering analysis, we found that light exerted more effects on the expression of PSI genes than PSS genes. For PSI genes, light has both function of induction (cluster 1) and suppression (cluster 2). Those light-promoted genes in cluster 1 were highly enriched in response to wounding and fungus, cellular response to decreased oxygen levels, glucosinolate biosynthesis from methionine, response to salt, and hormone-related processes, such as salicylic acid, auxin, abscisic acid, and jasmonic acid (Figure 6; Supplementary Figure S14A). This result was similar with the GO enrichment of specific light-affected PSI genes. Consistently, Arabidopsis roots grown under light have been demonstrated to have altered responses to stresses (osmotic and salt) and phytohormones compared to those grown in the dark (Yokawa et al., 2013, 2014; Silva-Navas et al., 2015; Cabrera et al., 2021). The light-repressed genes in cluster 2 were highly enriched in cellular response to phosphate starvation, glycerophospholipid metabolism, trichoblast differentiation, inositol phosphate metabolism, sulfolipid metabolic process, cell wall organization or biogenesis (Figure 6; Supplementary Figure S14B). We also detected many known or putative PSI genes in cluster 2. By drawing a detailed heatmap for these typical PSI genes, we found that light has both induction and repression effects on the expression of these genes (Figure 7A). Earlier researches have shown that active light signaling is required for the induction of PHR1 expression and simulated shade conditions compromised Pi starvation responses via repress PHR1 activity by JAZ proteins (Liu et al., 2017; Sun et al., 2023). By transcriptomic analyses, we confirmed the upregulation of a series of PSI genes under light and also found the downregulation of PSI genes by light exposure, suggesting that there might have other unknown ways of repressing the expression of PSI genes on –Pi medium under light. Further study is still needed to clarify whether PHR1, or other components, are involved in this process.
By assessing the root surface-associated APase activity on roots and anthocyanin accumulation in shoots on +Pi and –Pi media under light or in darkness (Figure 8), we found that light affects Pi starvation responses both in local and systemic manners. The anthocyanin biosynthetic pathway is derived from the flavonoid pathway. Previous studies have shown that the flavonoid pathway is highly induced in illuminated shoots and roots (Silva-Navas et al., 2016; Qu et al., 2017; Miotto et al., 2019) and it has been reported that flavonoids are systemically mobile (Buer et al., 2007). Therefore, it is plausible that root-synthesized anthocyanin precursors are transported to the shoot, increasing anthocyanin content, which will be interesting to determine the underlying mechanism.
Conclusion
This work clarified that light illumination on roots, but not shoots, is both required and sufficient for Pi deficiency-induced inhibition of PR growth. Besides, the Pi deficiency-induced accumulations of Fe, callose, lignin, and ·OH are attenuated in roots grown in darkness. Blue light signaling pathway plays a minor role in regulating the inhibition of PR growth under -Pi condition. Light promotes the expression of a large number of genes involved in stress and phytohormone-related processes and has both upregulated and downregulated effects on the expression of the typical PSI genes. Light affects Pi starvation responses both in local and systemic manners. All these again indicate that the inhibition of PR growth under –Pi condition may be artificial phenotype, encouraging us carefully evaluate the phenotype under illuminated, transparent Petri dishes.
Data availability statement
The datasets presented in this study can be found in online repositories. The names of the repository/repositories and accession number(s) can be found in the article/Supplementary Material.
Author contributions
ZW: Conceptualization, Data curation, Formal Analysis, Investigation, Validation, Writing – original draft, Writing – review & editing. MX: Data curation, Formal Analysis, Investigation, Methodology, Validation, Writing – original draft. RM: Data curation, Formal Analysis, Investigation, Methodology, Project administration, Validation, Writing – original draft. ZZ: Conceptualization, Data curation, Formal Analysis, Funding acquisition, Investigation, Project administration, Supervision, Validation, Writing – original draft, Writing – review & editing.
Funding
The author(s) declare that financial support was received for the research and/or publication of this article. This work was supported by funds from the National Natural Science Foundation of China (grant no. 32000148), China Postdoctoral Science Foundation (grant no. 2020M670287), and the National Key Laboratory for Tropical Crop Breeding (NKLTCB-RC202403, NKLTCBZRJJ4).
Acknowledgments
We thank Dr. Hongquan Yang (Shanghai Normal University, China) for providing us with seeds of cry1-104cry2-1 mutant. We thank Dr. Haodong Chen (Tsinghua University, China) for providing us with seeds of hy5-215, HY5 OX-1, and HY5 OX-2 lines. We thank Dr. Thierry Desnos (CEA Cadarache, France) for providing us with seeds of lpr1lpr2 mutant. We thank Dr. Dong Liu of Tsinghua University for generous helpful guidance.
Conflict of interest
The authors declare that the research was conducted in the absence of any commercial or financial relationships that could be construed as a potential conflict of interest.
Generative AI statement
The author(s) declare that no Generative AI was used in the creation of this manuscript.
Publisher’s note
All claims expressed in this article are solely those of the authors and do not necessarily represent those of their affiliated organizations, or those of the publisher, the editors and the reviewers. Any product that may be evaluated in this article, or claim that may be made by its manufacturer, is not guaranteed or endorsed by the publisher.
Supplementary material
The Supplementary Material for this article can be found online at: https://www.frontiersin.org/articles/10.3389/fpls.2025.1557118/full#supplementary-material
References
Balzergue, C., Dartevelle, T., Godon, C., Laugier, E., Meisrimler, C., Teulon, J. M., et al. (2017). Low phosphate activates STOP1-ALMT1 to rapidly inhibit root cell elongation. Nat. Commu. 8, 15300. doi: 10.1038/ncomms15300
Bariola, P. A., Howard, C. J., Taylor, C. B., Verburg, M. T., Jaglan, V. D., Green, P. J. (1994). The Arabidopsis ribonuclease gene RNS1 is tightly controlled in response to phosphate limitation. Plant J. 6, 673–685. doi: 10.1046/j.1365-313X.1994.6050673.x
Buer, C. S., Muday, G. K., Djordjevic, M. A. (2007). Flavonoids are differentially taken up and transported long distances in Arabidopsis. Plant Physiol. 145, 478–490. doi: 10.1104/pp.107.101824
Burleigh, S. H., Harrison, M. J. (1999). The down-regulation of Mt4-like genes by phosphate fertilization occurs systemically and involves phosphate translocation to the shoots. Plant Physiol. 119, 241–248. doi: 10.1104/pp.119.1.241
Bustos, R., Castrillo, G., Linhares, F., Puga, M. I., Rubio, V., Pérez-Pérez, J., et al. (2010). A central regulatory system largely controls transcriptional activation and repression responses to phosphate starvation in Arabidopsis. PloS Genet. 6, e1001102. doi: 10.1371/journal.pgen.1001102
Cabrera, J., Conesa, C. M., Del Pozo, J. C. (2021). May the dark be with roots: a perspective on how root illumination may bias in vitro research on plant-environment interactions. New Phytol. 233, 1988–1997. doi: 10.1111/nph.17936
Chen, X., Yao, Q., Gao, X., Jiang, C., Harberd, N. P., Fu, X. (2016). Shoot-to-root mobile transcription factor HY5 coordinates plant carbon and nitrogen acquisition. Curr. Biol. 26, 640–646. doi: 10.1016/j.cub.2015.12.066
Clúa, J., Montpetit, J., Jimenez-Sandoval, P., Naumann, C., Santiago, J., Poirier, Y. (2024). A CYBDOM protein impacts iron homeostasis and primary root growth under phosphate deficiency in Arabidopsis. Nat. Commun. 15, 423. doi: 10.1038/s41467-023-43911-x
Cong, W. F., Suriyagoda, L. D. B., Lambers, H. (2020). Tightening the phosphorus cycle through phosphorus-efficient crop genotypes. Trends Plant Sci. 25, 967–975. doi: 10.1016/j.tplants.2020.04.013
del Pozo, J. C., Allona, I., Rubio, V., Leyva, A., de la Pena, A., Aragoncillo, C., et al. (1999). A type 5 acid phosphatase gene from Arabidopsis thaliana is induced by phosphate starvation and by some other types of phosphate mobilising/oxidative stress conditions. Plant J. 19, 579–589. doi: 10.1046/j.1365-313X.1999.00562.x
Dong, J., Piñeros, M. A., Li, X., Yang, H., Liu, Y., Murphy, A. S., et al. (2017). An Arabidopsis ABC transporter mediates phosphate deficiency-induced remodeling of root architecture by modulating iron homeostasis in roots. Mol. Plant 10, 244–259. doi: 10.1016/j.molp.2016.11.001
Fujii, H., Chiou, T., Lin, S., Aung, K., Zhu, J. (2005). A miRNA involved in phosphate-starvation response in Arabidopsis. Curr. Biol. 15, 2038–2043. doi: 10.1016/j.cub.2005.10.016
Gao, H., Wang, T., Zhang, Y., Li, L., Wang, C., Guo, S., et al. (2021). GTPase ROP6 negatively modulates phosphate deficiency through inhibition of PHT1;1 and PHT1;4 in Arabidopsis thaliana. J. Integr. Plant Biol. 63, 1775–1786. doi: 10.1111/jipb.13153
Gao, Y. Q., Bu, L. H., Han, M. L., Wang, Y. L., Li, Z. Y., Liu, H. T., et al. (2021). Long-distance blue light signalling regulates phosphate deficiency-induced primary root growth inhibition. Mol. Plant 14, 1539–1553. doi: 10.1016/j.molp.2021.06.002
Hani, S., Cuyas, L., David, P., Secco, D., Whelan, J., Thibaud, M. C., et al. (2021). Live single-cell transcriptional dynamics via RNA labelling during the phosphate response in plants. Nat. Plants 7, 1050–1064. doi: 10.1038/s41477-021-00981-3
Jiang, C., Gao, X., Liao, L., Harberd, N. P., Fu, X. (2007). Phosphate starvation root architecture and anthocyanin accumulation responses are modulated by the gibberellin-DELLA signaling pathway in Arabidopsis. Plant Physiol. 145, 1460–1470. doi: 10.1104/pp.107.103788
Khandal, H., Singh, A. P., Chattopadhyay, D. (2020). The microRNA397b-LACCASE2 module regulates root lignification under water and phosphate deficiency. Plant Physiol. 182, 1387–1403. doi: 10.1104/pp.19.00921
Lee, S., Wang, W., Huq, E. (2021). Spatial regulation of thermomorphogenesis by HY5 and PIF4 in Arabidopsis. Nat. Commu. 12, 3656. doi: 10.1038/s41467-021-24018-7
Lei, M., Liu, Y., Zhang, B., Zhao, Y., Wang, X., Zhou, Y., et al. (2011). Genetic and genomic evidence that sucrose is a global regulator of plant responses to phosphate starvation in Arabidopsis. Plant Physiol. 156, 1116–1130. doi: 10.1104/pp.110.171736
Li, T., Lian, H., Li, H., Xu, Y., Zhang, H. (2021). HY5 regulates light-responsive transcription ofmicroRNA163 to promote primary root elongation in Arabidopsis seedlings. J. Integr. Plant Biol. 63, 1437–1450. doi: 10.1111/jipb.13099
Li, J., Terzaghi, W., Gong, Y., Li, C., Ling, J. J., Fan, Y., et al. (2020). Modulation of BIN2 kinase activity by HY5 controls hypocotyl elongation in the light. Nat. Commun. 11, 1592. doi: 10.1038/s41467-020-15394-7
Liu, D. (2021). Root developmental responses to phosphorus nutrition. J. Integr. Plant Biol. 63, 1065–1090. doi: 10.1111/jipb.13090
Liu, Y., Xie, Y., Wang, H., Ma, X., Yao, W., Wang, H. (2017). Light and ethylene coordinately regulate the phosphate starvation response through transcriptional regulation of PHOSPHATE STARVATION RESPONSE1. Plant Cell 29, 2269–2284. doi: 10.1105/tpc.17.00268
Livak, K. J., Schmittgen, T. D. (2001). Analysis of relative gene expression data using real-time quantitative PCR and the 2–ΔΔCT method. Methods 25, 402–408. doi: 10.1006/meth.2001.1262
López-Arredondo, D. L., Leyva-Gonzalez, M. A., Gonzalez-Morales, S. I., Lopez-Bucio, J., Herrera-Estrella, L. (2014). Phosphate nutrition: improving low-phosphate tolerance in crops. Annu. Rev. Plant Biol. 65, 95–123. doi: 10.1146/annurev-arplant-050213-035949
Ma, Z., Baskin, T. I., Brown, K. M., Lynch, J. P. (2003). Regulation of root elongation under phosphorus stress involves changes in ethylene responsiveness. Plant Physiol. 131, 1381–1390. doi: 10.1104/pp.012161
Maniero, R. A., Picco, C., Hartmann, A., Engelberger, F., Gradogna, A., Scholz-Starke, J., et al. (2024). Ferric reduction by a CYBDOM protein counteracts increased iron availability in root meristems induced by phosphorus deficiency. Nat. Commun. 15, 422. doi: 10.1038/s41467-023-43912-w
Mao, J., Zhang, Y., Sang, Y., Li, Q., Yang, H. Q. (2005). A role for Arabidopsis cryptochromes and COP1 in the regulation of stomatal opening. Proc. Natl. Acad. Sci. U.S.A. 102, 12270–12275. doi: 10.1073/pnas.0501011102
Mayzlish-Gati, E., De-Cuyper, C., Goormachtig, S., Beeckman, T., Vuylsteke, M., Brewer, P. B., et al. (2012). Strigolactones are involved in root response to low phosphate conditions in Arabidopsis. Plant Physiol. 160, 1329–1341. doi: 10.1104/pp.112.202358
Miotto, Y. E., Tesser da Costa, C., de Oliveira, B. H., Guzman, F., Margis, R., de Almeida, R. M. C., et al. (2019). Identification of root transcriptional responses to shoot illumination in Arabidopsis thaliana. Plant Mol. Biol. 101, 487–498. doi: 10.1007/s11103-019-00918-7
Misson, J., Raghothama, K. G., Jain, A., Jouhet, J., Block, M. A., Bligny, R., et al. (2005). A genome-wide transcriptional analysis using Arabidopsis thaliana Affymetrix gene chips determined plant responses to phosphate deprivation. Proc. Natl. Acad. Sci. U. S. A. 102, 11934–11939. doi: 10.1073/pnas.0505266102
Miura, K., Rus, A., Sharkhuu, A., Yokoi, S., Karthikeyan, A. S., Raghothama, K. G., et al. (2005). The Arabidopsis SUMO E3 ligase SIZ1 controls phosphate deficiency responses. Proc. Natl. Acad. Sci. U.S.A. 102, 7760–7765. doi: 10.1073/pnas.0500778102
Mora-Macías, J., Ojeda-Rivera, J. O., Gutiérrez-Alanís, D., Yong-Villalobos, L., Oropeza-Aburto, A., Raya-González, J., et al. (2017). Malate-dependent Fe accumulation is a critical checkpoint in the root developmental response to low phosphate. Proc. Natl. Acad. Sci. U. S. A. 114, E3563–E3572. doi: 10.1073/pnas.1701952114
Morcuende, R., Bari, R., Gibon, Y., Zheng, W., Pant, B. D., Bläsing, O., et al. (2007). Genome-wide reprogramming of metabolism and regulatory networks of Arabidopsis in response to phosphorus. Plant Cell Environ. 30, 85–112. doi: 10.1111/j.1365-3040.2006.01608.x
Muchhal, U. S., Pardo, J. M., Raghothama, K. G. (1996). Phosphate transporters from the higher plant Arabidopsis thaliana. Proc. Natl. Acad. Sci. U.S.A. 93, 10519–10523. doi: 10.1073/pnas.93.19.10519
Müller, J., Toev, T., Heisters, M., Teller, J., Moore, K. L., Hause, G., et al. (2015). Iron-dependent callose deposition adjusts root meristem maintenance to phosphate availability. Dev. Cell 33, 216–230. doi: 10.1016/j.devcel.2015.02.007
Nacry, P., Canivenc, G., Muller, B., Azmi, A., Van Onckelen, H., Rossignol, M., et al. (2005). A role for auxin redistribution in the responses of the root system architecture to phosphate starvation in Arabidopsis. Plant Physiol. 138, 2061–2074. doi: 10.1104/pp.105.060061
Nussaume, L., Kanno, S., Javot, H., Marin, E., Pochon, N., Ayadi, A., et al. (2011). Phosphate import in plants: focus on the PHT1 transporters. Front. Plant Sci. 2. doi: 10.3389/fpls.2011.00083
Osorio, M. B., Ng, S., Berkowitz, O., De Clercq, I., Mao, C., Shou, H., et al. (2019). SPX4 acts on PHR1-dependent and -independent regulation of shoot phosphorus status in Arabidopsis. Plant Physiol. 181, 332–352. doi: 10.1104/pp.18.00594
Oyama, T., Shimura, Y., Okada, K. (1997). The Arabidopsis HY5 gene encodes a bZIP protein that regulates stimulus-induced development of root and hypocotyl. Genes Dev. 11, 2983–2995. doi: 10.1101/gad.11.22.2983
Qu, Y., Liu, S., Bao, W., Xue, X., Ma, Z., Yokawa, K., et al. (2017). Expression of root genes in Arabidopsis seedlings grown by standard and improved growing methods. Int. J. Mol. Sci. 18, 951. doi: 10.3390/ijms18050951
Roschzttardtz, H., Conejero, G., Curie, C., Mari, S. (2009). Identification of the endodermal vacuole as the iron storage compartment in the Arabidopsis embryo. Plant Physiol. 151, 1329–1338. doi: 10.1104/pp.109.144444
Sanchez-Calderon, L., Lopez-Bucio, J., Chacon-Lopez, A., Cruz-Ramirez, A., Nieto-Jacobo, F., Dubrovsky, J. G., et al. (2005). Phosphate starvation induces a determinate developmental program in the roots of Arabidopsis thaliana. Plant Cell Physiol. 46, 174–184. doi: 10.1093/pcp/pci011
Sassi, M., Lu, Y., Zhang, Y., Wang, J., Dhonukshe, P., Blilou, I., et al. (2012). COP1 mediates the coordination of root and shoot growth by light through modulation of PIN1- and PIN2-dependent auxin transport in Arabidopsis. Development 139, 3402–3412. doi: 10.1242/dev.078212
Setsukinai, K., Urano, Y., Kakinuma, K., Majima, H., Nagano, T., Nagano, T. (2003). Development of novel fluorescence probes that can reliably detect reactive oxygen species and distinguish specific species, and their application to living neutrophils. J. Biol. Chem. 278, 3170–3175. doi: 10.1074/jbc.M209264200
Silva-Navas, J., Conesa, C. M., Saez, A., Navarro-Neila, S., Garcia-Mina, J. M., Zamarreno, A. M., et al. (2019). Role of cis-zeatin in root responses to phosphate starvation. New Phytol. 224, 242–257. doi: 10.1111/nph.16020
Silva-Navas, J., Moreno-Risueno, M. A., Manzano, C., Pallero-Baena, M., Navarro-Neila, S., Tellez-Robledo, B., et al. (2015). D-Root: a system for cultivating plants with the roots in darkness or under different light conditions. Plant J. 84, 244–255. doi: 10.1111/tpj.12998
Silva-Navas, J., Moreno-Risueno, M. A., Manzano, C., Téllez-Robledo, B., Navarro-Neila, S., Carrasco, V., et al. (2016). Flavonols mediate root phototropism and growth through regulation of proliferation-to-differentiation transition. Plant Cell 28, 1372–1387. doi: 10.1105/tpc.15.00857
Singh, A. P., Fridman, Y., Friedlander-Shani, L., Tarkowska, D., Strnad, M., Savaldi-Goldstein, S. (2014). Activity of the brassinosteroid transcription factors BRASSINAZOLE RESISTANT1 and BRASSINOSTEROID INSENSITIVE1-ETHYL METHANESULFONATE-SUPPRESSOR1/BRASSINAZOLE RESISTANT2 blocks developmental reprogramming in response to low phosphate availability. Plant Physiol. 166, 678–688. doi: 10.1104/pp.114.245019
Sun, Y., Zheng, Y., Yao, H., Ma, Z., Xiao, M., Wang, H., et al. (2023). Light and jasmonic acid coordinately regulate the phosphate responses under shade and phosphate starvation conditions in Arabidopsis. Plant Direct 7, e504. doi: 10.1002/pld3.504
Svistoonoff, S., Creff, A., Reymond, M., Sigoillot-Claude, C., Ricaud, L., Blanchet, A., et al. (2007). Root tip contact with low-phosphate media reprograms plant root architecture. Nat. Genet. 39, 792–796. doi: 10.1038/ng2041
Tian, W. H., Ye, J. Y., Cui, M. Q., Chang, J. B., Liu, Y., Li, G. X., et al. (2021). A transcription factor STOP1-centered pathway coordinates ammonium and phosphate acquisition in Arabidopsis. Mol. Plant 14, 1554–1568. doi: 10.1016/j.molp.2021.06.024
Wang, L., Li, Z., Qian, W., Guo, W., Gao, X., Huang, L., et al. (2011). The Arabidopsis purple acid phosphatase AtPAP10 is predominantly associated with the root surface and plays an important role in plant tolerance to phosphate limitation. Plant Physiol. 157, 1283–1299. doi: 10.1104/pp.111.183723
Wang, X., Wang, Z., Zheng, Z., Dong, J., Song, L., Sui, L., et al. (2019). Genetic dissection of Fe-dependent signaling in root developmental responses to phosphate deficiency. Plant Physiol. 179, 300–316. doi: 10.1104/pp.18.00907
Wang, Z., Zheng, Z., Liu, D. (2024). Comparative functional analyses of PHR1, PHL1, and PHL4 transcription factors in regulating Arabidopsis responses to phosphate starvation. Front. Plant Sci. 15. doi: 10.3389/fpls.2024.1379562
Wang, Z., Zheng, Z., Zhu, Y., Kong, S., Liu, D. (2023). PHOSPHATE RESPONSE 1 family members act distinctly to regulate transcriptional responses to phosphate starvation. Plant Physiol. 191, 1324–1343. doi: 10.1093/plphys/kiac521
Williamson, L. C., Ribrioux, S. P., Fitter, A. H., Leyser, H. M. (2001). Phosphate availability regulates root system architecture in Arabidopsis. Plant Physiol. 126, 875–882. doi: 10.1104/pp.126.2.875
Wu, P., Ma, L. G., Hou, X. L., Wang, M. Y., Wu, Y. R., Liu, F. Y., et al. (2003). Phosphate starvation triggers distinct alterations of genome expression in Arabidopsis roots and leaves. Plant Physiol. 132, 1260–1271. doi: 10.1104/pp.103.021022
Xiao, Y., Chu, L., Zhang, Y., Bian, Y., Xiao, J., Xu, D. (2022). HY5: A pivotal regulator of light-dependent development in higher plants. Front. Plant Sci. 12. doi: 10.3389/fpls.2021.800989
Xu, Z. R., Cai, M. L., Yang, Y., You, T. T., Ma, J. F., Wang, P., et al (2022). The ferroxidases LPR1 and LPR2 control iron translocation in the xylem of Arabidopsis plants. Mol. Plant 15, 1962–1975. doi: 10.1016/j.molp.2022.11.003
Yeh, C. M., Kobayashi, K., Fujii, S., Fukaki, H., Mitsuda, N., Ohme-Takagi, M. (2020). Blue light regulates phosphate deficiency-dependent primary root growth inhibition in Arabidopsis. Front. Plant Sci. 10. doi: 10.3389/fpls.2019.01803
Yokawa, K., Fasano, R., Kagenishi, T., Baluska, F. (2014). Light as stress factor to plant roots - case of root halotropism. Front. Plant Sci. 5. doi: 10.3389/fpls.2014.00718
Yokawa, K., Kagenishi, T., Baluska, F. (2013). Root photomorphogenesis in laboratory-maintained Arabidopsis seedlings. Trends Plant Sci. 18, 117–119. doi: 10.1016/j.tplants.2013.01.002
Zhang, Y., Wang, C., Xu, H., Shi, X., Zhen, W., Hu, Z., et al. (2019). HY5 contributes to light-regulated root system architecture under a root-covered culture system. Front. Plant Sci. 10. doi: 10.3389/fpls.2019.01490
Zheng, Z., Wang, Z., Wang, X., Liu, D. (2019). Blue light-triggered chemical reactions underlie phosphate deficiency-induced inhibition of root elongation of Arabidopsis seedlings grown in petri dishes. Mol. Plant 12, 1515–1523. doi: 10.1016/j.molp.2019.08.001
Ziegler, J., Schmidt, S., Chutia, R., Müller, J., Böttcher, C., Strehmel, N., et al. (2016). Non-targeted profiling of semi-polar metabolites in Arabidopsis root exudates uncovers a role for coumarin secretion and lignification during the local response to phosphate limitation. J. Exp. Bot. 67, 1421–1432. doi: 10.1093/jxb/erv539
Keywords: Pi starvation, light illumination, primary root growth, transcriptomic analyses, cryptochromes, HY5
Citation: Wang Z, Xia M, Ma R and Zheng Z (2025) Physiological and transcriptional analyses of Arabidopsis primary root growth in response to phosphate starvation under light and dark conditions. Front. Plant Sci. 16:1557118. doi: 10.3389/fpls.2025.1557118
Received: 08 January 2025; Accepted: 19 March 2025;
Published: 10 April 2025.
Edited by:
Weiqiang Li, Chinese Academy of Sciences (CAS), ChinaReviewed by:
Felipe Dos Santos Maraschin, Federal University of Rio Grande do Sul, BrazilYukari Nagatoshi, Japan International Research Center for Agricultural Sciences (JIRCAS), Japan
Copyright © 2025 Wang, Xia, Ma and Zheng. This is an open-access article distributed under the terms of the Creative Commons Attribution License (CC BY). The use, distribution or reproduction in other forums is permitted, provided the original author(s) and the copyright owner(s) are credited and that the original publication in this journal is cited, in accordance with accepted academic practice. No use, distribution or reproduction is permitted which does not comply with these terms.
*Correspondence: Rui Ma, bW9sbHlzZGF1QDE2My5jb20=; Zai Zheng, emhlbmd6YWlAaXRiYi5vcmcuY24=