- 1College of Agronomy, College of Life Science and Technology, State Key Laboratory of Aridland Crop Science, Gansu Agricultural University, Lanzhou, China
- 2College of Economics and Management, Hexi University, Zhangye, China
Plant tissue culture is a fundamental and widely applied technique in plant biology and agriculture. In medicinal plant research, tissue culture plays an indispensable role in the conservation of endangered species, the rapid propagation of valuable resources, the preservation of germplasm, and the production of secondary metabolites. As a representative medicinal plant of the Lonicera genus, L. japonica is widely utilized worldwide due to its significant economic, ecological, medicinal, and ornamental value. By using tissue culture technology, it is possible to significantly enhance the production of secondary metabolites in L. japonica and effectively alleviate resource shortages, providing a new approach for its sustainable utilization. This review summarizes the recent research progress on L. japonica in the field of tissue culture, covering aspects such as direct organogenesis, indirect organogenesis through callus tissues, protoplast culture, hairy root culture, and polyploid culture. Additionally, the biosynthetic pathway of chlorogenic acid was explored in detail, and the mechanism of action of inducers in plant cells was analyzed. The study focused on the potential regulatory mechanisms of inducers on chlorogenic acid. Eventually, the future development trends of medicinal plant biotechnology are envisioned, aiming to provide a broader perspective for the in-depth study of medicinal plants and to promote continuous development and innovation in this field.
1 Introduction
Lonicera are usually erect or dwarf shrubs, with a few species in the form of small trees, occasionally as twining vines, and are deciduous or evergreen (Liu et al., 2022). Its corolla is richly colored, commonly including white yellow, pale pink or purplish-red. The fruits are berries, with colors ranging from red to bluish-black or black. The Lonicera genus comprises approximately 200 species, primarily distributed across temperate and subtropical regions of North America, Europe, Asia, and northern Africa. In Asia, its distribution extends as far south as the Philippines and southern Malaysia (Jacobs et al., 2009; Park et al., 2019). In China, approximately 98 species, including 4 subspecies, 18 varieties, and 1 forma, are widely distributed across various provinces, with the highest abundance in the southwestern regions (Hsu and Wang, 1988; Yang et al., 2019a). Lonicera has significant medicinal value, with L. japonica being the most renowned. It is listed among the 35 valuable Chinese medicinal herbs by the Administration of Traditional Chinese Medicine of China. Surveys indicate that approximately 18 types of flower buds from this genus are used as Chinese herbal medicine commodities (Hsu and Wang, 1988) (Table 1). L. japonica contains diverse chemical constituents, including flavonoids, phenolic acids, cyclic enol ether terpene glycosides, volatile oils, and other bioactive substances, of which chlorogenic acid (CGA) and lignans are the main active components (Shang et al., 2011; Yang et al., 2019b). These components have various pharmacological effects such as antioxidant, anti-inflammatory, antibacterial and antiviral (Su et al., 2021; Liu et al., 2016). Therefore, L. japonica has been widely used in the fields of traditional Chinese medicine, functional foods, nutraceuticals and cosmetics (Fang et al., 2022; Liu et al., 2023) (Figure 1). Additionally, L. japonica has gained significant attention as a natural plant resource owing to its abundant bioactive compounds and high safety.
The main sources of medicinal plants include wild and cultivated resources. However, overexploitation and land degradation have led to a sharp decline in the availability of wild resources. Field cultivation is time-consuming and labor-intensive, with continuous cropping often resulting in failures due to increased disease incidence (Shi et al., 2018; Sun et al., 2011). These problems exacerbate the scarcity of medicinal plant resources and raise the risk of extinction of endangered species. According to the National Economic Forestry Association (NEFA), the global market demand for L. japonica has exceeded 20 million kilograms, with a value of $860 million, making it one of the most economically valuable medicinal plants (Lai et al., 2022). However, the long cultivation period of L. japonica, its susceptibility to pathogens and replanting diseases, and the instability of active compound content in cultivated plants severely limited farmers in meeting the growing market demand (Jia and Yan, 2024; Pan et al., 2021a).
To address these challenges, plant tissue culture technology has developed rapidly and is widely regarded as a critical tool for plant regeneration, rapid propagation of pathogen-free and disease-free materials, and efficient production of secondary metabolites. This technology offers significant advantages, including enhanced plant responses to biotic and abiotic stresses, as well as substantial improvements in the levels of desired traits and specific compounds (Tomasiak et al., 2022). Research on plant tissue culture and cell culture began in the late 19th century, when Haberlandt first introduced the concept of cell culture and pioneered the use of artificial media for the cultivation of isolated plant cells (Haberlandt, 1902). In 1934, White achieved a major breakthrough in the cultivation of isolated roots by establishing the first actively growing asexual line from isolated tomato roots, and discovered the important role of the B vitamins B1, B6 and niacin in plant growth (White, 1934). In 1965, Vasil and Hildebrandt successfully regenerated whole plants from a single isolated cell culture, scientifically validating the theory of plant cell totipotency (Vasil and Hildebrandt, 1965). During the 1960s, microspore and protoplast cultures were successfully provided. Subsequently, a large number of studies have demonstrated that undifferentiated plant cells (e.g. callus and cell suspensions) have broad application prospects in the large-scale production of secondary metabolites for pharmaceuticals and cosmetics (Xu et al., 2023; Asyakina et al., 2021).
Recent studies have shown that phenolic acids can be successfully obtained from L. japonica employing in vitro tissue cultures. This paper reviews the research progress on tissue culture of L. japonica conducted both domestically and internationally, covering the direct and indirect organogenesis pathways (as shown in Figure 2), protoplast culture, cell suspension culture and hairy root culture techniques of L. japonica. Additionally, the biosynthetic pathway of CGA, microbial transformation and metabolic regulation engineering were discussed in detail. Finally, it explores future trends in medicinal plant biotechnology, offering new perspectives and potential for further research and broader applications in this field.
2 In vitro culture techniques of L. japonica
Tissue culture can be classified based on the purpose of cultivation and the source of explants. In the study of L. japonica, several key processes of tissue culture based on cells and organs have been established (Figure 3), providing technical support for the development of L. japonica resources and further promoting the sustainable utilization of this species.
2.1 Direct organogenesis of L. japonica
The direct organogenesis pathway induces the existing organ primordia of explants to directly form adventitious shoots or roots, without the presence of callus morphology throughout the induction process (Kong et al., 2021).
In the direct organogenesis pathway, shoot induction is typically based on MS medium, with 6-BA (a cytokinin) as the primary exogenous hormone. Research by Xun (2004). indicated that the optimal medium for inducing multiple shoots and subculturing of L. japonica is MS + 6-BA 0.5 mg/L + IBA 0.05 mg/L, the optimal rooting medium is MS + IBA 1.0 mg/L + NAA 0.05 mg/L. The study also found that browning of explants is associated with the activity of polyphenol oxidase (PPO) in the tissue. PPO catalyzes the oxidation of phenolic compounds, resulting in the formation of dark-colored substances that inhibit the activity of other enzymes and cause toxicity to the explants. To mitigate the toxicity caused by browning, it is recommended to perform continuous subculturing during the early stages of cultivation. Gao and Dong (2018) used one-year-old stem segments of wild L. japonica as explants to determine the optimal medium for adventitious bud induction as MS + 6-BA 1.5 mg/L + NAA 0.12 mg/L, the best subculture medium for propagation as MS + 6-BA 1.5 mg/L + NAA 0.02 mg/L, and the optimal rooting induction medium as 1/2 MS + IBA 2.5 mg/L + NAA 0.2 - 0.6 mg/L. Yang et al. (2017) used young stems as explants and determined the optimal medium for adventitious bud induction as MS + 6-BA 2.0 mg/L + IBA 0.01-0.05 mg/L, the most suitable medium for subculturing plantlets as MS + 6-BA 1.0 mg/L + IBA 0.01-0.05 mg/L, and the best rooting induction medium as 1/2 MS + IBA 2.0 mg/L + NAA 0.5 mg/L. Wang et al. (2013) showed that the apical bud shoot induction medium of L. japonica was MS + KT 1.0 mg/L + 6-BA 2.0 mg/L + NAA 0.1 mg/L, the proliferation medium was MS + KT 1.5 mg/L + 6-BA 1.5 mg/L + NAA 0.3 mg/L, and the rooting medium was 1/2 MS + NAA 2.0 mg/L + IAA 0.15-0.20 mg/L. However, Yang et al. (2003) used B5 medium in the tissue culture of Mongolian L. japonica and found that B5 + 6-BA 2.0 mg/L + KT 0.5 mg/L + IAA 1.0 mg/L + LH 1000 mg/L had a significant effect on the induction of axillary buds.
2.2 Callus tissue
To date, various explants, including stems, leaves, buds, flower buds, and roots, have been widely used for inducing callus tissue in L. japonica, with leaves and stem segments being the most commonly used explants. Typical callus induction cultures generally use Murashige and Skoog (MS), B5, or LS basal media containing 3% sucrose and various concentrations of plant growth regulators (PGRs). Researchers have explored the effects of plant growth regulators, such as cytokinins (6-BA, KT, ZT), among which 6-BA is the most commonly used, with an optimal concentration typically ranging from 1.0 to 2.5 mg/L. Auxins play a critical role in callus induction by promoting the dedifferentiation of cells to form callus tissue and maintaining a continuous state of division and proliferation. NAA is commonly used in callus induction, typically at concentrations ranging from 0.01 to 1 mg/L. The culture temperature is typically 25°C, with a photoperiod of 14 h per day and a light intensity of approximately 2000 lux. Georges et al. (1993) found that the optimal medium for inducing L. japonica callus was LS + BA 2.7 μM + NAA 10.7 μM. However, substituting 6-BA with 2,4-D led to rapid browning of the explants, significantly reducing the induction efficiency. Liu and Qian (2007) optimized the callus induction medium by adjusting the concentrations of exogenous hormones in MS basal medium, resulting in the ideal formulation of MS + 6-BA 0.1 mg/L + NAA 1.0 mg/L. Their study showed that 6-BA was most effective at concentrations of 0.1 mg/L and 0.5 mg/L, with the former achieving an induction rate of 95.1%. NAA and IAA were both effective within the range of 0.5 to 1.5 mg/L, with NAA at 1.0 mg/L yielding the highest induction rate (97.5%), whereas IAA at the same concentration only reached 88.3%. Xiang and Gao (2007) found that although MS medium could induce callus tissue, its growth was poor due to the possible inhibitory effect of ammonium salts. In contrast, B5 medium, with a lower ammonium salt content, significantly promoted callus growth when supplemented with IAA 0.5 mg/L, BA 2.0 mg/L, and KT 0.5 mg/L. Song and Liang (1987) used young L. japonica stems and leaves as explants, and added 1 ppm 2,4-D and 0.1 ppm KT to the MS medium. The results showed that the callus grew well, with the content of CGA and isochlorogenic acid reaching approximately 50% of that in the flower buds. The callus tissues are summarised in Table 2.
2.3 Research progress on the culture of L. japonica suspension cells
In recent years, with the rapid development of biotechnology, cell suspension culture has been widely applied in physiology, biochemistry, cytology, developmental biology, genetics and molecular biology due to its advantages such as rapid cell proliferation, large-scale culture capability, and uniformity of cultured materials (Moscatiello et al., 2013; Owis et al., 2016). Different from animal cells, plant cell suspension culture can establish stable culture systems without malignant mutation, As a result, they have been widely used in studies of metabolism (Popova et al., 2023; García-Pérez et al., 2021; Meyer and Fricker, 2002), cell cycle (Fabian-Marwedel et al., 2002; Kobayashi et al., 2021), and in the research of cellular biology (Yin et al., 2024; Moniruzzaman et al., 2021; Menges et al., 2003) and other cellular processes (Errico et al., 2025; Zagorskaya and Deineko, 2017; Hosseini and Mulligan, 2002). Kim et al. (2003) conducted embryogenic cell suspension culture of L. japonica using MS medium supplemented with 2,4-D at a concentration of 4.52 μmol/L, achieving an induction rate of 46.7%. After inoculation of embryonic cell suspension into MS solid medium, a large number of somatic embryos were obtained, with a regeneration rate of 68.0%. Liu (2010) used yellowish-white, small-grained, loosely structured and fast-growing callus as explants and transferred them into liquid medium with MS + 6-BA 1.5 mg/L + KT 0.75 mg/L + NAA 0.5 mg/L, established a cell suspension culture system, and investigated the physiological and biochemical changes of cells during the cultivation process. Hu et al. (2019) transferred induced callus into liquid MS medium containing BA (1.5 mg/L), NAA (0.2 mg/L), and 2,4-D (0.1 mg/L). The study revealed that the cell growth curve followed an “S” pattern, with the biomass reaching its peak on day 12. Additionally, the suspension culture was found to contain major secondary metabolites, including 3,5-di-O-caffeoylquinic acid, 3-O-caffeoylquinic acid, 4,5-di-O-caffeoylquinic acid, and 3,4-di-O-caffeoylquinic acid. Hu (2016) successfully established a L. japonica cell suspension culture system by inoculating 0.2 g/mL callus into liquid MS medium supplemented with 6-BA (1.4 mg/L), NAA (0.5 mg/L), and 2,4-D (0.7 mg/L) under dark conditions for 7-10 days. The cell biomass peaked on the 10th day, with 826.7 mg of CGA per 100 g of dry cells. Further studies demonstrated that CGA and isochlorogenic acid A exhibit significant nitrosation inhibitory activities, which are influenced by changes in the reaction environment. Wang et al. (2022) reported that after 18 days of suspension culture, the fresh cell weight reached 676.0 ± 12.66 g/L, which was 13.52 times the initial inoculation amount. Horiike and Ohshiro (1997) isolated six curdione derivatives from the cell suspension culture of L. japonica, including (2S)-2-hydroxycurdione, (2R)-2-hydroxycurdione, (8S)-6-hydroxycurdione, (2R, 8S)-8-hydro-2-hydroxycurdione, (1S, 10S)-1,10-epoxycurdione, and (1R, 10R)-1,10-epoxycurdione. Yamamoto et al. (1999) discovered that cell suspension cultures of L. japonica do not produce iridoid or secoiridoid glycosides. However, the cells can utilize 7-deoxyloganin 7-hydroxylase to convert 7-deoxyloganin into loganin and secoiridoid glycosides. Suspension cultures are summarized in Table 3.
2.4 Culture of L. japonica protoplasts
Plant protoplasts refer to naked cells that retain cellular totipotency after the removal of the cell wall (Avila-Peltroche et al., 2020). As an important tool in modern plant biology, protoplasts serve not only as ideal recipient materials for foreign gene introduction but also as an effective means for somatic hybridization to develop new cultivars (Seidel et al., 2024). Liu et al. (2012a) used vigorously growing and loosely structured Lonicera callus as material for protoplast isolation. The enzymatic solution was prepared with 1.5% cellulase and 0.25% pectinase, supplemented with 0.7 mol/L mannitol as an osmotic stabilizer in CPW (cell-protoplast washing solution). The enzymatic digestion was performed under static conditions at 25°C for 12 h, yielding a large quantity of high-quality protoplasts.
2.5 Research progress of the hairy roots culture of L. japonica
The transformation of hairy roots and their tissue and organ culture offers multiple advantages, including genetic and biochemical stability, rapid growth, independence from exogenous plant hormones, and the ability to stably produce active compounds. Furthermore, as hairy roots originate from single cells, they facilitate extensive clonal selection, making the use of hairy root cultures for high-yield secondary metabolite production a prominent research focus worldwide (Sharma et al., 2013; Kundu et al., 2018). The results showed that when the Agrobacterium rhizogenes R1000 culture reached an OD600 value of approximately 0.6 and was diluted 40-fold before immersing explants for 4 minutes, the induction rate was highest, reaching 58%. Pre-culturing explants for 5 days, followed by co-cultivation with Agrobacterium for 3 days and subsequent transfer to a medium containing 500 mg/L carbenicillin for sterilization, was identified as the key condition for achieving optimal induction (Yuan and He, 2007). He and Zhou (2008) further optimized the induction conditions for L. japonica hairy roots. Their results indicated that leaf explants from tissue culture seedlings, after 5 days of pre-cultivation, immersion in bacterial suspension diluted 45-fold for 4 minutes, and 3 days of co-cultivation, followed by transfer to 1/2 MS medium containing 500 mg/L carbenicillin, achieved the highest induction rate of 54.3%. The successful establishment of L. japonica hairy roots provides an important foundation and evidence for the construction of Ri plasmid-mediated genetic transformation systems and the industrial-scale production of medicinal active compounds through hairy root cultures.
2.6 Polyploid induction of L. japonica
Compared to diploid plants, polyploid plants generally exhibit greater vigor, yield, and quality, as well as enhanced resistance to various biotic and abiotic stresses. Additionally, polyploid breeding can overcome the incompatibility issues of distant hybridization, making it an important approach for germplasm innovation in medicinal plants (Schatlowski and Köhler, 2012; van Berkum et al., 2010). Liu et al. (2012b) induced tetraploid plants from L. japonica “Hongxing No. 2” callus tissues by soaking them in 0.1% colchicine for 15 h. Compared to diploid plants, the tetraploid plants exhibited deeper leaf color, slower growth, shorter and thicker internodes, as well as significantly enlarged stomata and guard cells with reduced stomatal density. Wang and Zhao (2012; 2014) used a modified L.D. Cua method to artificially mutate diploid L. japonica. Cytological and early morphological analyses of the M1 mutant plants revealed that while the control plants were diploid (2n = 2x = 18), the M1 mutant plants were tetraploid (2n = 4x = 36). The tetraploid plants exhibited enhanced growth vigor, deeper leaf color, larger and thicker leaves, and a reduced leaf shape index after chromosome doubling. “Jiufeng No. 1”, a homologous tetraploid bred using polyploid breeding techniques, was developed from the traditional L. japonica cultivar “Damaohua” from Pingyi. Compared to its parent “Damaohua”, its CGA content increased by 30%, and its average yield improved by 58.79% (Xing, 2006). Zhang et al. (2011) induced allopolyploid lines of hybrid L. japonica under in vitro tissue culture conditions. Field agronomic observations showed that the flower bud size of the polyploid lines was significantly larger, and the average CGA content in the allopolyploid lines was 1.34 times that of the hybrid diploid control (CK).
3 Research on the metabolic regulation of CGA in L. japonica
The synthesis of CGA originates from the complex phenylpropanoid metabolic pathway (Hu et al., 2024; Pan et al., 2021b). Inducers are substances capable of activating or enhancing the synthesis of secondary metabolites in plants (Petrova et al., 2024; Bhaskar et al., 2022). Under the influence of inducers, plants undergo stress responses characterized by the activation of defense-related genes or the inactivation of non-defense-related genes, transient protein phosphorylation or dephosphorylation, and the expression of related enzymes. These molecular changes provide critical insights into the biosynthetic pathways of secondary metabolites (Narayani and Srivastava, 2017; Yamaner et al., 2013).
3.1 Metabolic pathway of CGA biosynthesis
The synthesis of CGA originates from the complex phenylpropanoid pathway. Based on existing literature, three main biosynthetic pathways for CGA have been summarized (Figure 4). Initially, glucose in plants is catalyzed by specific enzymes to form shikimic acid, which is further converted into phenylalanine. Subsequently, through the sequential catalysis of phenylalanine ammonia-lyase (PAL), cinnamate-4-hydroxylase (C4H), and 4-coumarate-CoA ligase (4CL), phenylalanine is transformed into p-coumaroyl-CoA. p-Coumaroyl-CoA acts as a precursor for the biosynthesis of flavonoids and lignin, and it also represents a critical starting point for the CGA biosynthetic pathway. (1) Route 1: p-Coumaroyl-CoA reacts with quinic acid (QA) under the catalysis of hydroxycinnamoyl-CoA shikimate/quinate hydroxycinnamoyl transferase (HCT) to produce p-coumaroyl quinic acid, which is subsequently hydroxylated by coumarate-3-hydroxylase (C3H) to form CGA (Valiñas et al., 2015) (Figure 4, Route 1). (2) Route 2: p-Coumaroyl-CoA combines with shikimic acid to form p-coumaroyl shikimic acid, which is hydroxylated by coumaric acid-3-hydroxylase (C3H) to produce caffeoyl shikimic acid. Subsequently, caffeoyl-CoA is synthesized by hydroxycinnamoyl-CoA shikimate/quinate hydroxycinnamoyl transferase (HCT). Finally, caffeoyl-CoA reacts with quinic acid under the catalysis of hydroxycinnamoyl-CoA: quinate hydroxycinnamoyl transferase (HQT) to form CGA via esterification (Tang et al., 2021; Niggeweg et al., 2004) (Figure 4, Route 2). (3) Route 3: Caffeoyl glucose is converted into CGA by quinic acid hydroxycinnamoyl transferase (Tang et al., 2021; Niggeweg et al., 2004) (Figure 4, Route 3).
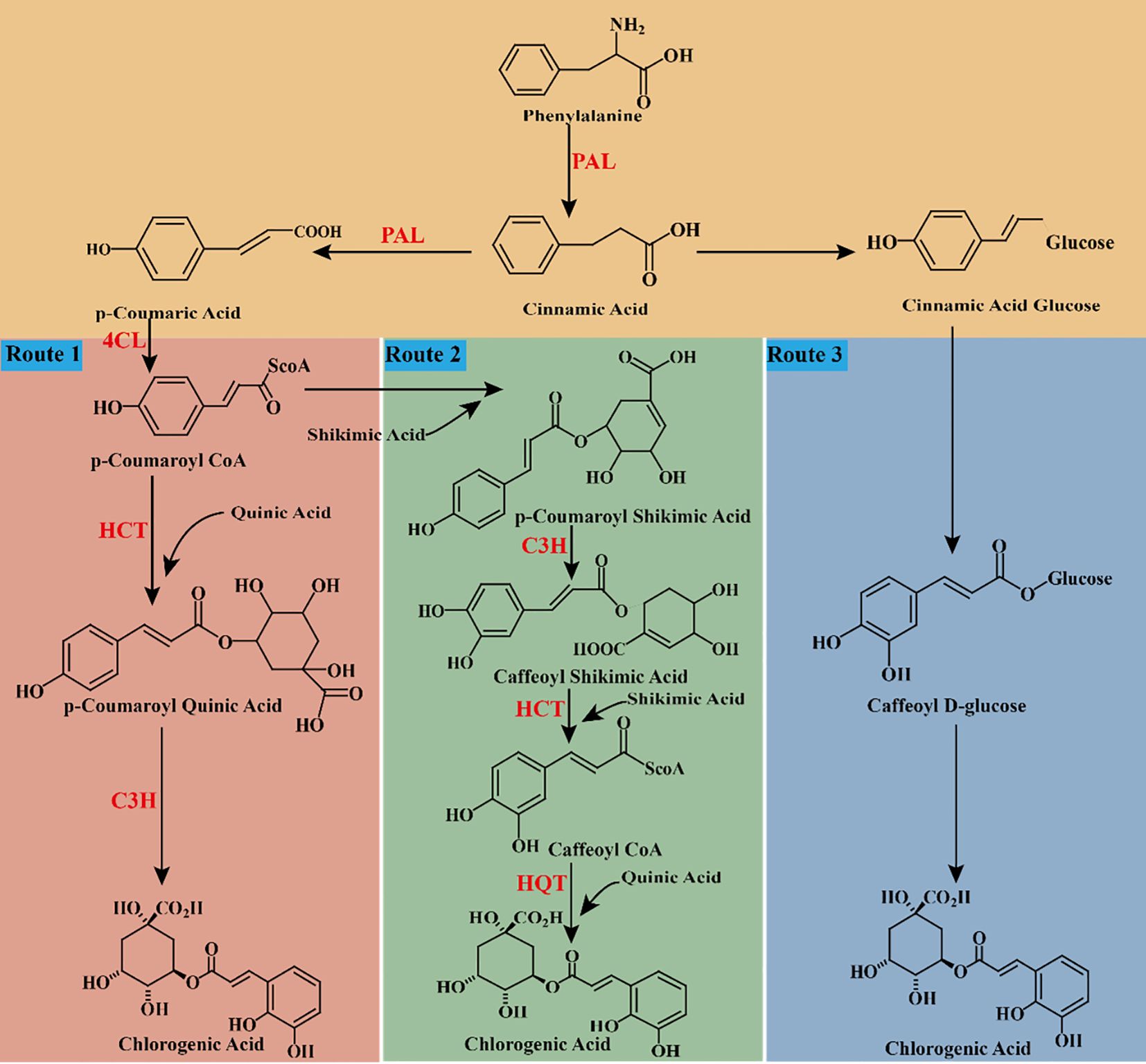
Figure 4. chematic cartoon displays 3 synthetic pathways of CGA. PAL: phenylalanine ammonia-lyase. C4H: cinnamate-4-hydroxylase. 4CL: 4-coumarate-CoA ligase. C3H, coumaric acid-3-hydroxylase; HCT, shikimate/quinate hydroxycinnamoyl transferase; HQT, quinate hydroxycinnamoyl transferase.
3.2 The function of key rate-limiting enzyme genes in the CGA synthesis pathway
Studies have shown that HQT (Luo et al., 2018; Zhang et al., 2016; Wu et al., 2014; Liu et al., 2012c), PAL (Wu et al., 2024; Li et al., 2024a), 4CL (Li et al., 2015), HCT (Chen et al., 2024; He et al., 2013), and C3H (Chen et al., 2020) are key enzymes in the biosynthetic pathway of CGA. These enzymes play a crucial role in the biosynthesis of CGA in L. japonica.
PAL is a key bridge between primary and secondary metabolism, catalyzing the conversion of phenylalanine to cinnamic acid. It controls the metabolic flux of precursors into the phenolic pathway and plays an essential role in regulating the overall level of accumulated phenolic compounds (Pan et al., 2021b; Clé et al., 2008) Therefore, PAL plays a crucial role in plant growth and development, as well as in responding to biotic and abiotic stress defenses, such as mechanical damage and ultraviolet radiation (Zhao et al., 2022). C4H is the second key rate-limiting enzyme in the phenylpropanoid pathway, localized to the endoplasmic reticulum of plant cells. It belongs to the cytochrome P450 enzyme family, specifically the CYP73 subgroup (Zhang et al., 2020). As a P450 protein, C4H catalyzes an irreversible reaction, using NADPH as an electron donor in the presence of oxygen and reduced nicotinamide adenine dinucleotide phosphate (NADPH). This enzyme catalyzes the conversion of trans-cinnamic acid to p-coumaric acid (Cheng et al., 2018). 4CL is a major branch point for cinnamic acid derivatives, converting them into different types of coenzyme A derivatives, including p-coumaric acid, caffeic acid, ferulic acid, and cinnamic acid. These intermediates are the precursors for synthesizing phenylpropanoid compounds such as lignin, CGA, and flavonoids (Li et al., 2015; Li and Nair, 2015). C3H is another key enzyme in the CGA biosynthesis pathway and the second hydroxylase in the biosynthesis of fatty acids, caffeic acid, and other phenolic acids. It belongs to the CYP98A family of cytochrome P450 monooxygenases (Yang et al., 2020). HCT belongs to the BAHD acyltransferase family and contains two conserved structural domains, HXXXD and DFGWG, which are present in all terrestrial plants (Yang et al., 2003). In the phenylpropanoid metabolic pathway, HCT is located at both the upstream and downstream junctions of the hydroxylation process catalyzed by C3H, enabling it to regulate the target genes of both the upstream and downstream pathways. It not only generates p-coumaroyl shikimate/quinic acid as substrates for C3H but also converts the products generated by C3H, caffeoyl shikimate/quinic acid, into caffeoyl-CoA. Therefore, HCT is considered to play a dual role in the phenylpropanoid hydroxylation pathway (Chao et al., 2021). HQT also belongs to the BAHD acyltransferase family and contains the two gene sequences HXXXD and DFGWG (Yang et al., 2003). HQT is tightly linked with HCT. Unlike the substrate versatility of HCT, HQT has substrate specificity and is the key rate-limiting enzyme in the final step of CGA biosynthesis. It catalyzes the ester exchange between caffeoyl-CoA and quinic acid, producing structurally different CGA derivatives (Li et al., 2019).
3.3 Elicitors
Inducers are a class of chemical substances or biological factors that promote the production of target metabolites and induce physiological changes in plants (Petrova et al., 2024; Hatami et al., 2019). Studies have shown that adding inducers can effectively reduce cultivation costs, increase cultivation efficiency, promote the production of medicinal plants, and enhance the yield of secondary metabolites, ultimately boosting the production of medicinal plants (Bhaskar et al., 2022; Narayani and Srivastava, 2017; Thakur et al., 2020). Inducers come in a wide variety of types and can be classified based on their origin into biological inducers, abiotic inducers, and novel nanoparticle inducers (Hussain et al., 2022). The transduction of induction signals within plants is mediated by multiple parallel or interconnected signaling pathways. These pathways establish an effective defense system in response to various external stimuli, leading to different reactions both inside and outside the plant (Zhang et al., 2012; Malik et al., 2011). Generally, the induction effect of inducers in plants can be divided into four steps: first, the induction signal is recognized and bound by receptors on the cell membrane; next, ion channels across the cell membrane are altered; then, the second messenger transduces the signal to the cell nucleus, activating gene regulatory factors; and finally, specific biological effects are generated in the plant (Baenas et al., 2014). The interaction between the inducer molecules and specific receptors on the surface of plant cell membranes is the most critical step in the action of inducers (Ferrari, 2010; Shasmita et al., 2018). After the induction signal is sensed, ion channels on the cell membrane are altered, leading to an increase in cytoplasmic Ca2+, efflux of Cl-/K+, and influx of H+, resulting in cytoplasmic acidification. Subsequently, the second messenger transduces the inducer signal into the cell (Abdul Malik et al., 2020; Ludwig et al., 2004; Shabala and Pottosin, 2014). Inside the cell, mitogen-activated protein kinases (MAPKs) and reduced coenzyme II (NADPH) are activated, producing reactive oxygen species (ROS) and reactive nitrogen species (RNS) (Zhao et al., 2005; Mishra et al., 2012). Ultimately, regulatory factors within the cell are activated, regulating the expression of intracellular defense genes. This may directly or indirectly lead to the synthesis of secondary metabolites to resist changes in the external environment (as shown in Figure 5).
3.4 Effect of inducers on CGA of L. japonica
To enhance the CGA content in L. japonica as in Figure 6, Gu et al. (2024) proposed an induction strategy based on yeast polysaccharide and revealed its induction mechanism. The study revealed significant differences in active component content between the 0.1 g/L yeast polysaccharide treatment group and the control group. Transcriptome analysis identified 218 differentially expressed genes, with 60 upregulated and 158 downregulated. Further analysis revealed 12 key genes involved in the biosynthesis of phenolic compounds, including PAL1, PAL2, PAL3, 4CL1, 4CL, CHS1, CHS2, CHS, CHI1, CHI2, F3H, and SOH, of which SOH being a specific gene for the synthesis of caffeic acid and CGA. Methyl jasmonate (MeJA), salicylic acid (SA), and ultraviolet radiation (UV) can significantly induced and regulated the synthesis of CGA compounds in L. japonica suspension-cultured cells. Under conditions of MeJA at 200 μmol/L, SA at 50 μmol/L, UV exposure for 2 h, and a 5-day induction period, the total CGA content in suspension cells reached 4.6 times that of the control group. Further transcriptome analysis revealed that, after MeJA induction (200 μmol/L) for 4, 10, and 20 h, the expression levels of key CGA biosynthetic enzyme genes: C4H, PAL, HCT, C3′H, CAD, and 4CLwere significantly upregulated compared to the control (0 h) (Li, 2018). Ecological conditions play a critical role in the accumulation and synthesis of secondary metabolites in medicinal plants, including altitude, temperature, light intensity, and light quality (Li et al., 2020). Chen et al. (2023) found that, after 7 days of shading treatment, CGA content in L. japonica leaves significantly decreased by 1.78-fold. Concurrently, the overall transcriptional expression of CGA biosynthetic pathway-related genes was downregulated, while the expression of photosynthetic signal protein-related genes was upregulated. During the light signaling transduction process, the transcriptional expression of PHOT, HY5, and PIF was significantly influenced by shading treatment, regulating the expression of transcription factors (TFs) such as MYB, bHLH, and WRKY. These regulatory effects collectively led to a reduction in CGA content. Moreover, it was demonstrated that the transcriptional expression of HY5 in L. japonica leaves positively correlates with CGA content, confirming that decreased light intensity downregulates HY5 expression, which is a key factor contributing to the reduction in CGA levels. Salt-alkali stress can also promote the accumulation of phenolic compounds in L. japonica leaves. Yan et al. (2017) demonstrated that NaCl stress activates the synthesis of phenolic compounds, as evidenced by a significant increase in the transcription level and activity of the PAL gene, accompanied by an elevated concentration of phenolic compounds. After 15 days of 150 and 300 mM NaCl stress, the CGA content in leaves increased by 67.43% and 48.86%, respectively, while the luteoloside content increased by 54.26% and 39.74%, respectively. Cai et al. (2021) further revealed that the synthesis of phenolic acid metabolites in L. japonica under NaCl stress is closely related to the expression of upstream enzymes in the phenylalanine metabolism pathway, including PAL, C4H, and 4CL. Additionally, HCT, C3′H, and COMT were significantly upregulated during phenolic acid synthesis, while FLS and CHI showed marked increases in expression during flavonoid biosynthesis. Zhang et al. (2022a) found that after 30 days of 10°C low-temperature stress, the CGA and luteoloside contents in L. japonica significantly increased, reaching 23 times and 17 times that of the control group, respectively. Simultaneously, the expression of CGA biosynthesis-related genes PAL and 4CL was significantly upregulated under low-temperature stress, while the upregulation of CHS and CYP75B1 activated the luteoloside biosynthesis pathway. These findings suggest that low-temperature stress promotes the accumulation of metabolites by regulating the phenylalanine metabolism and associated secondary metabolic pathways. The inducers on CGA content in L. japonica are summarised in Table 4.
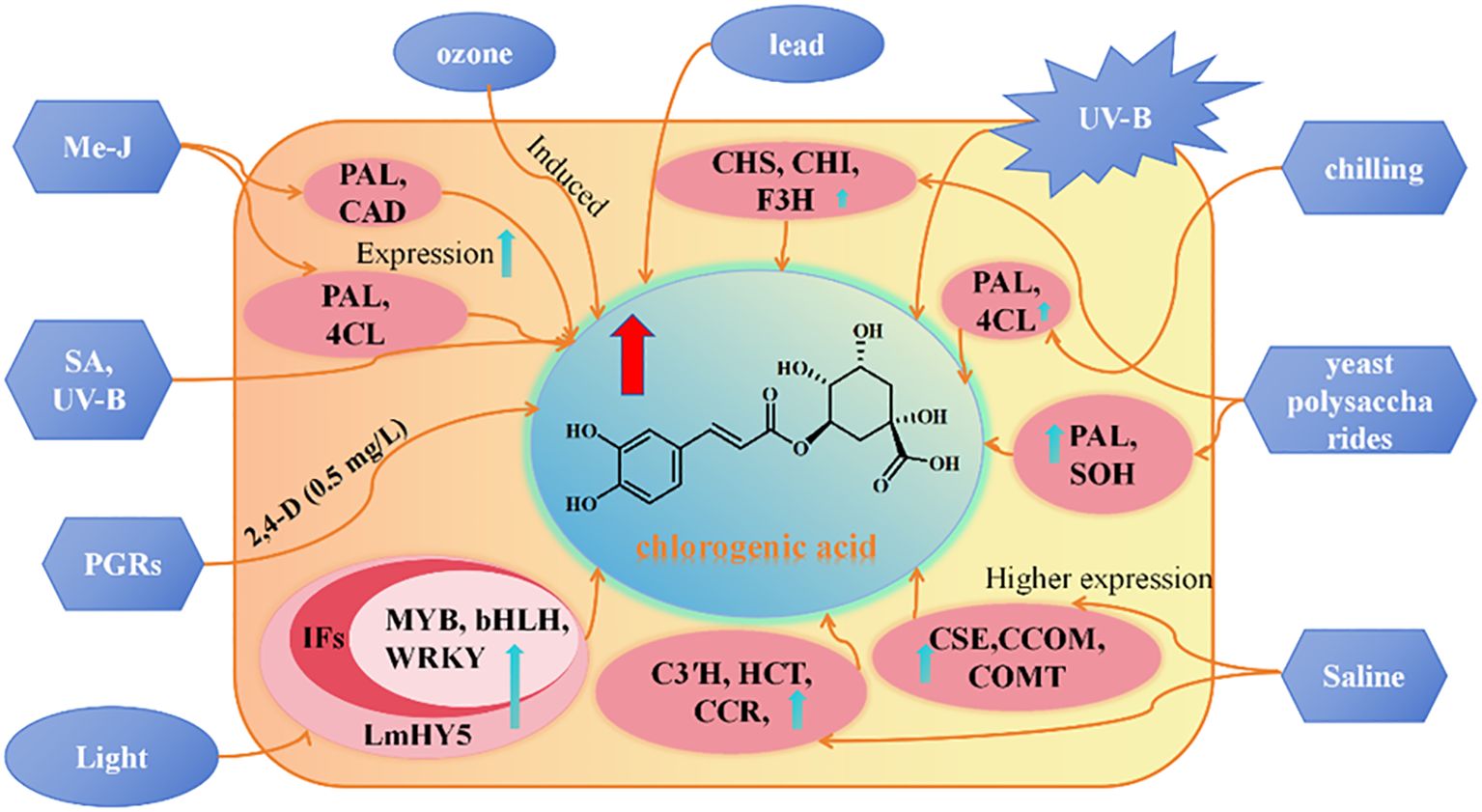
Figure 6. Study on the regulation of secondary metabolites of L. japonica. Note: The blue boxesrepresent biological and abiotic elicitors, the pink boxes represent genes, and the brown boxesrepresent secondary metabolite types. methyl jas-monate (MJ), salicylic acid (SA), chalcone synthase (CHS), chal-cone isomerase (CHI), phenylalanine ammonia (PAL), cinnamic acid 4-hydroxylase (C4H), Cinnamyl alcohol dehydrogenase (CAD), 4-coumarate-CoA ligase (4CL), flavanone 3-hydroxylase (F3H), caffeoylshikimate esterase (CSE), caffeoyl-CoAO-methyltransferase (CCOM), caffeic acid 3-O-methyltransferase (COMT), candidate catalytic enzymes (C3′H), hydroxycinnamoyl transferase (HCT), cinnamoyl-CoA reductase (CCR), Light sig-nalling of L. macranthoides (LmHY5), transcription factors (TFs).
4 Conclusion and prospects
4.1 Conclusion
Tissue culture technology, as an essential component of modern plant biotechnology, holds significant application potential. It not only provides technological support for the efficient utilization of medicinal plant resources and the production of active ingredients, but also offers innovative solutions for plant breeding, genetic improvement, and resource conservation. With the continuous development of biotechnology, the integration of tissue culture technology with other modern biotechnologies is expected to drive the efficient and sustainable development of the medicinal plant industry, providing richer resources and technological support for global sectors such as pharmaceuticals and agriculture. This review summarizes the latest research progress on the in vitro culture techniques of L. japonica and explores the application and impact of elicitors in the CGA biosynthesis pathway. In vitro culture techniques, such as direct organogenesis, callus culture, suspension cell culture, protoplast culture, hairy root culture, and polyploid induction, provide various potential pathways for the production of secondary metabolites and resource conservation in L. japonica. Regarding the biosynthesis of CGA, this review also discusses the key enzyme genes involved in the process, including PAL, C4H, 4CL, C3’H, HCT, and HQT, which play critical roles in the metabolic pathway of L. japonica.
4.2 Prospects
Although current research has revealed the potential of L. japonica in vitro culture techniques for CGA synthesis, future studies still face several challenges and research directions.
Firstly, breakthroughs in anther culture techniques. During the literature review, no previous research on the anther culture of L. japonica via the indirect organogenesis pathway was found. Compared to other explant culture methods, anther culture can directly form haploid plants from pollen, which can then be converted into homozygous plants through polyploid induction. The resulting homozygous plants exhibit higher genetic stability due to identical alleles at each locus in their genome. It can also accelerate germplasm innovation by selecting and cultivating specific pollen to obtain plants with particular traits or superior genes. More importantly, anther culture provides a powerful platform for genomics research and genetic improvement. By combining gene editing technologies, such as CRISPR/Cas9, precise gene manipulation can be conducted during the anther culture process, further advancing molecular breeding and genomic selection applications in plants. Furthermore, when combined with molecular marker techniques, anther culture can more accurately select plants with desired traits, thus improving breeding efficiency. Therefore, it is evident that the anther culture technology for L. japonica needs to be advanced.
Secondly, in-depth study of the regulatory mechanisms of elicitors. Although elicitors have been confirmed to promote the synthesis of CGA, the molecular mechanisms by which they regulate the expression of key enzyme genes through signal transduction pathways remain poorly understood. Future research should focus on how elicitors regulate the expression of key enzyme genes in the CGA biosynthesis pathway through pathways such as MAPK signaling, ROS production, and Ca2+ signaling, to reveal their complex regulatory mechanisms.
Thirdly, challenges in the Scalable Production of In Vitro Culture Systems. Tissue culture technology is one of the application areas of plant biotechnology that allows the extraction of valuable plant metabolites under controlled conditions. Compared to traditional methods, the production of secondary metabolites through plant cell and tissue culture offers significant advantages. These advantages include the ability to produce target metabolites under controlled conditions, independent of environmental factors, and the potential to optimize culture conditions to enhance secondary metabolite yields. the ability to provide adequate yields, if necessary, taking into account the balance between supply and demand, and thus to regulate the market on a regular basis. Additionally, in vitro culture can help balance supply and demand by ensuring sufficient production when needed, allowing for market regulation. It also offers a rapid production rate that is unaffected by political constraints. Furthermore, it enables the production of disease-free and contaminant-free plant materials, and any plant species, regardless of whether they originate from tropical or subtropical regions, can be cultivated in vitro. Although in vitro culture technology has a high potential for metabolite accumulation, it still faces several technical challenges in large-scale production, such as standardization of culture conditions, medium cost, contamination control and production efficiency. In order to overcome these challenges and maximize its potential, future research needs to focus on optimizing scale-up strategies for in vitro culture systems, improving the balance between cell growth and metabolism, reducing production costs while enhancing economic viability, and exploring the application of gene editing and regulatory approaches in large-scale production.
Fourthly, comprehensive optimization of in vitro culture technology. Different in vitro culture techniques, such as direct organogenesis, callus culture, suspension cell culture, protoplast culture, hairy root culture, and polyploid breeding, each have their own advantages. However, there is a lack of detailed mechanistic studies on how these techniques influence the biosynthetic pathway of CGA at the molecular level. Future research could integrate advanced technologies such as transcriptomics, proteomics, and metabolomics to comprehensively analyze the regulatory mechanisms by which different culture techniques influence CGA biosynthesis. This would help elucidate the intricate regulatory effects of various culture methods on secondary metabolite synthesis in plants, providing a theoretical foundation for optimizing culture conditions at an industrial scale to enhance CGA yield. Additionally, studies should explore how culture techniques affect CGA biosynthesis by modulating plant signaling pathways, hormone levels, and stress responses. Building on this foundation, the integration of molecular biology and gene editing technologies may enable precise regulation of plant secondary metabolism, thereby improving the efficiency and stability of CGA production.
Fifthly, exploration of Genotypic Differences. Current research primarily focuses on a single genotype of L. japonica, whereas different genotypes may respond differently to in vitro culture conditions and elicitors. Different genotypes may exhibit significant variations in response to in vitro culture conditions and elicitors, and these differences may lead to distinct accumulations of bioactive compounds by regulating the expression of key enzyme genes in secondary metabolic pathways. Comparative studies across multiple genotypes can help identify superior germplasm with high yields of specific metabolites, providing a stable raw material supply for pharmaceutical development. Significant differences in the response of different genotypes of L. japonica in tissue culture underscore the importance of including multiple genotypes in research. This approach helps comprehensively evaluate the universality and specificity of key culture parameters (such as explant selection, sterilization methods, and hormone ratios) across different genotypes, providing theoretical foundations and technical support for establishing more efficient and standardized culture systems. Research on multi-genotype tissue culture can identify genotypes with faster growth and stronger metabolic product synthesis capabilities, and by combining rapid propagation techniques, it can achieve sustainable resource utilization. Furthermore, based on research data from different genotypes, a “genotype-phenotype-metabolome” database for L. japonica can be established, supporting precision breeding and the development of functional products. Therefore, it is crucial to include multiple genotypes of L. japonica in future research to conduct in-depth studies on tissue culture and secondary metabolite biosynthesis pathways.
Author contributions
JC: Resources, Writing – original draft. YC: Conceptualization, Resources, Writing – review & editing. FG: Resources, Writing – original draft, Writing – review & editing. PD: Conceptualization, Resources, Writing – original draft. CZ: Resources, Writing – original draft, Writing – review & editing. WL: Resources, Software, Writing – original draft. HW: Resources, Software, Writing – review & editing.
Funding
The author(s) declare that financial support was received for the research and/or publication of this article. This study was supported by the “Star of Innovation” project for graduate students in Gansu Province in 2023 (NO.2023CXZX-648); Lonicera japonica Flos seedling breeding and integrated application of cost-saving and efficiency technology (2021YFD11005042); Agriculture and Rural Affairs of the People’s Republic of China Foundation for Traditional Chinese Medicinal Materials Advantaged Characteristic Industry Cluster Construction of Improved Variety Breeding (No. 2022GSCYJQ07-33); National Key R & D Project of China (No. 2018YFC1706301); A Grant for a Chief Expert of Traditional Chinese Medicinal Industry in Modern Agricultural Industry System &Safety (No. GARS-ZYC-1); and the Breeding and Base Construction of New Varieties of Chinese Medicinal Materials (NO. 7010722087). Gansu Provincial Youth Science and Technology Fund (No.23JRRA1444); Gansu Provincial Department of Education: Young Doctoral Support Project (No. 2023-QB-125); Lanzhou youth science and technology talent innovation project (No. 2023-QN-162); Gansu Agricultural University Public Recruitment Doctoral Research Initiation Fund Project (No. GAU-KYQD-2022-07).
Conflict of interest
The authors declare that the research was conducted in the absence of any commercial or financial relationships that could be constructed as a potential conflict of interest.
Generative AI statement
The author(s) declare that no Generative AI was used in the creation of this manuscript.
Publisher’s note
All claims expressed in this article are solely those of the authors and do not necessarily represent those of their affiliated organizations, or those of the publisher, the editors and the reviewers. Any product that may be evaluated in this article, or claim that may be made by its manufacturer, is not guaranteed or endorsed by the publisher.
References
Abdul Malik, N. A., Kumar, I. S., Nadarajah, K. (2020). Elicitor and receptor molecules: orchestrators of plant defense and immunity. Int. J. Mol. Sci. 21, 963. doi: 10.3390/ijms21030963
Asyakina, L., Sukhikh, S., Ivanova, S., Prosekov, A., Ulrikh, E., Chupahin, E. (2021). Determination of the qualitative composition of biologically-active substances of extracts of in vitro callus, cell suspension, and root cultures of the medicinal plant Rhodiola rosea. Biomolecules 11, 365. doi: 10.3390/biom11030365
Avila-Peltroche, J., Won, B. Y., Cho, T. O. (2020). Optimization of protoplast isolation from the gametophytes of brown alga Undaria pinnatifida using response surface methodology. J. Appl. Phycol. 32, 2233–2244. doi: 10.1007/s10811-020-02095-3
Baenas, N., García-Viguera, C., Moreno, D. A. (2014). Elicitation: A tool for enriching the bioactive composition of foods. Molecules 19, 13541–13563. doi: 10.3390/molecules190913541
Bhaskar, R., Xavier, L. S. E., Udayakumaran, G., Kumar, D. S., Venkatesh, R., Nagella, P. (2022). Biotic elicitors: A boon for the in-vitro production of plant secondary metabolites. Plant Cell Tiss. Organ. Cult. 149, 7–24. doi: 10.1007/s11240-021-02131-1
Cai, Z. C., Wang, C. C., Chen, C. H., Chen, H., Yang, R., Chen, J. J., et al. (2021). Omics map of bioactive constituents in Lonicera japonica flowers under salt stress. Ind. Crops Prod. 167, 113526. doi: 10.1016/j.indcrop.2021.113526
Cambecèdes, J., Duron, M., Decourtye, L. (1991). Adventitious bud regeneration from leaf expiants of the shrubby ornamental honeysuckle, Lonicera nitida Wils. cv. ‘Maigrün’: effects of thidiazuron and 2,3,5-triiodobenzoic acid. Plant Cell Rep. 10, 471–474. doi: 10.1007/BF00233817
Chao, N., Qi, Q., Li, S., Ruan, B., Jiang, X. N., Gai, Y. (2021). Characterization and functional analysis of the Hydroxycinnamoyl-CoA: shikimate hydroxycinnamoyl transferase (HCT) gene family in poplar. PeerJ 9, e10741. doi: 10.7717/peerj.10741
Chen, Z. M., Li, C., Wei, J. T., Li, X. R., Liu, Y., Guo, Q. (2024). Research progress in the regulation of chlorogenic acid biosynthesis and its application. Biotechnol. Bull. 40, 57–71. doi: 10.13560/j.cnki.biotech.bull.1985.2023-0728
Chen, Y., Wang, A. Q., Liu, C., Long, Y. Q., Liu, X., Zeng, J., et al. (2020). Cloning and expression patterns of LmC3H1 gene in Lonicera macranthoides and its correlation with chlorogenic acid content. Chin. J. Exp. Tradit. Med. Formulae 26, 167–175. doi: 10.13422/j.cnki.syfjx.20200913
Chen, Y. C., Xu, N., Du, L. H., Zhang, J. H., Chen, R., Zhu, Q. F., et al. (2023). Light plays a critical role in the accumulation of chlorogenic acid in Lonicera macranthoides Hand.-Mazz. Plant Physiol. Biochem. 196, 793–806. doi: 10.1016/j.plaphy.2023.02.016
Cheng, S. Y., Yan, J. P., Meng, X. X., Zhang, W. W., Liao, Y. L., Ye, J. B., et al. (2018). Characterization and expression patterns of a cinnamate-4-hydroxylase gene involved in lignin biosynthesis and in response to various stresses and hormonal treatments in Ginkgo biloba. Acta Physiol. Plant 40, 7. doi: 10.1007/s11738-017-2585-4
Clé, C., Hill, L. M., Niggeweg, R., Martin, C. R., Guisez, Y., Prinsen, E., et al. (2008). Modulation of chlorogenic acid biosynthesis in Solanum lycopersicum; consequences for phenolic accumulation and UV-tolerance. Phytochemistry 69, 2149–2156. doi: 10.1016/j.phytochem.2008.04.024
Du, L. D., Li, D. M., Zhang, J. J. (2020). Elicitation of Lonicera japonica Thunb suspension cell for enhancement of secondary metabolites and antioxidant activity. Ind. Crops Prod. 156, 112877. doi: 10.1016/j.indcrop.2020.112877
Errico, S., Mastrobuono, V., Pagliarello, R., Bennici, E., Tavazza, R., Verardi, A., et al. (2025). Consumer acceptance of edible hydrogels obtained by plant cell culture technology and by-products valorization: An Italian case study for future innovation of the plate. Innov. Food Sci. Emerg. Technol. 100, 103893. doi: 10.1016/j.ifset.2024.103893
Fabian-Marwedel, T., Umeda, M., Sauter, M. (2002). The rice cyclin-dependent kinase-activation kinase R2 regulates S-phase progression. Plant Cell 14, 197–210. doi: 10.1105/tpc.010386
Fang, L., Long, N., Li, Y., Liao, X., Shi, L., Zhao, H. (2022). Transfer behavior of pesticides from honeysuckle into tea infusions: Establishment of an empirical model for transfer rate prediction. Ecotoxicol. Environ. Saf. 234, 113377. doi: 10.1016/j.ecoenv.2022.113377
Ferrari, S. (2010). “Biological elicitors of plant secondary metabolites: Mode of action and use in the production of nutraceutics,” in Bio-Farms for nutraceuticals, vol. 698 . Eds. Giardi, M. T., Rea, G., Berra, B. (Springer US, Boston, MA), 152–166. doi: 10.1007/978-1-4419-7347-4_12
Gao, Q. M., Dong, C. Y. (2018). High efficient regeneration of wild Lonicera japonica Thunb. J. West China Forest. Sci. 47, 59–63+79. doi: 10.16473/j.cnki.xblykx1972.2018.04.011
García-Pérez, R., Miras-Moreno, B., Lucini, L., Gallego, P. P. (2021). The metabolomics reveals intraspecies variability of bioactive compounds in elicited suspension cell cultures of three Bryophyllum species. Ind. Crops Prod. 163, 113322. doi: 10.1016/j.indcrop.2021.113322
Georges, D., Chenieux, J. C., Ochatt, S. J. (1993). Plant regeneration from aged-callus of the woody ornamental species Lonicera japonica cv. “Hall’s Prolific. Plant Cell Rep. 13, 91–94. doi: 10.1007/BF00235297
Gu, X. P., Yang, L. L., Qi, D. M., Zhang, D., Liu, T. L., Dong, C. M. (2024). Regulation of gene expression in the secondary metabolic synthesis pathway of Lonicera japonica Flos by yeast polysaccharides. Physiol. Mol. Biol. Plants 30, 1071–1084 -. doi: 10.1007/s12298-024-01482-1
Haberlandt, G. (1902). Kulturversuche mit isolierten Pflanzenzellen. Sitzungsber K Preuss Akad Wiss Wien Math-Naturwiss 111, 69–92. doi: 10.1038/152126a0
Hatami, M., Naghdi Badi, H., Ghorbanpour, M. (2019). Nano-elicitation of secondary pharmaceutical metabolites in plant cells: A review. J. Med. Plants 18, 6–36. doi: 10.29252/jmp.3.71.6
He, L., Xu, X. L., Li., Y., Li, C. F., Zhu, Y. J., Yan, H. X., et al. (2013). Transcriptome analysis of buds and leaves using 454 pyrosequencing to discover genes associated with the biosynthesis of active ingredients in Lonicera japonica Thunb. PloS One 8, e62922. doi: 10.1371/journal.pone.0062922
He, M. Y., Yang, Y., Chen, Z. Y. (2022). Study on rapid propagation of Lonicera japonica and measurement of total flavonoids content. Hunan Agric. Sci. 1, 38–41. doi: 10.16498/j.cnki.hnnykx.2022.001.011
He, G., Zhou, Z. K. (2008). A study of hairy roots culture of Flos Lonicerae induced by Agrobacterium rhizogenes Ri. J. Cent. South Univ. Forest. Technol. 28, 77–80.
Heng, J., Han, C. Y., Wang, M. S., Ma, L. (2018). Induction of Callus of Stem Segments of Lonicera japonica Thunb. var. chinensis (Wats.) Bak. Northern Horticult. 19), 94–99.
Horiike, T., Ohshiro, M. (1997). Biotransformation of the germacrane type sesquiterpene curdione by suspension cultured cells of Lonicera japonica. Phytochemistry 44, 627–632. doi: 10.1016/S0031-9422(96)00569-9
Hosseini, R., Mulligan, B. (2002). Application of rice (Oryza Sativa L.) suspension culture in studying senescence in vitro (I) single strand preferring nuclease activity. Electron. J. Biotechnol. 5, 42–54. doi: 10.2225/vol5-issue1-fulltext-8
Hsu, P. S., Wang, H. J. (1988). “Lonicera,” in Flora Reipublicae Popularis Sinicae, vol. 72. (Science Press, Beijing), 153–257.
Hu, Z. J. (2016). Preliminary Establishment of Cell Suspension System of Lonicera japonica Thunb and Study on the Inhibition of Nitrosation by Chlorogenic Acid (Jiangxi Agricultural University). doi: 10.27177/d.cnki.gjxnu.2016.000023
Hu, M., Hu, Z., Du, L., Du, J., Luo, Q., Xiong, J. (2019). Establishment of cell suspension culture of Lonicera japonica Thunb and analysis its major secondary metabolites. Ind. Crops Prod. 137, 98–104. doi: 10.1016/j.indcrop.2019.05.024
Hu, Z. J., Luo, Q. S., Li, D. M., Du, H. Y., Du, J., Xiong, J. H. (2016). A study on induction of the Lonicera japonica Thunb callus and its chlorogenic acid content. Acta Agricult. Universitatis Jiangxiensis 38, 549–556. doi: 10.13836/j.jjau.2016079
Hu, L. F., Yu, L., Weng, Y. H., Qu, H. X., Yang, D. J., Lu, Y., et al. (2024). Combined non-targeted metabolomic and transcriptomic analysis explains the biosynthetic pathway and differential accumulation of chlorogenic acid in the Liriodendron petal. Sci. Hortic. 328, 112791. doi: 10.1016/j.scienta.2023.112791
Hussain, M. J., Abbas, Y., Nazli, N., Fatima, S., Drouet, S., Hano, C., et al. (2022). Root cultures, a boon for the production of valuable compounds: A comparative review. Plants 11, 439. doi: 10.3390/plants11030439
Jacobs, B., Lens, F., Smets, E. (2009). Evolution of fruit and seed characters in the Diervilla and Lonicera clades (Caprifoliaceae, Dipsacales). Ann. Bot. 104, 253–276. doi: 10.1093/aob/mcp131
Jia, Z. Q., Yan, J. (2024). Screening of fungicides to control powdery mildew on honeysuckle. Agrochemicals 63, 446–450. doi: 10.16820/j.nyzz.2024.0610
Jiang, C., Wu, S., Feng, X., Yang, C., Yu, Z. (2021). The complete chloroplast genome of Lonicera pampaninii Levl. and its phylogenetic analysis. Mitochondrial DNA Part B 6, 3025–3027. doi: 10.1080/23802359.2021.1978891
Kim, S. W., Oh, S. C., In, D. S., Liu, J. R. (2003). High frequency somatic embryogenesis and plant regeneration in zygotic embryo cultures of Japanese honeysuckle. Plant Cell Tissue Organ Cult. 72, 277–280. doi: 10.1023/A:1022360115275
Kobayashi, Y., Kärkkäinen, Y., Häkkinen, S. T., Nohynek, L., Ritala, A., Rischer, H., et al. (2021). Life cycle assessment of plant cell cultures. Sci. Total Environ. 808, 151990. doi: 10.1016/j.scitotenv.2021.151990
Kong, E. Y. Y., Biddle, J., Foale, M., Panis, B., Adkins, S. W. (2021). The potential to propagate coconut clones through direct shoot organogenesis: A review. Sci. Hortic. 289, 110400. doi: 10.1016/j.scienta.2021.110400
Kundu, S., Salma, U., Ali, M. N., Hazra, A. K., Mandal, N. (2018). Development of transgenic hairy roots and augmentation of secondary metabolites by precursor feeding in Sphagneticola calendulacea (L.) Pruski. Ind. Crops Prod. 121, 206–215. doi: 10.1016/j.indcrop.2018.05.009
Lai, K. H., Chen, Y. L., Lin, M. F., El-Shazly, M., Chang, Y. C., Chen, P. J. (2022). Lonicerae japonicae Flos attenuates neutrophilic inflammation by inhibiting oxidative stress. Antioxidants 11, (9). doi: 10.3390/antiox11091781
Li, D. M. (2018). Study on Exploration the Elicitor and Key Enzyme Genes for Enhancement Accumulation of Chlorogenic Acids in Lonicera japonica Thunb, Suspension Cells (Jiangxi Agricultural University). doi: 10.27177/d.cnki.gjxnu.000064
Li, F. M., Chen, L. X., Li, J. J., Zhang, M., Qi, Y. T., Xu, X., et al. (2024a). Identification and bioinformatics analysis of gene for chlorogenic acid in Lonicera japonica Thunb. Mol. Plant Breed 22, 7389–7399. doi: 10.13271/j.mpb.022.007389
Li, Y., Kim, J. I., Pysh, L., Chapple, C. (2015). Four isoforms of Arabidopsis 4-coumarate: CoA ligase have overlapping yet distinct roles in phenylpropanoid metabolism. Plant Physiol. 169, 2409–2421. doi: 10.1104/pp.15.00838
Li, Y. Q., Kong, D. X., Bai, M., He, H. J., Wang, H. Y., Wu, H. (2019). Correlation of the temporal and spatial expression patterns of HQT with the biosynthesis and accumulation of chlorogenic acid in Lonicera japonica flowers. Hortic. Res. 6, 73. doi: 10.1038/s41438-019-0154-2
Li, Y. Q., Kong, D. X., Fu, Y., Sussman, M. R., Wu, H. (2020). The effect of developmental and environmental factors on secondary metabolites in medicinal plants. Plant Physiol. Biochem. 148, 80–89. doi: 10.1016/j.plaphy.2020.01.006
Li, G. J., Li, Y. X., Lu, H. Y., Zhang, Y. Y., Zhou, Z. J. (2012). Research on tissue culture technology of Russian Lonicera caerulea. Forest. Sci. Technol. 37, 4–6.
Li, Z., Nair, S. K. (2015). Structural basis for specificity and flexibility in a plant 4-coumarate: CoA ligase. Structure 23, 2032–2042. doi: 10.1016/j.str.2015.08.012
Li, J. G., Sun, M. Z. (2004). Research on the tissue culture and rapid propagation technology of high-quality Lonicera. japonica varieties. J. Shandong Forest. Sci. Technol. 6), 36–37.
Li, Q., Tang, M., Tan, Y. Y., Ma, D. W., Wang, Y. N., Zhang, H. (2016). Improved production of chlorogenic acid from cell suspension cultures of Lonicera macranthoids. Trop. J. Pharm. Res. 15, 919. doi: 10.4314/tjpr.v15i5.4
Li, W., Zhang, L., He, P., Li, H., Pan, X., Zhang, W., et al. (2024b). Traditional uses, botany, phytochemistry, and pharmacology of Lonicerae japonicae flos and Lonicerae flos: A systematic comparative review. J. Ethnopharmacol 322, 117278. doi: 10.1016/j.jep.2023.117278
Liu, W. Y. (2010). Study on in virtio culture of Yu-Producing authentic chinese herbal medicines Lonicera japonica Thunb (Henan Normal University).
Liu, A. C., Bi, Y., Yu, Q., Wang, H., Wang, Q., Li, Y. (2022). Research progress on breeding of Lonicera linn. in China. Northern Horticult. 5), 133–139.
Liu, Z., Cheng, Y., Chao, Z. A. (2023). Comprehensive quality analysis of different colors of medicinal and edible honeysuckle. Foods 12, 3126. doi: 10.3390/foods12163126
Liu, Z., Cheng, Z., He, Q., Lin, B., Gao, P., Li, L. (2016). Secondary metabolites from the flower buds of Lonicera japonica and their in vitro anti-diabetic activities. Fitoterapia 110, 44–51. doi: 10.1016/j.fitote.2016.02.011
Liu, Y., Guo, M. Y., Wang, C. Y., Bai, G. M. (2012a). Researches on isolation conditions of protoplasts of Lonicera japonica. Chin. J. Exp. Tradit. Med. Formulae 19, 125–129. doi: 10.13422/j.cnki.syfjx.2012.19.041
Liu, Y., Peng, X. X., Zhu, S. S., Bai, G. B. (2012c). Researches on the relative expression of HQT gene in different organs of Lonicera japonica. J. Chin. Med. Mater 35, 1032–1036. doi: 10.13863/j.issn1001-4454.2012.07.005
Liu, L. F., Qian, G. Z. (2007). The inducement and differentiation of Calli in tissue culture of Lonicera japonica Thunb. J. Liaocheng Univ. (Natural Sci. Edition). 2007, 48–50+107.
Liu, Y. Q., Wu, L., Chen, Z. X., Zhu, F. F. (2012b). In vitro Induction of Polyploidy of Lonicera japonica by Colchicine and Its Ploidy Identification. J. Chin. Medicinal Materials 35, 1729–1732. doi: 10.13863/j.issn1001-4454.2012.11.007
Ludwig, A. A., Romeis, T., Jones, J. D. G. (2004). CDPK-mediated signalling pathways: specificity and cross-talk. J. Exp. Bot. 55, 181–188. doi: 10.1093/jxb/erh008
Luo, Y., Zhu, P. P., Liao, Y. (2018). Bioinformatics analysis of LjHQT gene in Lonicera japonica. Mol. Plant Breed 16, 1403–1408. doi: 10.13271/j.mpb.016.001403
Malik, S., Cusidó, R. M., Mirjalili, M. H., Moyano, E., Palazón, Z., Bonfill, M. (2011). Production of the anticancer drug taxol in Taxus baccata suspension cultures: A review. Process Biochem. 46, 23–34. doi: 10.1016/j.procbio.2010.09.004
Mao, X. F., Hen, J. J., Han, Z. K., Wu, F. F. (2019). Effects of lead stress on growth and physiological metabolism and accumu⁃lation characteristics of Lonicera japonica. J. Northeast Agric. Sci. 44, 69–75. doi: 10.16423/j.cnki.1003-8701.2019.05.016
Menges, M., Hennig, L., Gruissem, W., Murray, J. A. (2003). Genome-wide gene expression in an Arabidopsis cell suspension. Plant Mol. Biol. 53, 423–442. doi: 10.1023/B:PLAN.0000019059.56489.ca
Meyer, A. J., Fricker, M. D. (2002). Control of demand-driven biosynthesis of glutathione in green Arabidopsis suspension culture cells. Plant Physiol. 130, 1927–1937. doi: 10.1104/pp.008243
Mishra, A. K., Sharma, K., Misra, R. S. (2012). Elicitor recognition, signal transduction and induced resistance in plants. J. Plant Interact. 7, 95–120. doi: 10.1080/17429145.2011.597517
Moniruzzaman, M., Zhong, Y., Huang, Z., Yan, H., Yuanda, L., Jiang, B., et al. (2021). Citrus cell suspension culture establishment, maintenance, efficient transformation and regeneration to complete transgenic plant. Plants 10, 664. doi: 10.3390/plants10040664
Moscatiello, R., Baldan, B., Navazio, L. (2013). Plant cell suspension cultures. Methods Mol. Biol. 953, 77–93. doi: 10.1007/978-1-62703-152-3_5
Narayani, M., Srivastava, S. (2017). Elicitation: a stimulation of stress in in vitro plant cell/tissue cultures for enhancement of secondary metabolite production. Phytochem. Rev. 16, 1227–1252. doi: 10.1007/s11101-017-9534-0
Niggeweg, R., Michael, A. J., Martin, C. (2004). Engineering plants with increased levels of the antioxidant chlorogenic acid. Nat. Biotechnol. 22, 746–754. doi: 10.1038/nbt966
Ning, W. (2012). “Studies on intervention in secondary metabolites in Lonicera japonica Thunb. and Mahonia bealei (Fort.) Carr,” in Response to the technology of ultraviolet induction (Shandong University of Traditional Chinese Medicine).
Ochatt, S. J. (1991). Requirements for plant regeneration from protoplasts of the shrubby ornamental honeysuckle, Lonicera nitida cv. Maigrun. Plant Cell Tiss Organ Cult. 25, 161–167. doi: 10.1007/BF00042188
Owis, A. I., Abdelwahab, N. S., Abul-Soad, A. A. (2016). Elicitation of phenolics from the micropropagated endangered medicinal plant Calligonum polygonoides L. (Polygonoaceae). Pharmacogn Mag 12, 465–470. doi: 10.4103/0973-1296.191458
Pan, Y., Zhao, X., Wu, X. L., Wang, Y., Tan, J., Chen, D. X. (2021b). Transcriptomic and metabolomic analyses provide insights into the biosynthesis of chlorogenic acids in Lonicera macranthoides Hand.-Mazz. PloS One 16, e0251390. doi: 10.1371/journal.pone.0251390
Pan, H. Q., Zhou, H., Miao, S., Guo., D. A., Li, Z. X., Hu, Q. (2021a). Plant metabolomics for studying the effect of two insecticides on comprehensive constituents of Lonicerae japonicae flos. Chin. J. Natural Medicines 19, 70–80. doi: 10.1016/S1875-5364(21)60008-0
Park, H. B., Ko, C. H., Lee, S. Y., Kim, S. Y., Lee, K. C. (2019). Ecophysiology of seed dormancy and germination in four Lonicera (caprifoliaceae) species native to Korea. J. Ecol. Environ. 43, 25. doi: 10.1186/s41610-019-0121-8
Petrova, M., Miladinova-Georgieva, K., Geneva, M. (2024). Influence of abiotic and biotic elicitors on organogenesis, biomass accumulation, and production of key secondary metabolites in asteraceae plants. Int. J. Mol. Sci. 25, 4197. doi: 10.3390/ijms25084197
Popova, E., Titova, M., Tynykulov, M., Zakirova, R. P., Kulichenko, I., Prudnikova, O., et al. (2023). Sustainable production of Ajuga bioactive metabolites using cell culture technologies: A review. Nutrients 15, 1246. doi: 10.3390/nu15051246
Qiu, J., Tan, X. F. (2005). Tissue culture and rapid propagation of Lonicera japonica. J. Cent. South Univ. Forest. Technolog 4, 53–56.
Ren, M., Wu, Y. C., Zhang, J. Y., Zhao, Z. Y. (2015). Induction of Lonicera japonica callus. Jiangsu Agric. Sci. 43, 38–40. doi: 10.15889/j.issn.1002-1302.2015.03.012
Schatlowski, N., Köhler, C. (2012). Tearing down barriers: understanding the molecular mechanisms of interploidy hybridizations. J. Exp. Bot. 63, 6059–6067. doi: 10.1093/jxb/ers288
Seidel, T., Artmann, P. J., Gkekas, I., Illies, F., Baack, A. L., Viefhues, M. (2024). Microfluidic single-cell study on Arabidopsis thaliana protoplast fusion—New insights on timescales and reversibilities. Plants 13, 295. doi: 10.3390/plants13020295
Shabala, S., Pottosin, I. (2014). Regulation of potassium transport in plants under hostile conditions: implications for abiotic and biotic stress tolerance. Physiol. Plant 151, 257–279. doi: 10.1111/ppl.12165
Shan, G. F., Zhang, X. L., Li, M. J., Chen, M. X., Liu, W. Y., Lu, J. (2013). Study on callus induction and subculture of Lonicera japonica Thunb. Northern Horticult. 5), 106–109.
Shang, X., Pan, H., Li, M., Miao, X., Ding, H. (2011). Lonicera japonica Thunb.: Ethnopharmacology, phytochemistry and pharmacology of an important traditional Chinese medicine. J. Ethnopharmacol 138, 1–21. doi: 10.1016/j.jep.2011.08.016
Sharma, P., Padh, H., Shrivastava, N. (2013). Hairy root cultures: A suitable biological system for studying secondary metabolic pathways in plants. Eng. Life Sci. 13, 62–75. doi: 10.1002/elsc.201200030
Shasmita, Singh, N. R., Rath, S. K., Behera, S., Naik, S. K. (2018). “In vitro secondary metabolite production through fungal elicitation: An Approach for sustainability,” in Fungal Nanobionics: Principles and Applications. Eds. Prasad, R., Kumar, V., Kumar, M., Wang, S. (Springer Singapore, Singapore), 215–242. doi: 10.1007/978-981-10-8666-3_9
Shi, S., Tian, L., Ma, L., Tian, C. (2018). Community structure of rhizomicrobiomes in four medicinal herbs and its implication on growth management. Microbiol 87, 425–436. doi: 10.1134/S0026261718030098
Song, G. Y., Liang, H. (1987). Studies on Lonicera japonica tissue culture and its secondary metabolites. Chin. Pharm. J. 10, 588–589. doi: CNKI:SUN:ZGYX.0.1987-10-004
Su, X., Zhu, Z., Zhang, L., Wang, Q., Xu, M., Lu, C. (2021). Anti-inflammatory property and functional substances of Lonicerae Japonicae Caulis. J. Ethnopharmacol 267, 113502. doi: 10.1016/j.jep.2020.113502
Sun, Y. C., Lin, S. F., Huang, L. Q., Zhang, X. B., Guo, L. P. (2011). Review: Autotoxic effects of medicinal plants and control measures. China J. Chin. Materia Med. 36, 387–390.
Sun, Q. H., Morales-Briones, D. F., Wang, H. X., Landis, J. B., Wen, J., Wang, H. F. (2023). Target sequence capture data shed light on the deeper evolutionary relationships of subgenus Chamaecerasus in Lonicera (Caprifoliaceae). Mol. Phylogenet. Evol. 184, 107808. doi: 10.1016/j.ympev.2023.107808
Tang, N., Cao, Z. Y., Yang, C., Ran, D. S., Wu, P. Y., Gao, H. M., et al. (2021). A R2R3-MYB transcriptional activator LmMYB15 regulates chlorogenic acid biosynthesis and phenylpropanoid metabolism in Lonicera macranthoides. Plant Sci. 308, 110924. doi: 10.1016/j.plantsci.2021.110924
Tao, Y. (2023). Effects of Cadmium and Ozone on Physicochemical Properties and Properties of Lonicera japonica Thunb Transcriptome analysis Vol. 2023 (Shenyang Agricultural University). doi: 10.27327/d.cnki.gshnu.2023.001007
Thakur, M., Bhattacharya, S., Kumar Khosla, P., Puri, S. (2020). Improving production of plant secondary metabolites through biotic and abiotic elicitation. J. Appl. Res. Med. Aromat. Plants 12, 1–12. doi: 10.1016/j.jarmap.2018.11.004
Tomasiak, A., Zhou, M., Betekhtin, A. (2022). Buckwheat in tissue culture research: current status and future perspectives. Int. J. Mol. Sci. 23, 2298. doi: 10.3390/ijms23042298
Valiñas, M. A., Lanteri, M. L., Have, T. A., Andreu, A. B. (2015). Chlorogenic acid biosynthesis appears linked with suberin production in potato Tuber(Solanum tuberosum). J. Agric. Food Chem. 63, 4902–4913. doi: 10.1021/jf505777p
van Berkum, N. L., Lieberman-Aiden, E., Williams, L., Imakaev, M., Gnirke, A., Mirny, L. A., et al. (2010). Hi-C: a method to study the three-dimensional architecture of genomes. J. Vis. Exp. 39), e1869. doi: 10.3791/1869-v
Vasil, V., Hildebrandt, A. C. (1965). Differentiation of tobacco plants from single, isolated cells in microcultures. Science 150, 889–892. doi: 10.1126/science.150.3698.889
Wang, N., Chen, H., Gao, Q., Qian, J. (2022). Establishment of an efficient cell suspension culture system for Lonicera japonica Thunb. Pak. J. Bot. 54, 2167–2172. doi: 10.30848/PJB2022-6(7
Wang, W. J., Wang, P., Li, W. Q. (2013). Study on the Tissue Culture and Rapid Propagation of Lonicera japonica var. chinensis. Northern Horticult. 18), 100–102.
Wang, H. L., Zhao, X. M. (2012). Study on induction and morphological identification of autotertraploid in Lonicera japonica Thunb. J. Shanxi Agric. Sci. 40, 1240–1242,1253.
Wang, H. L., Zhao, X. M. (2014). Cytology identification of tetraploid Lonicera japonica Thunb. Northern Horticult. 6), 92–94.
Wei, X. M., Hu, Y., Wei, K. H., Wu, Q. H., Huang, Y., Wei, F. (2021). Characterization of the complete chloroplast genome of Lonicera similis (Caprifoliaceae). Mitochondrial DNA Part B 6, 3067–3069. doi: 10.1080/23802359.2021.1981161
White, P. R. (1934). Potentially unlimited growth of excised tomato root tips in a liquid medium. Plant Physiol. 9, 585–600. doi: 10.1104/pp.9.3.585
Wu, L. N. (2012). Study on the loose callus induction and cell suspension culture of Lonicera japonica. Northern Horticult. 20), 107–109.
Wu, X. Q., Cui, Z. T., Li, X. Y., Yu, Z. H., Li, P. P., Xue, L., et al. (2024). Identification and characterization of PAL genes involved in the regulation of stem development in saccharum spontaneum L. BMC Genom Data 25, 38–38. doi: 10.1186/s12863-024-01219-9
Wu, M. L., Sun, X. L., Zhu, S. S., Li, W. D., Bai, G. B. (2014). Correlation analysis of HQT gene mutation and chlorogenic acid content of Flos Lonicerae. Jilin J. Chin. Med. 34, 728–731. doi: 10.13463/j.cnki.jlzyy.2014.07.026
Wu, X. L., Zhang, S. Z., Li, X., Zhang, F., Fan, Y. J., Liu, Q. L., et al. (2021). Postharvest UV-B radiation increases enzyme activity, polysaccharide and secondary metabolites in honeysuckle (Lonicera japonica Thunb.). Ind. Crops Prod. 171, 113907. doi: 10.1016/j.indcrop.2021.113907
Xiang, Z. X., Gao, S. L. (2007). Establishment and optimisation of tissue culture system for Lonicera japonica. China J. Chin. Materia Med. 2007, 2662–2663.
Xing, Y. X. (2006). Research on the breeding of a new tetraploid Lonicera japonica variety ‘Jiufeng no. 1’. Agricultire Eng. Technol. 1), 44–46.
Xu, F., Valappil, A. K., Mathiyalagan, R., Tran, T. N. A., Ramadhania, Z. M., Awais, M., et al. (2023). In vitro cultivation and ginsenosides accumulation in Panax ginseng: A review. Plants 12, 3165. doi: 10.3390/plants12173165
Xu, M. L., Wu, J. H., Min, L. X. (2011). Tissue culture and rapid propagation of Lonicerra tellmanniana Spaeth. J. Anhui Agri 39, 13977–13978. doi: 10.13989/j.cnki.0517-6611.2011.23.170
Xun, Y. L. (2004). A preliminary study of tissue culture and rapid propagation of Lonicera japonica Champ. J. Hechi Univ. 24, 32–34.
Yamamoto, H., Katano, N., Ooi, A., Inoue, K. (1999). Transformation of loganin and 7-deoxyloganin into secologanin by Lonicera japonica cell suspension cultures. Phylochemistry 50, 417–422. doi: 10.1016/S0031-9422(98)00613-X
Yamaner, Ö., Erdağ, B., Gökbulut, C. (2013). Stimulation of the production of hypericins in in vitro seedlings of Hypericum adenotrichum by some biotic elicitors. Turk J. Bot. 37, 153–159. doi: 10.3906/bot-1202-1
Yan, K., Zhao, S., Bian, L., Chen, X. (2017). Saline stress enhanced accumulation of leaf phenolics in honeysuckle (Lonicera japonica Thunb.) without induction of oxidative stress. Plant Physiol. Biochem. 112, 326–334. doi: 10.1016/j.plaphy.2017.01.020
Yang, P. J., Chen, D. J., Zhao, Y., Li, H. N. (2003). Tissue culture and rapid propagation of Lonicera japonica. Acta Botanica Boreali-Occidentalia Sin. 7), 248–251.
Yang, R., Fang, L., Li, J., Zhang, Y. Q. (2019a). A new anti-inflammatory lignan from Lonicerae Japonicae flos. Nat. Prod. Res. 35, 587–592. doi: 10.1080/14786419.2019.1587430
Yang, R., Fang, L., Li, J., Zhao, Z., Zhang, H., Zhang, Y., et al. (2019b). Separation of five iridoid glycosides from Lonicerae japonicae Flos using high-speed counter-current chromatography and their anti-inflammatory and antibacterial activities. Molecules 24, 197. doi: 10.3390/molecules24010197
Yang, C., Jiang, C., Wu, S., Feng, X., Yu, Z. (2022). The complete chloroplast genome of Lonicera acuminata Wall. and its phylogenetic analysis. Mitochondrial DNA Part B 7, 807–809. doi: 10.1080/23802359.2022.2073836
Yang, D. Y., Zhang, L. Z., Li, X. P. (2017). High effect regeneration system establishment of Lonicera japonica Thunb. Mol. Plant Breed. 15, 1461–1465. doi: 10.13271/j.mpb.015.001461
Yang, Y. H., Zhang, Z. Y., Li, R. F., Yi, Y. J., Yang, H., Wang, C. J., et al. (2020). RgC3H involves in the biosynthesis of allelopathic phenolic acids and alters their release amount in Rehmannia glutinosa roots. Plants 9, 567. doi: 10.3390/plants9050567
Yin, J. Y., Lai, M., Yu, X. Y., Su, D. D., Xiong, X. Y., Li, Y. L. (2024). Comprehensive strategies for paclitaxel production: insights from plant cell culture, endophytic microorganisms, and synthetic biology. Hortic. Res. 346, 1–12. doi: 10.1093/hr/uhae346
Yuan, J., He, G. (2007). Preliminary study on induction of hairy roots in Lonicera macranthoides. Non-wood For. Res. 1), 55–58.
Zagorskaya, A. A., Deineko, E. V. (2017). Suspension-cultured plant cells as a platform for obtaining recombinant proteins. Russ J. Plant Physiol. 64, 795–807. doi: 10.1134/S102144371705017X
Zhang, H. C., Gao, S. L., Xue, X. (2011). Determination of chlorogenic acid content and selection of allotetraploid plants of Lonicera japonica. Pharm. Biotechnol. 18, 242–245.
Zhang, B. X., Lewis, K. M., Abril, A., Davydov, D. R., Vermerris, W., Sattler, S. E., et al. (2020). Structure and function of the cytochrome P450 monooxygenase cinnamate 4-hydroxylase from Sorghum bicolor. Plant Physiol. 183, 957–973. doi: 10.1104/pp.20.00406
Zhang, M., Li, M., Fu, H., Wang, K., Tian, X., Qiu., R., et al. (2022a). Transcriptomic analysis unravels the molecular response of Lonicera japonica leaves to chilling stress. Front. Plant Sci. 13. doi: 10.3389/fpls.2022.1092857
Zhang, S. W., Liu, S. T., Hou, A. J., Wang, S., Na, Y. X., Hu, J. H., et al. (2022b). Systematic review of Lonicerae Japonicae Flos: A significant food and traditional Chinese medicine. Front. Pharmacol. 19. doi: 10.3389/fphar.2022.1013992
Zhang, J. R., Wu, M. L., Li, W. D., Bai, G. B. (2016). Regulation of honeysuckle HQT gene to chlorogenic acid biosynthesis in eukaryotic plant cells of Lonicerae Flos. Chin. Tradit. Herb Drugs 47, 3683–3687.
Zhang, B., Zheng, L. P., Wang, J. W. (2012). Nitric oxide elicitation for secondary metabolite production in cultured plant cells. Appl. Microbiol. Biotechnol. 93, 455–466. doi: 10.1007/s00253-011-3658-8
Zhao, J., Davis, L. C., Verpoorte, R. (2005). Elicitor signal transduction leading to production of plant secondary metabolites. Biotechnol. Advances: Int. Rev. J. 4), 23. doi: 10.1016/j.bioteChadv.2005.01.003
Zhao, Y., Dou, D. Q., Guo, Y. Q., Qi, Y., Li, J., Jia, D. (2018). Comparison of the Trace Elements and Active Components of Lonicera japonica flos and Lonicera flos Using ICP-MS and HPLC-PDA. Biol. Trace Elem Res. 183, 379–388. doi: 10.1007/s12011-017-1138-4
Zhao, Z. C., Gao, A. P., Luo, R. X., Liu, K. L., Yu, K. L. (2022). The different deletion mutation in the phenylalanine ammonia-lyase(PAL)gene affects the peel color of mango (Mangifera indica L.). Genet. Resour Crop Evol. 69, 2301–2306. doi: 10.1007/s10722-022-01393-8
Keywords: L. japonica, tissue culture, chlorogenic acid, elicitors, synthetic biology
Citation: Cheng J, Chen Y, Guo F, Dong P, Zhou C, Liang W and Wang H (2025) Regulatory mechanisms and biosynthesis of chlorogenic acid in Lonicera japonica: insights from tissue culture and inducer treatments. Front. Plant Sci. 16:1567140. doi: 10.3389/fpls.2025.1567140
Received: 26 January 2025; Accepted: 25 March 2025;
Published: 24 April 2025.
Edited by:
Zishan Ahmad, Nanjing Forestry University, ChinaReviewed by:
Pawan Kumar, Agricultural Research Organization (ARO), IsraelMohammad Aqa Mohammadi, Chinese Academy of Sciences (CAS), China
Copyright © 2025 Cheng, Chen, Guo, Dong, Zhou, Liang and Wang. This is an open-access article distributed under the terms of the Creative Commons Attribution License (CC BY). The use, distribution or reproduction in other forums is permitted, provided the original author(s) and the copyright owner(s) are credited and that the original publication in this journal is cited, in accordance with accepted academic practice. No use, distribution or reproduction is permitted which does not comply with these terms.
*Correspondence: Yuan Chen, Y2hlbnl1YW5AZ3NhdS5lZHUuY24=; Fengxia Guo, Z3VvZnhAZ3NhdS5lZHUuY24uY29t