- 1Biotechnology Laboratory, Agricultural Research Institute (ARI) Tarnab Peshawar, Peshawar, Pakistan
- 2Department of Chemical Sciences, University of Laki Marwat, Khyber Pakhtunkhwa, Pakistan
- 3The State Key Laboratory of Freshwater Ecology and Biotechnology, Institute of Hydrobiology, Chinese Academy of Sciences, Wuhan, Hubei, China
- 4The Key Laboratory of Aquatic Biodiversity and Conservation of Chinese Academy of Sciences, Institute of Hydrobiology, Chinese Academy of Sciences, Wuhan, Hubei, China
- 5Department of Botany, PMAS Arid Agriculture University, Rawalpindi, Pakistan
- 6Department of Cell Biology, and Nanoscience and Nanotechnology Ph.D. Program, Center for Research and Advanced Studies of the National Polytechnic Institute (CINVESTAV), Mexico City, Mexico
- 7Institute of Agricultural Sciences, Autonomous University of the State of Hidalgo, Hidalgo, Mexico
- 8Department of Clinical Pharmacy, College of Pharmacy, King Saud University, Riyadh, Saudi Arabia
Nanotechnology, particularly the use of iron oxide nanoparticles (IONPs), has gained significant attention in agricultural research due to its potential to enhance plant growth, development, and stress tolerance. However, the green synthesis of IONPs using plant extracts remains underexplored, especially in the context of agricultural applications. In this study, the green synthesis of IONPs using Moringa oleifera leaf extract is reported, with the extract serving as both a reducing and capping agent. The synthesized nanoparticles were characterized using scanning electron microscopy (SEM) and energy-dispersive X-ray spectroscopy (EDX), revealing spherical and polygonal shapes with an iron peak at 6.5-7.5 keV, consistent with the expected size and composition. These IONPs were incorporated into Murashige and Skoog (MS) medium to replace the conventional iron source and evaluate their effects on Stevia rebaudiana micropropagation. The results demonstrate that IONPs at lower concentrations (5.60 mg/L) significantly promoted early shoot and root initiation (5.2 and 5.3 days, respectively), while higher concentrations (11.20 mg/L and 22.40 mg/L) delayed growth initiation and inhibited development. Notably, 22.4 mg/L IONPs enhanced leaf growth (length: 3.20 cm, width: 1.90 cm), fresh weight (238.90 mg), and dry weight (20.67 mg), outperforming the positive control (FeSO4·7H2O). IONPs also increased the total phenolic content (TPC) and total flavonoid content (TFC) in plant tissues, with the highest values (4.54 mg GAE/g and 2.07 mg QAE/g) observed at 22.40 mg/L. The antioxidant capacity, measured by DPPH scavenging activity, was significantly enhanced, reaching 89.70%. Additionally, IONPs promoted the accumulation of diterpene glycosides, including stevioside (4.30 mg/g DW) and rebaudioside A (6.70 mg/g DW), especially at higher concentrations. These findings suggest that IONPs, particularly at 22.40 mg/L, are a promising and environmentally friendly alternative to traditional iron sources, offering enhanced plant growth, improved antioxidant defenses, and increased production of valuable secondary metabolites in S. rebaudiana.
Introduction
Stevia rebaudiana Bertoni, an Asteraceae family member, is famous for producing sweet diterpene glycosides (stevioside and rebaudioside A) (de Andrade et al., 2024). These compounds are non-toxic, non-mutagenic, and 200-300 times sweeter than sucrose (Lankarani et al., 2024). Stevia is widely used as a sugar substitute in the food and pharmaceutical industries because it is calorie-free and has no known adverse health effects. It is commonly used to sweeten soft drinks, confectionery, chewing gum, chocolates, baked goods, jams, and even certain medicines (Huang et al., 2024; Kazmi et al., 2019b). Despite its potential, large-scale production of stevia confronts challenges such as low seed viability and labour-intensive propagation by stem cuttings (Kazmi et al., 2019a). Additionally, the lack of established techniques for growing plants with optimal concentrations of steviol glycosides prevents it from being widely used, which leads to an imbalance between supply and demand in the market. To deal with these challenges, researchers are increasingly focussing on biotechnological approaches to enhance the production of stevia farming (Mahajan et al., 2024; Rukh et al., 2023). Among them, nanotechnology has emerged as an appealing option for agricultural practices. Nanomaterials have the potential to improve plant growth and production, offering an innovative solution to agricultural challenges (Shereen et al., 2024; Parveen et al., 2023; Ahmad et al., 2022; Ehsan et al., 2022). Over the past decade, the application of nanoparticles (NPs) in agriculture has demonstrated significant potential for enhancing crop yield and performance. Depending on their size, shape, and concentration, NPs can exert positive, negative, or neutral effects on plant physiology when absorbed through the roots or leaves (Thiruvengadam et al., 2024; Dey and Nandy, 2024).
In plant tissue culture, elicitation is a potential approach for increasing secondary metabolite production by altering growth conditions and adding particular elicitors (Anum et al., 2021; Murthy et al., 2024; Ghazal et al., 2024). While several studies have investigated the effects of conventional elicitors, such as salicylic acid and jasmonates, on the biosynthesis of steviol glycosides in Stevia, research on the effects of nanoparticles (NPs) on plant micropropagation and steviol glycoside production remains limited (Tavakoli et al., 2019; Moharramnejad et al., 2019; Alsafawi and Şahin, 2024). Research indicates that the effect of metal nanoparticles on Stevia varies with concentration (Ramezani et al., 2019; Khan et al., 2020; Lankarani et al., 2024). At low NP concentrations, plant growth is often stimulated, steviol glycoside synthesis is enhanced, and antioxidant activity is improved (Khan et al., 2020; Sichanova et al., 2022). However, at higher concentrations, NPs can induce phytotoxicity, reducing biomass and limiting metabolite accumulation (Biswas and Pal, 2024; Mathur et al., 2023).
In vitro studies have revealed that the specific effects of NPs on plant growth and metabolite production differ significantly depending on the type of NP employed, its concentration, and the environmental conditions under which the plants are propagated (Javed et al., 2022; Iqbal et al., 2022; Ali et al., 2019, 2023; Pathak et al., 2023). Silver nanoparticles, in particular, have been extensively investigated for their impact on plant growth and metabolite production in tissue cultures (Ali et al., 2019; Chung et al., 2018; Gupta et al., 2018; Natarajan et al., 2024; Ali Naghizadeh et al., 2024). Silicon nanoparticles have been shown to promote growth and secondary metabolites production in Mentha pulegium L. tissue culture (El-Naggar and Osman, 2024). Moringa oleifera, a member of the Moringaceae family, a small tree native to the sub-Himalayan regions of North West India, now found in many parts of the world, including Islands and South America. This plant has been a staple in local diets and holds significant cultural and medicinal value Known as the “miracle tree” due to its reputed health benefits. Moringa oleifera has been used for centuries to treat a wide range of ailments, including asthma, bronchitis, chest congestion, and blood impurities, among others (Mahmood et al., 2010; Hamza, 2010). In addition to its traditional use as a vegetable, Moringa is recognized for its therapeutic properties, which include antipyretic, anti-ulcer, anti-epileptic, diuretic, cholesterol-lowering, renal protective, anti-diabetic, and hepatoprotective activities (Abalaka et al., 2009; Guevara et al., 1999).
The plant’s bioactive compounds are diverse and include alkaloids such as quinine, saponins, flavonoids, tannins, steroids, glycosides, niazinins, and niaziminins (Figure 1). These secondary metabolites contribute to M. oleifera’s potential as a green resource for the synthesis of nanoparticles (NPs) with various biological activities. Research has shown that compounds like flavonoids, phenolics, polysaccharides, and terpenoids, found in the leaves, flowers, stems, peel, and pods, play a critical role in the production of NPs with distinct sizes and shapes. These nanoparticles exhibit a range of notable biological properties, including antibacterial, antioxidant, cytotoxic, and anticancer effects (Aisida et al., 2019; Aliyu et al., 2017; El-Khadragy et al., 2018; Guevara et al., 1999; Shousha et al., 2019; Surendra et al., 2016; Vasanth et al., 2014). Iron is a crucial micronutrient for plant growth, playing a key role in chlorophyll biosynthesis, enzyme activation, and metabolic pathways. Conventionally, ferrous sulfate is used as an iron source in plant tissue culture media. However, its bioavailability is limited due to rapid oxidation, leading to the formation of insoluble ferric hydroxides that reduce nutrient absorption efficiency (Rout and Sahoo, 2015). Additionally, ferrous sulfate is prone to leaching, causing nutrient loss and environmental concerns. To address these limitations, iron oxide nanoparticles (IONPs) offer a novel and efficient alternative due to their higher stability, controlled release, and enhanced bioavailability in plant tissue culture systems. IONPs exhibit a sustained iron supply, reducing the risk of nutrient deficiencies and oxidative stress, which can hinder plant development. Furthermore, their nano-scale properties facilitate better uptake and transport within plant tissues, potentially improving overall growth, physiological responses, and secondary metabolite accumulation (Shirsat, 2024); (Khan et al., 2020).
Despite the recognized benefits of iron oxide nanoparticles (IONPs) in agriculture, their application as an iron source in Stevia rebaudiana micropropagation remains unexplored. This study introduces a novel approach by utilizing Moringa oleifera leaf extract for the green synthesis of IONPs and incorporating them into Murashige and Skoog (MS) medium as a replacement for ferrous sulfate. Unlike previous studies that focused on conventional elicitors to enhance steviol glycoside production, this research examines how IONPs influence micropropagation, antioxidant activity, and secondary metabolite accumulation in Stevia. The findings provide insights into dose-dependent effects, identifying optimal IONP concentrations that promote growth and the synthesis of bioactive compounds, including diterpene glycosides, in micropropagated Stevia plants while minimizing toxicity risks. By demonstrating the eco-friendly synthesis and agricultural potential of IONPs, this study paves the way for sustainable nanotechnology applications in plant tissue culture, enhancing crop growth and facilitating the production of medicinal metabolites for pharmaceutical industries.
Methodology
Preparation of plant extract and synthesis of iron oxide nanoparticles
A green synthesis approach was applied to synthesise IONPs in the Aquatic Plant Physiology Lab at the Institute of Hydrobiology, Chinese Academy of Sciences, Hubei Province. Iron sulphate (FeSO4) was chosen as the precursor salt due to its common use in nanoparticle formation. The IONPs were developed using an ethanolic extract of fresh, healthy leaves from Moringa oleifera, a heterophyllous aquatic plant.
Fresh leaves of Moringa oleifera were harvested from vegetatively propagated plants cultivated under controlled conditions. Only healthy, disease-free leaves were used to ensure the extract’s quality and consistency. The collected leaves were thoroughly washed with distilled water to remove any dirt or contaminants. After washing, the leaves were sliced into small pieces (approximately 10-20 g) and air-dried at room temperature for 24 hours to reduce moisture content.
The dried leaves were then used to prepare an ethanolic extract by mixing the plant material with 2:8 (v/v) ethanol to distilled water. To ensure that all phytochemicals were extracted, the mixture was agitated at room temperature for 24 hours. Following extraction, the mixture was filtered using Whatman No. 1 filter paper to remove any solid debris, leaving a clear, greenish solution. This filtered extract was maintained in a sterile container at 4°C in a refrigerator for future use. All experiments were carried out under sterile conditions to avoid contamination and retain the extract’s purity.
IONPs were synthesised by adding an ethanolic leaf extract to an aqueous FeSO4 solution (Shabbir et al., 2023). The leaf extract served as a reducing agent and stabiliser for nanoparticle production. The mixture was continuously stirred at room temperature in the dark for 2–3 hours. As the reaction progressed, the color of the solution changed, indicating the formation of iron oxide nanoparticles. After allowing the reaction to proceed for 24 hours, the solution was left undisturbed to ensure complete nanoparticle formation.
Following synthesis, the IONPs were isolated from the reaction solution by centrifugation at 10,000 rpm for 10 minutes. The supernatant was removed, and the pellet containing the nanoparticles was thoroughly rinsed with distilled water to eliminate any remaining chemicals or byproducts. The purified IONPs were resuspended in distilled water and analysed to validate their production as well as their morphological and structural features.
Characterization of iron nanoparticles
Scanning electron microscopy
The morphology of biosynthesised iron nanoparticles was examined using scanning electron microscopy (SEM). The SEM imaging was carried out using a TESCAN MIRA3 model (TESCAN, Czech Republic), which has advance electron optics for high-resolution imaging. A small amount of nanoparticle suspension was drop-cast onto a carbon-coated copper grid, which was then air-dried. The grid was put in the SEM and imaged at various magnifications to determine the size, shape, and distribution of the nanoparticles.
Energy-dispersive x-ray spectroscopy
The elemental composition of the synthesised iron nanoparticles was determined using energy-dispersive X-ray spectroscopy (EDX). The EDX analysis was performed using a TESCAN MIRA3 SEM (TESCAN, Czech Republic), which had an integrated EDX detector. A little amount of nanoparticle sample was drop-coated onto a carbon film and let to dry. The coated sample was then subjected to an EDX detector, which produced a comprehensive spectrum of the elements contained in the nanoparticles. This examination validated the presence of iron (Fe) in the nanoparticle structure and enabled the detection of any potential contaminants or impurities.
Preparation of media for micropropagation of S. rebaudiana
To assess the effects of IONPs on the growth, development, antioxidant profile, and stevioside accumulation in micropropagated S. rebaudiana, five different medium formulations were developed (Table 1). Micropropagation was carried out adopting the protocol established by Khan et al (Khan et al., 2020), with slight modifications matched to our experimental conditions. Sterile nodal segments of S. rebaudiana were employed as explants in the in vitro culture.
The MS (Murashige and Skoog) medium was prepared by dissolving 30 g/L sucrose, 3 mg/L benzylaminopurine (BA), and 7 g/L agar in distilled water. The pH of the medium was adjusted to 5.80 with a dilute solution of hydrochloric acid (HCl) or sodium hydroxide (NaOH). After complete mixing, the medium was autoclaved at 121°C for 15 minutes to remove any microbial contaminants.
After sterilisation and cooling to room temperature, IONPs suspensions with concentrations of 5.60 mg/L, 11.20 mg/L, and 22.40 mg/L were added to the medium. The IONP suspensions were made under sterile conditions and filtered through a 0.22 µm microfilter to eliminate aggregates and ensure uniform dispersion. To prevent nanoparticle aggregation, the suspension was gently added to the cool MS medium. To ensure a uniform distribution of IONPs, the final medium was poured into sterile test tubes (6 ± 1 mL each) and gently mixed by hand. The tubes were then placed in a refrigerator at 4°C to solidify the medium.
Sterile nodal explants were placed in prepared culture tubes and maintained under controlled environmental conditions for four weeks. During this period, data on morphological characteristics, including shoot and root initiation time, shoot and root count, and leaf number, were collected at regular intervals. The fresh and dry weights of the plantlets were also measured to assess overall growth. To determine the dry weight, the plantlets were carefully removed from the culture tubes, rinsed with distilled water, and dried at 50°C for two days until a constant weight was achieved.
Determination of total phenolic content
The total phenolic content (TPC) was determined using the previously reported method (Ali et al., 2019). A 20 μL sample (10 mg/mL) was put to a 96-well microplate, followed by 90 μL of 10X diluted Folin-Ciocalteu reagent. After 5-minute incubation at room temperature, 90 μL of sodium carbonate (20%) was added to make a final volume of 200 μL. A calibration curve was constructed with gallic acid (1-10 mg/mL) as a positive control and methanol (20 μL) as the negative control. After 90 minutes of incubation, the absorbance was measured at 630 nm with a Biotek microplate reader (ELX 800, BIOTEK). The results were represented in milligrams of gallic acid equivalents (GAE) per gram.
Assessment of antioxidant activity using the DPPH assay
The antioxidant capacity of S. rebaudiana plantlets micropropagated under various treatments (positive control, negative control, and 5.60, 11.20, and 22.40 mg/L IONPs concentrations) was assessed using the DPPH (2,2-diphenyl-1-picrylhydrazyl) radical scavenging assay (Abbasi et al., 2010). For sample preparation, 1 mg of plant material from each treatment group was dissolved in 1 mL of dimethyl sulfoxide (DMSO) to make a stock solution. This stock was serially diluted to reach concentrations ranging from 25 to 100 μL. After each dilution, 50 μL was aliquoted into Eppendorf tubes, followed by 950 μL methanol. The tubes were then filled with 50 μL of DPPH solution (0.004%) for a total reaction volume of 1000 μL.
Ascorbic acid was used as a positive control, and DMSO as a negative control. The reaction mixture was incubated in dark at room temperature (25°C) for 30 minutes. The colour shift from purple to yellow was visually observed, with yellow signifying antioxidant activity in the sample. The absorbance of the reaction mixtures was determined spectrophotometrically at 517 nm using a UV-Vis spectrophotometer. The antioxidant activity was represented as a percentage of radical scavenging and calculated using the following formula:
Determination of total flavonoid content
The total flavonoid content (TFC) was determined by adopting the protocol described by Khan et al (Khan et al., 2020). A 96-well microplate was filled with 20 μL of a 10 mg/mL sample, 10 μL of aluminium chloride (10 g/L), and 10 μL of potassium acetate (98.15 g/L). The negative and positive controls were methanol (20 μL) and quercetin (1.0 mg/mL), respectively. The final volume was adjusted to 200 μL with distilled water and incubated for 30 minutes. A standard curve was created with rutin (1-10 mg/mL). The absorbance was measured at 450 nm, and the findings were presented as milligrams of quercetin equivalents (QE) per gram.
Antioxidant enzyme activity assays
Antioxidant enzyme activities were evaluated using the method described by Ali et al. (2018). Samples were homogenised in 2 mL of potassium phosphate buffer (pH 7.8) containing 1% polyvinylpyrrolidone (PVP) and 0.1 mM ethylenediaminetetraacetic acid (EDTA) before centrifugation at 12,000 rpm for 15 minutes at 4°C. The supernatant was used to evaluate the activity of superoxide dismutase (SOD) (Giannopolitis and Ries, 1977). A 3 mL reaction mixture containing 63 mM nitro blue tetrazolium chloride (NBT), 13 mM methionine, 50 mM phosphate buffer, 1.4 mM riboflavin, and 50 µL of enzyme extract was incubated for 15 minutes, after which the absorbance was measured at 560 nm. For peroxidase (POD) activity, (Abeles and Biles, 1991) protocol was following and the oxidation of guaiacol in the presence of hydrogen peroxide (H2O2) was measured at 470 nm. To determine catalase (CAT) activity, 3 mL of the reaction mixture (50 mM PBS, 15 mM H2O2) was mixed with 50 mL of enzyme extract. The reaction was started by the addition of 100 μL of extract; an absorption decrease in H2O2 was measured at 240 nm (Arrigoni et al., 1992). The enzyme activity of ascorbate peroxidase (APX) was determined based on the oxidation of ascorbic acid, with absorbance measured at 290 nm. using a UV-Vis spectrophotometer (Miyake et al., 2006).
HPLC quantification of Stevioside and Rebaudioside A
Stevioside and Rebaudioside A were quantified using HPLC, as described by Kazmi et al (Kazmi et al., 2019b). Dried plant samples were extracted with a water-methanol mixture (1:9 v/v) at 30°C overnight. The extract was filtered, centrifuged, concentrated by vacuum, re-filtered (0.45 μm), and transferred to HPLC vials. Reference standards of stevioside and rebaudioside A (Sigma-Aldrich, USA) were lyophilized and dissolved in HPLC-grade MiliQ water. HPLC analysis was carried out using an Agilent 100 HPLC system with a Luna C18 column (250 mm × 4.6 mm, 5 μm). The mobile phases were acetonitrile/water (85:15 v/v) for phase A and acetonitrile/water (75:25 v/v) for phase B, each with a flow rate of 1 mL/min. Detection was performed at 210 nm, with spectra ranging from 200 to 700 nm. Stevioside and rebaudioside A were identified based on their retention times compared to reference standards.
Statistical analysis
Statistical analyses for all the selected parameters were conducted using Statistix version 8.1 to ensure the reliability and accuracy of the results. One-way analysis of variance (ANOVA) was applied to evaluate significant differences among different treatment groups. To further identify specific group differences, Tukey’s post-hoc test was performed, with a significance threshold set at p ≤ 0.05. This approach allowed for multiple comparisons while minimizing the risk of type I errors. Additionally, all experimental data were graphically represented using GraphPad Prism 5, which facilitated clear visualization and interpretation of trends across different parameters.
Results and discussion
Leaf extract of Moringa oleifera proficiently synthesized IONPs
The application of cost-effective, sustainable, and environment friendly nanotechnology is an appealing approach of increasing agricultural productivity (Wahab et al., 2024; Shereen et al., 2024). Green-synthesized IONPs have received significant attention for their ability to enhance plant growth and development, making them an important tool to improve agricultural systems (Selvaraj et al., 2022; Dowlath et al., 2021). Plant extracts play an important role in the synthesis of these nanoparticles as their active compounds facilitate the reduction of iron ions to nanoparticles (Bouafia and Laouini, 2021; Ikhuoria et al., 2024; Saod et al., 2024; Duan et al., 2024). Despite its traditional medicinal uses, the potential of Moringa oleifera mediated IONPs application in Stevia rebaudiana in vitro plantlet development remains unexplored. Notably, no published research has reported the synthesis of iron oxide nanoparticles (IONPs) using Moringa oleifera leaf extract, and their replacement with ferrus sulfate in stevia micropropagation, a gap this study aims to address. Moringa oleifera was selected for the green synthesis of IONPs due to its rich phytochemical profile, which plays a crucial role in nanoparticle reduction and stabilization (Kiwumulo et al., 2022). The plant is known for its high content of polyphenols, flavonoids, alkaloids, tannins, and proteins, which act as natural capping and stabilizing agents, enabling efficient nanoparticle synthesis without the need for toxic chemical reagents (Yadav et al., 2024). Additionally, Moringa oleifera has been extensively studied for its antioxidant, antimicrobial, and biocompatible properties, making it a promising candidate for eco-friendly nanomaterial synthesis. Its abundant availability, cost-effectiveness, and sustainability further support its selection as a green reducing agent for nanoparticle fabrication (Ahmed et al., 2022). The bioactive compounds in Moringa not only aid in the synthesis of stable IONPs but may also enhance their biological activity, improving their effectiveness in plant tissue culture applications.By utilizing Moringa oleifera for IONP synthesis, this study aligns with the principles of green chemistry, offering an eco-friendly, non-toxic, and sustainable approach to developing nanoparticles with enhanced biocompatibility and bioactivity (Angle, 2024). In the present study, Moringa oleifera leaf extract was used to produce IONPs, which were subsequently added into Murashige and Skoog (MS) medium to replace the conventional iron source. The goal was to assess the effects of these nanoparticles on S. rebaudiana micropropagation, with an emphasis on antioxidant potential, antioxidant enzyme activity, and secondary metabolite accumulation, including phenolics, flavonoids, and diterpene glycosides. At room temperature, IONPs of adequate size and shape were synthesised. SEM and EDX analysis revealed spherical or polygonal IONPs (Figure 2), with the highest iron peak detected in the 6.5-7.5 keV range (Figure 1), which supports previous findings (Elemike et al., 2023; Samuel et al., 2021). This eco-friendly synthesis process reduces toxicity and offers a sustainable option for nanoparticle production.
IONPs promoted micropropagation, leaf development, polyphenols production in of S. rebaudiana
The effects of IONPs on morphology, antioxidant defence system, and stevioside synthesis in S. rebaudiana were investigated. Our results indicate that the effect of IONPs on S. rebaudiana growth is dose-dependent. Plants grown on MS basal medium containing Fe²+ (as FeSO4·7H2O) exhibited normal growth, as did plants grown on MS media supplemented with various concentrations of FeNPs. Notably, lower FeNP concentrations (5.6 mg/L) promoted early shoot and root initiation, with shoots appearing after 5.2 days and roots after 5.3 days. Higher IONP concentrations (11.20 and 22.40 mg/L) resulted in delayed shoot and root initiation (7.5 and 9.1 days, respectively) (Figure 3). These findings indicate that excessive IONPs may cause toxicity, affecting plant growth and development, which is consistent with finding of similar effects on other plant species.
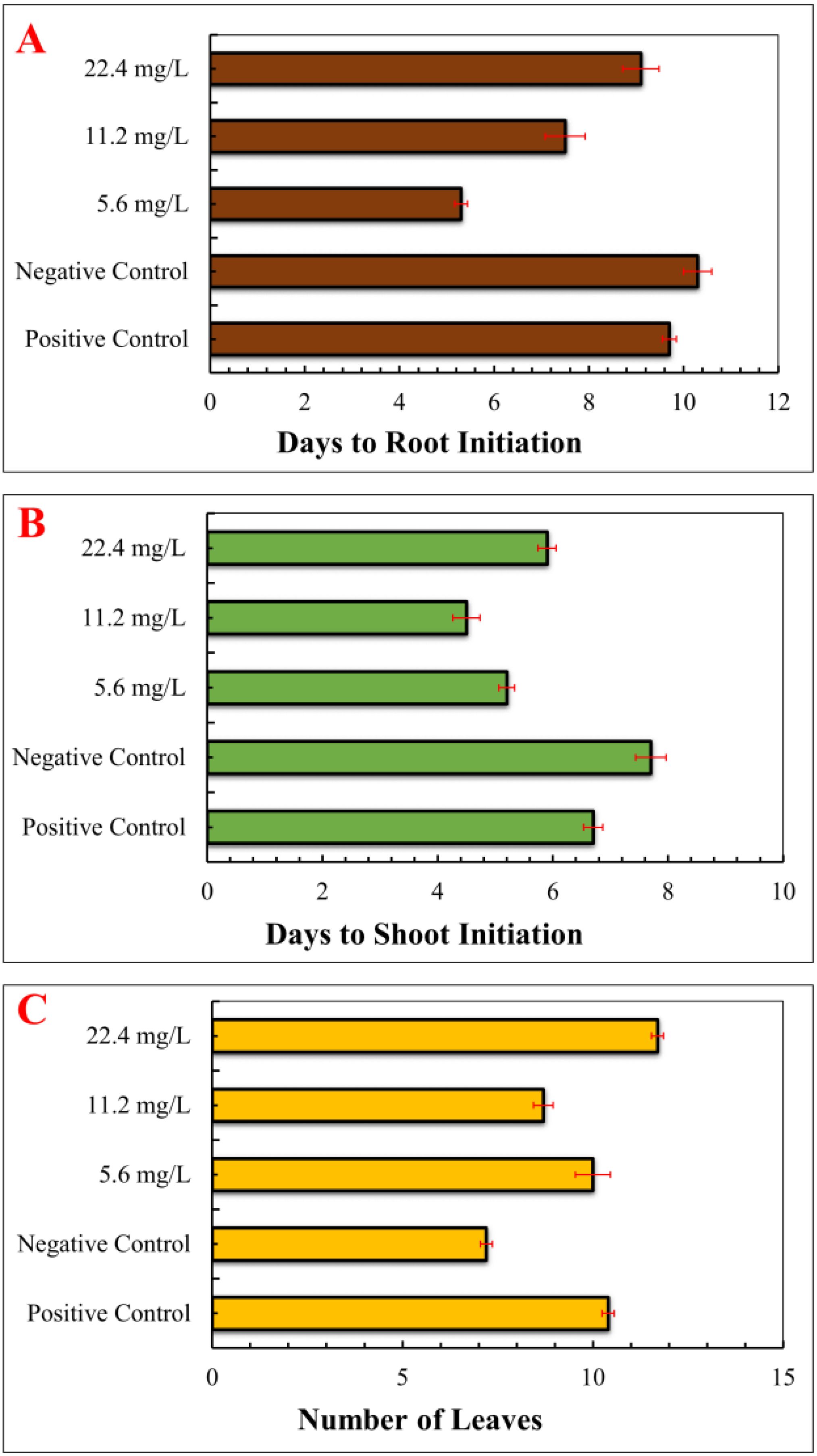
Figure 3. Effect of various concentrations of IONPs on the micropropagation of S. rebaudiana. (A) Days to root initiation measured under different treatments: Positive Control (MS medium with ferrous sulfate), Negative Control (MS medium without ferrous sulfate), and iron oxide nanoparticle (IONP)-supplemented treatments (5.6 mg/L, 11.2 mg/L, and 22.4 mg/L IONPs). (B) Days to shoot initiation of plantlets grown under the same conditions. (C) Number of leaves. Error bars represent standard deviation (SD) from triplicate measurements. Different letters indicate statistically significant differences between treatments (p ≤ 0.05, Tukey’s post-hoc test, one-way ANOVA).
Overall, lower concentrations of IONPs aided plant development, though higher concentrations considerably inhibited growth parameters. These results are consistent with the findings of Khan et al (Khan et al., 2020), who reported that early root and shoot initiation was promoted by lower FeNP concentrations (45 µg/L) and delayed by higher concentrations (90 and 135 µg/L). According to Jamzad Fard et al (Jamzad Fard et al., 2013), Rosa chinensis developed better when exposed to ideal FeNP concentrations in vitro. Similarly, Sheykhbaglou et al. (2010) and Rui et al. (2016) reported that IONPs at low concentrations boosted soybean and peanut biomass, respectively, however higher doses had detrimental effect. In a recently published study IONPs considerably inhibited growth at high concentrations but had a favourable effect on Carum copticum L. at low amount (Razavizadeh et al., 2024). Numerous scientific studies have reported that various nanoparticles can have both positive and negative effects on plant growth and productivity. While some nanoparticles enhance plant development, others may lead to growth inhibition and reduced yield, depending on their concentration and exposure conditions (Ali et al., 2019; Khan et al., 2019b; Ma et al., 2016; Mohammad et al., 2019; Sarmast et al., 2015).
Interestingly, IONPs improved various growth parameters in S. rebaudiana, including leaf length, leaf width, number of leaves, fresh weight, and dry weight. The IONPs treatment at 22.4 mg/L increased leaf width (1.92 cm), length (3.20 cm), number of leaves (n = 11.70), fresh weight (238.90 mg), and dry weight (20.67 mg) in comparison to the positive control (FeSO4·7H2O) and other treatments (Figure 4). IONPs effectively replaced FeSO4·7H2O, increasing leaf length, width, fresh and dry weight to levels comparable to the control. These results are supported by Desai Heta et al (Desai Heta et al., 2017), who reported that magnesium nanoparticles (MgNPs) increased the length and width of S. rebaudiana leaves. IONPs have also been shown to have positive impacts on plant development in date palm (Arafa et al., 2023), Cicer arietinum (Irum et al., 2020), and Oryza sativa (Ullah et al., 2024).
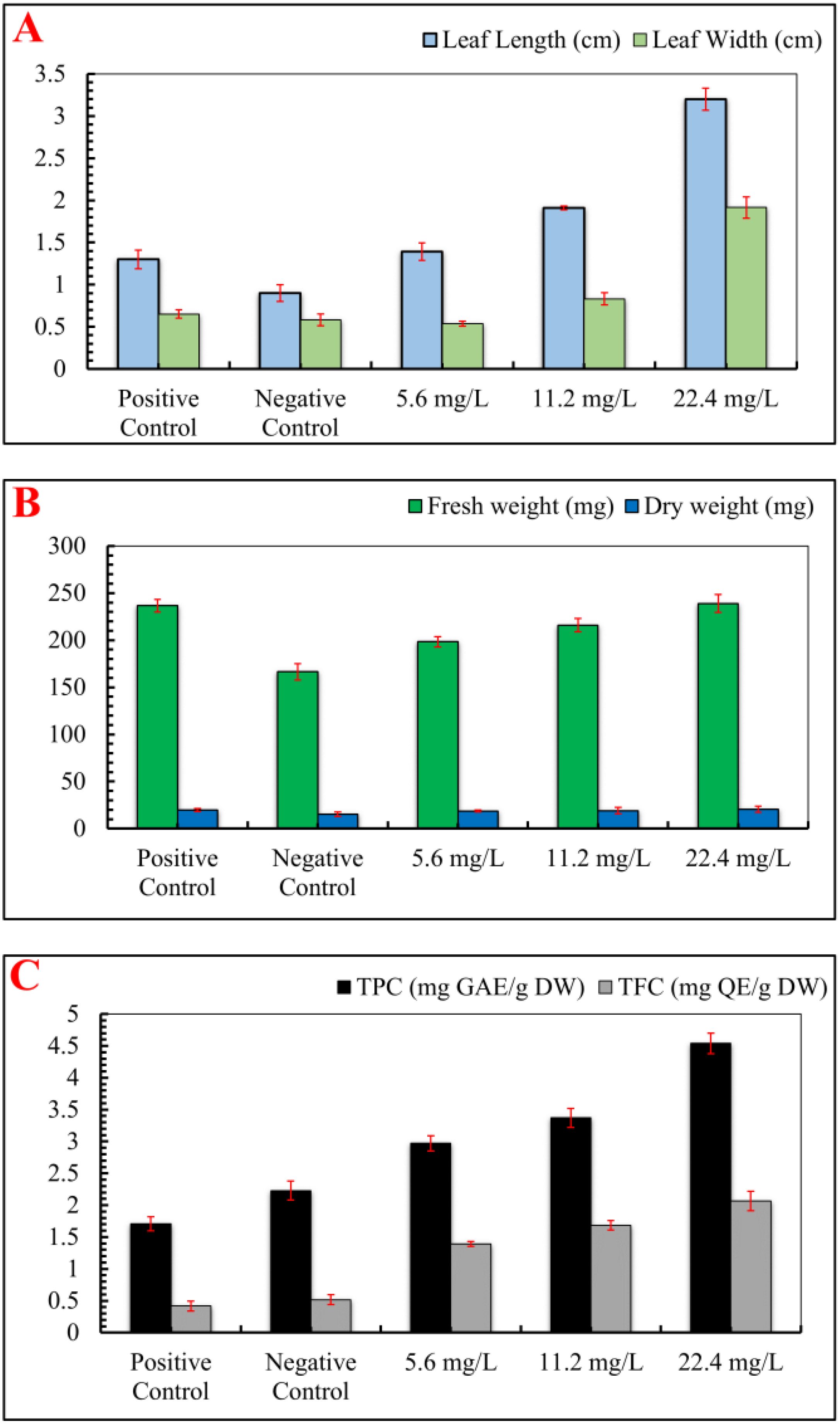
Figure 4. Effect of various concentrations of IONPs on the leaf size, biomass and phytochemicals of micropropagated S. rebaudiana. (A) Leaf length (cm) and leaf width (cm) measured under different treatments: Positive Control (MS medium with ferrous sulfate), Negative Control (MS medium without ferrous sulfate), and iron oxide nanoparticle (IONP)-supplemented treatments (5.6 mg/L, 11.2 mg/L, and 22.4 mg/L IONPs). (B) Fresh weight (mg) and dry weight (mg) of plantlets grown under the same conditions. (C) Total phenolic content (TPC) expressed as mg gallic acid equivalent per gram of dry weight (mg GAE/g DW) and total flavonoid content (TFC) expressed as mg quercetin equivalent per gram of dry weight (mg QE/g DW). Error bars represent standard deviation (SD) from triplicate measurements. Different letters indicate statistically significant differences between treatments (p ≤ 0.05, Tukey’s post-hoc test, one-way ANOVA).
Subsequent analysis revealed that IONPs increased the concentrations of flavonoids and phenolic compounds in S. rebaudiana. In comparison to the positive control (1.71 mg GAE/g and 0.42 mg QAE/g), the total phenolic content (TPC) and total flavonoid content (TFC) were maximum at 22.40 mg/L FeNPs (4.54 mg GAE/g and 2.07 mg GAE/g, respectively). Lower IONPs concentrations (5.6 and 11.2 mg/L) also increased TPC and TFC, though moderately. Our findings correlate with the previous studies, which found enhanced phenolic and flavonoid content in response to nanoparticles (Rastegaran et al., 2022, Sahraei et al., 2023; Khanizadeh et al., 2024; Ayoobi et al., 2024; Khan et al., 2021). Previous studies utilizing different nanoparticles on in vitro cultures of Stevia rebaudiana have demonstrated significant enhancements in phenolic and flavonoid content (Ghazal et al., 2018; Golkar et al., 2019; Javed et al., 2017a). While iron oxide nanoparticles (IONPs) serve as a potential alternative to conventional iron supplements in plant tissue culture, their effects are highly concentration-dependent. At optimal concentrations, IONPs enhance plant growth, biomass accumulation, and secondary metabolite production by facilitating iron uptake and promoting physiological activities (Irum et al., 2020). However, at higher concentrations (e.g., 22.40 mg/L IONPs), phytotoxic effects become evident, leading to growth inhibition, oxidative stress, and metabolic disturbances. One of the primary mechanisms of IONP-induced phytotoxicity is the generation of reactive oxygen species (ROS), which can result in lipid peroxidation, protein oxidation, and DNA damage. Excessive ROS levels disrupt cellular homeostasis, impairing photosynthesis, enzymatic activity, and nutrient absorption (Khan et al., 2023). In the current study, higher IONP concentrations negatively impacted leaf morphology, biomass accumulation, and antioxidant enzyme activities, indicating oxidative stress and cellular toxicity. Moreover, excessive IONP exposure can lead to iron overload, causing iron-induced Fenton reactions, which further exacerbate oxidative damage. This can alter chlorophyll biosynthesis, reduce photosynthetic efficiency, and impair root and shoot development (Onaga et al., 2016). The study’s findings suggest that while moderate IONP supplementation (5.60-11.20 mg/L) promotes plant growth, exceeding this threshold results in detrimental effects on overall plant health and metabolic stability. Thus, careful dose optimization is crucial to harness the benefits of IONPs while minimizing their phytotoxic effects. Future studies should explore mechanisms of nanoparticle uptake, accumulation, and detoxification pathways to mitigate toxicity risks and enhance the application of nanotechnology in plant culture systems.
IONPs significantly enhanced antioxidant potential and diterpene glycosides accumulation in micro-propagated plantlets of S. rebaudiana
When exposed to abiotic stress, plants generate reactive oxygen species (ROS), which disrupt normal physiological functions. To counteract this, plants produce enzymes that neutralize ROS (Wang et al., 2024; Fan and Li, 2024). In the present study, DPPH free radical scavenging activity (%) was assessed, and it was observed that IONP treatment significantly enhanced free radical scavenging activity (89.70%). At lower IONP concentrations (5.60 and 11.20 mg/L), DPPH activity showed a moderate increase (Figure 5). Iron oxide nanoparticles are known to facilitate iron release in plant cells and stimulate the Fenton reaction, ultimately leading to the generation of reactive free radicals (Taghizadeh et al., 2019). Similarly, the activity of ROS-scavenging enzymes, such as catalase (CAT), ascorbate peroxidase (APX), peroxidase (POD), and superoxide dismutase (SOD) was also increased as compared to the negative control. Compared to the Fe-deficient plants (1.77 U/mg protein), the maximum activity of APX (3.77 U/mg protein) was detected at 22.40 mg/L IONPs. Similarly, the maximum CAT activity (3 U/mg protein) was detected at 22.4 mg/L IONPs, followed by considerable increases in SOD (3.46 U/mg protein) and POD (2.73 U/mg protein) activities.
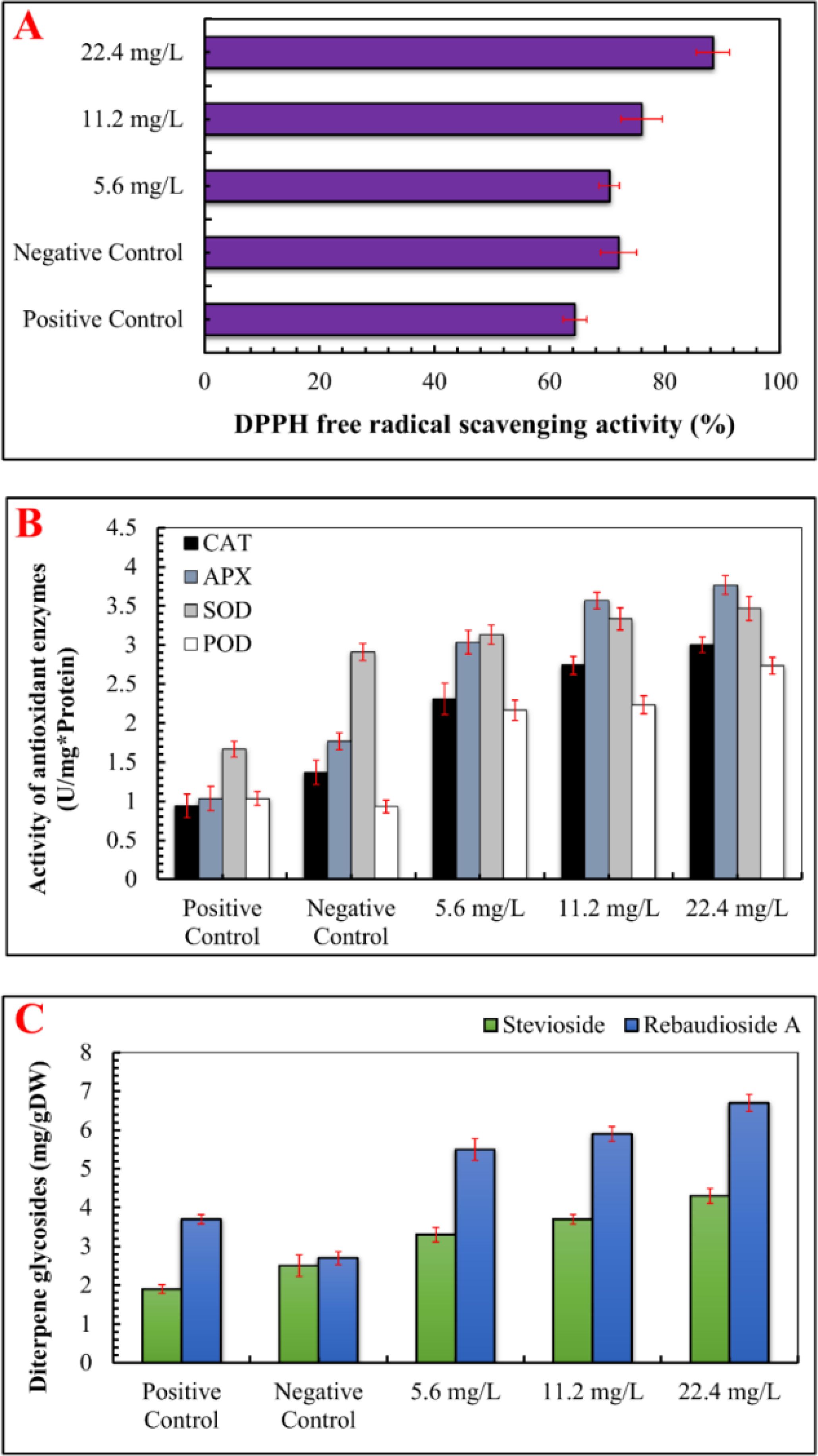
Figure 5. Effect of various concentrations of IONPs on the antioxidant profile and accumulation of secondary metabolites in micropropagated S. rebaudiana. (A) DPPH free radical scavenging activity (%) measured under different treatments: Positive Control (MS medium with ferrous sulfate), Negative Control (MS medium without ferrous sulfate), and iron oxide nanoparticle (IONP)-supplemented treatments (5.6 mg/L, 11.2 mg/L, and 22.4 mg/L IONPs). (B) B) Activity of antioxidant enzymes i.e SOD, POD, CAT, APx (U mg*/Protien) of plantlets grown under the same conditions. (C) Concentration of diterpene glycosides expressed as mg per gram of dry weight (mg/g DW). Error bars represent standard deviation (SD) from triplicate measurements. Different letters indicate statistically significant differences between treatments (p ≤ 0.05, Tukey’s post-hoc test, one-way ANOVA).
ROS-induced oxidative damage might considerably impair macromolecules, eventually leading to cell death (Demidchik, 2015). ROS can degrade chlorophyll content and activate secondary metabolic pathways as a stress response (Yasmeen et al., 2024). Previous studies have shown that metal nanoparticles promote the formation of ROS, which triggers secondary metabolism and reduces plant damage (Hatami and Ghorbanpour, 2024; Prasad et al., 2024; Selvakesavan et al., 2023; Lala, 2021). Furthermore, our results revealed that maximum levels of stevioside (4.30 mg/g DW) and Rebaudioside A (6.70 mg/g DW) accumulation were promoted by IONPs at higher concentration (22.40 mg/L). A moderate level of increase in diterpene glycosides (Stevioside: 3.3 mg/g DW, Rebaudioside A: 5.5 mg/g DW) was also detected in plants micrpropagated under lower IONP concentrations (5.60 and 11.20 mg/L). The increase in steviol glycoside production can be attributed to improved leaf health, as these compounds are synthesized in chloroplasts (Figures 6, 7). Ensuring effective steviol glycoside synthesis and maintaining chloroplast integrity depend on optimal iron levels. Iron plays a crucial role in over 100 enzymes essential for various metabolic processes (Briat et al., 2007).
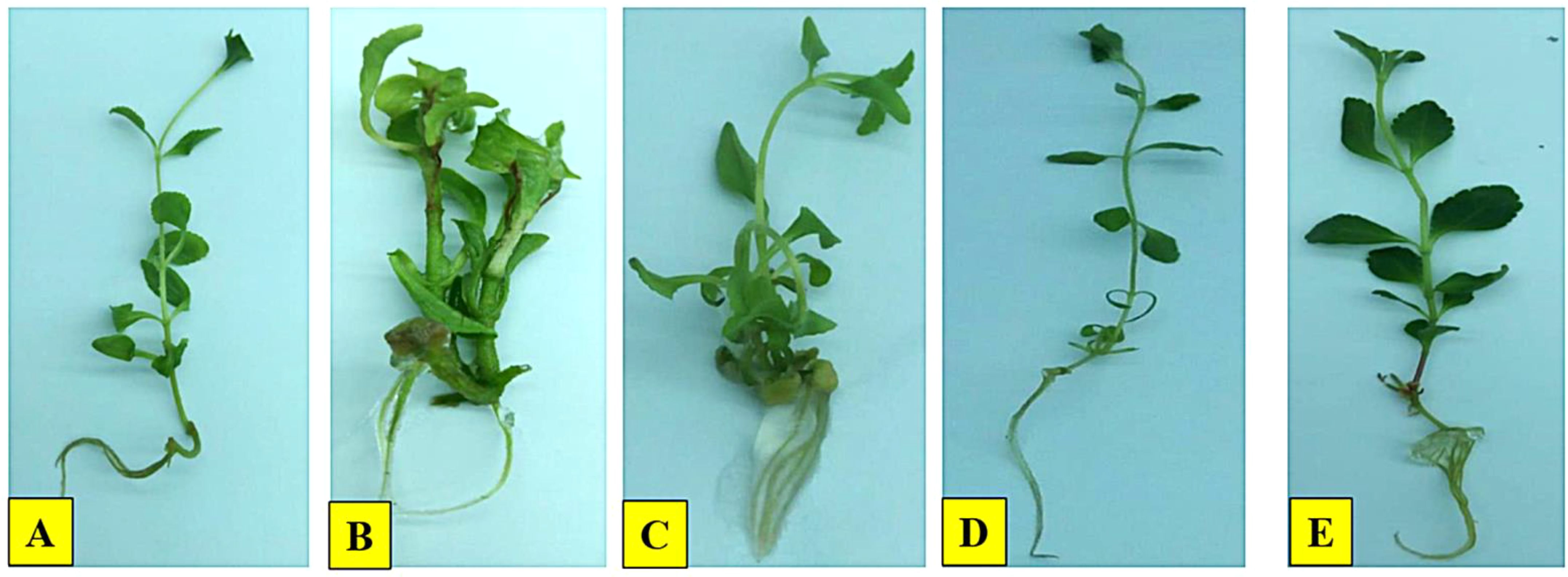
Figure 6. Effect of IONPs on micropropagation of S. rebaudiana. (A) Positive control, (B) Negative control, (C) 5.60 mg/L, (D) 11.20 mg/L, (E) 22.40 mg/L.
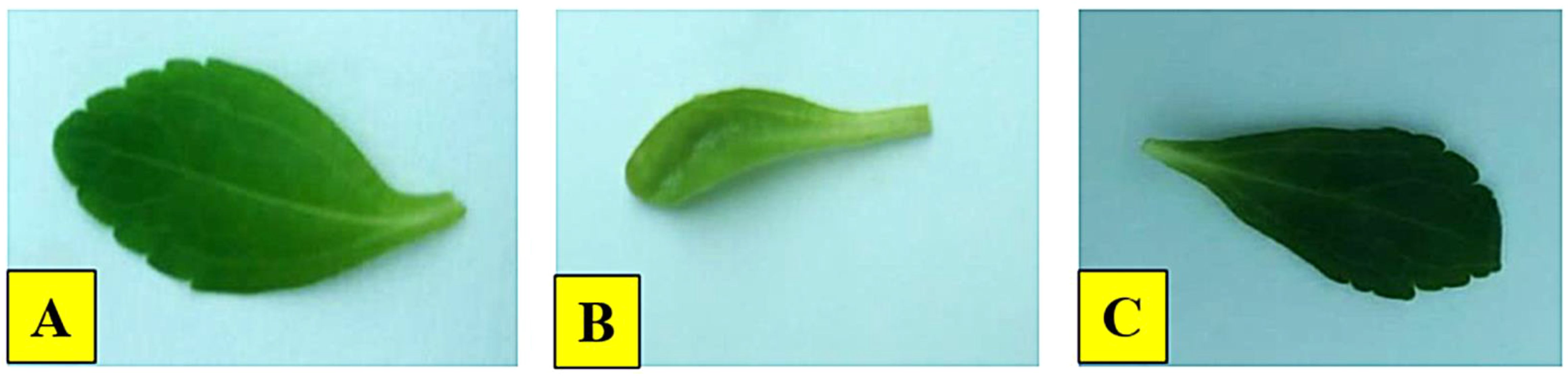
Figure 7. Effect of IONPs on the leaves of micropropagated S. rebaudiana. (A) 22.4 mg/L, (B) Negative control, (C) Positive control.
The enhancement of steviol glycosides in Stevia rebaudiana has been linked to the activation of glycosyltransferase enzymes, which are influenced by external stress-inducing compounds. These enzymes facilitate the transfer of sugar residues from donor molecules to acceptors, thereby promoting the biosynthesis of steviol glycosides (Vázquez-Hernández et al., 2019). Iron is another crucial factor for optimal plant growth and proper chloroplast development. Both deficiency and excess of iron can disrupt metabolic processes. Iron plays a key role in activating over 100 enzymes essential for biochemical reactions. Several studies have reported that various elicitors can significantly increase the production of stevioside and rebaudioside A in S. rebaudiana by upregulating enzymes involved in secondary metabolism (Kazmi et al., 2019a). For instance, Golkar et al. (2019) found that treating callus cultures of S. rebaudiana with 45 mg/L of silver nanoparticles (AgNPs) enhanced the accumulation of rebaudioside A and stevioside. Similarly, Javed et al. (2017b) investigated the effects of zinc oxide nanoparticles (ZnO NPs) on shoot regeneration and steviol glycoside production, reporting that a concentration of 1 mg/L ZnO NPs promoted optimal shoot formation and increased glycoside content. However, higher doses had adverse effects on growth and biosynthesis. Additionally, Magangana et al. (2018) examined the influence of nitrogen and phosphate on S. rebaudiana cultures and found that these nutrients had varying impacts on growth and stevioside biosynthesis.
Conclusion
This study successfully demonstrates the green synthesis of iron oxide nanoparticles (IONPs) using Moringa oleifera leaf extract, which functioned as both a reducing and capping agent. The synthesized IONPs exhibited spherical and polygonal morphologies, and their integration into Murashige and Skoog (MS) medium as a substitute for conventional iron sources significantly influenced the micropropagation of Stevia rebaudiana. A dose-dependent response was observed, wherein lower IONP concentrations (5.60 mg/L) promoted early shoot and root initiation, whereas higher concentrations (11.20 mg/L and 22.40 mg/L) led to growth delays and inhibited development. At optimal concentrations, IONPs enhanced leaf morphology, increased phenolic and flavonoid content, improved antioxidant activity, and stimulated the accumulation of diterpene glycosides, particularly stevioside and rebaudioside A. These findings underscore the potential of IONPs as a sustainable and effective strategy for enhancing plant growth and secondary metabolite production in S. rebaudiana, offering valuable insights for agricultural and pharmaceutical applications.
Future prospects
The promising effects of IONPs on plant growth and secondary metabolite biosynthesis warrant further investigation into the underlying mechanisms, particularly the molecular pathways regulating antioxidant defense and metabolic responses. Future research should focus on optimizing the concentration and application of IONPs across various crops to enhance agricultural productivity. Additionally, long-term studies assessing the environmental impact and potential bioaccumulation of IONPs in plant tissues are crucial to ensuring their safety and sustainability in agricultural systems. Such investigations could facilitate the broader application of nanotechnology in sustainable agriculture, providing innovative solutions to improve crop yield and quality.
Data availability statement
The raw data supporting the conclusions of this article will be made available by the authors, without undue reservation.
Author contributions
ShM: Conceptualization, Data curation, Validation, Visualization, Writing – original draft. AAK: Data curation, Writing – review & editing. MK: Data curation, Formal Analysis, Writing – review & editing. SiM: Data curation, Formal Analysis, Writing – review & editing. AK: Data curation, Methodology, Writing – original draft, Writing – review & editing. AAl: Validation, Visualization, Writing – original draft, Writing – review & editing. AS: Methodology, Writing – review & editing. KR: Data curation, Writing – review & editing. JL-A: Data curation, Formal Analysis, Writing – review & editing. GM-P: Data curation, Writing – review & editing. AP-A: Data curation, Formal Analysis, Writing – review & editing. SA: Formal Analysis, Writing – review & editing. AAh: Data curation, Formal Analysis, Writing – review & editing.
Funding
The author(s) declare that no financial support was received for the research and/or publication of this article.
Acknowledgments
The authors are deeply grateful and extend their sincere appreciation to the Biotechnology Laboratory, Agricultural Research Institute (ARI) Tarnab Peshawar, Pakistan. The authors would also like to extend their sincere appreciation to the Researchers Supporting Project Number (RSP2025R350), King Saud University, Riyadh, Saudi Arabia.
Conflict of interest
The authors declare that the research was conducted in the absence of any commercial or financial relationships that could be construed as a potential conflict of interest.
Generative AI statement
The author(s) declare that no Generative AI was used in the creation of this manuscript.
Publisher’s note
All claims expressed in this article are solely those of the authors and do not necessarily represent those of their affiliated organizations, or those of the publisher, the editors and the reviewers. Any product that may be evaluated in this article, or claim that may be made by its manufacturer, is not guaranteed or endorsed by the publisher.
References
Abalaka, M., Olonitola, O., Onaolapo, J., Inabo, H. I. (2009). Evaluation of acute toxicity of Momordica charantia extract, using wistar rats to determine safety level and usefulness of the plant ethnochemotheraphy. Int. J. Appl. Sci. 3, 1557–9261.
Abbasi, B., Khan, M. A., Mahmood, T., Ahmad, M., Chaudhary, M. F., Khan, M. A. (2010). Shoot regeneration and free-radical scavenging activity in Silybum marianum L. Plant Cell Tissue Organ Culture (PCTOC) 101, 371–376. doi: 10.1007/s11240-010-9692-x
Abeles, F. B., Biles, C. L. (1991). Characterization of peroxidases in lignifying peach fruit endocarp. Plant Physiol. 95 (1), 269–273.
Ahmad, I., Mashwani, Z.-U.-R., Raja, N. I., Kazmi, A., Wahab, A., Ali, A., et al. (2022). Comprehensive approaches of nanoparticles for growth performance and health benefits in poultry: An update on the current scenario. BioMed. Res. Int. 2022, 9539908. doi: 10.1155/2022/9539908
Ahmed, S. F., Mofijur, M., Rafa, N., Chowdhury, A. T., Chowdhury, S., Nahrin, M., et al. (2022). Green approaches in synthesising nanomaterials for environmental nanobioremediation: Technological advancements, applications, benefits and challenges. Environ. Res. 204, 111967. doi: 10.1016/j.envres.2021.111967
Aisida, S. O., Ugwoke, E., Uwais, A., Iroegbu, C., Botha, S., Ahmad, I., et al. (2019). Incubation period induced biogenic synthesis of PEG enhanced Moringa oleifera silver nanocapsules and its antibacterial activity. J. Polymer Res. 26, 1–11. doi: 10.1007/s10965-019-1897-z
Ali, A., Mashwani, Z.-U.-R., Raja, N. I., Mohammad, S., Luna-Arias, J. P., Ahmad, A., et al. (2023). Phytomediated selenium nanoparticles and light regimes elicited in vitro callus cultures for biomass accumulation and secondary metabolite production in Caralluma tuberculata. Front. Plant Sci. 14, 1253193. doi: 10.3389/fpls.2023.1253193
Ali, A., Mohammad, S., Khan, M. A., Raja, N. I., Arif, M., Kamil, A., et al. (2019). Silver nanoparticles elicited in vitro callus cultures for accumulation of biomass and secondary metabolites in Caralluma tuberculata. Artif. cells nanomed. Biotechnol. 47, 715–724. doi: 10.1080/21691401.2019.1577884
Ali, H., Khan, M. A., Ullah, N., Khan, R. S. (2018). Impacts of hormonal elicitors and photoperiod regimes on elicitation of bioactive secondary volatiles in cell cultures of ajuga bracteosa. J. Photochem. Photobiol. B: Biol. 183, 242–250.
Ali Naghizadeh, A., Zarandi, M. M., Khoshroo, S., Davarani, F. (2024). Investigating the Effect of Green Silver Nanoparticles on Seed Germination and Physiological Parameters of Spinach (Spinacia oleracea L.) under Salt Stress. Russian J. Plant Physiol. 71, 102. doi: 10.1134/S102144372460586X
Aliyu, A., Garba, S., Bognet, O. (2017). Green synthesis, characterization and antimicrobial activity of vanadium nanoparticles using leaf extract of Moringa oleifera. Int. J. Chem. Sci. 16, 231.
Alsafawi, A., Şahin, G. (2024). Boosting Stevia’s sweetness: Growth regulators mitigate salt stress and enhance steviol glycosides. South Afr. J. Bot. 174, 1–8. doi: 10.1016/j.sajb.2024.08.040
Angle, A. S. (2024). “Green Synthesis Methods for Metallic Zinc Oxide Nanoparticles,” in Synthesizing and Characterizing Plant-Mediated Biocompatible Metal Nanoparticles, (IGI Global Scientific Publishing Platform), 63.
Anum, F., Raja, N. I., Sultana, T., Kazmi, A., Ali, A., Qayyum, B., et al. (2021). Spectral lights based treatment enhanced biomass accumulation and secondary metabolites production in callus culture of citrus reticulata. Philippine Agric. Sci. 104, 287–298. doi: 10.62550/DAB05034021
Arafa, R. N., Elsayh, S. A., El-Din, I., El-Habashy, S., Ahmed, E. A., Afifi, E., et al. (2023). Impact of iron nanoparticles application on date palm in-vitro regeneration stability. Plant Cell Biotechnol. AND Mol. Biol. 24, 89–107.
Arrigoni, O., De Gara, L. (1992). Franca Tommasi, and Rosalia Liso. “Changes in the ascorbate system during seed development of Vicia faba L.”. Plant Physiol. 99 (1), 235–238.
Ayoobi, A., Saboora, A., Asgarani, E., Efferth, T. (2024). Iron oxide nanoparticles (Fe3O4-NPs) elicited Artemisia annua L. in vitro, toward enhancing artemisinin production through overexpression of key genes in the terpenoids biosynthetic pathway and induction of oxidative stress. Plant Cell Tissue Organ Culture (PCTOC) 156, 85.
Biswas, A., Pal, S. (2024). Plant-nano interactions: a new insight of nano-phytotoxicity. Plant Physiol. Biochem. 108646. doi: 10.1016/j.plaphy.2024.108646
Bouafia, A., Laouini, S. E. (2021). Plant-mediated synthesis of iron oxide nanoparticles and evaluation of the antimicrobial activity: A review. Mini-Reviews Organic Chem. 18, 725–734. doi: 10.2174/18756298MTA5jNzYp2
Briat, J.-F., Curie, C., Gaymard, F. (2007). Iron utilization and metabolism in plants. Curr. Opin. Plant Biol. 10, 276–282. doi: 10.1016/j.pbi.2007.04.003
Chung, I.-M., Rekha, K., Rajakumar, G., THIRUVENGADAM, M. (2018). Influence of silver nanoparticles on the enhancement and transcriptional changes of glucosinolates and phenolic compounds in genetically transformed root cultures of Brassica rapa ssp. rapa. Bioprocess Biosyst. Eng. 41, 1665–1677. doi: 10.1007/s00449-018-1991-3
De Andrade, M. V. S., Lucho, S. R., De Castro, R. D., Ribeiro, P. R. (2024). Alternative for natural sweeteners: Improving the use of stevia as a source of steviol glycosides. Industrial Crops Products 208, 117801. doi: 10.1016/j.indcrop.2023.117801
Demidchik, V. (2015). Mechanisms of oxidative stress in plants: from classical chemistry to cell biology. Environ. Exp. Bot. 109, 212–228. doi: 10.1016/j.envexpbot.2014.06.021
Desai Heta, B., Desai Charmi, V., Desai Charmi, P., Singh, D., Suthar, H. (2017). Effect of magnesium nanoparticles on physiology and stevioside in Stevia rebaudiana Bertoni. Eur. J. Biomed. Pharm. Sci. 4, 642–646.
Dey, A., Nandy, P. (2024). Size, shape, and dose—three crucial determinants for applying nanoparticles in sustainable plant biology. Academia Biol. 2.
Dowlath, M. J., Musthafa, S. A., Khalith, S. M., Varjani, S., Karuppannan, S. K., Ramanujam, G. M., et al. (2021). Comparison of characteristics and biocompatibility of green synthesized iron oxide nanoparticles with chemical synthesized nanoparticles. Environ. Res. 201, 111585.
Duan, Y.-T., Soni, K., Patel, D., Sangani, C. B., Saeed, W. S., Ameta, K. L., et al. (2024). Green synthesis of iron oxide nanoparticles using Nicotiana plumbaginifolia and their biological evaluation. J. Mol. Liquids 396, 123985.
Ehsan, M., Waheed, A., Ullah, A., Kazmi, A., Ali, A., Raja, N. I., et al. (2022). Plant-based bimetallic silver-zinc oxide nanoparticles: A comprehensive perspective of synthesis, biomedical applications, and future trends. BioMed. Res. Int. 2022, 1215183.
Elemike, E. E., Nna, P. J., Ikenweke, C., Onwudiwe, D., Omotade, E. T., Singh, M. (2023). Synthesis, characterization, anti-cancer and antimicrobial studies of iron oxide nanoparticles mediated by Terminalia catappa (Indian almond) leaf extract. Inorganic Chem. Commun. 155, 111048.
El-Khadragy, M., Alolayan, E. M., Metwally, D. M., El-Din, M. F. S., Alobud, S. S., Alsultan, N. I., et al. (2018). Clinical efficacy associated with enhanced antioxidant enzyme activities of silver nanoparticles biosynthesized using Moringa oleifera leaf extract, against cutaneous leishmaniasis in a murine model of Leishmania major. Int. J. Environ. Res. Public Health 15, 1037.
El-Naggar, M., Osman, A. R. (2024). Enhancing growth and bioactive metabolites characteristics in Mentha pulegium L. via silicon nanoparticles during in vitro drought stress. BMC Plant Biol. 24, 657.
Fan, M., Li, Q. (2024). The metabolism, detrimental effects, and signal transduction mechanism of reactive oxygen species in plants under abiotic stress. J. Biobased Materials Bioenergy 18, 359–376.
Ghazal, B., Fareed, A., Ahmad, N., Azra, Salmen, S., Ansari, M. J., et al. (2024). Elicitors directed in vitro growth and production of stevioside and other secondary metabolites in Stevia rebaudiana (Bertoni) Bertoni. Sci. Rep. 14, 14714.
Ghazal, B., Saif, S., Farid, K., Khan, A., Rehman, S., Reshma, A., et al. (2018). Stimulation of secondary metabolites by copper and gold nanoparticles in submerge adventitious root cultures of stevia rebaudiana (Bert.). IET Nanobiotechnology 12 (5), 569–573.
Giannopolitis, C. N., Ries, S. K. (1977). Superoxide dismutases: I. Occurrence in higher plants. Plant Physiol. 59 (2), 309–314.
Golkar, P., Moradi, M., Garousi, G. A. (2019). Elicitation of Stevia glycosides using salicylic acid and silver nanoparticles under callus culture. Sugar Technol. 21, 569–577.
Guevara, A. P., Vargas, C., Fujiwara, Y., Hashimoto, K., Maoka, T., Kozuka, M., et al. (1999). An antitumor promoter from Moringa oleifera Lam. Mutat. Research/Genetic Toxicol. Environ. Mutagenesis 440, 181–188.
Gupta, S. D., Agarwal, A., Pradhan, S. (2018). Phytostimulatory effect of silver nanoparticles (AgNPs) on rice seedling growth: An insight from antioxidative enzyme activities and gene expression patterns. Ecotoxicol. Environ. Saf. 161, 624–633.
Hamza, A. A. (2010). Ameliorative effects of Moringa oleifera Lam seed extract on liver fibrosis in rats. Food Chem. Toxicol. 48, 345–355.
Hatami, M., Ghorbanpour, M. (2024). Metal and metal oxide nanoparticles-induced reactive oxygen species: Phytotoxicity and detoxification mechanisms in plant cell. Plant Physiol. Biochem., 108847.
Huang, C., Wang, Y., Zhou, C., Fan, X., Sun, Q., Han, J., et al. (2024). Properties, extraction and purification technologies of Stevia rebaudiana steviol glycosides: A review. Food Chem., 139622.
Ikhuoria, E. U., Uwidia, I. E., Okojie, R. O., Ifijen, I., Chikaodili, I. D., Fatiqin, A. (2024). Advancing green nanotechnology: harnessing the bio-reducing properties of Musa paradisiaca peel extract for sustainable synthesis of iron oxide nanoparticles. J. Multidiscip. Appl. Natural Sci. 4, 108–119.
Iqbal, Z., Javad, S., Naz, S., Shah, A. A., Shah, A. N., Paray, B. A., et al. (2022). Elicitation of the in vitro cultures of selected varieties of Vigna radiata L. with zinc oxide and copper oxide nanoparticles for enhanced phytochemicals production. Front. Plant Sci. 13, 908532.
Irum, S., Jabeen, N., Ahmad, K. S., Shafique, S., Khan, T. F., Anwaar, S., et al. (2020). Biogenic iron oxide nanoparticles enhance callogenesis and regeneration pattern of recalcitrant Cicer arietinum L. PloS One 15, e0242829. doi: 10.1371/journal.pone.0242829
Jamzad Fard, M., Mousavi, M., Ghafarian Mogharab, M. (2013). “Investigation on effect of Fe oxide nanoparticles on shoot proliferation and rooting in miniature rose in vitro,” in The 1st national conference on solution to access sustainable development in agriculture, natural resources and environment, 7. Available online at: http://www.civilica.com/Paper-FNCAES01-FNCAES01_231.html.
Javed, R., Mohamed, A., Yücesan, B., Gürel, E., Kausar, R., Zia, M. (2017a). CuO nanoparticles signifcantly infuence in vitro culture, steviol glycosides, and antioxidant activities of Stevia rebaudiana Bertoni. Plant Cell Tissue Organ Cult 131, 611–620. doi: 10.1007/s11240-017-1312-6
Javed, R., Usman, M., Yücesan, B., Zia, M., Gürel, E. (2017b). Efect of zinc oxide (ZnO) nanoparticles on physiology and steviol glycosides production in micropropagated shoots of Stevia rebaudiana Bertoni. Plant Physiol. Biochem. 110, 94–99. doi: 10.1016/j.plaphy.2016.05.032
Javed, R., Yucesan, B., Zia, M., Gurel, E. (2022). “Nanoelicitation: a promising and emerging technology for triggering the sustainable in vitro production of secondary metabolites in medicinal plants,” in Plant and nanoparticles (Springer Nature Germany: Springer).
Kazmi, A., Khan, M. A., Mohammad, S., Ali, A., Ali, H. (2019a). Biotechnological production of natural calorie free Steviol glycosides in stevia Rebaudiana: an update on current scenario. Curr. Biotechnol. 8, 70–84. doi: 10.2174/2211550108666191210100751
Kazmi, A., Khan, M. A., Mohammad, S., Ali, A., Kamil, A., Arif, M., et al. (2019b). Elicitation directed growth and production of steviol glycosides in the adventitious roots of Stevia rebaudiana Bertoni. Industrial Crops Products 139, 111530. doi: 10.1016/j.indcrop.2019.111530
Khan, S., Akhtar, N., Rehman, S. U., Jamil, M. (2023). “Iron oxide nanoparticles: plant response, interaction, phytotoxicity and defense mechanisms,” in Nanomaterials and nanocomposites exposures to plants: response, interaction, phytotoxicity and defense mechanisms (Springer Nature Singapore, Singapore), 227–245.
Khan, M. A., Ali, A., Mohammad, S., Ali, H., Khan, T., Mashwani, Z.-U.-R., et al. (2020). Iron nano modulated growth and biosynthesis of steviol glycosides in Stevia rebaudiana. Plant Cell Tissue Organ Culture (PCTOC) 143, 121–130. doi: 10.1007/s11240-020-01902-6
Khan, A. U., Khan, T., Khan, M. A., Nadhman, A., Aasim, M., Khan, N. Z., et al. (2021). Iron-doped zinc oxide nanoparticles-triggered elicitation of important phenolic compounds in cell cultures of Fagonia indica. Plant Cell Tissue Organ Culture (PCTOC) 147, 287–296. doi: 10.1007/s11240-021-02123-1
Khan, M. A., Khan, T., Riaz, M. S., Ullah, N., Ali, H., Nadhman, A. (2019). “Plant cell nanomaterials interaction: Growth, physiology and secondary metabolism,” in Comprehensive analytical chemistry, vol. 84. (Elsevier's Netherlands: Elsevier), 23–54.
Khanizadeh, P., Morshedloo, M. R., Maggi, F. (2024). Application of Fe2O3 nanoparticles improves the growth, antioxidant power, flavonoid content, and essential oil yield and composition of Dracocephalum kotschyi Boiss. Front. Plant Sci. 15, 1475284. doi: 10.3389/fpls.2024.1475284
Kiwumulo, H. F., Muwonge, H., Ibingira, C., Lubwama, M., Kirabira, J. B., Ssekitoleko, R. T. (2022). Green synthesis and characterization of iron-oxide nanoparticles using Moringa oleifera: a potential protocol for use in low and middle income countries. BMC Res. Notes 15, 149. doi: 10.1186/s13104-022-06039-7
Lala, S. (2021). Nanoparticles as elicitors and harvesters of economically important secondary metabolites in higher plants: A review. IET nanobiotechnol. 15, 28–57. doi: 10.1049/nbt2.12005
Lankarani, S. M. J., Karimi, J., Rezaei, A. (2024). Impact of zinc oxide nanoparticles and iron on Stevia rebaudiana Bertoni growth, nutrient uptake, and bioactive compounds under in vitro conditions. Plant Cell Tissue Organ Culture (PCTOC) 159, 18. doi: 10.1007/s11240-024-02871-w
Ma, C., Liu, H., Guo, H., Musante, C., Coskun, S. H., Nelson, B. C., et al. (2016). Defense mechanisms and nutrient displacement in Arabidopsis thaliana upon exposure to CeO 2 and In 2 O 3 nanoparticles. Environ. Sci.: Nano 3, 1369–1379.
Magangana, T. P., Stander, M. A., Makunga, N. P. (2018). Efect of nitrogen and phosphate on in vitro growth and metabolite profles of Stevia rebaudiana Bertoni (Asteraceae). Plant Cell Tissue Organ Cult 134, 141–151. doi: 10.1007/s11240-018-1407-8
Mahajan, M., Naveen, P., Pal, P. K. (2024). Agronomical and biotechnological strategies for modulating biosynthesis of steviol glycosides of Stevia rebaudiana Bertoni. J. Appl. Res. Medicinal Aromatic Plants 100580. doi: 10.1016/j.jarmap.2024.100580
Mahmood, K. T., Mugal, T., Haq, I. U. (2010). Moringa oleifera: a natural gift-A review. J. Pharmaceutical Sci. Res. 2, 775.
Mathur, P., Chakraborty, R., Aftab, T., Roy, S. (2023). Engineered nanoparticles in plant growth: Phytotoxicity concerns and the strategies for their attenuation. Plant Physiol. Biochem. 199, 107721. doi: 10.1016/j.plaphy.2023.107721
Miyake, C., Shinzaki, Y., Nishioka, M., Horiguchi, S., Tomizawa, K. I. (2006). Photoinactivation of ascorbate peroxidase in isolated tobacco chloroplasts: Galdieria partita APX maintains the electron flux through the water–water cycle in transplastomic tobacco plants. Plant Cell Physiol. 47 (2), 200–210.
Mohammad, S., Khan, M. A., Ali, A., Khan, L., Khan, M. S., Mashwani, Z. U. R. (2019). Feasible production of biomass and natural antioxidants through callus cultures in response to varying light intensities in olive (Olea europaea. L) cult. Arbosana. J. Photochem. Photobiol. B: Biol. 193, 140–147.
Moharramnejad, S., Azam, A. T., Panahandeh, J., Dehghanian, Z., Ashraf, M. (2019). Effect of methyl jasmonate and salicylic acid on in vitro growth, stevioside production, and oxidative defense system in Stevia rebaudiana. Sugar Tech 21, 1031–1038. doi: 10.1007/s12355-019-00727-8
Murthy, N., Joseph, K. S., Paek, K. Y., Park, S. Y. (2024). Light as an elicitor for enhanced production of secondary metabolites in plant cell, tissue, and organ cultures. Plant Growth Regul., 1–19. doi: 10.1007/s10725-024-01139-9
Natarajan, K., Adhimoolam, K., Santhanu, K., Vinod, S., Natesan, S., Min, T., et al. (2024). In planta synthesis of silver nanoparticles and its effect on adventitious shoot growth and withanolide production in Withania somnifera (L.) Dunal. Plant Physiol. Biochem. 214, 108882. doi: 10.1016/j.plaphy.2024.108882
Onaga, G., Dramé, K. N., Ismail, A. M. (2016). Understanding the regulation of iron nutrition: can it contribute to improving iron toxicity tolerance in rice? Funct. Plant Biol. 43, 709–726. doi: 10.1071/FP15305
Parveen, J., Sultana, T., Kazmi, A., Malik, K., Ullah, A., Ali, A., et al. (2023). Phytosynthesized nanoparticles as novel antifungal agent for sustainable agriculture: A mechanistic approach, current advances, and future directions. J. Nanotechnol. 2023, 8011189. doi: 10.1155/2023/8011189
Pathak, A., Haq, S., Meena, N., Dwivedi, P., Kothari, S. L., Kachhwaha, S. (2023). Multifaceted role of nanomaterials in modulating in vitro seed germination, plant morphogenesis, metabolism and genetic engineering. Plants 12, 3126. doi: 10.3390/plants12173126
Prasad, A., Sidhic, J., Sarbadhikary, P., Narayanankutty, A., George, S., George, B. P., et al. (2024). Role of metal nanoparticles in organogenesis, secondary metabolite production and genetic transformation of plants under in vitro condition: a comprehensive review. Plant Cell Tissue Organ Culture (PCTOC) 158, 33. doi: 10.1007/s11240-024-02833-2
Ramezani, M., Gerami, M., Majlesi, Z. (2019). Comparison between various concentrations of commercial and synthesized silver nanoparticles on biochemical parameters and growth of Stevia rebaudiana B. Plant Physiol. Rep. 24, 141–152. doi: 10.1007/s40502-018-0413-5
Rastegaran, M., Hassanpour, H., Ziyadi, H. (2022). Synthesized Fe3O4 nanoparticles induced antioxidant activity and total phenolic and flavonoid content in Matricaria chamomilla seedlings. Iranian J. Plant Physiol. 1, 4003.
Razavizadeh, R., Anwari, A. A.-S., Forghani, A., Mirmazloum, I. (2024). Application of iron oxide nanoparticles improves growth and phytochemical constituents of in vitro cultured Carum copticum L. J. Agric. Food Res. 18, 101402. doi: 10.1016/j.jafr.2024.101402
Rout, G. R., Sahoo, S. (2015). Role of iron in plant growth and metabolism. Rev. Agric. Sci. 3, 1–24. doi: 10.7831/ras.3.1
Rui, M., Ma, C., Hao, Y., Guo, J., Rui, Y., Tang, X., et al. (2016). Iron oxide nanoparticles as a potential iron fertilizer for peanut (Arachis hypogaea). Front. Plant Sci. 7, 815. doi: 10.3389/fpls.2016.00815
Rukh, S., Kazmi, A., Nabi, G., Irshad, M., Ali, A., Muhammad, S., et al. (2023). Improvement of in vitro regeneration frequency, polyphenolic and antioxidant profile of Strawberry (Fragaria ananassa Cv. Chandler) via indirect organogenesis. J. Pure Appl. Agric. 8.
Sahraei, M., Hassanpour, H., Ziyadi, H. (2023). Iron oxide nanoparticle-induced changes in the growth, oxidative responses, and antioxidant capacity of physalis alkekengi seedlings. Russian J. Plant Physiol. 70, 32. doi: 10.1134/S1021443722602944
Samuel, M. S., Datta, S., Chandrasekar, N., Balaji, R., Selvarajan, E., Vuppala, S. (2021). Biogenic synthesis of iron oxide nanoparticles using Enterococcus faecalis: Adsorption of hexavalent chromium from aqueous solution and in vitro cytotoxicity analysis. Nanomaterials 11, 3290. doi: 10.3390/nano11123290
Saod, W. M., Al-Janaby, M. S., Gayadh, E. W., Ramizy, A., Hamid, L. L. (2024). Biogenic synthesis of iron oxide nanoparticles using Hibiscus sabdariffa extract: Potential for antibiotic development and antibacterial activity against multidrug-resistant bacteria. Curr. Res. Green Sustain. Chem. 8, 100397. doi: 10.1016/j.crgsc.2024.100397
Sarmast, M. K., Niazi, A., Salehi, H., Abolimoghadam, A. (2015). Silver nanoparticles affect ACS expression in Tecomella undulata in vitro culture. Plant Cell Tissue Organ Culture (PCTOC) 121, 227–236. doi: 10.1007/s11240-014-0697-8
Selvakesavan, R. K., Kruszka, D., Shakya, P., Mondal, D., Franklin, G. (2023). “Impact of nanomaterials on plant secondary metabolism,” in Nanomaterial interactions with plant cellular mechanisms and macromolecules and agricultural implications. Springer Nature, 133–170.
Selvaraj, R., Pai, S., Vinayagam, R., Varadavenkatesan, T., Kumar, P. S., Duc, P. A., et al. (2022). A recent update on green synthesized iron and iron oxide nanoparticles for environmental applications. Chemosphere 308, 136331. doi: 10.1016/j.chemosphere.2022.136331
Shabbir, M. A., Naveed, M., Rehman, S. U., Ain, N. U., Aziz, T., Alharbi, M., et al. (2023). Synthesis of iron oxide nanoparticles from Madhuca indica plant extract and assessment of their cytotoxic, antioxidant, anti-inflammatory, and anti-diabetic properties via different nanoinformatics approaches. ACS omega 8, 33358–33366. doi: 10.1021/acsomega.3c02744
Shereen, M. A., Ahmad, A., Satti, S. M., Kazmi, A., Bashir, N., Shehroz, M., et al. (2024). Plant extract preparation and green synthesis of silver nanoparticles using Swertia chirata: Characterization and antimicrobial activity against selected human pathogens. Heliyon 10. doi: 10.1016/j.heliyon.2024.e28038
Sheykhbaglou, R., Sedghi, M., Shishevan, M. T., Sharifi, R. S. (2010). Effects of nano-iron oxide particles on agronomic traits of soybean. Notulae Scientia Biologicae 2, 112–113. doi: 10.15835/nsb224667
Shirsat, S. (2024). Iron oxide nanoparticles as iron micronutrient fertilizer—Opportunities and limitations. J. Plant Nutr. Soil Sci. 187, 565–588. doi: 10.1002/jpln.202300203
Shousha, W. G., Aboulthana, W. M., Salama, A., Saleh, M., Essawy, E. A. (2019). Evaluation of the biological activity of Moringa oleifera leaves extract after incorporating silver nanoparticles, in vitro study. Bull. Natl. Res. Centre 43, 1–13. doi: 10.1186/s42269-019-0221-8
Sichanova, M., Geneva, M., Petrova, M., Miladinova-Georgieva, K., Kirova, E., Nedev, T., et al. (2022). Improvement of Stevia rebaudiana Bertoni in vitro propagation and steviol glycoside content using aminoacid silver nanofibers. Plants 11, 2468.
Surendra, T., Roopan, S. M., Arasu, M. V., Al-Dhabi, N. A., Rayalu, G. M. (2016). RSM optimized Moringa oleifera peel extract for green synthesis of M. oleifera capped palladium nanoparticles with antibacterial and hemolytic property. J. Photochem. Photobiol. B: Biol. 162, 550–557.
Taghizadeh, M., Nasibi, F., Kalantari, K. M., Ghanati, F. (2019). Evaluation of secondary metabolites and antioxidant activity in Dracocephalum polychaetum Bornm. cell suspension culture under magnetite nanoparticles and static magnetic field elicitation. Plant Cell Tissue Organ Culture (PCTOC) 136, 489–498.
Tavakoli, H., Tavakoli, N., Moradi, F. (2019). The effect of the elicitors on the steviol glycosides biosynthesis pathway in Stevia rebaudiana. Funct. Plant Biol. 46, 787–795.
Thiruvengadam, M., Chi, Y., Kim, S.-H. (2024). Impact of nanopollution on plant growth, photosynthesis, toxicity, and metabolism in the agricultural sector: An updated review. Plant Physiol. Biochem., 108370.
Ullah, J., Gul, A., Khan, I., Shehzad, J., Kausar, R., Ahmed, M. S., et al. (2024). Green synthesized iron oxide nanoparticles as a potential regulator of callus growth, plant physiology, antioxidative and microbial contamination in Oryza sativa L. BMC Plant Biol. 24, 939.
Vasanth, K., Ilango, K., Mohankumar, R., Agrawal, A., Dubey, G. P. (2014). Anticancer activity of Moringa oleifera mediated silver nanoparticles on human cervical carcinoma cells by apoptosis induction. Colloids surfaces B: Biointerfaces 117, 354–359.
Vazquez-Hernandez, C., Feregrino-Perez, A. A., Perez-Ramirez, I., Ocampo-Velazquez, R. V., Rico-García, E., Torres-Pacheco, I., et al. (2019). Controlled elicitation increases steviol glycosides (SGs) content and gene expression-associated to biosynthesis of SGs in Stevia rebaudiana B. cv. Morita II. Industrial Crops Products 139, 111479. doi: 10.1016/j.indcrop.2019.111479
Wahab, A., Muhammad, M., Ullah, S., Abdi, G., Shah, G. M., Zaman, W., et al. (2024). Agriculture and environmental management through nanotechnology: Eco-friendly nanomaterial synthesis for soil-plant systems, food safety, and sustainability. Sci. Total Environ., 171862.
Wang, P., Liu, W. C., Han, C., Wang, S., Bai, M. Y., Song, C. P. (2024). Reactive oxygen species: multidimensional regulators of plant adaptation to abiotic stress and development. J. Integr. Plant Biol. 66, 330–367. doi: 10.1111/jipb.13601
Yadav, J., Chauhan, P., Rawat, R. K., Pathak, S. K., Srivastava, S. (2024). Syzygium aromaticum-mediated green synthesis of iron oxide nanoparticles for efficient heavy metal removal from aqueous solutions. J. Indian Chem. Soc. 101, 101201. doi: 10.1016/j.jics.2024.101201
Keywords: Moringa oleifera, Stevia Rebaudiana, nanotechnology, iron oxide nanoparticles, secondary metabolites
Citation: Muhammad S, Khan AA, Khan MR, Mukhtar S, Kazmi A, Ali A, Siddiqa A, Aileen Hernández Ramírez K, Luna-Arias JP, Medina-Pérez G, Pelaez-Acero A, Armenta S and Ahmad A (2025) Effective substitution of ferrous sulfate with iron oxide nanoparticles enhances growth, antioxidant activity, and stevioside accumulation in micro-propagated Stevia rebaudiana. Front. Plant Sci. 16:1569613. doi: 10.3389/fpls.2025.1569613
Received: 05 February 2025; Accepted: 03 April 2025;
Published: 25 April 2025.
Edited by:
Azamal Husen, Wolaita Sodo University, EthiopiaReviewed by:
Rathinapriya Periyasamy, Rural Development Administration, Republic of KoreaSailendra Singh, Council of Scientific and Industrial Research (CSIR), India
Copyright © 2025 Muhammad, Khan, Khan, Mukhtar, Kazmi, Ali, Siddiqa, Aileen Hernández Ramírez, Luna-Arias, Medina-Pérez, Pelaez-Acero, Armenta and Ahmad. This is an open-access article distributed under the terms of the Creative Commons Attribution License (CC BY). The use, distribution or reproduction in other forums is permitted, provided the original author(s) and the copyright owner(s) are credited and that the original publication in this journal is cited, in accordance with accepted academic practice. No use, distribution or reproduction is permitted which does not comply with these terms.
*Correspondence: Amir Ali, YW1pcmtoYW4zMTUzMEBnbWFpbC5jb20=; Sher Muhammad, ZHJzaGVybW9oYW1tYWRAZ21haWwuY29t