- 1Department of Plant Science, Federal University of Santa Catarina, Florianopolis, Brazil
- 2Department of Chemical and Biological Sciences, São Paulo State University, Botucatu, Brazil
- 3Department of Biotechnology, University of Caxias do Sul, Caxias do Sul, Brazil
The increasing frequency of adverse environmental events, driven by ongoing climate change, has intensified the search for new technological alternatives in crop production and plant protection. Thermal stress can limit plant adaptation and negatively impact metabolism, physiology, morphology, and yield. Cold stress in plants has been extensively studied and can affect various stages of plant’s life cycle, from seed formation to development, causing damage to cell membranes, impairing cell division, and disrupting water absorption. Consequently, researchers have focused on mitigating the impacts of abiotic stress by investigating bioactive molecules and biostimulants derived from various organisms, which enhance tolerance mechanisms in plants. In aquatic environments, macro- and microalgae have emerged as key sources of plant elicitors, providing extractable molecules such as polysaccharides, polyamines, polyphenols, and amino acids that enhance plant defense responses. Similarly, certain terrestrial plants have shown potential as sources of biostimulant compounds. Thus, this study aims to highlight advancements in crop systems by emphasizing the potential of algae-based and terrestrial biostimulant elicitors in enhancing tolerance to cold stress. Ultimately, the goal is to improve understanding of promising biological models for food production, fostering innovative developments that can contribute to economically and ecologically sustainable technologies.
1 Introduction
The search for new bioactive molecules as a solution to reduce agrochemicals in agriculture has led to countless experiments focusing on genetic resources, either from terrestrial or marine environments. In this context, extracts and molecules derived from plants and algae have proven effective as elicitors of plant development and health, enhancing root growth, flowering, and tolerance to adverse environmental conditions (Irani et al., 2021; Ziogas et al., 2022; Riyazuddin et al., 2023).
Bioprospecting approaches are considered sustainable and innovative alternatives to mitigate the harmful effects of climate events on plants of agricultural importance. These approaches range from monitoring methods (Tewari et al., 2017) to interventions in the microbiota, such as the use of bioinoculants developed from organisms native to frozen soils (Pandey and Yarzábal, 2019) and those from desert soils (Zhang and White, 2021).
Particularly in marine environments, microalgae and macroalgae have been identified as potential sources of biofertilizers and biostimulants for plants. They contribute to nutrient transport, plant protection, and growth, besides serving as nutritional additives or elicitors for tolerance induction through bioactive molecules. These include polysaccharides such as ulvans, fucans, alginates, and carrageenans, as well as phytohormones, betaines, polyamines, and amino acids. Compilations by Bello et al. (2021), Poveda and Díez-Méndez (2023), and Di Sario et al. (2025) have highlighted that algal biomass and their constituents have been extensively studied for applications in agriculture (Potijun et al., 2021; Cvetkovska et al., 2022). Algal species from all divisions, including Chlorophyta (green), Rhodophyta (red), and Phaeophyta (brown), have been used for mitigate the impacts of abiotic stress in plants. The results from foliar and soil applications have shown, for instance, increased root emission and shoot growth, along with enhanced defense responses and plant tolerance to (a)biotic stress factors (Sharma et al., 2014; Xu and Leskovar, 2015; Ali et al., 2021; Munaro et al., 2021; Nunes et al., 2024).
In addition to algae, many terrestrial plant species contain bioactive molecules that may benefit cultivated plants. Numerous studies support these findings (Matsumiya and Kubo, 2011; Hayat et al., 2018; Meena et al., 2019; Pacifico et al., 2021; Souza et al., 2021). Plant extracts and oils can serve as additives, biofertilizers, biostimulants, biopesticides, and protectors of crop species (Puglia et al., 2021; Khursheed et al., 2022). The market for these biomolecules has been steadily expanding, driven by farmers’ recognition of their effectiveness in low-carbon food production systems (Rouphael and Colla, 2020; Rakkammal et al., 2023).
Given the potential of algae and plant-based biostimulant compounds, research has focused on developing new technologies to enhance plant tolerance to abiotic stresses, such as water deficit, salinity, and thermal stress – common climatic events that pose significant challenges and lead to major production losses (Bulgari et al., 2019; Deolu-Ajayi et al., 2022; Rakkammal et al., 2023). Among these, cold stress is particularly notable for causing substantial losses, both in the field and post-harvest (Gavelienė et al., 2014; Puzanskiy et al., 2018). However, studies addressing this issue remain limited, especially regarding its impact on global agricultural production (Mahajan and Tuteja, 2005). Thus, this review aims to present and critically discuss the findings of peer-reviewed articles and books that have described and confirmed the effectiveness of seaweed-based and plant biostimulants in mitigating cold stress in various crops.
2 Information collection
For the integrative review, several databases were searched, including the Portal de Periódicos Capes, Web of Science, Scopus, and SciELO. This choice was made to ensure a comprehensive collection of information on the use of biostimulants to enhance cold stress tolerance in plants. Additionally, no time filters were applied, however, the selection was limited to peer-reviewed articles or books. This strategy allowed us to expand the search and avoid limitations that could restrict access to relevant information. It is important to note that the specific literature on the use of biostimulants in the context of interest is still relatively scarce, which justifies the need for a broader approach to data collection.
3 Biostimulants – definition, market, and legislation
Biostimulants are products used in agriculture that can be applied to plants and soil and are capable of regulating and/or enhancing physiological processes in crops (du Jardin, 2015; Franzoni et al., 2022). Considered environmentally safe and cost-effective alternatives to traditional fertilizers and chemical treatments, they minimize negative impacts on ecosystems while promoting sustainable agricultural practices. They influence plant biochemistry through various metabolic pathways, enhancing morphological yields, increasing quality and shelf life, aiding in the absorption of essential nutrients, and boosting tolerance to abiotic stresses (Ronga et al., 2019).
In industry, the definition of biostimulant was originally proposed in 2012, highlighting that these are plant-based products containing compounds and/or microorganisms that stimulate natural processes when applied to plants and the rhizosphere. Importantly, they do not fit into the regulatory framework for pesticides, as they do not have a direct action against pests (van Oosten et al., 2017). According to du Jardin (2015), a plant biostimulant refers to any substance or microorganism applied to plants to improve nutritional efficiency, enhance tolerance to abiotic stress, and/or improve crop quality characteristics, irrespective of its nutrient content.
Apparently, the term was coined by horticultural experts to describe compounds that promote plant growth without being defined as nutrients, soil amendments, or pesticides (du Jardin, 2015). However, the discussion on this topic can be traced back to 1933, when Professor Filatov in the Soviet Union introduced the concept of biogenic stimulants. Filatov suggested that biological materials from various organisms, including stressed plants, could influence metabolic and energy processes in plant, animal, and even human cells. Other contributions to the discussion came from Blagoveshchensky in 1945, when he described that biogenic stimulants, such as organic acids, would have a stimulating effect due to their dibasic properties, which can increase enzymatic activity in plants. Filatov, however, continued to expand the discussion by not limiting it to organic acids (Yakhin et al., 2017).
In the historical context, Hervé, in 1994, suggested that the development of new “biorational products” should be grounded in chemical, biochemical, and biotechnological principles. These products should be applied in low dosages, be environmentally friendly, and provide reproducible benefits in the cultivation of agricultural species (Yakhin et al., 2017). Supporting this view, Zhang and Schmidt (2000) emphasized the importance of conducting comprehensive and empirical analyses of these products, particularly focusing on hormonal and antioxidant systems. Since then, there have been continuous advancements in the field, encompassing not only the morphological and physiological analyses of treated plants but also the physicochemical characterization of product compositions and the mechanisms of action of biostimulant compounds on the metabolic pathways of crop species (Yakhin et al., 2017).
Building on these foundational insights, recent advancements have highlighted key benefits for crop production, such as enhanced growth, increased yield, and improved fruit quality (Soppelsa et al., 2019; Francesca et al., 2020; Mannino et al., 2020; Navarro-López et al., 2020; Hassan et al., 2021; Gitau et al., 2022; Santini et al., 2022; Riyazuddin et al., 2023). Additionally, there has been progress in mitigating biotic and abiotic stresses (Elansary et al., 2019; Fleming et al., 2019; Campobenedetto et al., 2021; D’Amato and Del Buono, 2021; Desoky et al., 2021; Irani et al., 2021; Riyazuddin et al., 2023; Borella et al., 2023; Kumar et al., 2024), enhancing beneficial soil microbiota and improving nitrogen use efficiency and crop yield (Carillo et al., 2019; Hellequin et al., 2020; Macias-Benitez et al., 2020; Acin-Albiac et al., 2023). Other notable advancements include increased shelf life (Shehata et al., 2017; Mannino et al., 2020; Góes et al., 2021; Ziogas et al., 2022) and the enhancement of bioactive compounds in plants (Rouphael et al., 2018; Jędrszczyk et al., 2018; Mannino et al., 2020; Ashour et al., 2021; Azad et al., 2021; Cirillo et al., 2022; Graziani et al., 2022).
Based on the various scientific evidence already reported, as well as the increased production of organic foods resulting from the growing demand of the population for healthy and sustainable food, biostimulant products have received greater acceptance from the sector, including farmers/producers and government agencies. According to Fortune Business Insights report, the global biostimulant market was valued at USD 2.85 billion in 2021 and it is expected to reach USD 6.69 billion by the end of 2029, exhibiting a compound annual growth rate (CAGR) of 11.43% during the forecast period (Fortune Business Insights, 2022).
In the sense of the legal framework, Yakhin et al. (2017) describe a discrepancy among various countries, states, and regions regarding the categorization and registration of biostimulant products, which can impact trade hinder further developments. The terminologies associated with biostimulants in legislation include planting conditioners, other fertilizers, supplements, soil correctives, plant fortifiers, and phytofortifiers, for example. In addition to the terminology used for product registration, many jurisdictions require detailed information identifying all substances present in the product, while others allow registration without complete identification, noting that these products are considered matrices of complex composition (Yakhin et al., 2017).
In the European Union, for example, the last regulation from 2019 stipulates that biostimulants are fertilizer products that confer: (a) nutrient use efficiency, (b) tolerance to abiotic stress, (c) quality traits, (d) availability of confined nutrients in soil or rhizosphere. Products must clearly indicate on the label the declared effects tested on plants, the physical form, production and expiration dates, methods of application, and other relevant instructions, such as soil management practices, chemical fertilization, and incompatibility with plant protection products. In addition, the regulation sets limit values for contaminants such as cadmium, chromium, lead, mercury, nickel, and arsenic (EU, 2019).
In the United States, the regulation of biostimulants still has significant gaps, with no clearly defined legislation despite a gradual increase in the commercialization of these products. In 2018, the Farm Bill, approved as the Agricultural Improvement Act, established some delimitations regarding biostimulants. According to the regulation, products must be evaluated for their active components; thus, a plant biostimulant could be defined as a plant regulator under the Federal Insecticide, Fungicide and Rodenticide Act (FIFRA), transferring its regulation to the Environmental Protection Agency (EPA). Other biostimulant products, in turn, can be regulated by state departments of agriculture, which legislate on fertilizers, plant and soil amendments, and others that are not included in FIFRA (Madende and Hayes, 2020).
In Brazil, biostimulants are considered bioinputs and are part of the National Bioinputs Program (Brazil, 2020). In Bill No. 3,668 of 2021, biostimulants are defined as products containing microorganisms, metabolites of microorganism action, or organic components, either isolated or combined, that stimulate biological processes and must be registered with the Ministry of Agriculture, Livestock, and Supply. However, the legislation lacks clarity regarding the type of labeling required for the commercialization of the biostimulant (bioinput) in the Brazilian market (Brazil, 2021).
Finally, one of the biggest obstacles to the standardization of biostimulant products regarding their production, registration, marketing, and use is the large number of molecules in their composition that are claimed to have stimulant properties for plant development, as well as the differing regulatory requirements. Because of this, different countries have sought to harmonize production and evaluation processes in the search for a common standard for these bioactive molecules (Caradonia et al., 2019).
4 Compounds of interest
Over the years, researchers have proposed different categorizations of biostimulant products, especially based on the main component or modes of action. In European Union countries, e.g., this information is included on the label of commercialized products and is serves as a means of registering biostimulants. The classification based on the origin of the raw material is the most commonly used (Bulgari et al., 2019). Thus, biostimulants can be divided into two groups: those of biological origin, where the components and molecules come from the plant or other organic sources, and those of physical or chemical origin (Shahrajabian et al., 2021).
In this context, certain groups of compounds can be mentioned, including phytohormones, polyamines, amino acids, polyphenolic compounds, and polysaccharides (Ali et al., 2021; Garg et al., 2024; Gholizadeh et al., 2024; Kumar et al., 2024; Mughunth et al., 2024). Given the significance of these compounds, the literature review conducted by Mughunth et al. (2024) describes various biological activities of marine algae for use as biostimulants in plants. These activities include increased productivity and quality of agricultural crops, enhancement of biochemical compounds, improved yield and essential oil content, as well as increased tolerance to (a)biotic stress factors. Similarly, plant biostimulants can also achieve comparable results (Sible et al., 2021; Garg et al., 2024). Thus, it is evident that these substances possess considerable potential for enhancing plant growth and resilience.
Regarding phytohormones, these low-molecular-weight compounds play crucial roles in regulating plant growth and development, being associated with both morphophysiological and biochemical responses (Roychoudhury and Aftab, 2021; Aguilar-Ayala and Herrera-Rojas, 2023). One of the main phytohormones is abscisic acid (ABA), which has long been considered the primary phytohormone linked to stomatal regulation and plant stress responses. However, other phytohormones also play significant roles in plants, including cytokinins, auxins, gibberellins, and ethylene (Roychoudhury and Aftab, 2021).
ABA, a bioactive terpenoid phytohormone, is recognized as one of the primary components in seaweed extracts (Yalçın et al., 2019). Its significance in plant physiology arises from its pivotal role in regulating various stress responses and developmental processes. As a biostimulant, ABA enhances several biochemical parameters, such as chlorophyll content, antioxidant enzyme activity, and osmotic adjustment compounds, which collectively contribute to improved plant resilience under adverse conditions (Souza et al., 2013; Marcińska et al., 2013; Ghassemi et al., 2019).
Cytokinins, along with auxins, are responsible for complex biochemical processes within plant systems, although many of these processes remain to be fully elucidated (Sakakibara, 2021). Together with auxins, they are well-known for their roles in plant growth and are commonly found in macroalgae, where they, along with macro and micronutrients, enhance developmental processes in plants (Patel et al., 2018; Kalasariya et al., 2021). Additionally, the review by Cortleven et al. (2019) reports its action in plant defense mechanisms against light, temperature, drought, osmotic, salinity, and nutritional stress factors, as well as a complete response to certain plant pathogens and herbivores.
Auxins constitute a group of low-molecular-weight phytohormones essential for plant growth and development. These molecules regulate multiple physiological processes, including cell division, elongation, differentiation, organogenesis, and responses to environmental stimuli (Gomes and Scortecci, 2021). The most abundant and biologically active auxin is indole-3-acetic acid (IAA), although other naturally occurring auxins, such as indole-3-butyric acid (IBA), 4-chloroindole-3-acetic acid (4-Cl-IAA), and phenylacetic acid (PAA), also contribute to plant development (Cao et al., 2019). In addition to growth regulation, auxins play a key role in stress responses, particularly in modulating root architecture under adverse conditions, such as low temperatures (Tiwari et al., 2023).
Gibberellins are classified as carboxylated diterpenoids, with modifications in their chemical structure depending on their source. Their biosynthesis occurs through the methylerythritol 4-phosphate (MEP) pathway, and they are primarily found in higher plants (Lichtenthaler et al., 1997). The most abundant and the first gibberellin to be characterized was the gibberellic acid (i.e., gibberellin A3), derived from the fungus Fusarium fujikuroi (Curtis and Cross, 1954). This compound is still produced on an industrial scale for use in agriculture (Rademacher, 2016). Notably, among their various biochemical properties, these molecules in biostimulants contribute to hormonal regulation (Achard et al., 2008). Gibberellins, due to their interactions with other phytohormones, help maintain a balance in the physiological state of plants during both abiotic and biotic stress (Castro-Camba et al., 2022; Li et al., 2021).
Ethylene, in turn, is one of the simplest and most accessible molecules for biosynthesis in plant systems. Recognized as an essential growth regulator, ethylene is involved in a variety of physiological and developmental processes, ranging from growth regulation to the induction of fruit ripening, as well as in multiple stress responses (Chen et al., 2021a). In response to biotic stress, ethylene plays a role in disease resistance by inducing the expression of genes involved in plant defense, such as pathogen-related proteins (PRs) and phytoalexins (Jasrotia and Jasrotia, 2022). Moreover, Chen et al. (2021a) have shown that ethylene content is augmented in responses to abiotic stress factors, such as drought, flooding/hypoxia, osmotic pressure, salinity, heat, cold, and heavy metals.
In the group of plant growth regulator compounds, polyamines play an important role. These small, naturally occurring organic polycations, with contain two or more amino groups, are found in both prokaryotes and eukaryotes, and they are crucial for various physiological processes in plants, such as growth and the increase of bioactive compounds (Blázquez, 2024; Gholizadeh et al., 2024). They have also demonstrated the ability to act in stress responses (Shao et al., 2022; Gholizadeh et al., 2024). Among the main exogenous polyamines used as potential biostimulants are putrescine, spermidine, spermine, and termospermin (Blázquez, 2024).
Putrescine is a simple polyamine composed of two amino acids, ornithine and arginine. It plays a crucial role in cell growth, differentiation, and apoptosis. Putrescine is involved in various physiological processes, including stress responses and the regulation of gene expression in plants. In addition to its direct relation to plant growth and developmental processes, it contributes to tolerance against various abiotic stresses, such as salinity, low and high temperatures, and drought (González-Hernández et al., 2022).
Spermidine is a higher-order polyamine derived from putrescine. It is involved in cellular processes such as cell proliferation, differentiation, and apoptosis. Additionally, spermidine has been linked to the regulation of ion channels and is recognized for its antioxidant properties, which contribute to stress tolerance in plants (Li et al., 2022; Xie et al., 2023).
Spermine is another polyamine formed from spermidine. It has a more complex structure and is involved in various cellular functions, including protection against oxidative stress and regulation of ion transport. Spermine also plays a significant role in plant growth and development (Hasan et al., 2021; Hai et al., 2022). Furthermore, it serves as a regulator of biosynthetic pathways, decreasing ethylene levels while increasing phenolic compounds and sugars. This modulation enables plants to better tolerate harmful abiotic effects, such as drought and salinity (Silva et al., 2023).
Thermospermine is a polyamine derivative of spermine, particularly important in certain plant species and associated with plant growth-related genes (Kakehi et al., 2008; Furumoto et al., 2024). Additionally, it has been linked to adaptation to environmental stresses (Furumoto et al., 2024).
Amino acids are essential for plant metabolism, serving not only as building blocks for proteins but also as key regulators of stress responses. Proline aids in osmotic adjustment and provides protection against oxidative stress, while glutamate acts as a precursor for proline and GABA (γ-aminobutyric acid), both crucial for stress adaptation (Gramazio et al., 2020). Arginine contributes to nitrogen storage and oxidative stress signaling, whereas methionine and cysteine help regulate redox homeostasis through glutathione and polyamines (Acheampong et al., 2024). Lysine catabolism produces proline and pipecolic acid, enhancing plant stress tolerance (Arruda and Barreto, 2020), while tryptophan is vital for auxin biosynthesis and root development. These amino acids interact with phytohormones like ABA and ethylene, influencing drought resilience and salinity responses (Soda et al., 2022). Advances in metabolic engineering have targeted amino acid biosynthesis to improve stress tolerance, revealing both benefits and metabolic trade-offs in crop improvement.
Polyphenolics are a class of secondary metabolites that play crucial roles in plant metabolism. They are widely distributed throughout various plant parts, including leaves, flowers, fruits, and seeds (Zagoskina et al., 2023). A broad diversity of polyphenols exists, and these compounds positively affect plant growth and development while also serving as elicitors of tolerance to (a)biotic stress factors. Examples include salicylic acid (Song et al., 2023), anthocyanins (Li and Ahammed, 2023), gallic acid (Zhang et al., 2022a), quercetin (Jańczak-Pieniążek et al., 2021), and the stilbene resveratrol (Wagner et al., 2022).
Finally, polysaccharides are macromolecules formed by long chains of monosaccharides, which are simple sugars. In plants, these compounds play essential structural and functional roles, serving as fundamental components of plant cell walls (Kabir et al., 2022). The application of polysaccharides derived from both marine and terrestrial sources has shown significant positive responses in plants (González-Pérez et al., 2018; Mamede et al., 2023). The study by Mamede et al. (2023) indicates that alga-derived polysaccharides such as agar, alginate, and carrageenan can enhance seed germination and plant vigor, increase nutrient absorption from the soil, and protect plants against various abiotic and biotic stresses, including salinity, drought, temperature extremes, and pathogens. Similarly, plant-derived polysaccharides like as xyloglucan, found in various plant species, contribute to soil aggregation and the maintenance of its physical properties (Galloway et al., 2018). Under stress conditions such as salinity, xyloglucans can induce the expression of tolerance genes, mitigating the negative effects of oxidative stress in plants (González-Pérez et al., 2018).
5 Adverse weather conditions and impact on agriculture
Global warming, industrialization, and climate change are interconnected through complex relationships. Significant increases in greenhouse gas (GHG) emissions, mainly carbon dioxide (CO2), from the burning of fossil fuels (Shahbaz and Sinha, 2019; Ahmed et al., 2022), have occurred since the beginning of industrialization. The increase in CO2 concentrations and other GHGs in the atmosphere traps heat and contributes to global warming, altering climate events such as droughts, more frequent and intense heat waves, changes in precipitation patterns and temperatures, and the melting of glaciers, as well as rising temperatures and sea level (Rummukainen, 2013; Shahgedanova, 2021; Ballinger et al., 2023).
Such changes can directly impact crop production due to the adverse environmental conditions which plants will be exposed (Warsi et al., 2021).
Climate plays a crucial role in determining the success or failure of agricultural practices and overall crop productivity. Climatic conditions such as temperature, sunlight, and rain directly influence the growth and development of plants, as each crop has specific temperature and photoperiod requirements for optimal growth (Assad et al., 2013). These variables affect the progression of crop stages across a wide spectrum, encompassing both vegetative and reproductive phases (flowering and fruiting). Deviations in climate patterns can disrupt these phenological stages (Körner, 2021). Furthermore, precipitation impacts water availability by altering soil moisture levels, with insufficient or excessive precipitation leading to droughts or floods, respectively, which consequently influences the availability of nutrients that plants can absorb (Dhaliwal et al., 2022; Elbasiouny et al., 2022) According to the FAO (2015; 2023), extreme adverse weather events such as droughts, floods, heat waves, and cold spells significantly impact agriculture and affect crop yields. In summary, climate change threatens global crop production, requiring adaptive agronomic strategies to enhance resilience (Zuma et al., 2023). Among these strategies, biostimulants play a key role in mitigating stress by modulating plant physiology and improving tolerance to environmental challenges (Bhupenchandra et al., 2022).
5.1 Cold stress
Temperature plays a fundamental role in the growth and development of plants, and extremely low or high temperatures cause great stress, restricting natural processes at the genetic, biochemical, and physiological levels. Especially in recent decades, due to climate change this environmental factor has been widely discussed by experts in the field of food production, who seek to explore new possibilities to face this challenge. In fact, low temperatures have represented one of the main environmental factors for the suppression of agricultural crops, which leads to drastic losses annually in the world (Saleem et al., 2021).
Estimates indicate losses of 50% in the field due to plant stresses resulting from temperature changes (Sangiorgio et al., 2020). In 1972 and 1976, losses of 42% and 37%, respectively, were reported in rice production in the northeast region of China due to severe cold stress (Chen et al., 2021b). In horticulture, for example, countries such as France, Germany, Italy, Belgium, Switzerland, and the USA recorded large agricultural losses due to increased frosts in the past years (Sangiorgio et al., 2020).
Regarding the low temperatures, plants can be categorized as cold-sensitive, cold-tolerant, and freeze tolerant. Plants sensitive to cold are those that die at the beginning of stress conditions, not resisting extreme conditions. In turn, freezing-tolerant plants are those that face the greatest cold stress (Saleem et al., 2021). Due to their cold adaptation characteristics, the geographic distribution of plants is directly affected, where tropical and subtropical plants (e.g., rice, soybeans, tomatoes, corn, and cotton) are sensitive to cold stress, lacking the ability to acclimate to cold, as temperate species (e.g., barley, wheat, and rye) have a greater capacity for freezing tolerance (Ritonga and Chen, 2020).
Low temperatures above freezing conditions can slow plant metabolism, decreasing photosynthetic levels, leaf growth, and early senescence. In conditions lower than freezing, the development of buds can be seriously impaired, resulting in the destruction of rehydrated buds. In cases of frost, plant tissues dehydrate, resulting in an increase in the concentrations of osmolytes in the cell cytoplasm, consequently, resulting in the rupture of the plasma membrane (Sangiorgio et al., 2020). Generally speaking, damage to plant tissues can lead to poor growth, delayed flowering, reduced fruit set, and lower productivity overall. The severity and duration of cold stress can determine the extent of yield and quality losses and, in extreme cases, result in plant death or total crop loss, which can extend beyond the immediate growing season and affect the production of future crop cycles (Bhattacharya, 2022).
Plants respond to cold stress through several physiological mechanisms to deal with adverse conditions, namely changes in gene expression, cellular metabolism, and adjustments in water and nutrient absorption. The plant’s response can occur through different metabolic pathways, each of which can represent tolerance, attempts to avoid, escape, and recover (Chen et al., 2021b). For example, as a response to cold stress, several pathways can be affected, such as calcium channels (Ca2+). From the moment extracellular ice is formed, which leads to water loss in cells, the integrity of cell membrane systems is affected, leading to the opening of Ca2+ channels, due to the loss of membrane fluidity. The change in Ca2+ concentration induces the effect of signaling cascades through mitogen-active protein kinases (MAPKs), which can reach transcriptomic levels. In the carbohydrate pathway, in turn, immediate reprogramming occurs, as it is necessary to avoid any type of imbalance that could cause cell damage or death (Fürtauer et al., 2019). Thus, the effects of cold stress include physiological adaptations to water deficiency, such as the accumulation of various osmolytes and antioxidants, changes in phospholipid composition, production of reactive oxygen and nitrogen species, and the activation of phosphoprotein cascades. In fact, the knowledge about stress detection and plant response is a key issue so that preventive measures can be implemented at these times (Chen et al., 2021b).
In this sense, biostimulant elicitors can play a crucial role in increasing plant resilience and improving tolerance to cold stress, as they can activate the plant’s natural defense mechanisms against stresses, stimulating them to grow. Biostimulants can help in the production of protective compounds, such as antioxidants, osmoprotectors, and heat shock proteins, which help the plant to cope with the cold, and modulate gene expression in plants, activating specific genes associated with cold tolerance, increasing the plant’s ability to withstand low temperatures and reduces the negative impact of cold stress. Furthermore, biostimulants can improve various physiological processes in plants, including photosynthesis, respiration, and nutrient metabolism, which improve overall health and vigor, making them more resistant to cold stress (Askari-Khorasgani et al., 2019).
6 Mechanisms of action of biostimulants and biofertilizers
Biostimulants enhance plant tolerance to cold stress by activating multiple protective pathways (Zulfiqar and Ashraf, 2021; Martínez-Lorente et al., 2024; Navarro-Morillo et al., 2024). These compounds operate through three primary mechanisms: enhancing antioxidant defense systems, accumulating osmoprotectants, and modulating hormonal signaling (Figure 1). Together, these mechanisms maintain membrane integrity, stabilize cellular proteins, and sustain essential metabolic functions, thereby mitigating the detrimental effects of cold temperatures on plant physiology (Dreyer and Dietz, 2018; Monterisi et al., 2024a; van Oosten et al., 2017).
6.1 Antioxidant defense enhancement
Reactive oxygen species (ROS) are byproducts of cellular metabolism that can accumulate under stress conditions, such as extreme temperatures. While low levels of ROS function as signaling molecules, excessive accumulation leads to oxidative damage to lipids, proteins, and DNA (Demidchik, 2017). Plants possess sophisticated antioxidant defense systems to maintain ROS homeostasis (Nadarajah, 2020), and the redox-regulatory network is critical for cold stress acclimation (Dreyer and Dietz, 2018).
Biostimulants significantly enhance these natural defense mechanisms by increasing the activities of antioxidant enzymes, including superoxide dismutase, catalase, and ascorbate peroxidase, which are crucial for scavenging ROS (Cerruti et al., 2024; Vasconcelos, 2020). Navarro-Morillo et al. (2024) demonstrated that algae-based biostimulants improved the physiological condition of zucchini plants under cold stress, contributing to the limitation ROS accumulation while simultaneously providing osmoprotection.
6.2 Osmoprotectant accumulation
Osmoprotection is a critical mechanism through which biostimulants enhance plant tolerance to cold stress. Under low-temperature conditions, plants face cellular dehydration and potential ice crystal formation, which can damage cellular structures. Biostimulants stimulate the biosynthesis and accumulation of key osmolytes, including secondary metabolites (proline, betaine, and putrescine) and compatible solutes (sucrose, glucose, raffinose, fructose, and trehalose) (Jahed et al., 2023).
This elevated concentration of osmolytes within the cytoplasm maintains a lower cellular water potential than the external environment, even under cold-induced dehydration. The resulting osmotic adjustment promotes water influx into cells, preserving cellular hydration and turgor pressure – both essential for cell expansion, stomatal regulation, and overall metabolic function (Jiménez-Arias et al., 2021; Aloui et al., 2023). Additionally, these osmolytes interact directly with proteins and membranes, preventing denaturation and maintaining the functional integrity of essential cellular components, including metabolic enzymes, photosynthetic complexes, and membrane-bound transporters (Rydeen et al., 2018; Jahed et al., 2023).
6.3 Hormonal signaling modulation
Biostimulants exert significant effects on plant hormone pathways that regulate cold stress responses. Cold stress directly impacts auxin transport by inhibiting the recycling of transmembrane proteins responsible for hormone redistribution, which compromises the establishment of auxin gradients essential for regulated growth (Rahman, 2013). While the relationship between exogenous auxin application and cold tolerance remains incompletely understood, research suggests that auxins mediate plant responses to low-temperature stress. For instance, IAA stimulates arbuscular mycorrhizal development, potentially contributing to plant adaptation under adverse environmental conditions such as cold (Kunkel et al., 2024).
Tiwari et al. (2023) demonstrated that disruptions in auxin transport and signaling pathways alter root morphology under cold stress, characterized by reduced auxin levels in root tips. This reduction inhibits positive cell cycle regulators while enhancing negative regulators. Notably, exogenous auxin application promotes root growth under cold stress conditions. However, it does not fully restore normal growth, suggesting the involvement of additional factors in the comprehensive cold stress response.
Cytokinins (CKs) represent another crucial hormone class modulated by biostimulants. Prerostova et al. (2021) investigated the impact of CK levels on cold stress responses using Arabidopsis thaliana transformants with either enhanced biosynthesis (DEX: IPT) or increased degradation (DEX: CKX) of Cks. Under various light conditions, plants with elevated CK and auxin levels, along with increased salicylic acid concentrations, demonstrated enhanced stress acclimation. Conversely, plants with reduced CK and auxin contents displayed weakened stress tolerance. This study highlighted the critical interplay between CKs and light signaling in regulating cold stress responses. Biostimulant application stimulates cytokinin modulation, effectively improving plant responses to the physiological stress caused by low temperatures (Benito et al., 2024; Monterisi et al., 2024b).
Ethylene, another plant hormone implicated in stress responses, exhibits context-dependent effects on cold stress tolerance (Huang et al., 2023). While it can contribute to acclimation, excessive ethylene accumulation can intensify cellular damage under cold conditions. Biostimulants help maintain optimal ethylene levels, preventing the potentially detrimental effects of low temperatures on cell physiology (Navarro-Morillo et al., 2024).
7 Biostimulants with defense-inducing effects against low temperatures
7.1 Commercial products from aquatic environments
As a marine representative, the brown macroalga Ascophylum nodosum is the species most used in commercial biostimulant products (Fleurence, 2022). Products derived from A. nodosum (alone or together with other bioactive compounds) have been tested for their ability to induce chilling and freezing tolerance in plants (Table 1).
The application of the commercial product Acadian® (a powdered alkaline extract of A. nodosum) in Arabidopsis thaliana significantly increased tolerance to freezing temperatures both in both in vitro and in vivo assays. Extract-treated plants recovered from freezing temperatures of -7.5°C in vitro and -5.5°C in in vivo. The plants exhibited reduced expression of the chlorophyllase genes AtCHL1 and AtCHL2 during freezing stress, resulting in a 70% reduction in chlorophyll damage (Rayirath et al., 2009). This product also protected A. thaliana plants from induced cold stress (-2°C) by enhancing chlorophyll content, possibly due to downregulation of chlorophyll degradation genes (AtCLH1 and AtCLH2) and the upregulation of the transcription factor DREB1A and the COR78/RD29A genes, which encode cryoprotective chloroplast stromal protein – key regulators of cold stress tolerance (Nair et al., 2012).
Acadian® also improved the survival of in vitro tobacco cells (BY-2) after exposure to freezing temperatures (0, -3, and -5°C). The treatment enhanced cell growth, membrane stability, and nuclear integrity, while reducing cell death of cold-stressed BY-2 tobacco cell lines. This seaweed extract influenced cellular and molecular regulation, triggering mechanisms such as osmolyte accumulation and antioxidant activity to combat freezing stress. This response was associated with the upregulation of key freezing tolerance genes, including galactinol synthase 2, pyrroline 5-carboxylate synthase, and acetyl-CoA carboxylase (Zamani-Babgohari et al., 2019).
Another alkaline powdered extract, the commercial products Algafect® and Algavyt+Zn/Mn® (a combination of the brown seaweeds A. nodosum, Fuccus spp., and Laminaria spp.), demonstrated positive effects on maize plants subjected to low temperatures (12 – 14°C). The combination of these two products reduced leaf necrosis in maize (with only 0–15% of the leaf area affected) and promoted plant growth, particularly in root development, as observed with Algavyt Zn/Mn®. The beneficial effect of the Zn/Mn treatments and seaweed extracts were associated with increased superoxide dismutase (SOD) activity in the root and leaf tissues, playing a key role in defense against oxidative stress, with Zn, Mn, Cu, and Fe serving as essential enzymatic co-factors (Bradáčová et al., 2016).
The use of a product combining A. nodosum extract (Cytolan Star®) with garlic oil improved the quality of Valencia orange fruits during cold storage at 5°C. Specifically, a solution of 1 g/l Cytolan Star® extract combined with 0.5% garlic oil resulted in the lowest percentage of fruit decay, the smallest reduction in total acidity, and the highest total sugar content. Additionally, treating the fruits with a mixture of 0.5% or 1% garlic oil and 3 g/l Cytolan Star® led to the lowest loss of weight (%) (Kamel, 2014).
7.2 Commercial products from terrestrial sources
Biostimulants from terrestrial sources (e.g., plant extracts, agricultural residues, soil microorganisms, and animal protein hydrolysates) have also been tested in plants to induce tolerance to low temperatures (Table 2).
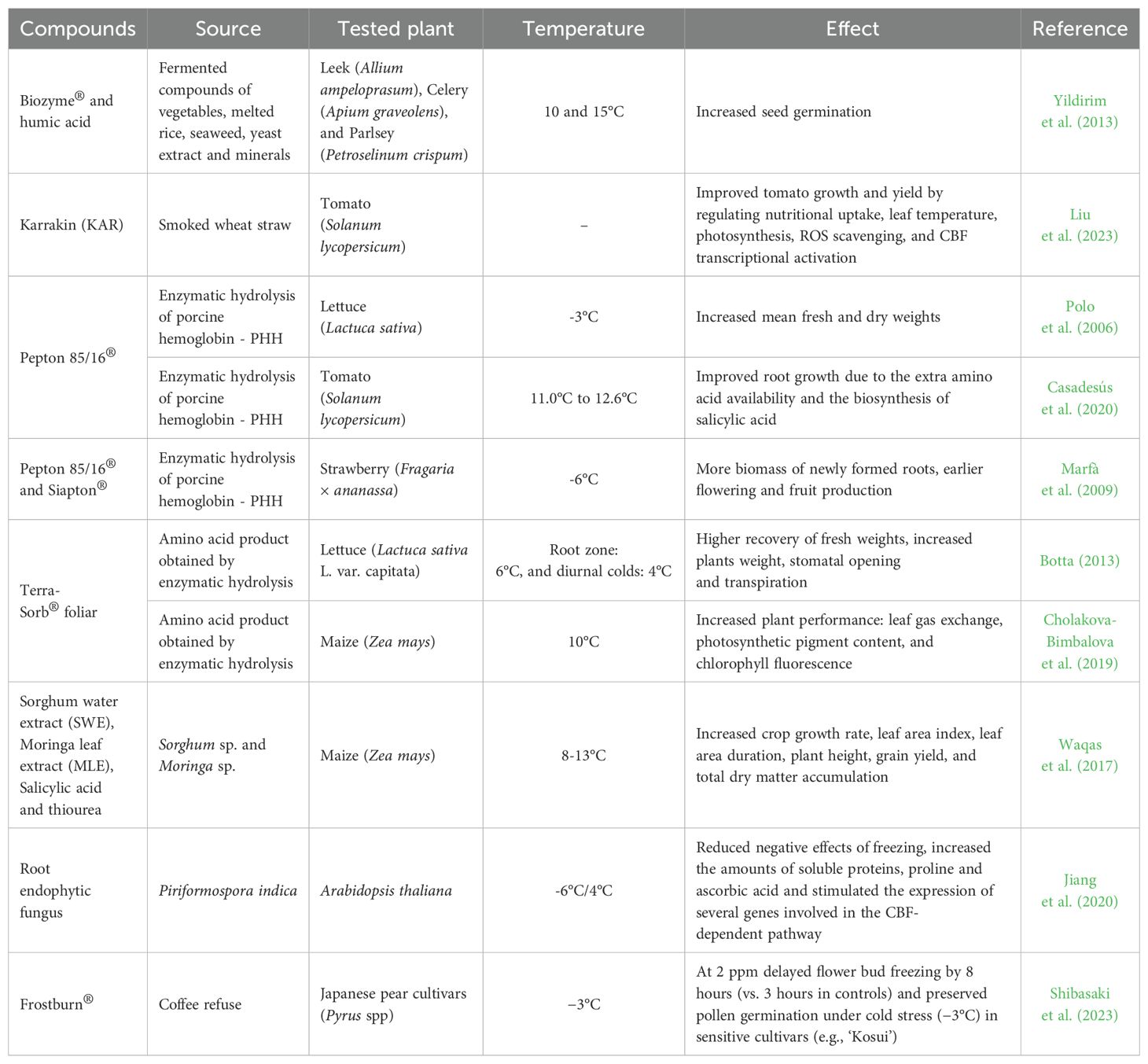
Table 2. Biostimulants derived from terrestrial sources inducers of chilling and frost tolerance in plants.
The commercial product Pepton 85/16® (an enzymatic hydrolyzed porcine hemoglobin – PHH) was tested in crop species such as tomato, lettuce, and strawberry plants. In the lettuce experiment, the mean fresh and dry weights of plants subjected to intense cold (-3°C, for 4h) and then treated with PHH at concentrations of 0.4, 0.8, and 1.6 mg/mL H2O were significantly greater than those of untreated plants (Polo et al., 2006). In tomato plants, Pepton (430 mg PHH/container) in a hydroponic system at low temperature (water temperature between 11.0°C and 12.6°C) promoted root growth by day 4 of the experiment, likely due to increased amino acid availability for growth and/or stimulation of specific hormonal pathways, such as salicylic acid (Casadesús et al., 2020). Strawberry plants were exposed to nighttime temperatures below zero, reaching -6°C, showed that the highest concentration of PHH (4 L/ha) resulted in a greater biomass of newly formed roots compared to the 2 L/ha treatment. Furthermore, both PHH treatments (2 and 4 L/ha) stimulated early flowering and significantly enhanced initial fruit production (Marfà et al., 2009).
Other commercial products found to induce cold tolerance in plants include Terra-Sorb® Foliar and Biozyme®. Terra-Sorb® Foliar is a protein hydrolyzate rich in amino acids obtained through enzymatic hydrolysis. In lettuce, a foliar application of 3 mL/L led to a greater recovery of fresh weight in both the root zone (at 6°C) and during daytime cooling (at 4°C). Additionally, treated lettuce exhibited significantly higher stomatal conductance at the onset of cold stress, resulting in enhanced stomatal opening and increased transpiration, which promotes the flow of water and nutrients from roots to shoots - an effect likely due either to the direct action of amino acids on stomata or to improved plant water status via stimulated root growth (Botta, 2013). When applied to maize plants grown at 10°C, Terra-Sorb® Foliar improved leaf gas exchange parameters by 22–23% and increased photosynthetic pigment content by around 15%, further supporting its potential to enhance recovery and overall cold tolerance in crops (Cholakova-Bimbalova et al., 2019).
In its turn, Biozyme® is a fermented product of vegetables, seaweed, and yeast. When applied in combination with humic acid, it resulted in enhanced seed germination of leek, celery, and parsley across all temperature treatments, including 10°C and 15°C (Yildirim et al., 2013). In addition to commercial products, other plant extracts have also been tested for chilling and freezing tolerance (Waqas et al., 2017; Jiang et al., 2020). A mix of two plant extracts (sorghum and moringa) combined with salicylic acid and thiourea was tested in maize plants and demonstrated stress-alleviating effects by improving physiological and biochemical attributes, as well as overall maize growth, under suboptimal temperature stress (8-13°C) (Waqas et al., 2017). Furthermore, the co-cultivation of the endophytic root fungus Piriformospora indica with A. thaliana, which was exposed to -6°C for 6 h, showed reduced negative effects of freezing. The plants exhibited higher levels of soluble proteins, proline, and ascorbic acid during the post-thaw recovery period at 4°C for 12 h (Jiang et al., 2020).
Frostburn®, a coffee-derived extract with anti-ice-nucleation properties, was evaluated at 2 ppm for mitigating cold-induced damage in the Japanese pear cultivars (Pyrus spp.) ‘Kosui’ and ‘Hosui’. When applied during the scale-separation stage (from pollen mother cell to tetrad phase), the extract delayed the onset of flower bud freezing by 8h, compared to just 3h in water-treated controls. This intervention preserved pollen germination rates under low-temperature stress (−3°C for 10h), attributed to reduced cellular dehydration and membrane disruption. Notably, ‘Hosui’ cultivar exhibited inherent cold tolerance, maintaining germination rates even at -6°C, while Frostburn® specifically enhanced resilience in the more sensitive ‘Kosui’ cultivar, highlighting its potential as a cost-effective commercial solution for cold stress management in orchards (Shibasaki et al., 2023).
An interesting group of natural plant growth regulators called karrakins (KAR), found in smoke from wheat straw, also has the ability to induce tolerance to low temperatures in plants (Liu et al., 2023). When applied alongside smoked water (SW) on tomato plants, KAR improved growth and yield under suboptimal temperature conditions by regulating nutrient uptake, leaf temperature control, photosynthetic defense mechanisms, reactive oxygen species scavenging, and the transcriptional activation of C-repeat binding factors (CBF). Thus, SW, which operates through the KAR-mediated strigolactones (SLs) and ABA signaling network, holds potential for enhancing cold tolerance in tomato production (Liu et al., 2023).
7.3 Non-commercial products
In addition to established commercial formulations, scientific research has increasingly explored naturally derived and non-commercial blends for their potential to enhance cold tolerance in plants (Table 3). A recent review by Akhtar et al. (2024) highlighted the use of nanobiotechnology and bacteria to promote cold tolerance in crop species. To better understand the current landscape of developing new products that induce cold tolerance in plants, it is relevant to expand the focus include other alternatives, such as crude algal extracts, plant-based extracts, and multi-component botanical and/or microbial mixtures. Although these formulations are not yet standardized or commercially available, they have been investigated for their ability to induce cold tolerance in crops.
Studies have investigated the role of Bacillus spp. in mitigating cold stress, demonstrating their ability to enhance plant resilience through multiple mechanisms. For instance, cold-tolerant strains such as Bacillus GBAC46 and RJGP41 promote growth by increasing the IAA biosynthesis and the activity of enzymes like SOD, CAT, and APX. Additionally, their volatile organic compounds (VOCs) improve root development and upregulate stress-related genes (Khan et al., 2023). Similarly, Lysinibacillus fusiformis and L. sphaericus enhance phosphorus solubilization and osmolyte accumulation (proline and glycine betaine), which improves photosynthesis and membrane stability under low temperatures (Jha and Mohamed, 2023). Furthermore, the synergistic action of Bacillus subtilis and Piriformospora indica further strengthens cold tolerance by enhancing biomass production, enzymatic defenses, and stress-related gene expression (Shi et al., 2024). These findings highlight Bacillus spp. as key contributors to plant adaptation under cold stress conditions.
In the study by Borella et al. (2023), a biostimulant derived from an unspecified brown alga species was evaluated in tomatoes exposed to low temperatures. Physiological and molecular analyses were conducted, revealing that stomatal conductance, net photosynthesis, and yield were significantly higher in the plants treated with the biostimulant compared to the untreated plants. Additionally, the molecular analysis indicated that the extract resulted in increased cellular contents of proline, polyphenols, flavonoids, tannins, and carotenoids.
In a postharvest study, Delgado-Vargas et al. (2022) evaluated the effects of hot water treatment (HWT – 45°C/5 min) on tomato fruits under chilling conditions (5°C/20 days). HWT-treated fruits exhibited reduced chilling injury (CI), higher total phenolics (TP), and greater antioxidant activity (AoxA) compared to controls. They also showed increased accumulation of phenolics, sugars, and certain alkaloids, potentially mediated by azelaic acid, glutamine, and tryptophan. Contrarily. N-feruloylputrescine, esculeoside AII, and hydroxy-α-tomatine II contents decreased. The improved metabolic performance of HWT-treated tomatoes during cold storage was linked to the accumulation of antioxidant and osmolyte compounds. Identifying metabolites associated with CI reduction enhances the understanding of tolerance mechanisms and offers targets for CI prevention, such as genetic improvements or direct metabolite application.
Chen et al. (2023) investigated the cold tolerance response of winter wheat (Triticum aestivum L.) treated with a composite of controlled-release diammonium phosphate (CRDAP) and the extract of the fungus species Paecilomyces variotii (ZNC). Under spring low-temperature stress, the composite synchronized phosphorus release with crop demand, boosting soil available phosphorus by 22% in the top 40 cm, enhancing root growth by nearly 30%, and elevating photosynthetic rates by up to 17% compared to conventional fertilizers. Moreover, there was an increased biosynthesis of IAA in roots and strengthened antioxidant defenses, with an 8.6% reduction in hydrogen peroxide (H2O2) concentration, further improving cold resilience.
Mastouri et al. (2010) investigated the effects of Trichoderma harzianum T22 seed treatment on tomato seeds under chilling stress. The results showed that T22-treated seeds germinated significantly faster than untreated seeds, even when exposed to chilling stress at 10°C for up to 3 days. Although T22 treatment accelerated germination speed, the final germination percentage did not differ significantly from that of the control seeds. This positive effect of T22 on germination under thermal stress suggests that the application of this fungal species may trigger a physiological response in seeds, enhancing germination speed under cold conditions without negatively affecting the final germination rate.
In the study conducted by Poveda et al. (2020), the inoculation of kale plants with various fungal isolates, including Pyrenophora sp., Fusarium sp., Phialocephala sp., Chaetomium sp., Diaporthe sp., and Acrocalymma sp., significantly increased plant growth and tolerance under cold stress (12°C). Inoculation with Phialocephala, Fusarium sp., and Acrocalymma sp. notably enhanced plant weight, with an almost twofold increase compared to the control. Similarly, dry weight also increased in the inoculated plants, highlighting the positive impact of fungal inoculation on cold tolerance.
Afrouz et al. (2023) determined the effect of seed biopriming with Trichoderma harzianum on maize tolerance to cold stress. The results showed that biopriming significantly enhanced seedling emergence and physiological parameters, particularly under cold conditions (5°C). Among the pretreatments, T. harzianum resulted in improved root dry weight and increased catalase activity in the maize cultivars investigated, with the highest root dry weight detected in the cultivar AR68. These findings suggest that T. harzianum priming effectively enhances tolerance in maize.
In the study by Ghorbanpour et al. (2018), the inoculation of T. harzianum AK20G strain in tomato seeds was investigated for its role inducing cold tolerance in plantlets exposed to 8°C for 6 days. The results showed that T. harzianum effectively mitigated the adverse effects of cold stress by enhancing photosynthesis and growth rates, reducing lipid peroxidation, and minimizing electrolyte leakage. Additionally, this fungal species improved leaf water content and proline accumulation while promoting the expression of genes involved in cold stress tolerance, such as TAS14 and P5CS.
Junaid et al. (2019) evaluated the effect of moringa leaf extract (MLE – Moringa oleifera) in maize (Zea mays L.) exposed to low temperatures (5°C-10°C) during early seedling development. Seed priming (24 h) with 3% MLE improved chilling tolerance by enhancing the stand establishment and increasing both emergence percentage and speed. The greatest improvements in agronomic traits, yield, and quality were observed in 20-day-old transplanted seedlings, while direct sowing and 30-day-old transplants showed inferior performance. These results highlight the potential of MLE priming to mitigate chilling stress and enhance maize productivity under temperature fluctuations.
7.4 Other exogenously applied cold and chilling tolerance inducers
Since commercial products or natural extracts that effectively induce tolerance to cold, chilling, or freezing effects are scarce, some isolated compounds with the intended biological effect have been investigated, yielding interesting results (Table 4).
The plant phytohormone ABA has been demonstrated to be a key regulator of plant responses to abiotic stresses, including chilling and freezing (Vishwakarma et al., 2017). ABA application reduced the chilling damage (5°C) in four rice genotypes. Pre-treatment with ABA decreased the levels of superoxide anion (O2-), H2O2 and malondialdehyde (MDA) caused by chilling stress through increasing the activities of SOD, CAT, APX, glutathione reductase and the contents of ascorbic acid and glutathione (Wang et al., 2013). ABA also alleviated chilling injury of banana fruits, by inducing the accumulation of endogenous ABA, (un)saturated fatty acids, and flavonoids, by upregulating the transcription levels of MaABI5-like, fatty acid desaturation genes, and flavonoid synthesis-related genes during cold storage (Song et al., 2022). The application of ABA (26 mg/mL) to dormant buds of grapevine (Vitis vinifera) increased the expression of the CBF/DREB1, VvCBF2, VvCBF3, VvCBF4, and VvCBF6 transcription factors (Rubio and Pérez, 2019). Liu et al. (2022) describe that applying ABA in the mangrove species Kandelia obovata under natural frost conditions at approximately 32°N latitude effectively alleviated the adverse effects of freezing stress. This was achieved by activating antioxidant enzyme activity and increasing the accumulation of osmolytes, such as proline. Notably, the effectiveness of these responses was proportional to the concentration of ABA applied.
Another interesting effect of the exogenous application of ABA is the increase in endogenous concentrations of glycinebetaine (GB) (Xing and Rajashekar, 2001). GB is an amino acid that helps protect plants against abiotic stresses through osmoregulation or osmoprotection, and it contributes to the differential expression of stress tolerance-related genes (Giri, 2011). When applied in isolation, GB has demonstrated various effects on cold or freezing tolerance in plants. For instance, a 10 mM GB foliar spray on Arabidopsis tahaliana increased the freezing tolerance of plants to -4.5°C (Xing and Rajashekar, 2001). Similarly, maize seeds treated with GB significant improvements in germination rate, root and shoot length, seedling fresh and dry weight, leaf and root scores, soluble sugars, α-amylase activity, and total antioxidants capacity compared to untreated seeds under both optimal (27°C) and stress conditions (15°C) (Farooq et al., 2008). GB-treated tomato seeds (10 mmol/L) also exhibited better germination rates under cold stress (14°C). Analysis of gene expression and metabolism revealed that GB positively regulated endogenous gibberellin content, while the opposite effect was observed for abscisic acid content. Moreover, GB reduced the starch content in the tomato seeds by upregulating amylase gene expression (Zhang et al., 2022b).
Another important compound with recognized tolerance-inducing activity in plants is SA (Khan et al., 2015). Under low-temperature stress conditions (2°C), the application of 0.5 mM SA led to the upregulation of antioxidant enzymes, including APX, SOD, guaiacol peroxidase (GPOX), and GR (Janda et al., 1999). It also inhibited the activity of isozymes CAT-1 and CAT-2 in Z. mays (Horváth, 2002). The application of SA at 50 mg/L on the leaves of Z. mays seedlings under 4°C increased the antioxidant activities of APX, CAT, SOD, and peroxidase (POD), which decreased relative electrolyte conductivity (REC) and the levels of MDA and ROS (H2O2 and O2−). Additionally, there was an increase in proline content and relative water content (RWC) in the maize seedlings, enhancing their osmotic adjustment capacity (Zhang et al., 2021). High activities of CAT and SOD were also found in cut anthurium flowers (Anthurium andraeanum) subjected to cold stress (4°C to 12°C), resulting in detoxification of ROS and maintenance of membrane integrity (Promyou et al., 2012).
In apple plants (Malus domestica), SA increased the expression of cytosolic malate dehydrogenase and improved the redox state of the plant cell. Furthermore, transgenic plants that overexpressed cytosolic malate dehydrogenase (MdcyMDH) demonstrated greater tolerance to cold stress compared to the wild type, as they produced higher amounts of free and total amino acids (Wang et al., 2016).
The application of SA also aids in post-harvest activities by increasing the chilling tolerance of fruits during cold storage through the upregulating of stress-related proteins. Low concentrations of methyl salicylate (MeSA), i.e., 0.01 mM, helped protect tomato fruits (S. lycopersicum) in cold storage (5°C) by inducing the synthesis of certain stress-related proteins, such as the PR proteins PR-2b and PR-3a mRNAs, while also slightly increasing PR-3b mRNA accumulation (Ding et al., 2002). MeSA treatment on peaches fruits (Prunus persica) during cold storage (0°C) helped overcome chilling stress by upregulating the expression of small heat shock protein (sHSP) genes, ultimately leading to the production of sHSP, particularly HSP17.6 (Wang et al., 2006). Furthermore, a concentration of 2.0 mM SA-mediated enhanced the synthesis of total phenolics and increased the activity of phenylalanine ammonia-lyase, thereby improving chilling tolerance in cold-stored lemon fruit (Citrus limon; Siboza et al., 2014).
The study by Wang et al. (2022) investigated the effects of cold and SA on the accumulation of osmolytes in wheat leaves under freezing stress. The results indicated that both treatments significantly increased the levels of sucrose and free proline, improving the leaf’s water potential and reducing cell death, which enhanced tolerance to freezing. The accumulation of proline was promoted by both increased synthesis and inhibition of its degradation. Additionally, cold and SA stimulated the synthesis and hydrolysis of sucrose, positively regulating glucose catabolism and ammonia assimilation. These findings suggest that both treatments favor the accumulation of proline and sucrose, coordinating carbon and nitrogen metabolism to confer resistance to freezing stress.
The combination of SA with other compounds has also yielded interesting results. When SA was applied alongside AsA and H2O2, it improved seedling growth, leaf relative water content, chlorophyll b levels, membrane stability, and enzymatic antioxidant activities in pot-cultivated maize (Ahmad et al., 2014). In field experiments, the application of these substances – either through seed priming or foliar spray – enhanced morphological and yield-related attributes, as well as grain yield of spring maize under suboptimal temperatures in the early stages (>2.9°C). Notably, seed priming proved to be more effective than foliar application (Ahmad et al., 2014).
Priming treatments have also been shown to induce cold tolerance in plants. For instance, melatonin (MT) priming in maternal wheat plants (T. aestivum) during the grain filling stage promoted seed germination at 10°C in offspring. This effect was attributed to accelerated starch degradation and improved cold tolerance of the seedlings (10°C day; 6°C night) through the activation of antioxidant enzymes and enhancement of photosynthetic electron transport efficiency (Li et al., 2018).
The application of MT has been linked to specific mechanism of cold tolerance, such as a decrease in MDA levels and electrolyte leakage (EL). Additionally, it has associated with increased amounts of chlorophyll and enhanced enzymatic activity (e.g., SOD and POX), as well as changes in 46 metabolites when exogenously applied to bermudagrass plants (Cynodon dactylon). Among the measured metabolites, five sugars (arabinose, mannose, glucopyranose, maltose, and turanose) and one organic acid (propanoic acid) were significantly increased (Fan et al., 2015). Tea plants (Camellia sinensis) treated with MT on their leaves exhibited a reduction in ROS burst, decreased MDA levels, and maintained high photosynthetic efficiency. Moreover, these tea plants demonstrated elevated levels of GSH and AsA, along with increased activities of SOD, POX, CAT, and APX under cold stress (4°C). Notably, MT treatments can positively upregulate the expression of genes involved in the biosynthesis of antioxidant enzyme, specifically CsSOD, CsPOX, CsCAT, and CsAPX (Li et al., 2019).
In a similar study, maize seed priming with MT (50 and 500 μM) enhanced stress tolerance by improving germination under suboptimal thermal conditions (i.e., 5°C) and modifying the seed proteome. Proteomic analysis revealed MT-induced changes in protein expression related to stress response, suggesting a biochemical mechanism for enhanced chilling tolerance. These findings indicate that MT priming can be an effective strategy to improve maize resilience under low-temperature conditions (Kołodziejczyk et al., 2016).
In another priming study, Cao et al. (2019) demonstrated that MT (50 and 100 μM) improved maize germination under chilling stress at 13°C by increasing germination potential, seed vigor, and root growth. MT-treated seeds exhibited reduced oxidative damage (lower H2O2 and MDA levels) and enhanced antioxidant enzyme activity (SOD, POX, CAT, APX), along with improved starch metabolism, further supporting its role in cold stress tolerance.
Additionally, the exogenous application of MT could restore the rhythmic circadian oscillation of clock genes, such as HvCCA1 and HvTOC1, in barley (Hordeum vulgare) seedlings, whose rhythmic phenotypes were disrupted due to environmental cold stress (5°C and 15°C). The results also confirmed that exogenous MT reduced the accumulation of key physiological indicators under cold stress, including MDA and soluble sugars (Chang et al., 2021).
Polyamines represent an important group of compounds known for their effects on enhancing cold tolerance in plants. A recent review by Shao et al. (2022) summarized the benefits of exogenous polyamine applications on plant stress responses to cold. Here, we briefly highlight a few recent studies. For instance, Gholizadeh et al. (2024) reported increases in morphophysiological and biochemical parameters in two wheat cultivars (‘Mihan’ and ‘Rakhshan’) grown under cold stress (4°C) when treated with putrescine and spermidine. The authors observed augmented activity of antioxidant-related enzymes such as catalase (CAT) and ascorbate peroxidase (APX), with concomitant upregulation of genes involved in polyamine synthesis (e.g., TaADC, TaSAMDC, and TaSPDS) and catabolism (TaPAO11–7B and TaPAO11–7D) (Gholizadeh et al., 2024). Similarly, He et al. (2024) demonstrated that exogenous spermidine enhanced cold resistance in 1-year-old apple branches by stabilizing tissue, reducing reactive oxygen species through increased proline content, and boosted antioxidant enzyme activity. They also noted the upregulation of key polyamine metabolism genes (MdADC1, MdSAMDC1, and MdSPDS1), alongside the cold-responsive genes (MdCBF1/2/3, MdCOR47, and MdKIN1). Collectively, these findings indicate that exogenous polyamines modulate metabolism and enhance antioxidant defenses, thereby improving cold tolerance in diverse crops.
8 Conclusions, gaps and perspectives
Rising environmental challenges, notably extreme temperatures, demand innovative solutions for crop production and protection. Low temperatures pose a significant threat, leading to substantial global agricultural losses. Plants respond to cold stress through various physiological mechanisms, including changes in gene expression, adjustments in cellular metabolism, and alterations in water and nutrient absorption. Therefore, finding effective solutions to address this issue is urgent.
Bioactive elicitors from macro- and microalgae enhance plant resilience to cold by activating natural defense mechanisms, promoting the expression of cold tolerance genes, and improving physiological processes. Commercial products derived from macroalgae, particularly Ascophyllum nodosum, are among the most widely used and show promise in boosting cold tolerance by increasing gene expression, reducing chlorophyll damage, and stimulating plant growth. Similarly, plant-derived biostimulants, such as extracts and soil microorganisms, can promote resilience in crops, by enhancing nutrient uptake, improving overall plant health, and stimulating natural defense mechanisms.
One of the key aspects highlighted in this review is the use of commercial products like Acadian® and Algafect®, along with natural extracts, microorganisms and compounds such as glycine betaine and salicylic acid, in enhancing plant tolerance to low temperatures. These inputs have shown promising results in improving plant growth, root development, antioxidant activity, and gene expression related to cold stress.
However, several potential research gaps remain regarding broader applicability, long-term effects, optimal dosages, and practical implementation in agricultural settings. Further investigation in this area is essential to develop more effective strategies for mitigating cold stress in crop systems. This research could ultimately bridge the gap between laboratory findings and practical agricultural solutions for challenging climates.
Investigating the potential synergy between melatonin and other stress-responsive compounds or hormones, e.g., remains an interesting avenue. Combining melatonin with other biostimulants, such as algae-based products, may unlock enhanced stress tolerance that surpasses the capabilities of each compound when used in isolation.
There is still a need for improved guidelines and analysis parameters for determining whether a product qualifies as a biostimulant, whether it derives from a terrestrial or marine organism, or from purified molecules. Additionally, it is crucial to ascertain the most effective concentrations, optimal timing, and suitable delivery methods, taking into account variations among plant species and stress conditions. An essential aspect of research involves understanding the kinetics of absorption and distribution of certain molecules within plants.
Author contributions
EO: Conceptualization, Formal Analysis, Investigation, Methodology, Supervision, Writing – original draft, Writing – review & editing. AN: Conceptualization, Formal Analysis, Investigation, Methodology, Supervision, Validation, Writing – original draft, Writing – review & editing. FD: Data curation, Investigation, Validation, Writing – review & editing. GA: Investigation, Methodology, Validation, Writing – review & editing. AS: Investigation, Methodology, Writing – review & editing. BS: Investigation, Methodology, Writing – review & editing. DM: Writing – review & editing. SM: Supervision, Visualization, Writing – review & editing. GL: Supervision, Visualization, Writing – review & editing. MM: Formal Analysis, Supervision, Validation, Visualization, Writing – original draft, Writing – review & editing.
Funding
The author(s) declare that financial support was received for the research and/or publication of this article. The research fellowship from the Foundation for Research Support of Santa Catarina (FAPESC) and the National Council for Scientific and Technological Development (CNPq) was awarded to EO (process no. 303956/2023-2). Research funding from CNPq was also provided for AS (process no. 142391/2020-4), MM (process no. 405949/2022-7), and GL (process no. 3311719/2023-6). A research fellowship from the São Paulo Research Foundation (FAPESP) was granted to AN (process no. 2023/03886−1). Additionally, funding from the Federal Agency for Support and Evaluation of Graduate Education (CAPES) was awarded to FD (process no. 88887.696139/2022-00). Finally, the research fellowship from the Research Support Foundation of the State of Rio Grande do Sul and CNPq was awarded to DM (process no. 23/2551-0001935-3).
Conflict of interest
The authors declare that the research was conducted in the absence of any commercial or financial relationships that could be construed as a potential conflict of interest.
Generative AI statement
The author(s) declare that no Generative AI was used in the creation of this manuscript.
Publisher’s note
All claims expressed in this article are solely those of the authors and do not necessarily represent those of their affiliated organizations, or those of the publisher, the editors and the reviewers. Any product that may be evaluated in this article, or claim that may be made by its manufacturer, is not guaranteed or endorsed by the publisher.
References
Achard, P., Gong, F., Cheminant, S., Alioua, M., Hedden, P., Genschik, P. (2008). The cold-inducible CBF1 factor–dependent signaling pathway modulates the accumulation of the growth-repressing DELLA proteins via its effect on gibberellin metabolism. Plant Cell 20, 2117–2129. doi: 10.1105/tpc.108.058941
Acheampong, A., Wang, R., Elsherbiny, S. M., Bondzie-Quaye, P., Huang, Q. (2024). Exogenous arginine promotes the coproduction of biomass and astaxanthin under high-light conditions in Haematococcus pluvialis. Bioresour Technol. 393, 130001. doi: 10.1016/j.biortech.2023.130001
Acin-Albiac, M., García-Jiménez, B., Marín Garrido, C., Borda Casas, E., Velasco-Alvarez, J., Serra, N. S., et al. (2023). Lettuce soil microbiome modulated by an L-α-amino acid-based biostimulant. Agriculture 13, 344. doi: 10.3390/agriculture13020344
Afrouz, M., Sayyed, R. Z., Fazeli-Nasab, B., Piri, R., Almalki, W., Fitriatin, B. N. (2023). Seed bio-priming with beneficial Trichoderma harzianum alleviates cold stress in maize. PeerJ 11, e15644. doi: 10.7717/peerj.15644
Aguilar-Ayala, I., Herrera-Rojas, D. (2023). Application of phytohormones, growth regulators, and calcium to preserve fruit quality in pre- and post-harvest. Em New Advances in Postharvest Technology. IntechOpen 1–14. doi: 10.5772/intechopen.109624
Ahmad, I., Basra, S. M. A., Wahid, A. (2014). Exogenous application of ascorbic acid, salicylic acid and hydrogen peroxide improves the productivity of hybrid maize under at low temperature stress. Int. J. Agric. Biol. 16, 825–830.
Ahmed, F., Ali, I., Kousar, S., Ahmed, S. (2022). The environmental impact of industrialization and foreign direct investment: Empirical evidence from Asia-Pacific region. Environ. Sci. pollut. Res. Int. 29, 29778–29792. doi: 10.1007/s11356-021-17560-w
Akhtar, N., Shahzad, A., Ilyas, N., Bostan, N., Jameel, M. A., Mukhtar, S., et al. (2024). Nanobiotechnology and microbial influence on cold adaptation in plants. Nanotechnol Rev. 13, 1–10. doi: 10.1515/ntrev-2024-0059
Ali, O., Ramsubhag, A., Jayaraman, J. (2021). Biostimulant properties of seaweed extracts in plants: Implications towards sustainable crop production. Plants 10, 531. doi: 10.3390/plants10030531
Aloui, D., Kalleli, F., M’Hamdi, M., Manaa, M., Abid, G., Karmous, C. (2023). Marine algal extract as a biostimulant to improve tolerance to salinity in lettuce plants. Emirates J. Food Agric. 35 (12), 1–18. doi: 10.9755/ejfa.2023.3199
Arruda, P., Barreto, P. (2020). Lysine catabolism through the saccharopine pathway: Enzymes and intermediates involved in plant responses to abiotic and biotic stress. Front. Plant Sci. 11. doi: 10.3389/fpls.2020.00587
Ashour, M., Hassan, S. M., Elshobary, M. E., Ammar, G. A. G., Gaber, A., Alsanie, W. F., et al. (2021). Impact of commercial seaweed liquid extract (TAM®) biostimulant and its bioactive molecules on growth and antioxidant activities of hot Pepper (Capsicum annuum). Plants 10, 1045. doi: 10.3390/plants10061045
Askari-Khorasgani, O., Hatterman-Valenti, H., Flores Pardo, F. B., Pessarakli, M. (2019). Plant and symbiont metabolic regulation and biostimulants application improve symbiotic performance and cold acclimation. J. Plant Nutr. 42, 2151–2163. doi: 10.1080/01904167.2019.1648681
Assad, E. D., Martins, S. C., Beltrão, N. E., de, M., Pinto, H. S. (2013). Impacts of climate change on the agricultural zoning of climate risk for cotton cultivation in Brazil. Pesquisa Agropecuaria Bras. 48, 1–8. doi: 10.1590/s0100-204x2013000100001
Azad, M. O. K., Park, B. S., Adnan, M., Germ, M., Kreft, I., Woo, S. H., et al. (2021). Silicon biostimulant enhances the growth characteristics and fortifies the bioactive compounds in common and Tartary buckwheat plant. J. Crop Sci. Biotechnol. 24, 51–59. doi: 10.1007/s12892-020-00058-1
Ballinger, T. J., Bigaalke, S., Walsh, J. E., Brettschneider, B., Thoman, R. L., Bhatt, U. S., et al. (2023). NOAA Arctic report card 2023: Surface air temperature. NOAA Global Ocean Monit. Observ Program 23-02, 1–6. doi: 10.25923/X3TA-6E63
Bello, A. S., Saadaoui, I., Ben-Hamadou, R. (2021). Beyond the source of bioenergy”: Microalgae in modern agriculture as a biostimulant, biofertilizer, and anti-abiotic stress. Agronomy 11, 1610. doi: 10.3390/agronomy11081610
Benito, P., Celdrán, M., Bellón, J., Arbona, V., González-Guzmán, M., Porcel, R., et al. (2024). The combination of a microbial and a non-microbial biostimulant increases yield in lettuce (Lactuca sativa) under salt stress conditions by up-regulating cytokinin biosynthesis. J. Integr. Plant Biol. 66, 2140–2157. doi: 10.1111/jipb.13755
Bhattacharya, A. (2022). “Effect of low-temperature stress on germination, growth, and phenology of plants,” in Physiological processes in plants under low temperature stress (Springer, Singapore), 1–106.
Bhupenchandra, I., Chongtham, S. K., Devi, E. L., R, R., Choudhary, A. K., Salam, M. D., Sahoo, M. R., et al. (2022). Role of biostimulants in mitigating the effects of climate change on crop performance. Front. Plant Sci. 13. doi: 10.3389/fpls.2022.967665
Blázquez, M. A. (2024). Polyamines: Their role in plant development and stress. Annu. Rev. Plant Biol. 75, 95–117. doi: 10.1146/annurev-arplant-070623-110056
Borella, M., Baghdadi, A., Bertoldo, G., Della Lucia, M. C., Chiodi, C., Celletti, S., et al. (2023). Transcriptomic and physiological approaches to decipher cold stress mitigation exerted by brown-seaweed extract application in tomato. Front. Plant Sci. 14. doi: 10.3389/fpls.2023.1232421
Botta, A. (2013). Enhancing plant tolerance to temperature stress with amino acids: An approach to their mode of action. Acta Hortic. 1009, 29–35. doi: 10.17660/actahortic.2013.1009.1
Bradáčová, K., Weber, N. F., Morad-Talab, N., Asim, M., Imran, M., Weinmann, M., et al. (2016). Micronutrients (Zn/Mn), seaweed extracts, and plant growth-promoting bacteria as cold-stress protectants in maize. Chem. Biol. Technol. Agric. 3, 1–10. doi: 10.1186/s40538-016-0069-1
Brazil (2020). Bioinsumos (Brasília: Ministry of Agriculture and Livestock). Available at: https://www.gov.br/agricultura/pt-br/assuntos/inovacao/bioinsumos (Accessed December 3, 2024).
Brazil (2021). Projeto de Lei n° 3668, de 2021 (Brasília: Senado Federal). Available at: https://legis.senado.leg.br/sdleg-getter/documento?dm=9028589&ts=1656603196022&disposition=inline (Accessed November 21, 2024).
Bulgari, R., Franzoni, G., Ferrante, A. (2019). Biostimulants application in horticultural crops under abiotic stress conditions. Agronomy 9, 306. doi: 10.3390/agronomy9060306
Campobenedetto, C., Mannino, G., Beekwilder, J., Contartese, V., Karlova, R., Bertea, C. M. (2021). The application of a biostimulant based on tannins affects root architecture and improves tolerance to salinity in tomato plants. Sci. Rep. 11, 354. doi: 10.1038/s41598-020-79770-5
Cao, Q., Li, G., Cui, Z., Yang, F., Jiang, X., Diallo, L., et al. (2019). Seed priming with melatonin improves the seed germination of waxy maize under chilling stress via promoting the antioxidant system and starch metabolism. Sci. Rep. 9, 15044. doi: 10.1038/s41598-019-51122-y
Caradonia, F., Battaglia, V., Righi, L., Pascali, G., La Torre, A. (2019). Plant biostimulant regulatory framework: Prospects in Europe and current situation at international level. J. Plant Growth Regul. 38, 438–448. doi: 10.1007/s00344-018-9853-4
Carillo, P., Colla, G., Fusco, G. M., Dell’Aversana, E., El-Nakhel, C., Giordano, M., et al. (2019). Morphological and physiological responses induced by protein hydrolysate-based biostimulant and nitrogen rates in greenhouse spinach. Agronomy 9, 450. doi: 10.3390/agronomy9080450
Casadesús, A., Pérez-Llorca, M., Munné-Bosch, S., Polo, J. (2020). An enzymatically hydrolyzed animal protein-based biostimulant (pepton) increases salicylic acid and promotes growth of tomato roots under temperature and nutrient stress. Front. Plant Sci. 11. doi: 10.3389/fpls.2020.00953
Castro-Camba, R., Sánchez, C., Vidal, N., Vielba, J. (2022). Interactions of gibberellins with phytohormones and their role in stress responses. Horticulturae 8, 241. doi: 10.3390/horticulturae8030241
Cerruti, P., Campobenedetto, C., Montrucchio, E., Agliassa, C., Contartese, V., Acquadro, A., et al. (2024). Antioxidant activity and comparative RNA-seq analysis support mitigating effects of an algae-based biostimulant on drought stress in tomato plants. Physiol Plant 176, e70007. doi: 10.1111/ppl.70007
Chang, T., Zhao, Y., He, H., Xi, Q., Fu, J., Zhao, Y. (2021). Exogenous melatonin improves growth in hulless barley seedlings under cold stress by influencing the expression rhythms of circadian clock genes. PeerJ 9, e10740. doi: 10.7717/peerj.10740
Chen, H., Bullock, D. A. J. R., Alonso, J. M., Stepanova, A. N. (2021a). To fight or to grow: The balancing role of ethylene in plant abiotic stress responses. Plants 11, 33. doi: 10.3390/plants11010033
Chen, X., Ding, Y., Yang, Y., Song, C., Wang, B., Yang, S., et al. (2021b). Protein kinases in plant responses to drought, salt, and cold stress. J. Integr. Plant Biol. 63, 53–78. doi: 10.1111/jipb.13061
Chen, Q., Qu, Z., Zhang, Z., Ma, G., Zhu, M., Dan, J., et al. (2023). Coated diammonium phosphate combined with Paecilomyces variotii extracts improves root architecture, enhances spring low temperature tolerance, and increases wheat yield. Soil Tillage Res. 227, 105613. doi: 10.1016/j.still.2022.105613
Cholakova-Bimbalova, R., Petrov, V., Vassilev, A. (2019). Photosynthetic performance of young maize (Zea mays L.) plants exposed to chilling stress can be improved by the application of protein hydrolysates. Acta Agrobot 72. doi: 10.5586/aa.1769
Cirillo, A., De Luca, L., Graziani, G., Cepparulo, M., El-Nakhel, C., Giordano, M., et al. (2022). Biostimulants application on Olea europaea L. @ in Mediterranean conditions increase the production and bioactive compounds of drupes and oil. Agriculture 12, 2173. doi: 10.3390/agriculture12122173
Cortleven, A., Leuendorf, J. E., Frank, M., Pezzetta, D., Bolt, S., Schmülling, T. (2019). Cytokinin action in response to abiotic and biotic stresses in plants. Plant Cell Environ. 42, 998–1018. doi: 10.1111/pce.13494
Curtis, P. J., Cross, B. E. (1954). Gibberellic acid – a new metabolite from the culture filtrates of Gibberella fujikuroi. Chem. Industry 1066, 1–8.
Cvetkovska, M., Zhang, X., Vakulenko, G., Benzaquen, S., Szyszka-Mroz, B., Malczewski, N., et al. (2022). A constitutive stress response is a result of low temperature growth in the Antarctic green algaChlamydomonassp.UWO241. Plant Cell Environ. 45, 156–177. doi: 10.1111/pce.14203
D’Amato, R., Del Buono, D. (2021). Use of a biostimulant to mitigate salt stress in maize plants. Agronomy 11, 1755. doi: 10.3390/agronomy11091755
Delgado-Vargas, F., Vega-Álvarez, M., Landeros Sánchez, A., López-Angulo, G., Salazar-Salas, N. Y., Quintero-Soto, M. F., et al. (2022). Metabolic changes associated with chilling injury tolerance in tomato fruit with hot water pretreatment. J. Food Biochem. 46, e14056. doi: 10.1111/jfbc.14056
Demidchik, V. (2017). “Reactive oxygen species and their role in plant oxidative stress,” in CABI eBooks (Burlington, Canada: Plant Stress Physiology, Delve Publishing), 64–96. doi: 10.1079/9781780647296.0064
Deolu-Ajayi, A. O., van der Meer, I. M., van der Werf, A., Karlova, R. (2022). The power of seaweeds as plant biostimulants to boost crop production under abiotic stress. Plant Cell Environ. 45, 2537–2553. doi: 10.1111/pce.14391
Desoky, E.-S. M., Elrys, A. S., Mansour, E., Eid, R. S. M., Selem, E., Rady, M. M., et al. (2021). Application of biostimulants promotes growth and productivity by fortifying the antioxidant machinery and suppressing oxidative stress in faba bean under various abiotic stresses. Sci Hortic. 288, 110340. doi: 10.1016/j.scienta.2021.110340
Dhaliwal, S. S., Sharma, V., Shukla, A. K. (2022). Impact of micronutrients in mitigation of abiotic stresses in soils and plants – A progressive step toward crop security and nutritional quality. Adv. Agron. 173, 1–78. doi: 10.1016/bs.agron.2022.02.001
Ding, C. K., Wang, C., Gross, K. C., Smith, D. L. (2002). Jasmonate and salicylate induce the expression of pathogenesis-related-protein genes and increase resistancetolerance to chilling injury in tomato fruit. Planta 214, 895–901. doi: 10.1007/s00425-001-0698-9
Di Sario, L., Boeri, P., Matus, J. T., Pizzio, G. A. (2025). Plant biostimulants to enhance abiotic stress resilience in crops. Int. J. Mol. Sci. 26, 1129. doi: 10.3390/ijms26031129
Dreyer, A., Dietz, K.-J. (2018). Reactive oxygen species and the redox-regulatory network in cold stress acclimation. Antioxidants 7, 169. doi: 10.3390/antiox7110169
du Jardin, P. (2015). Plant biostimulants: Definition, concept, main categories and regulation. Sci Hortic. 196, 3–14. doi: 10.1016/j.scienta.2015.09.021
Elansary, H. O., Mahmoud, E. A., El-Ansary, D. O., Mattar, M. A. (2019). Effects of water stress and modern biostimulants on growth and quality characteristics of mint. Agronomy 10, 6. doi: 10.3390/agronomy10010006
Elbasiouny, H., El-Ramady, H., Elbehiry, F., Rajput, V. D., Minkina, T., Mandzhieva, S. (2022). Plant nutrition under climate change and soil carbon sequestration. Sustainability 14, 914. doi: 10.3390/su14020914
EU (2019). European Union. Regulation (EU) 2019/1009 of the European Parliament and of the Council of 5 June 2019 laying down rules on the making available on the market of EU fertilizing products and amending Regulations (EC) No 1069/2009 and (EC) No 1107/2009 and repealing Regulation (EC) No 2003/2003 (Text with EEA relevance). Available online at: https://eur-lex.europa.eu/eli/reg/2019/1009/oj/eng (Accessed December 3, 2024).
Fan, J., Hu, Z., Xie, Y., Chan, Z., Chen, K., Amombo, E., et al. (2015). Alleviation of cold damage to photosystem II and metabolisms by melatonin in Bermudagrass. Front. Plant Sci. 6. doi: 10.3389/fpls.2015.00925
FAO (2015). “Food and agriculture organization of the united nations,” in The state of food and agriculture 2015. Available at: https://www.fao.org/family-farming/detail/en/c/336805/ (Accessed November 10, 2025).
FAO (2023). “Food and agriculture organization of the united nations,” in The state of food and agriculture 2023. Available at: https://openknowledge.fao.org/items/1516eb79-8b43-400e-b3cb-130fd70853b0 (Accessed November 10, 2025).
Farooq, M., Aziz, T., Hussain, M., Rehman, H., Jabran, K., Khan, M. B. (2008). Glycinebetaine improves chilling tolerance in hybrid maize. J. Agron. Crop Sci. 194, 152–160. doi: 10.1111/j.1439-037x.2008.00295.x
Fleming, T. R., Fleming, C. C., Levy, C. C. B., Repiso, C., Hennequart, F., Nolasco, J. B., et al. (2019). Biostimulants enhance growth and drought tolerance in Arabidopsis thaliana and exhibit chemical priming action. Ann. Appl. Biol. 174, 153–165. doi: 10.1111/aab.12482
Fleurence, J. (2022). “Biostimulant potential of seaweed extracts derived from laminaria and ascophyllum nodosum,” in Plant life and environment dynamics (Springer Nature, Singapore), 31–49.
Fortune Business Insights (2022). Biostimulants market size, share & COVID-19 impact analysis, by source (Microbial and non-microbial), by active ingredient (Seaweed extracts, humic substances, vitamins & Amino acids, microbial amendments, and others), by application (Foliar application, soil treatment, and seed treatment), by crop (Row crops, fruit and vegetables, turf & Ornamentals, and others), and regional forecast 2022-2029 (Pune, India: Fortune Business Insights Pvt. Ltd., India).
Francesca, S., Arena, C., Hay Mele, B., Schettini, C., Ambrosino, P., Barone, A., et al. (2020). The use of a plant-based biostimulant improves plant performances and fruit quality in tomato plants grown at elevated temperatures. Agronomy 10, 363. doi: 10.3390/agronomy10030363
Franzoni, G., Cocetta, G., Prinsi, B., Ferrante, A., Espen, L. (2022). Biostimulants on crops: Their impact under abiotic stress conditions. Horticulturae 8, 189. doi: 10.3390/horticulturae8030189
Fürtauer, L., Weiszmann, J., Weckwerth, W., Nägele, T. (2019). Dynamics of plant metabolism during cold acclimation. Int. J. Mol. Sci. 20, 5411. doi: 10.3390/ijms20215411
Furumoto, T., Yamaoka, S., Kohchi, T., Motose, H., Takahashi, T. (2024). Thermospermine is an evolutionarily ancestral phytohormone required for organ development and stress responses in Marchantia polymorpha. Plant Cell Physiol. 65, 460–471. doi: 10.1093/pcp/pcae002
Galloway, A. F., Pedersen, M. J., Merry, B., Marcus, S. E., Blacker, J., Benning, L. G., et al. (2018). Xyloglucan is released by plants and promotes soil particle aggregation. New Phytol. 217, 1128–1136. doi: 10.1111/nph.14897
Garg, S., Nain, P., Kumar, A., Joshi, S., Punetha, H., Sharma, P. K., et al. (2024). Next generation plant biostimulants & genome sequencing strategies for sustainable agriculture development. Front. Microbiol. 15. doi: 10.3389/fmicb.2024.1439561
Gavelienė, V., Pakalniškytė, L., Novickienė, L. (2014). Regulation of proline and ethylene levels in rape seedlings for freezing tolerance. Open Life Sci. 9, 1099–1107. doi: 10.2478/s11535-014-0340-z
Ghassemi, S., Ghassemi-Golezani, K., Salmasi, S. Z. (2019). Changes in antioxidant enzymes activities and physiological traits of ajowan in response to water stress and hormonal application. Sci Hortic. 246, 957–964. doi: 10.1016/j.scienta.2018.11.086
Gholizadeh, F., Mirzaghaderi, G., Marashi, S. H., Janda, T. (2024). Polyamines-Mediated amelioration of cold treatment in wheat: Insights from morpho-physiological and biochemical features and PAO genes expression analyses. Plant Stress 11, 100402. doi: 10.1016/j.stress.2024.100402
Ghorbanpour, A., Salimi, A., Ghanbary, M. A. T., Pirdashti, H., Dehestani, A. (2018). The effect of Trichoderma harzianum in mitigating low temperature stress in tomato (Solanum lycopersicum L.) plants. Sci Hortic. 230, 134–141. doi: 10.1016/j.scienta.2017.11.028
Giri, J. (2011). Glycinebetaine and abiotic stress tolerance in plants. Plant Signaling Behav. 6, 1746–1175. doi: 10.4161/psb.6.11.17801
Gitau, M. M., Farkas, A., Ördög, V., Maróti, G. (2022). Evaluation of the biostimulant effects of two Chlorophyta microalgae on tomato (Solanum lycopersicum). J. Cleaner Prod 364, 132689. doi: 10.1016/j.jclepro.2022.132689
Góes, G. B., Vilvert, J. C., Araújo, N. O., Medeiros, J. F., Aroucha, E. M. M. (2021). Application methods of biostimulants affect the production and postharvest conservation of yellow melon. Biosci J. 37, e37075. doi: 10.14393/bj-v37n0a2021-53682
Gomes, G. L. B., Scortecci, K. C. (2021). Auxin and its role in plant development: Structure, signalling, regulation and response mechanisms. Plant Biol. 23, 894–904. doi: 10.1111/plb.13303
González-Hernández, A. I., Scalschi, L., Vicedo, B., Marcos-Barbero, E. L., Morcuende, R., Camañes, G. (2022). Putrescine: A key metabolite involved in plant development, tolerance and resistance responses to stress. Int. J. Mol. Sci. 23, 2971. doi: 10.3390/ijms23062971
González-Pérez, L., Páez-Watson, T., Álvarez-Suarez, J. M., Obando-Rojas, M. C., Bonifaz-Arcos, E., Viteri, G., et al. (2018). Application of exogenous xyloglucan oligosaccharides affects molecular responses to salt stress in Arabidopsis thaliana seedlings. J. Soil Sci. Plant Nutr. 18 (4), 1187–1205. doi: 10.4067/s0718-95162018005003301
Gramazio, P., Takayama, M., Ezura, H. (2020). Challenges and prospects of new plant breeding techniques for GABA improvement in crops: Tomato as an example. Front. Plant Sci. 11. doi: 10.3389/fpls.2020.577980
Graziani, G., Cirillo, A., Giannini, P., Conti, S., El-Nakhel, C., Rouphael, Y., et al. (2022). Biostimulants improve plant growth and bioactive compounds of young Olive trees under abiotic stress conditions. Agriculture 12, 227. doi: 10.3390/agriculture12020227
Hai, X., Mi, J., Zhao, B., Zhang, B., Zhao, Z., Liu, J. (2022). Foliar application of spermidine reduced the negative effects of salt stress on oat seedlings. Front. Plant Sci. 13. doi: 10.3389/fpls.2022.846280
Hasan, M. M., Skalicky, M., Jahan, M. S., Hossain, M. N., Anwar, Z., Nie, Z.-F., et al. (2021). Spermine: Its emerging role in regulating drought stress responses in plants. Cells (Basel Switzerland) 10, 261. doi: 10.3390/cells10020261
Hassan, S. M., Ashour, M., Sakai, N., Zhang, L., Hassanien, H. A., Gaber, A., et al. (2021). Impact of seaweed liquid extract biostimulant on growth, yield, and chemical composition of cucumber (Cucumis sativus). Agriculture 11, 320. doi: 10.3390/agriculture11040320
Hayat, S., Ahmad, H., Ali, M., Ren, K., Cheng, Z. (2018). Aqueous garlic extract stimulates growth and antioxidant enzymes activity of tomato (Solanum lycopersicum). Sci Hortic. 240, 139–146. doi: 10.1016/j.scienta.2018.06.011
He, M., Zhou, J., Lyu, D., Xu, G., Qin, S. (2024). Exogenous spermidine alleviated low-temperature damage by affecting polyamine metabolism and antioxidant levels in apples. Plants 13, 1–14. doi: 10.3390/plants13081100
Hellequin, E., Monard, C., Chorin, M., Le Bris, N., Daburon, V., Klarzynski, O., et al. (2020). Responses of active soil microorganisms facing to a soil biostimulant input compared to plant legacy effects. Sci. Rep. 10, 13727. doi: 10.1038/s41598-020-70695-7
Horváth, E. (2002). Differences between the catalase isozymes of maize (Zea mays L.) in respect of inhibition by various phenolic compounds. Acta Biol Szegediensis 46, 33–34.
Huang, J., Zhao, X., Bürger, M., Chory, J., Wang, X. (2023). The role of ethylene in plant temperature stress response. Trends Plant Sci. 28, 808–824. doi: 10.1016/j.tplants.2023.03.001
Irani, H., ValizadehKaji, B., Naeini, M. R. (2021). Biostimulant-induced drought tolerance in grapevine is associated with physiological and biochemical changes. Chem. Biol. Technol. Agric. 8, 1–13. doi: 10.1186/s40538-020-00200-9
Jędrszczyk, E., Kopeć, A., Bucki, P., Ambroszczyk, A. M., Skowera, B. (2018). The enhancing effect of plants growth biostimulants in garlic cultivation on the chemical composition and level of bioactive compounds in the garlic leaves, stems and bulbs. Notulae Botan Horti Agrobot Cluj-Napoca 47, 81–91. doi: 10.15835/nbha47111074
Jahed, K. R., Saini, A. K., Sherif, S. M. (2023). Coping with the cold: Unveiling cryoprotectants, molecular signaling pathways, and strategies for cold stress resilience. Front. Plant Sci. 14. doi: 10.3389/fpls.2023.1246093
Jańczak-Pieniążek, M., Migut, D., Piechowiak, T., Buczek, J., Balawejder, M. (2021). The effect of exogenous application of quercetin derivative solutions on the course of physiological and biochemical processes in wheat seedlings. Int. J. Mol. Sci. 22, 6882. doi: 10.3390/ijms22136882
Janda, T., Szalai, G., Tari, I., Páldi, E. (1999). Hydroponic treatment with salicylic acid decreases the effects of chilling injury in maize (Zea mays L.) plants. Planta 208, 175–180. doi: 10.1007/s004250050547
Jasrotia, S., Jasrotia, R. (2022). “Role of ethylene in combating biotic stress,” in Em Ethylene in Plant Biology (Nova Jersey, EUA: John Wiley & Sons Ltd), 388–397. doi: 10.1002/9781119744719.ch18
Jha, Y., Mohamed, H. I. (2023). Inoculation with Lysinibacillus fusiformis strain YJ4 and Lysinibacillus sphaericus strain YJ5 alleviates the effects of cold stress in maize plants. Gesunde Pflanzen 75, 77–95. doi: 10.1007/s10343-022-00666-7
Jiang, W., Pan, R., Wu, C., Xu, L., Abdelaziz, M. E., Oelmüller, R., et al. (2020). Piriformospora indica enhances freezing tolerance and post-thaw recovery in Arabidopsis by stimulating the expression of CBF genes. Plant Signaling Behav. 15, 1745472. doi: 10.1080/15592324.2020.1745472
Jiménez-Arias, D., García-MaChado, F. J., Morales-Sierra, S., García-García, A. L., Herrera, A. J., Valdés, F., et al. (2021). A beginner’s guide to osmoprotection by biostimulants. Plants 10, 363. doi: 10.3390/plants10020363
Junaid, M. B., Alderfasi, A. A., Afzal, I., Wajid, H. A., Mahmood, A. (2019). Evaluation of different strategies for induction of chilling tolerance in spring maize using Moringa leaf extracts. Cercetari Agronom Moldova 52, 228–247. doi: 10.46909/cerce-2019-0023
Kabir, S. F., Rahman, A., Yeasmin, F., Sultana, S., Masud, R. A., Kanak, N. A., et al. (2022). “Occurrence, distribution, and structure of natural polysaccharides,” in Em radiation-processed polysaccharides (Amsterdam, Holanda: Elsevier Inc.), 1–27.
Kakehi, J.-I., Kuwashiro, Y., Niitsu, M., Takahashi, T. (2008). Thermospermine is required for stem elongation in arabidopsis thaliana. Plant Cell Physiol. 49, 1342–1349. doi: 10.1093/pcp/pcn109
Kalasariya, H. S., Patel, N. B., Jain, A., Prajapati, N. D., Patel, R. N. (2021). Marine Macroalgae: Plant growth stimulators. EPRA Int. J. Res. Dev. (IJRD) 6 (1), 110–118. doi: 10.36713/epra6093
Kamel, H. M. (2014). Impact of garlic oil, seaweed extract and imazalil on keeping quality of valencia orange fruits during cold storage. J. Hortic. Sci. Ornamental Plants 6, 116–125. doi: 10.5829/idosi.jhsop.2014.6.3.1145
Khan, A. R., Ali, Q., Ayaz, M., Bilal, M. S., Sheikh, T. M. M., Gu, Q., et al. (2023). Plant–microbes interaction: Exploring the impact of cold-tolerant Bacillus strains RJGP41 and GBAC46 volatiles on tomato growth promotion through different mechanisms. Biology 12, 940. doi: 10.3390/biology12070940
Khan, M. I. R., Fatma, M., Per, T. S., Anjum, N. A., Khan, N. A. (2015). Salicylic acid-induced abiotic stress tolerance and underlying mechanisms in plants. Front. Plant Sci. 6. doi: 10.3389/fpls.2015.00462
Khursheed, A., Rather, M. A., Jain, V., Wani, A. R., Rasool, S., Nazir, R., et al. (2022). Plant based natural products as potential ecofriendly and safer biopesticides: A comprehensive overview of their advantages over conventional pesticides, limitations and regulatory aspects. Microb Pathogen 173, 105854. doi: 10.1016/j.micpath.2022.105854
Kołodziejczyk, I., Dzitko, K., Szewczyk, R., Posmyk, M. M. (2016). Exogenous melatonin improves corn (Zea mays L.) embryo proteome in seeds subjected to chilling stress. J. Plant Physiol. 193, 47–56. doi: 10.1016/j.jplph.2016.01.012
Körner, C. (2021). “The climate plants experience,” in Alpine Plant Life: Functional plant ecology of high mountain ecosystems. (Switzerland: Alpine Plant Life, Springer Nature), 65–88. doi: 10.1007/978-3-030-59538-8_4
Kumar, G., Nanda, S., Singh, S. K., Kumar, S., Singh, D., Singh, B. N., et al. (2024). Seaweed extracts: Enhancing plant resilience to biotic and abiotic stresses. Front. Mar. Sci. 11. doi: 10.3389/fmars.2024.1457500
Kunkel, B. N., Ludwig-Müller, J., Ma, K.-W. (2024). Editorial: The role of auxin in plant-microbe interactions. Front. Plant Sci. 15. doi: 10.3389/fpls.2024.1500649
Li, Z., Ahammed, G. J. (2023). Plant stress response and adaptation via anthocyanins: A review. Plant Stress 10, 100230. doi: 10.1016/j.stress.2023.100230
Li, X., Brestic, M., Tan, D.-X., Zivcak, M., Zhu, X., Liu, S., et al. (2018). Melatonin alleviates low PS I-limited carbon assimilation under elevated CO2 and enhances the cold tolerance of offspring in chlorophyll b-deficient mutant wheat. J. Pineal Res. 64, e12453. doi: 10.1111/jpi.12453
Li, Z., Cheng, B., Zhao, Y., Luo, L., Zhang, Y., Feng, G., et al. (2022). Metabolic regulation and lipidomic remodeling in relation to spermidine-induced stress tolerance to high temperature in plants. Int. J. Mol. Sci. 23, 12247. doi: 10.3390/ijms232012247
Li, P., Tian, J., Guo, C., Luo, S., Li, J. (2021). Interaction of gibberellin and other hormones in almond anthers: Phenotypic and physiological changes and transcriptomic reprogramming. Horticulr Res. 8, 1–16. doi: 10.1038/s41438-021-00527-w
Li, J., Yang, Y., Sun, K., Chen, Y., Chen, X., Li, X. (2019). Exogenous melatonin enhances cold, salt and drought stress tolerance by improving antioxidant defense in tea plant (Camellia sinensis (L.) O. Kuntze). Molecules 24, 1826. doi: 10.3390/molecules24091826
Lichtenthaler, H. K., Rohmer, M., Schwender, J. (1997). Two independent biochemical pathways for isopentenyl diphosphate and isoprenoid biosynthesis in higher plants. Physiol Plant 101, 643–652. doi: 10.1111/j.1399-3054.1997.tb01049.x
Liu, X., Lu, X., Yang, S., Liu, Y., Wang, W., Wei, X., et al. (2022). Role of exogenous abscisic acid in freezing tolerance of mangrove Kandelia obovata under natural frost condition at near 32°N. BMC Plant Biol. 22, 1–13. doi: 10.1186/s12870-022-03990-2
Liu, M., Shan, Q., Ding, E., Gu, T., Gong, B. (2023). Karrik in increases tomato cold tolerance via strigolactone and the abscisic acid signaling network. Plant Sci. 332, 111720. doi: 10.1016/j.plantsci.2023.111720
Macias-Benitez, S., Garcia-Martinez, A. M., Caballero Jimenez, P., Gonzalez, J. M., Tejada Moral, M., Parrado Rubio, J. (2020). Rhizospheric organic acids as biostimulants: Monitoring feedbacks on soil microorganisms and biochemical properties. Front. Plant Sci. 11. doi: 10.3389/fpls.2020.00633
Madende, M., Hayes, M. (2020). Fish by-product use as biostimulants: An overview of the current state of the art, including relevant legislation and regulations within the EU and USA. Molecules 25, 1122. doi: 10.3390/molecules25051122
Mahajan, S., Tuteja, N. (2005). Cold, salinity and drought stresses: An overview. Arch. Biochem. Biophys 444, 139–158. doi: 10.1016/j.abb.2005.10.018
Mamede, M., Cotas, J., Bahcevandziev, K., Pereira, L. (2023). Seaweed polysaccharides in agriculture: A next step towards sustainability. Appl. Sci. (Basel Switzerland) 13, 6594. doi: 10.3390/app13116594
Mannino, G., Campobenedetto, C., Vigliante, I., Contartese, V., Gentile, C., Bertea, C. M. (2020). The application of a plant biostimulant based on seaweed and yeast extract improved tomato fruit development and quality. Biomolecules 10, 1662. doi: 10.3390/biom10121662
Marcińska, I., Czyczyło-Mysza, I., Skrzypek, E., Grzesiak, M., Janowiak, F., Filek, M., et al. (2013). Alleviation of osmotic stress effects by exogenous application of salicylic or abscisic acid on wheat seedlings. Int. J. Mol. Sci. 14, 13171–13193. doi: 10.3390/ijms140713171
Marfà, O., Cáceres, R., Polo, J., Ródenas, J. (2009). Animal protein hydrolysate as a biostimulant for transplanted strawberry plants subjected to cold stress. Acta Hortic. 842, 315–318. doi: 10.17660/actahortic.2009.842.57
Martínez-Lorente, S. E., Martí-Guillén, J. M., Pedreño, M.Á., Almagro, L., Sabater-Jara, A. B. (2024). Higher Plant-Derived Biostimulants: Mechanisms of action and their role in mitigating plant abiotic stress. Antioxidants 13, 318. doi: 10.3390/antiox13030318
Mastouri, F., Björkman, T., Harman, G. E. (2010). Seed treatment with Trichoderma harzianum alleviates biotic, abiotic, and physiological stresses in germinating seeds and seedlings. Phytopathology 100, 1213–1221. doi: 10.1094/PHYTO-03-10-0091
Matsumiya, Y., Kubo, M. (2011). “Soybean peptide: Novel plant growth promoting peptide from soybean,” in Soybean and Nutrition (London, United Kingdom: IntechOpen Limited). doi: 10.5772/19132
Meena, M., Divyanshu, K., Kumar, S., Swapnil, P., Zehra, A., Shukla, V., et al. (2019). Regulation of L-proline biosynthesis, signal transduction, transport, accumulation and its vital role in plants during variable environmental conditions. Heliyon 5, 1–20. doi: 10.1016/j.heliyon.2019.e02952
Monterisi, S., Garcia-Perez, P., Buffagni, V., Zuluaga, M. Y. A., Ciriello, M., Formisano, L., et al. (2024a). Unravelling the biostimulant activity of a protein hydrolysate in lettuce plants under optimal and low N availability: a multi-omics approach. Physiol Plant 176, 1–23. doi: 10.1111/ppl.14357
Monterisi, S., Zhang, L., Garcia-Perez, P., Zuluaga, M. Y. A., Ciriello, M., El-Nakhel, C., et al. (2024b). Integrated multi-omic approach reveals the effect of a Graminaceae-derived biostimulant and its lighter fraction on salt-stressed lettuce plants. Sci. Rep. 14, 1–25. doi: 10.1038/s41598-024-61576-4
Mughunth, R. J., Velmurugan, S., Mohanalakshmi, M., Vanitha, K. (2024). A review of seaweed extract’s potential as a biostimulant to enhance growth and mitigate stress in horticulture crops. Sci Hortic. 334, 113312. doi: 10.1016/j.scienta.2024.113312
Munaro, D., Nunes, A., Schmitz, C., Bauer, C., Coelho, D. S., Oliveira, E. R., et al. (2021). “Metabolites produced by macro- and microalgae as plant biostimulants,” in Studies in natural products chemistry (Amsterdam, Holanda: Elsevier Inc.), 87–120. doi: 10.1016/B978-0-323-91095-8.00011-8
Nadarajah, K. K. (2020). ROS homeostasis in abiotic stress tolerance in plants. Int. J. Mol. Sci. 21, 5208. doi: 10.3390/ijms21155208
Nair, P., Kandasamy, S., Zhang, J., Ji, X., Kirby, C., Benkel, B., et al. (2012). Transcriptional and metabolomic analysis of Ascophyllum nodosum mediated freezing tolerance in Arabidopsis thaliana. BMC Genomics 13, 1–23. doi: 10.1186/1471-2164-13-643
Navarro-López, E., Ruíz-Nieto, A., Ferreira, A., Acién, F. G., Gouveia, L. (2020). Biostimulant potential of scenedesmus obliquus grown in brewery wastewater. Molecules 25, 664. doi: 10.3390/molecules25030664
Navarro-Morillo, I., Navarro-León, E., Atero-Calvo, S., Rios, J. J., Ruiz, J. M., Blasco, B. (2024). Biostimulant-induced mitigation of cold and drought stresses in zucchini plants. Sci Hortic. 331, 113114. doi: 10.1016/j.scienta.2024.113114
Nunes, A., Azevedo, G. Z., de Souza Dutra, F., dos Santos, B. R., Schneider, A. R., Oliveira, E. R., et al. (2024). Uses and applications of the red seaweed Kappaphycus alvarezii: a systematic review. J. Appl. Phycol 36, 3409–3450. doi: 10.1007/s10811-024-03270-6
Pacifico, D., Lanzanova, C., Pagnotta, E., Bassolino, L., Mastrangelo, A. M., Marone, D., et al. (2021). Sustainable use of bioactive compounds from Solanum tuberosum and Brassicaceae wastes and by-products for crop protection—A review. Mol (Basel Switzerland) 26, 2174. doi: 10.3390/molecules26082174
Pandey, A., Yarzábal, L. A. (2019). Bioprospecting cold-adapted plant growth promoting microorganisms from mountain environments. Appl. Microbiol. Biotechnol. 103, 643–657. doi: 10.1007/s00253-018-9515-2
Patel, K., Agarwal, P., Agarwal, P. K. (2018). Kappaphycus alvarezii sap mitigates abiotic-induced stress in Triticum durum by modulating metabolic coordination and improves growth and yield. J. Appl. Phycol 30, 2659–2673. doi: 10.1007/s10811-018-1423-4
Polo, J., Barroso, R., Ródenas, J., Azcón-Bieto, J., Cáceres, R., Marfà, O. (2006). Porcine hemoglobin hydrolysate as a biostimulant for lettuce plants subjected to conditions of thermal stress. HortTechnology 16, 483–487. doi: 10.21273/horttech.16.3.0483
Potijun, S., Yaisamlee, C., Sirikhachornkit, A. (2021). Pigment production under cold stress in the green microalga Chlamydomonas reinhardtii. Agriculture 11, 564. doi: 10.3390/agriculture11060564
Poveda, J., Díez-Méndez, A. (2023). Use of elicitors from macroalgae and microalgae in the management of pests and diseases in agriculture. Phytoparasitica 51, 667–701. doi: 10.1007/s12600-022-01009-y
Poveda, J., Zabalgogeazcoa, I., Soengas, P., Rodríguez, V. M., Cartea, M. E., Abilleira, R., et al. (2020). Brassica oleracea var. acephala (kale) improvement by biological activity of root endophytic fungi. Sci. Rep. 10, 20224. doi: 10.1038/s41598-020-77215-7
Prerostova, S., Černý, M., Dobrev, P. I., Motyka, V., Hluskova, L., Zupkova, B., et al. (2021). Light regulates the Cytokinin-Dependent cold stress responses in Arabidopsis. Front. Plant Sci. 11. doi: 10.3389/fpls.2020.608711
Promyou, S., Ketsa, S., van Doorn, W. G. (2012). Salicylic acid alleviates chilling injury in anthurium (Anthurium andraeanum L.) flowers. Postharvest Biol. Technol. 64, 104–110. doi: 10.1016/j.postharvbio.2011.10.002
Puglia, D., Pezzolla, D., Gigliotti, G., Torre, L., Bartucca, M. L., Del Buono, D. (2021). The opportunity of valorizing agricultural waste, through its conversion into biostimulants, biofertilizers, and biopolymers. Sustainability 13, 2710. doi: 10.3390/su13052710
Puzanskiy, R. K., Yemelyanov, V. V., Shishova, M. F. (2018). Metabolomics as a modern approach for the investigation of potato plant adaptation to biotic and abiotic stress factors. Sel’skokhozyaistvennaya Biol. 53, 15–28. doi: 10.15389/agrobiology.2018.1.15eng
Rademacher, W. (2016). “Chemical regulators of gibberellin status and their application in plant production,” in Annual plant reviews, vol. 49. (Nova Jersey, EUA: John Wiley & Sons Ltd.), 359–404. doi: 10.1002/9781119210436.ch12
Rahman, A. (2013). Auxin: a regulator of cold stress response. Physiol Plant 147, 28–35. doi: 10.1111/j.1399-3054.2012.01617.x
Rakkammal, K., Maharajan, T., Ceasar, S. A., Ramesh, M. (2023). Biostimulants and their role in improving plant growth under drought and salinity. Cereal Res. Commun. 51, 61–74. doi: 10.1007/s42976-022-00299-6
Rayirath, P., Benkel, B., Mark Hodges, D., Allan-Wojtas, P., MacKinnon, S., Critchley, A. T., et al. (2009). Lipophilic components of the brown seaweed, Ascophyllum nodosum, enhance freezing tolerance in Arabidopsis thaliana. Planta 230, 135–147. doi: 10.1007/s00425-009-0920-8
Ritonga, F. N., Chen, S. (2020). Physiological and molecular mechanism involved in cold stress tolerance in plants. Plants 9, 560. doi: 10.3390/plants9050560
Riyazuddin, R., Singh, K., Iqbal, N., Nisha, N., Rani, A., Kumar, M., et al. (2023). Iodine: an emerging biostimulant of growth and stress responses in plants. Plant Soil 486, 119–133. doi: 10.1007/s11104-022-05750-5
Ronga, D., Biazzi, E., Parati, K., Carminati, D., Carminati, E., Tava, A. (2019). Microalgal biostimulants and biofertilisers in crop productions. Agronomy 9, 192. doi: 10.3390/agronomy9040192
Rouphael, Y., Colla, G. (2020). Editorial: Biostimulants in agriculture. Front. Plant Sci. 11. doi: 10.3389/fpls.2020.00040
Rouphael, Y., Giordano, M., Cardarelli, M., Cozzolino, E., Mori, M., Kyriacou, M., et al. (2018). Plant- and seaweed-based extracts increase yield but differentially modulate nutritional quality of greenhouse spinach through biostimulant action. Agronomy 8, 126. doi: 10.3390/agronomy8070126
Roychoudhury, A., Aftab, T. (2021). Phytohormones, plant growth regulators and signaling molecules: Cross-talk and stress responses. Plant Cell Rep. 40, 1301–1303. doi: 10.1007/s00299-021-02755-9
Rubio, S., Pérez, F. J. (2019). ABA and its signaling pathway are involved in the cold acclimation and deacclimation of grapevine buds. Sci Hortic. 256, 108565. doi: 10.1016/j.scienta.2019.108565
Rummukainen, M. (2013). Climate change: Changing means and changing extremes. Clim Change 121, 3–13. doi: 10.1007/s10584-013-0888-z
Rydeen, A. E., Brustad, E. M., Pielak, G. J. (2018). Osmolytes and protein–protein interactions. J. Am. Chem. Soc. 140, 7441–7444. doi: 10.1021/jacs.8b03903
Sakakibara, H. (2021). Cytokinin biosynthesis and transport for systemic nitrogen signaling. Plant J. Cell Mol. Biol. 105, 421–430. doi: 10.1111/tpj.15011
Saleem, M., Fariduddin, Q., Janda, T. (2021). Multifaceted role of salicylic acid in combating cold stress in plants: A review. J. Plant Growth Regul. 40, 464–485. doi: 10.1007/s00344-020-10152-x
Sangiorgio, D., Cellini, A., Donati, I., Pastore, C., Onofrietti, C., Spinelli, F. (2020). Facing climate change: Application of microbial biostimulants to mitigate stress in horticultural crops. Agronomy 10, 794. doi: 10.3390/agronomy10060794
Santini, G., Rodolfi, L., Biondi, N., Sampietro, G., Tredici, M. R. (2022). Effects of cyanobacterial-based biostimulants on plant growth and development: a case study on basil (Ocimum basilicum L.). J. Appl. Phycol 34, 2063–2073. doi: 10.1007/s10811-022-02781-4
Shahbaz, M., Sinha, A. (2019). Environmental Kuznets curve for CO2 emissions: a literature survey. J. Econ Stud. (Glasgow Scotland) 46, 106–168. doi: 10.1108/jes-09-2017-0249
Shahgedanova, M. (2021). “Climate change and melting glaciers,” in The impacts of climate change (Amsterdam, Holanda: Elsevier Inc.), 53–84. doi: 10.1016/B978-0-12-822373-4.00007-0
Shahrajabian, M. H., Chaski, C., Polyzos, N., Petropoulos, S. A. (2021). Biostimulants application: A low input cropping management tool for sustainable farming of vegetables. Biomolecules 11, 698. doi: 10.3390/biom11050698
Shao, J., Huang, K., Batool, M., Idrees, F., Afzal, R., Haroon, M., et al. (2022). Versatile roles of polyamines in improving abiotic stress tolerance of plants. Front. Plant Sci. 13. doi: 10.3389/fpls.2022.1003155
Sharma, H. S. S., Fleming, C., Selby, C., Rao, J. R., Martin, T. (2014). Plant biostimulants: a review on the processing of macroalgae and use of extracts for crop management to reduce abiotic and biotic stresses. J. Appl. Phycol 26, 465–490. doi: 10.1007/s10811-013-0101-9
Shehata, S. A., Abdelgawad, K. F., El-Mogy, M. M. (2017). Quality and shelf-life of onion bulbs influenced by biostimulants. Int. J. Vegetable Sci. 23, 362–371. doi: 10.1080/19315260.2017.1298170
Shi, F., Zhu, S., Li, H., Zhang, B., Liu, J., Song, F. (2024). Further enhancement of cold tolerance in rice seedlings by Piriformospora indica collaborating with plant growth-promoting bacteria: Evidence from the antioxidant defense, osmoregulation, photosynthesis, and related genes. Plant Stress 14, 100656. doi: 10.1016/j.stress.2024.100656
Shibasaki, A., Shimada, T., Kondo, S., Ohara, H., Ohkawa, K. (2023). Varietal tolerance of pear flower pollen to low-temperatures treatment during pollen development and damage inhibition by coffee extract. Horticulr J. 92, 151–161. doi: 10.2503/hortj.qh-030
Sible, C. N., Seebauer, J. R., Below, F. E. (2021). Plant biostimulants: A categorical review, their implications for row crop production, and relation to soil health indicators. Agron. (Basel Switzerland) 11, 1297. doi: 10.3390/agronomy11071297
Siboza, X. I., Bertling, I., Odindo, A. O. (2014). Salicylic acid and methyl jasmonate improve chilling tolerance in cold-stored lemon fruit (Citrus limon). J. Plant Physiol. 171, 1722–1731. doi: 10.1016/j.jplph.2014.05.012
Silva, T. I., Dias, M. G., Barbosa, L. B., Araújo, N. O., Ferreira, F. D., Grossi, J. A. S., et al. (2023). Spermine decreases ethylene and increases sugars and phenolic compounds in nasturtium flowers grown under drought and salt stress. Bragantia 82, 1–13. doi: 10.1590/1678-4499.20230041
Soda, M. N., Hayashi, Y., Takahashi, K., Kinoshita, T. (2022). Tryptophan synthase ß subunit 1 affects stomatal phenotypes in Arabidopsis thaliana. Front. Plant Sci. 13. doi: 10.3389/fpls.2022.1011360
Song, Z., Lai, X., Chen, H., Wang, L., Pang, X., Hao, Y., et al. (2022). ). Role of MaABI5-like in abscisic acid-induced cold tolerance of ‘Fenjiao’ banana fruit. Horticulr Res. 9, 1–16. doi: 10.1093/hr/uhac130
Song, W., Shao, H., Zheng, A., Zhao, L., Xu, Y. (2023). Advances in roles of salicylic acid in plant tolerance responses to biotic and abiotic stresses. Plants 12, 3475. doi: 10.3390/plants12193475
Soppelsa, S., Kelderer, M., Casera, C., Bassi, M., Robatscher, P., Matteazzi, A., et al. (2019). Foliar applications of biostimulants promote growth, yield and fruit quality of strawberry plants grown under nutrient limitation. Agronomy 9, 483. doi: 10.3390/agronomy9090483
Souza, T. S., Magalhães, P. C., Castro, E. M., Albuquerque, P. E. P., Marabesi, M. A. (2013). The influence of ABA on water relation, photosynthesis parameters, and chlorophyll fluorescence under drought conditions in two maize hybrids with contrasting drought resistance. Acta Physiol Plant 35, 515–527. doi: 10.1007/s11738-012-1093-9
Souza, A. C., Zandonadi, D. B., Santos, M. P., Canellas, N. O. A., de Paula Soares, C., da Silva Irineu, L. E. S., et al. (2021). Acclimation with humic acids enhances maize and tomato tolerance to salinity. Chem. Biol. Technol. Agric. 8, 1–13. doi: 10.1186/s40538-021-00239-2
Tewari, V. P., Verma, R. K., Von Gadow, K. (2017). Climate change effects in the Western Himalayan ecosystems of India: Evidence and strategies. For. Ecosyst. 4, (13). doi: 10.1186/s40663-017-0100-4
Tiwari, M., Kumar, R., Subramanian, S., Doherty, C. J., Jagadish, S. V. K. (2023). Auxin–cytokinin interplay shapes root functionality under low-temperature stress. Trends Plant Sci. 28, 447–459. doi: 10.1016/j.tplants.2022.12.004
van Oosten, M. J., Pepe, O., De Pascale, S., Silletti, S., Maggio, A. (2017). The role of biostimulants and bioeffectors as alleviators of abiotic stress in crop plants. Chem. Biol. Technol. Agric. 4, 1–12. doi: 10.1186/s40538-017-0089-5
Vasconcelos, A. C. F. (2020). Amelioration of drought stress on plants under biostimulant sources (London, United Kingdom: IntechOpen Limited). doi: 10.5772/intechopen.91975
Vishwakarma, K., Upadhyay, N., Kumar, N., Yadav, G., Singh, J., Mishra, R. K., et al. (2017). Abscisic acid signaling and abiotic stress tolerance in plants: A review on current knowledge and future prospects. Front. Plant Sci. 08. doi: 10.3389/fpls.2017.00161
Wagner, A. L. S., Araniti, F., Ishii-Iwamoto, E. L., Abenavoli, M. R. (2022). Resveratrol exerts beneficial effects on the growth and metabolism of Lactuca sativa L. Plant Physiol. Biochem. 171, 26–37. doi: 10.1016/j.plaphy.2021.12.023
Wang, L., Chen, S., Kong, W., Li, S., Archbold, D. D. (2006). Salicylic acid pretreatment alleviates chilling injury and affects the antioxidant system and heat shock proteins of peaches during cold storage. Postharvest Biol. Technol. 41, 244–251. doi: 10.1016/j.postharvbio.2006.04.010
Wang, G.-J., Miao, W., Wang, J.-Y., Ma, D.-R., Li, J.-Q., Chen, W.-F. (2013). Effects of exogenous abscisic acid on antioxidant system in weedy and cultivated rice with different chilling sensitivity under chilling stress. J. Agron. Crop Sci. 199, 200–208. doi: 10.1111/jac.12004
Wang, Q. J., Sun, H., Dong, Q. L., Sun, T. Y., Jin, Z. X., Hao, Y. J., et al. (2016). The enhancement of tolerance to salt and cold stresses by modifying the redox state and salicylic acid content via the cytosolic malate dehydrogenase gene in transgenic apple plants. Plant Biotechnol. J. 14, 1986–1997. doi: 10.1111/pbi.12556
Wang, W., Wang, X., Lv, Z., Khanzada, A., Huang, M., Cai, J., et al. (2022). Effects of cold and salicylic acid priming on free proline and sucrose accumulation in winter wheat under freezing stress. J. Plant Growth Regul. 41, 2171–2184. doi: 10.1007/s00344-021-10412-4
Waqas, M. A., Khan, I., Akhter, M. J., Noor, M. A., Ashraf, U. (2017). Exogenous application of plant growth regulators (PGRs) induces chilling tolerance in short-duration hybrid maize. Environ. Sci. pollut. Res. Int. 24, 11459–11471. doi: 10.1007/s11356-017-8768-0
Warsi, M. K., Howladar, S. M., Alsharif, M. A. (2021). Regulon: An overview of plant abiotic stress transcriptional regulatory system and role in transgenic plants. Braz. J. Biol. 83, e245379. doi: 10.1590/1519-6984.245379
Xie, X., Gu, Y., Wang, W., Abbas, F., Qin, S., Fu, S., et al. (2023). Exogenous spermidine improved drought tolerance in Ilex verticillata seedlings. Front. Plant Sci. 14. doi: 10.3389/fpls.2023.1065208
Xing, W., Rajashekar, C. B. (2001). Glycine betaine involvement in freezing tolerance and water stress in Arabidopsis thaliana. Environ. Exp. Bot. 46, 21–28. doi: 10.1016/s0098-8472(01)00078-8
Xu, C., Leskovar, D. I. (2015). Effects of A. nodosum seaweed extracts on spinach growth, physiology and nutrition value under drought stress. Sci Hortic. 183, 39–47. doi: 10.1016/j.scienta.2014.12.004
Yakhin, O. I., Lubyanov, A. A., Yakhin, I. A., Brown, P. H. (2017). Biostimulants in plant science: A global perspective. Front. Plant Sci. 7. doi: 10.3389/fpls.2016.02049
Yalçın, S., Şükran Okudan, E., Karakaş, Ö., Önem, A. N., Sözgen Başkan, K. (2019). Identification and quantification of some phytohormones in seaweeds using UPLC-MS/MS. J. Liquid Chromatogr. Related Technol. 42, 475–484. doi: 10.1080/10826076.2019.1625374
Yildirim, E., Dursun, A., Kumlay, M. A., Güvenç, Í. (2013). The effects of different salt, biostimulant and temperature levels on seed germination of some vegetable species. Acta Agrobot 55, 75–80. doi: 10.5586/aa.2002.045
Zagoskina, N. V., Zubova, M. Y., Nechaeva, T. L., Kazantseva, V. V., Goncharuk, E. A., Katanskaya, V. M., et al. (2023). Polyphenols in plants: Structure, biosynthesis, abiotic stress regulation, and practical applications (review). Int. J. Mol. Sci. 24, 13874. doi: 10.3390/ijms241813874
Zamani-Babgohari, M., Critchley, A. T., Norrie, J., Prithiviraj, B. (2019). Increased freezing stress tolerance of Nicotiana tabacum L. cv. Bright Yellow-2 cell cultures with the medium addition of Ascophyllum nodosum (L.) Le Jolis extract. In Vitro Cellular & Developmental Biology. Plant: J. Tissue Culture Assoc. 55, 321–333. doi: 10.1007/s11627-019-09972-8
Zhang, Y., Dai, T., Liu, Y., Wang, J., Wang, Q., Zhu, W. (2022b). Effect of exogenous Glycine betaine on the germination of tomato seeds under cold stress. Int. J. Mol. Sci. 23, 10474. doi: 10.3390/ijms231810474
Zhang, Q., Li, D., Wang, Q., Song, X., Wang, Y., Yang, X., et al. (2021). Exogenous salicylic acid improves chilling tolerance in maize seedlings by improving plant growth and physiological characteristics. Agron. (Basel Switzerland) 11, 1341. doi: 10.3390/agronomy11071341
Zhang, X., Ran, W., Li, X., Zhang, J., Ye, M., Lin, S., et al. (2022a). Exogenous application of Gallic acid induces the direct defense of tea plant against Ectropis obliqua caterpillars. Front. Plant Sci. 13. doi: 10.3389/fpls.2022.833489
Zhang, X., Schmidt, R. E. (2000). Hormone-containing products’ impact on antioxidant status of tall fescue and creeping bentgrass subjected to drought. Crop Sci. 40, 1344–1349. doi: 10.2135/cropsci2000.4051344x
Zhang, Q., White, J. F. (2021). Bioprospecting desert plants for endophytic and biostimulant microbes: a strategy for enhancing agricultural production in a hotter, drier future. Biology 10, 961. doi: 10.3390/biology10100961
Ziogas, V., Bravos, N., Hussain, S. B. (2022). Preharvest foliar application of Si–Ca-based biostimulant affects postharvest quality and shelf-life of clementine mandarin (Citrus clementina Hort. Ex tan). Horticulturae 8, 996. doi: 10.3390/horticulturae811099
Zulfiqar, F., Ashraf, M. (2021). Bioregulators: Unlocking their potential role in regulation of the plant oxidative defense system. Plant Mol. Biol. 105, 11–41. doi: 10.1007/s11103-020-01077-w
Keywords: seaweed, plants, molecules, phytohormones, bioactives
Citation: Oliveira ER, Nunes A, Dutra FdS, Azevedo GZ, Schneider AR, Santos BRd, Munaro D, Moura S, Lima GPP and Maraschin M (2025) Marine and terrestrial biostimulant elicitors of tolerance to cold stress. Front. Plant Sci. 16:1569516. doi: 10.3389/fpls.2025.1569516
Received: 31 January 2025; Accepted: 20 March 2025;
Published: 08 April 2025.
Edited by:
Fernanda Fidalgo, University of Porto, PortugalReviewed by:
Manoj Kumar, Central Queensland University, AustraliaMafalda Pinto, University of Porto, Portugal
Maria Martins, University of Porto, Portugal
Copyright © 2025 Oliveira, Nunes, Dutra, Azevedo, Schneider, Santos, Munaro, Moura, Lima and Maraschin. This is an open-access article distributed under the terms of the Creative Commons Attribution License (CC BY). The use, distribution or reproduction in other forums is permitted, provided the original author(s) and the copyright owner(s) are credited and that the original publication in this journal is cited, in accordance with accepted academic practice. No use, distribution or reproduction is permitted which does not comply with these terms.
*Correspondence: Eva Regina Oliveira, Z2luYWdyb0BnbWFpbC5jb20=; Marcelo Maraschin, bXRvY3N5QGdtYWlsLmNvbQ==