- State Key Laboratory of Hybrid Rice, Hunan Hybrid Rice Research Center, Hunan Academy of Agricultural Sciences, Changsha, China
Cold stress significantly limits rice productivity, particularly at the early seedling stage. Identifying key genes responsible for cold tolerance is crucial for breeding resilient rice varieties. In the study, we identified a quantitative trait locus (QTL), qESCT2, associated with cold tolerance at the early seedling stage. The QTL was mapped into an interval of RM1347–RM5356 on chromosome 2 using an F2:3 population derived from a cross between XZX45, a cold-sensitive early rice variety from China, and IL43, an introgression line developed by marker-assisted backcrossing. IL43 was created using XN1, a highly cold-resistant cultivar, as the donor parent and XZX45 as the recurrent parent. By integrating transcriptomic data from the target region, we identified Os02g0181300 as the candidate gene for qESCT2. This gene encodes a transcription factor, OsWRKY71. Edited lines of OsWRKY71 exhibited a significantly lower survival rate under cold tolerance compared to the wild type Nipponbare. Further analysis revealed that OsWRKY71 likely regulated cold tolerance at the early seedling stage by a glutathione metabolism related pathway. Additionally, OsWRKY71 exhibits differentiation between indica and japonica subspecies with distinct haplotypes. These findings will facilitate to further research into the genetic basis of cold tolerance at the early seedling stage and enhance the development of cold-resistant rice varieties by marker-assisted selection.
Introduction
Rice, a staple food for more than half of the world’s population, is highly sensitive to cold stress due to its tropical and subtropical origins. Cold stress at the early seedling stage has emerged as a significant constraint on rice production. It is estimated that about 7 million hectares of land worldwide are unsuitable for rice cultivation due to cold stress (Liu et al., 2013). Cold damage can affect rice at all developmental stages, but chilling injury at the early seedling stage is particularly detrimental, causing symptoms such as wilting, stunt growth, poor establishment, and ultimately, reduced yields. This issue is particularly critical in double-season rice cultivation areas, where early rice is more vulnerable to cold damage. Therefore, identifying and isolating cold tolerance genes to facilitate breeding cultivars with enhanced cold tolerance at the early seedling stage, especially for early rice in double-season rice areas, remains an urgent priority for improving rice production.
Cold tolerance is a complex quantitative trait controlled by multiple loci. With the rapid development of molecular markers and bioinformatics, significant progress has been made in dissecting complex quantitative traits. To date, a large number of QTLs associated with cold tolerance has been identified (Andaya and Mackill, 2003; Han et al., 2007). Among them, Various populations have been utilized, including F2 populations (Liu et al., 2015), recomnbinant inbred line (RIL) populations (Andaya and Mackill, 2003; Jiang et al., 2008; Suh et al., 2012), genome-wide association study (GWAS) populations (Song et al., 2018; Li et al., 2021), doubled haploid (DH) populations (Lou et al., 2007), backcross inbred line (BIL) populations (Fujino et al., 2004; Miura et al., 2002), and near isogenic line (NIL) populations (Saito et al., 1995, 2004, 2010; Park et al., 2013). Numerous genes associated with cold tolerance has been cloned, including HAN1 (Mao et al., 2019), COLD1(chilling-tolerance divergence) (Ma et al., 2015), CTB1 (cold tolerance at the booting stage) (Saito et al., 2010), qLTG3-1 (quantitative trait locus for low-temperature germinability) (Fujino et al., 2008), CTB4a (Zhang et al., 2017), COLD11 (Li Z et al., 2023), LTG1 (low temperature growth) (Lu et al., 2014), bZIP73 (Liu et al., 2019), COLD6 (Luo et al., 2024), CTB3 (Li et al., 2025), CTB5 (Guo et al., 2025), CTB6 (Gao et al., 2025), CTF1(cold tolerance at the flowering stage) (Dong et al., 2025). However, to date, only few genes for cold tolerance at the early stage were cloned and characterized. While genetic analysis by population construction is a powerful tool for QTL mapping, but it is often time-consuming and labor-intensive. Transcriptomic analysis, on the other hand, offers a more efficient approach by narrowing down candidate genes based on gene expression changes. NIL populations are particularly useful for mapping QTLs with minor effects due to uniform genetic background. Combining NIL population with expression profiling provides a fast and effective strategy for identifying candidate genes. Liu et al. (2013) successfully identified a candidate gene for cold tolerance at the early seedling stage by integrating RNA microarray analysis with an NIL population. This approach highlights the potential of combining genetic and transcriptomic tools to accelerate the discovery of genes underlying complex traits.
In this study, a set of introgression lines (ILs) was developed by marker-assisted backcrossing using Xiangnuo 1 (XN1) as the donor parent and Xiangzhaoxian (XZX45) as the recurrent parent. Among them, IL43, exhibiting high cold tolerance at the early seedling stage, was selected and crossed with the recipient parent XZX45 to construct an F2:3 population for mapping QTLs associated with cold tolerance. Furthermore, expression profiles of IL43 and XZX45 were generated. By integrating transcriptomic data with linkage mapping, the candidate gene, OsWRKY71, was identified and cloned. Functional validation revealed that it’s edited line significantly decreased cold tolerance at the early seedling stage. This finding offers a valuable genetic resource for enhancing cold tolerance in rice by molecular breeding.
Materials and methods
Rice material and population construction
XN1 is a cold-tolerant landrace at the early seedling stage, whereas XZX45 is an early-maturing but cold-sensitive variety. F1 plants were generated by crossing XN1, as the donor parent, and XZX45, as the recipient. These F1 plants were subsequently backcrossed with XZX45 for four consecutive generations to produce BC4F1 plants. Additionally, SSR (Simple Sequence Repeat) markers showing clear and polymorphic bands between XN1 and XZX45 were screened. Ultimately, 110 SSR markers evenly distributed across all 12 chromosomes were employed to select ILs from these BC4F1 plants (Supplementary Table S1).
IL43 was crossed with XZX45 to develop a F2 population consisting of 171 individual plants. Seeds collected from the F2 population were then used to establish the F2:3 lines, which were assessed for cold tolerance at the early seedling stage.
Cold stress scoring at the early seedling stage in rice
Cold treatment was conducted in the growth chamber (LT-361, Percival, USA) following the protocol described in a previous study (Liu et al., 2015). In brief, approximately 60 fully F3 seeds were dried at 50°C for 2 days to break dormancy. The seeds were then surface-sterilized and germinated in trays until their coleoptiles reached 5 mm in length. A total of 50 uniformly germinated seeds were selected and subjected to cold stress at 4°C with a relative humidity of 60% for 4 days. After the cold treatments, the seedlings were transferred to a recovery environment with a day/night temperature regime of 28/25°C with a relative humidity of 60% for 7 days. Seedling survival percentage, defined as the ratio of surviving seedlings to the total seedlings exposed to cold treatment, was calculated to evaluate cold tolerance at the early seedling stage. The experiment was arranged in a randomized complete block design with three independent replications. The mean values between replications were used for data analysis, which was performed using DPS software V.2.0 (Tang and Feng, 2007).
Extraction of DNAs and SSR markers
DNA from the F2 population and parental lines was extracted using the CTAB method, as described by Liu et al. (2015). SSR primers were amplified as the previous study (Liu et al., 2015). The resulting PCR products were detected on 6% non-denaturing polyacrylamide gels and visualized via silver staining.
Construction of linkage map and QTL mapping
Regional linkage maps on chromosomes 2 and 10 were constructed using Mapmaker v.3.0b (Lincoln, 1992). Genetic map distance in centiMorgans (cM) was calculated with the Kosambi function. QTL analysis was conducted using composite interval mapping (CIM) implemented in the Window QTL Cartographer 2.5 software (Wang et al., 2008). The standard CIM model was selected, with forward and backward regression employed for background control. In the CIM analysis, the window size was set to 10 cM (the default value). A threshold of LOD > 2.5 was used to declare the presence of a putative QTL.
Transicriptome analysis
Germinated seeds with 5 mm bud length from IL43 and XZX45 were subjected to cold stress at 4 °C, buds were harvested at 0, 3 and 12h after stress treatment. Three biological repeats were used for total RNA extraction with TRIzol reagent (Invitrogen). A cDNA library was constructed following Illumina standard protocol and sequenced on a HiSeq 2000 sequencer. All paired-end reads were aligned to the rice Nipponbare reference genome (https://rapdb.dna.affrc.go.jp/download/irgsp1.html). Gene expression levels were calculated using the reads per kilobase per million reads method (RPKM). The differentially expressed genes (DEGs) between IL43 and XZX45 were identified using the criteria of |log2Fold Change| ≥ 1 and a Q ≤0.05.
A hypergeometric test was applied (P ≤ 0.05) to identify significantly enriched Gene Ontology (GO) terms by comparing DEGs to the genomic background. Additionally, KEGG pathway analysis was conducted using the blastall program against the KEGG database (http://www.genome.jp/kegg). Pathway enrichment analysis was performed to identify significantly enriched metabolic and signal transduction pathways associated with DEGs (P ≤ 0.05).
Plasmid construct and transformation
To construct the clustered regularly inter-spaced short palindromic repeats (CRISPR)/CRISPR-associated nuclease 9 (Cas9) vector, single guide RNAs (sgRNAs) targeting the coding regions of Os02g0181300 and Os02g0207400 were designed. The expression cassettes, OsU3-Os02g0181300-sgRNA and OsU3-Os02g0207400-sgRNA, were subsequently assembled to create two new vectors, pYL-HU-U3-CrOs02g0181300 and pYL-HU-U3-CrOs02g0207400. These constructs were introduced into Nipponbare mature embryo-induced calli by Agrobacterium-mediated transformation (EHA105). The primers used are listed in Supplementary Table S2.
Allelic variation and haplotype analysis
The primers were designed for amplifying coding regions of Os02g0181300 from IL43 and XZX45. Haplotype network analysis was used from the website (http://ricevarmap.ncpgr.cn/). The primers used are listed in Supplementary Table S2.
Results
Development and identification of ILs
A total of 16 ILs were selected on the basis of SSR marker genotypes, collectively covering approximately one-third of the donor genome. Among these, chromosomes 1, 9, and 12 of the donor genome were almost entirely represented. Each IL contained more than two donor chromosomal segments, ranging in size from approximately 2.1 to 27.3 Mb. Specifically, IL43 carried two donor segments: one in the interval of RM1347–RM341 on chromosome 2 (14.0 Mb) and another in the interval of RM271–RM228 on chromosome 10 (5.6 Mb) (Figure 1).
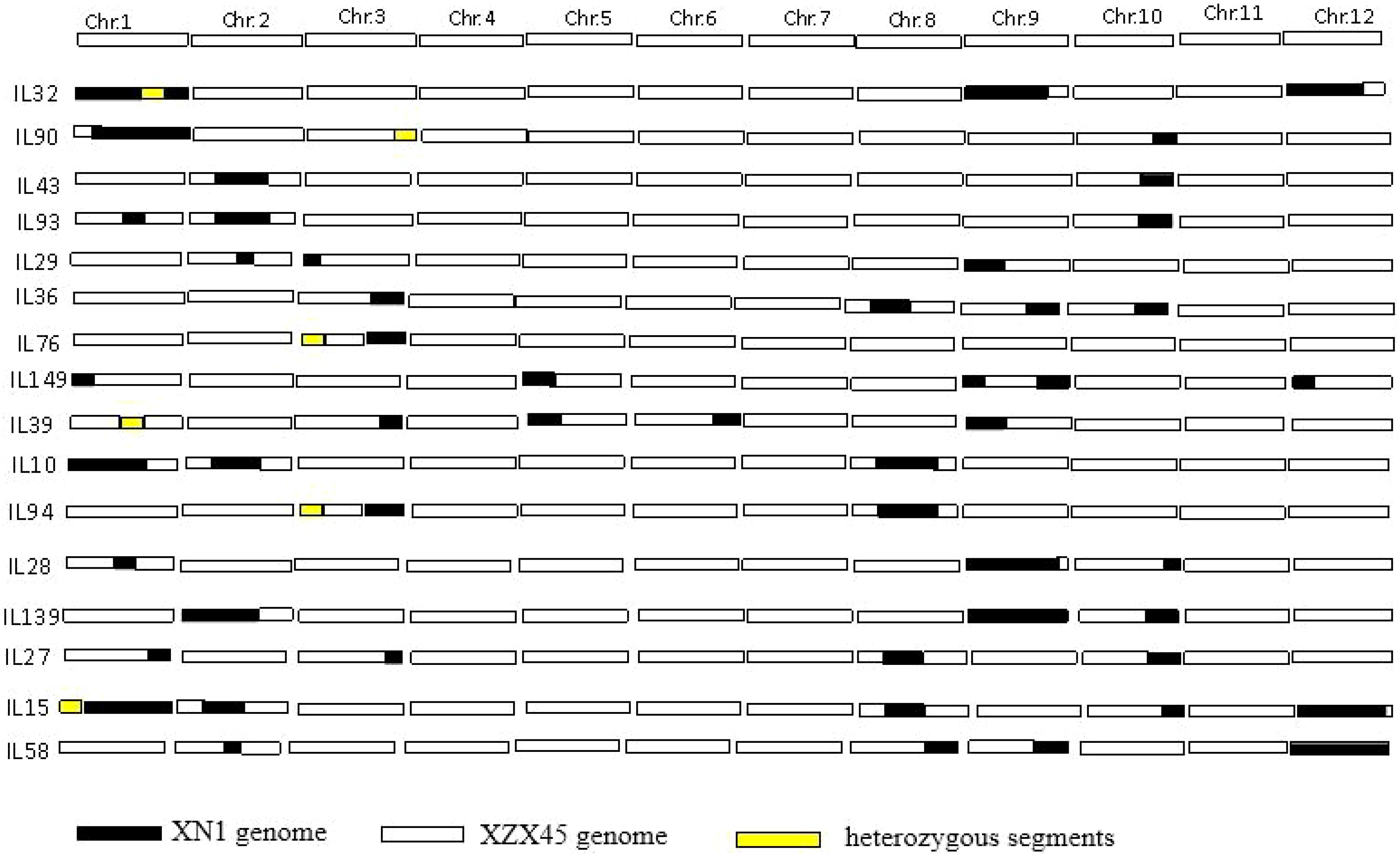
Figure 1. Selected ILs by marker-assisted backcross derived from XN1 as donor parent and XZX45 as recurrent parent. XN1, Xiangnuo 1. XZX45, Xiangzaoxian45.
Cold tolerance evaluation between IL43 and XZX45 revealed that IL43 exhibited significantly higher cold tolerance at the early seedling stage, with a survival rate of 63.5%, compared to 32.6% for XZX45 (Figure 2A). These results suggest that IL43 likely harbors QTLs associated with cold tolerance, potentially derived from the donor genome.
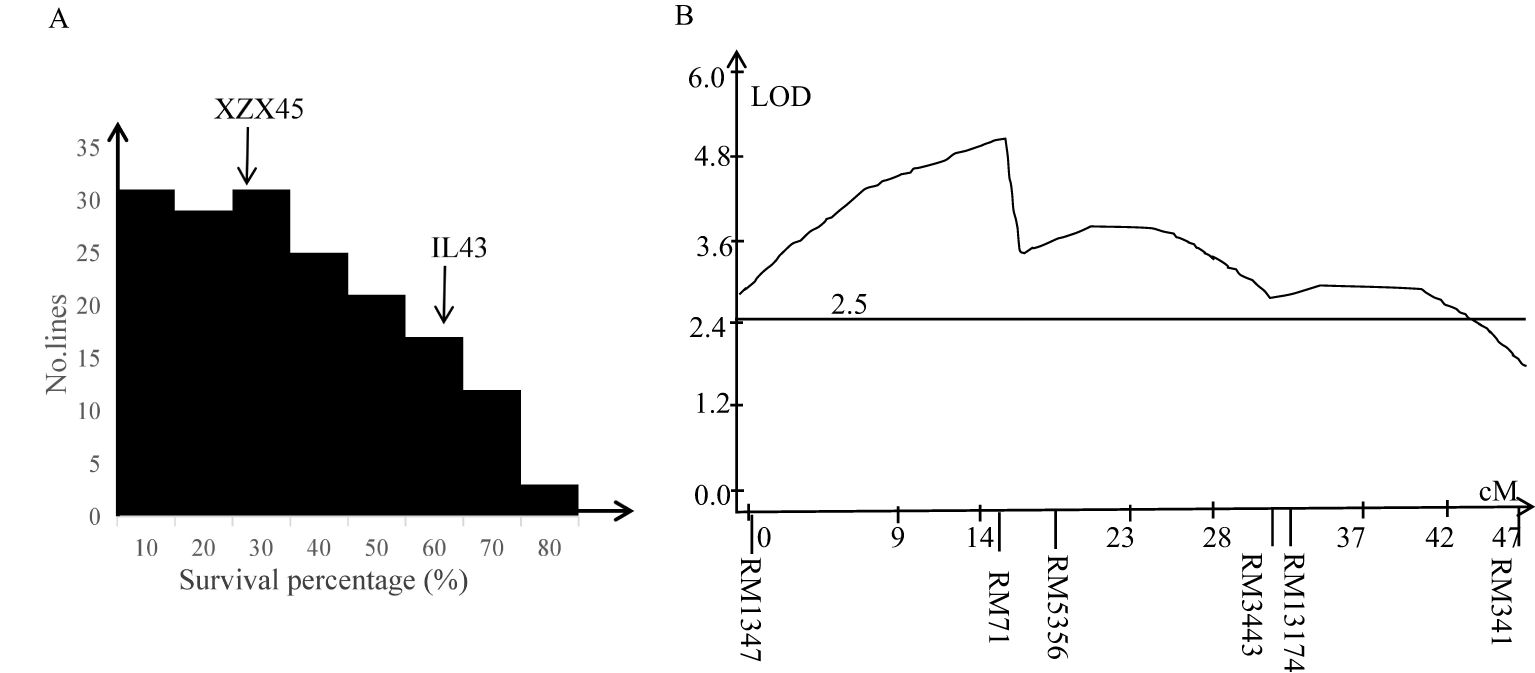
Figure 2. Frequency distribution of survival percentage (A) and a curve line diagram for QTL mapping (B) in an F2:3 population derived from the cross between XZX45 and IL43.
Delimitation of confidence interval of QTL for cold tolerance
To dissect the QTL associated with cold tolerance at the early seedling stage, an F2 population derived from XZX45/IL43 cross was developed. Two regional linkage maps were constructed on chromosome 2 and 10 in the F2 population, spanning 46.8 cM and 37.5 cM, respectively. The frequency distribution of seedling survival percentage in the F2:3 lines showed continuous variation (Figure 2A), suggesting that the QTL primarily played an additive effect.
QTL analysis identified a significant QTL with peak located in the interval of RM1347–RM5356 on chromosome 2. This QTL had a LOD score of 5.15 and accounted for 29.51% of the phenotypic variation (Figure 2B). The donor-derived allele at this locus was found to enhance cold tolerance in the population. In contrast, no significant QTL was detected in the interval of RM271–RM228 on chromosome 10.
DEGs identified in the target region
In order to identify candidate genes within the interval of RM1347–RM5356, genome-wide gene expression profiling of IL43 and XZX45 was investigated at 0, 3 and 12h after cold stress. Principal component analysis (PCA) of the RNA-sequencing (RNA-seq) data revealed that principal components 1 accounted for 99.5% of the total variability. The PCA results showed three distinct clusters, with each cluster containing samples from IL43 and XZX45 corresponding to the same time point (Figure 3A). This clustering pattern suggests that IL43 and XZX45 had high expression similarity. The result further supported that IL43 and XZX45 share nearly identical genetic backgrounds.
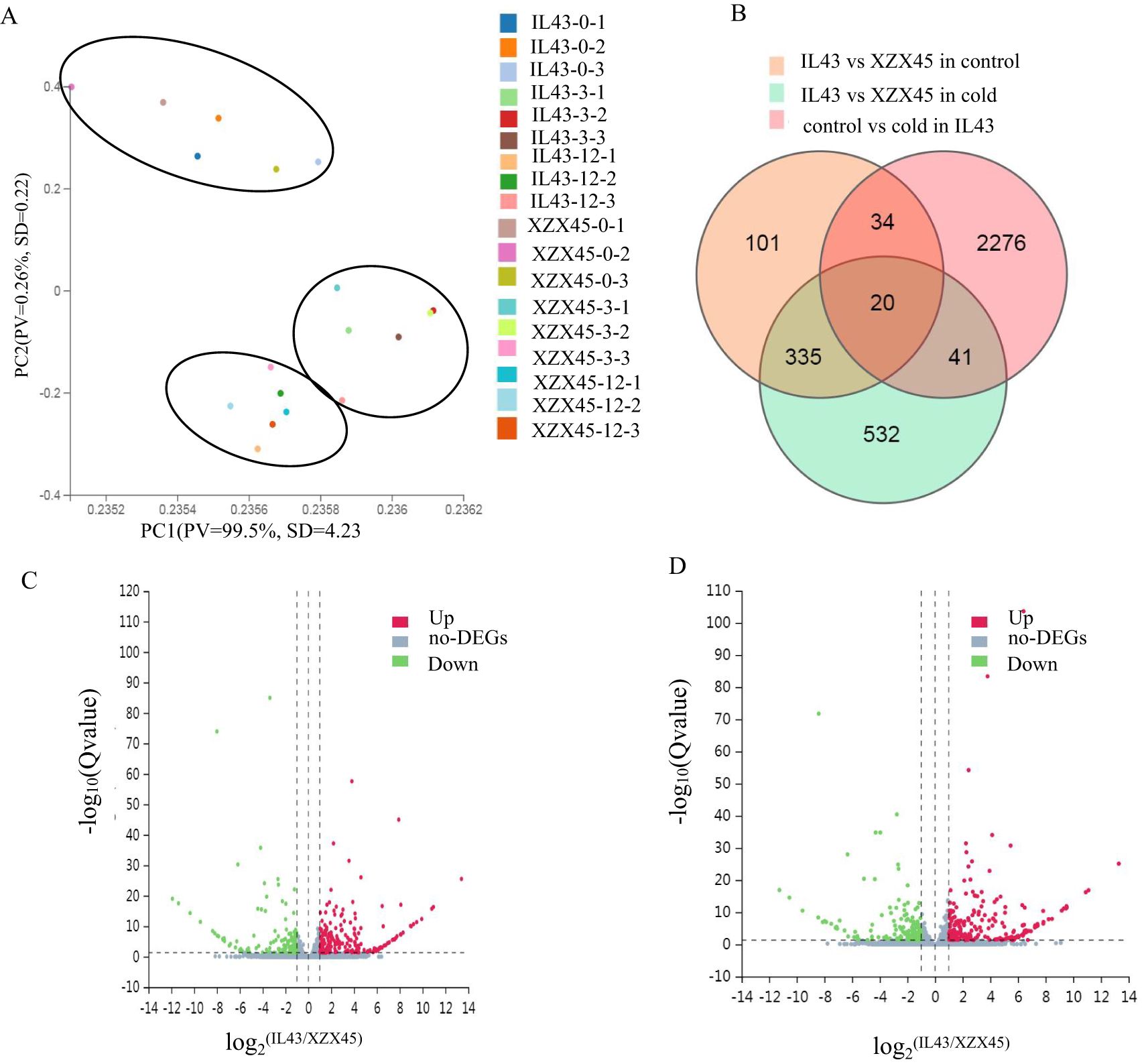
Figure 3. Principal component analysis (PCA), identification of differentially expressed genes (DEGs), and volcano plots. (A) Distribution of XZX45 and IL43 along the first two principal components (PC1 and PC2). (B) Venn diagram showing DEGs between XZX45 and IL43 under different conditions. (C) Volcano plot comparing XZX45 with IL43 under normal temperatures. (D) Volcano plot comparing XZX45 with IL43 under low temperatures.
A total of 490 and 928 DEGs were identified under normal conditions and cold stress, respectively. Under normal condition, 284 genes were up-regulated and 206 genes were down-regulated (Figure 3B). Under cold stress, 401 genes were up-regulated and 527 genes were down-regulated (Figure 3C). The relatively small number of DEGs under both conditions further supports the conclusion that IL43 and XZX45 share nearly identical genetic backgrounds. This finding is consistent with the SSR marker detection results, which exhibited that IL43 carries only two short donor segments.
Analysis of candidate genes
A Venn diagram comparing gene expression profiles among IL43 vs XZX45 under control conditions, IL43 vs XZX45 under cold stress, and IL43 under both control and cold stress revealed 20 genes with significant expression changes (Figure 3D). When combined with the QTL mapping region, four genes were identified within the RM1347–RM5356 interval. Among them, two genes (Os02g0181300 and Os02g0207400) exhibited relatively high expression fold changes across different treatment time points, with Os02g0181300 being up-regulated and Os02g0207400 down-regulated (Table 1). In order to further validate these findings, RT-qPCR (real time quantitative polymerase chain reaction) was performed for Os02g0181300 and Os02g0207400. The RT-qPCR results revealed expression patterns consistent with the RNA-seq data between IL43 and XZX45 (Supplementary Figure S1). Consequently, Os02g0181300 and Os02g0207400 were selected as candidate genes based on its higher expression fold change.
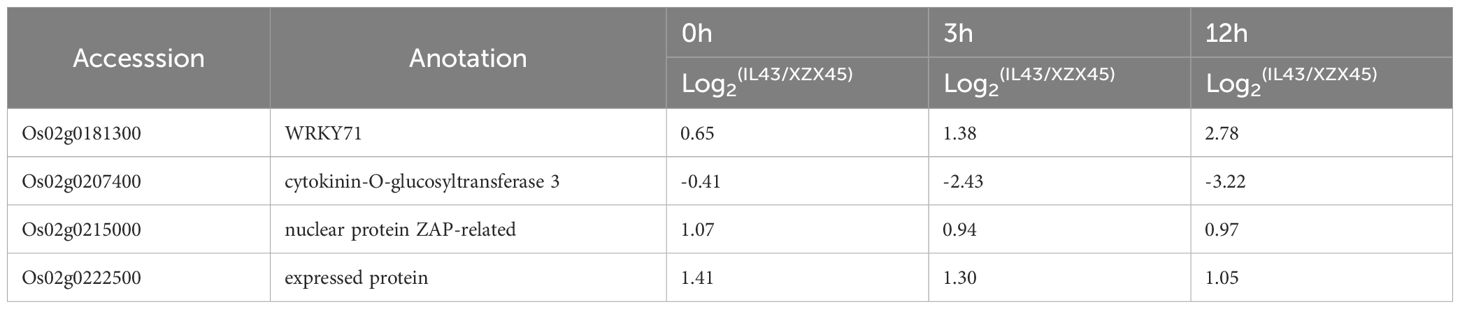
Table 1. Fold changes of differentially expressed genes (DEGs) in the interval of RM1347–RM5356 between IL43 and XZX45.
Functional validation of Os02g0181300 in cold tolerance
In order to further determine which of Os02g0181300 and Os02g0207400 is responsible for cold tolerance at the early seedling stage, each gene was separately knocked out in the Japonica rice variety Nipponbare (NIP) using Crisp/Cas9 technology. We obtained five transgenic lines for Os02g0181300 and four for Os02g0207400. Sequencing of the edited lines identified a homozygous line (NIPKO) with a 4-bp deletion in Os02g0181300 (Figure 4A). Evaluation of cold tolerance showed that the seedling survival rate of NIPKO was significantly lower than that of the wild-type following low-temperature treatment (Figures 4B, C). Besides, the homozygous seeds for the edited Os02g0207400 were not available from the segregating progeny of the four heterozgyous transgenic lines, we speculated that seeds could not survive in the absence of Os02g0207400. These results demonstrate that Os02g0181300 plays a critical role in cold tolerance at the early seedling stage. Os02g0181300 encoded a WRKY transcription factor and has been annotated as OsWRKY71. OsWRKY71 was reported to be involved in rice defense response and cold-responsive at the seedling stage (Liu et al., 2007; Kim et al., 2016).
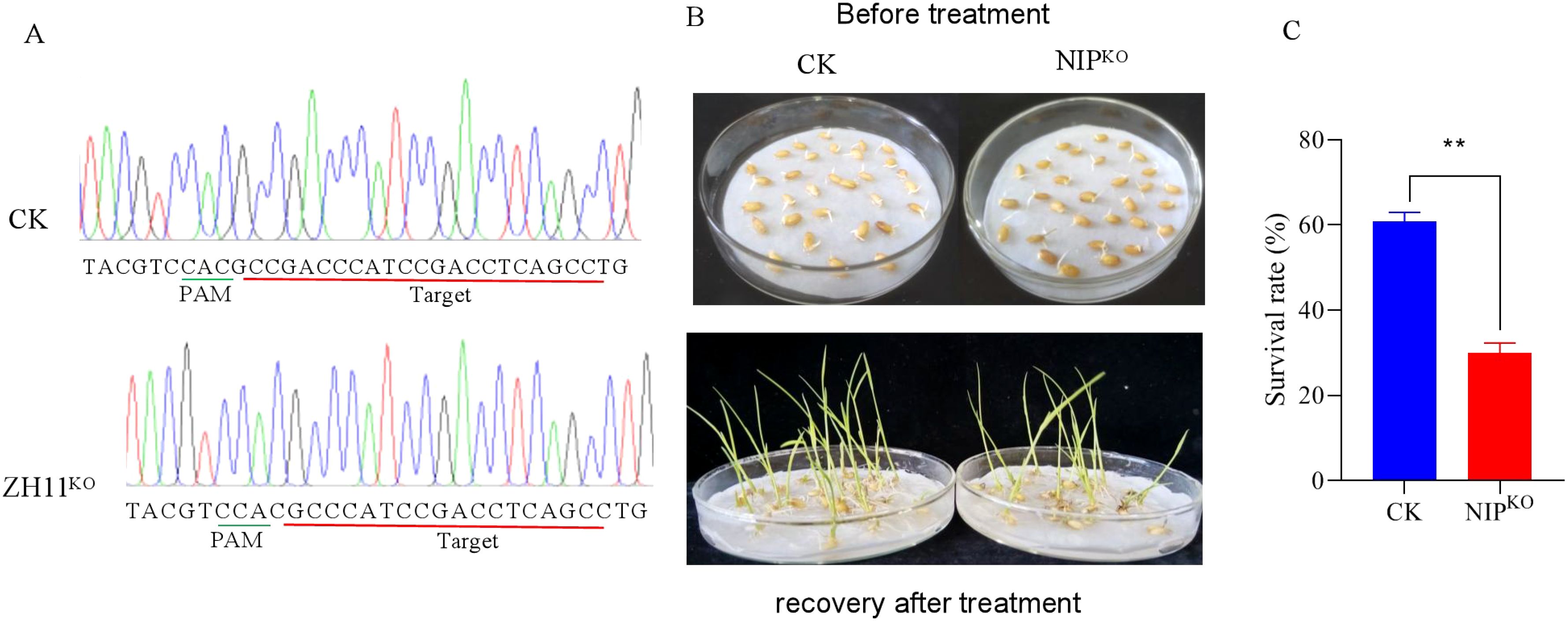
Figure 4. CRISPR/Cas9 knockout of Os02g0181300 (NIPKO) in rice. (A) Targeted mutation in Os02g0181300. (B) Phenotypic comparison of wild-type and NIPKO after cold treatment at 4°C for 5 days followed by a 7-day recovery. (C) Seedling survival rates of NIPKO and wild-type plants. ** significant difference at P <0.01 level.
Allelic variation and haplotype analysis of OsWRKY71
To further investigate the potential role of OsWRKY71 in cold tolerance, the alleles from IL43 and XZX45 were cloned and sequenced. Sequence comparison revealed two single nucleotide polymorphisms (SNPs) and one 3-bp insertion/deletion (INDEL) in the coding regions of OsWRKY71. These variations resulted in two amino acid changes and one amino acid deletion in IL43 (Figure 5), suggesting that these variations likely contributed to the varying level of cold tolerance.
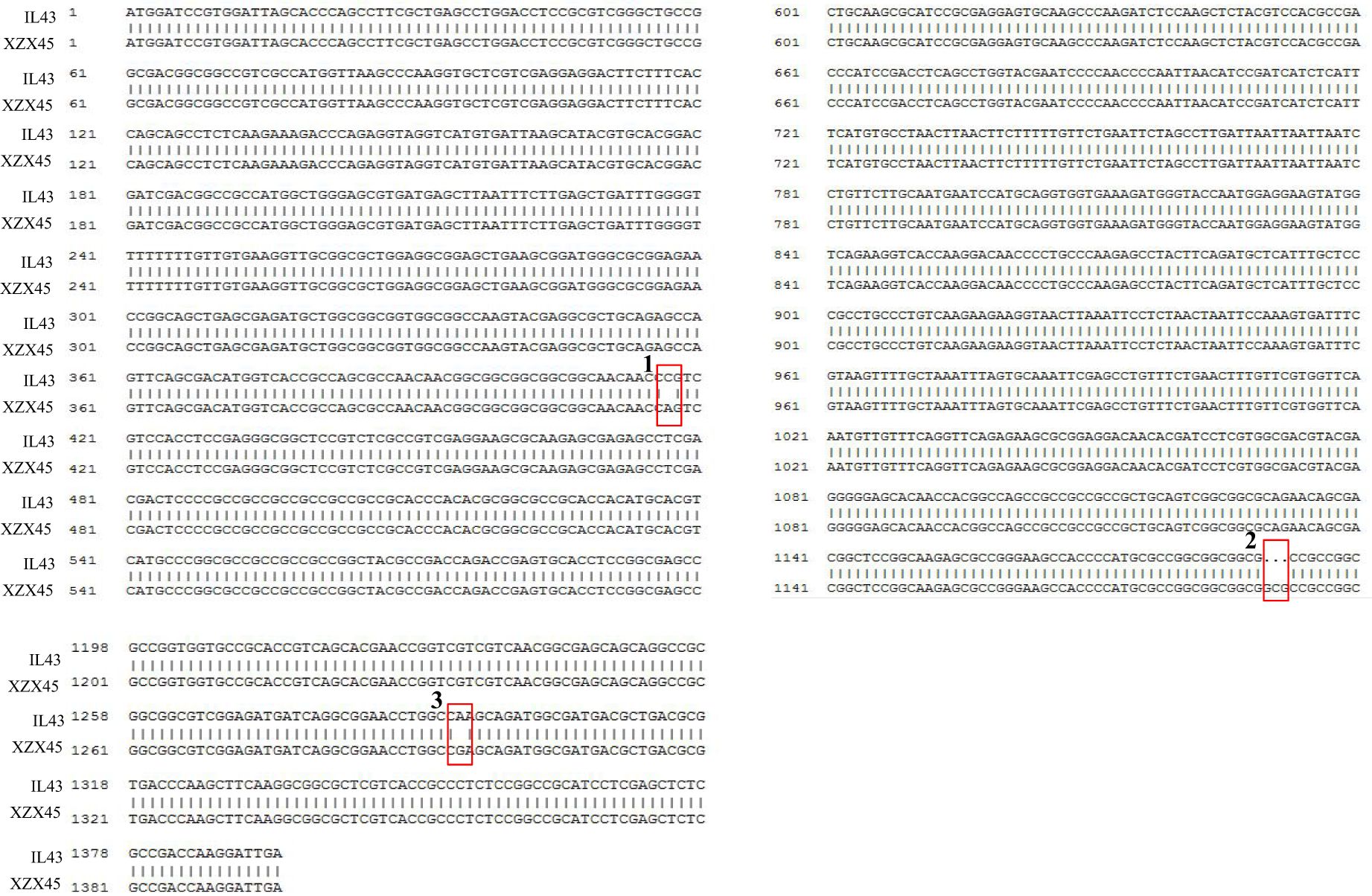
Figure 5. Sequence alignment of the coding region of Os02g0181300 between IL43 and XZX45. In red rectangular box 1, the nucleotide has a substitution from C in IL43 to A in XZX45, resulting in an amino acid change from proline (P) to glutamine (Q). In red rectangular box 2, a 3-bp deletion in IL43 result in an amino acid deletion of alanine (A). In red rectangular box 3, the nucleotide has a substitution from C in IL43 to A in XZX45, resulting in an amino acid change from lysine (K) to glutamic acid (E).
To further clarify the haplotypes of OsWRKY71, natural variations in the coding region of the gene was subsequently investigated from the website (http://ricevarmap.ncpgr.cn/). As a result, a total of five sites with amino acid changes were identified among 4696 rice accessions. Based on these polymorphisms, sequences of these accessions were categorized into five haplotypes. Among these, Hap1,Hap2 and Hap5 were predominantly presented in japonica cultivar, while Hap4 was almostly exclusively found in indica cultivar. Notably, Hap3 was observed in both indica and japonica cultivar, representing approximately half of total rice accessions (Figure 6). Hap3 appeared to be undergoing differentiation toward indica and japonica subspecies. The IL43 allele was found to belong to Hap1, while the XZX45 allele was classified as Hap3. These findings suggest that IL43 allele could be utilized to improve cold tolerance at the early seedling stage in rice.
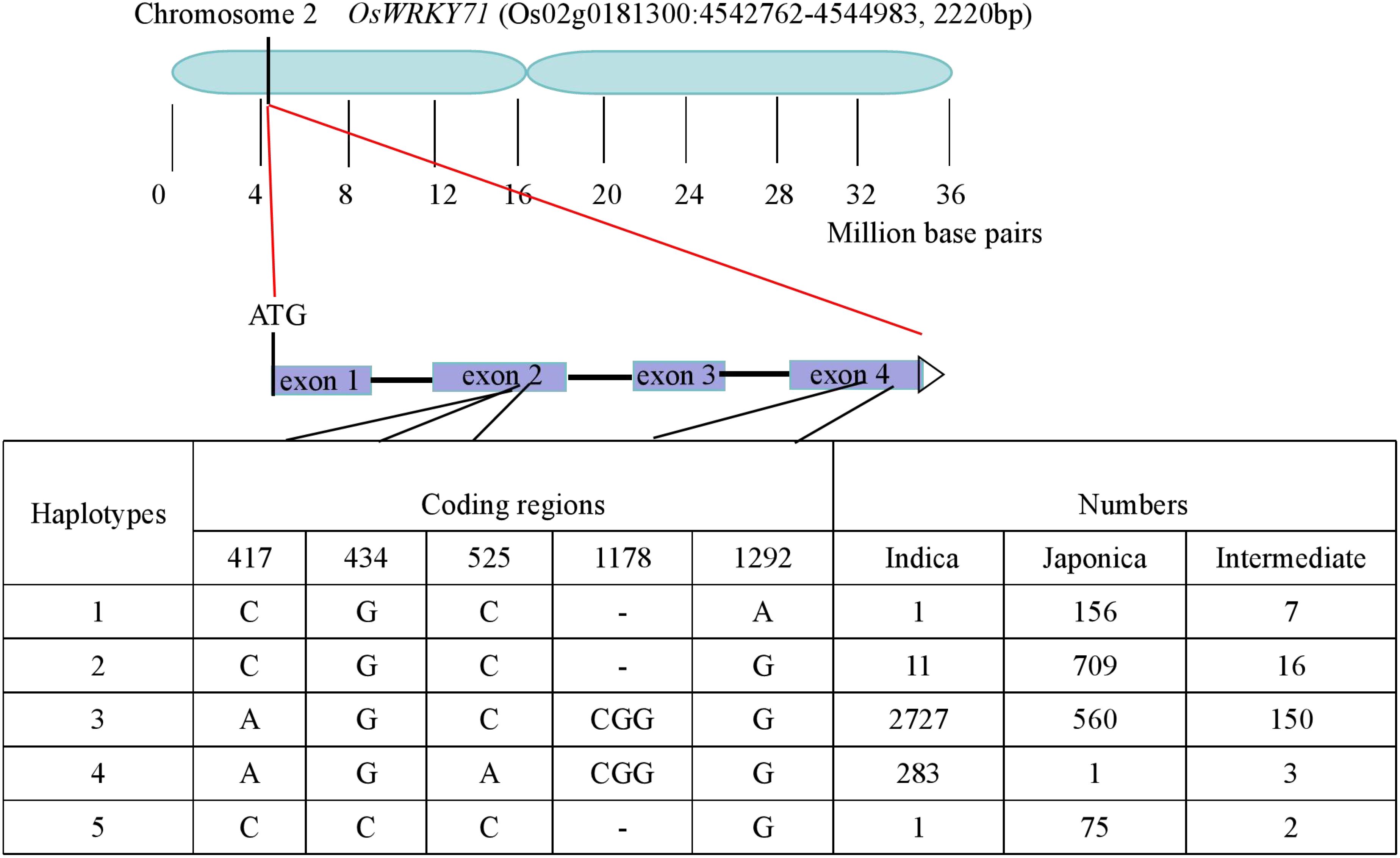
Figure 6. Haplotype analysis of OsWRKY71 according to the data from the website (http://ricevarmap.ncpgr.cn/).
Regulatory network underlying OsWRKY71-mediated cold tolerance
To elucidate the regulatory network underlying OsWRKY71-mediated cold tolerance, we conducted transcriptome analysis to identify DEGs between IL43 and XZX45 after cold treatment. GO enrichment analysis of these DEGs revealed significant terms in the cellular component category, including “respiratory chain,” “plasmodesma,” “extracellular region,” “Noc1p-Noc2p complex,” and “Noc2p-Noc3p complex” (Figure 7A). In the biological process category, enriched terms encompassed “plant-type primary cell wall biogenesis,” “response to water deprivation,” “glutathione metabolic process,” “glutamine biosynthetic process,” and “response to cold” (Figure 7B). For molecular function, enriched terms included “cellulose synthase activity,” “glutamate-ammonia ligase activity,” “hydroperoxide dehydratase activity,” “oxidoreductase activity,” and “cellulose synthase (UDP-forming) activity” (Figure 7C). These findings suggest that OsWRKY71 may regulate cellulose synthase activity and modulate glutamine and glutathione metabolism in rice. To further explore pathways associated with OsWRKY71, we mapped the DEGs in the KEGG database and performed pathway enrichment analysis. The top five enriched pathways were “Alanine, aspartate, and glutamate metabolism,” “Carbon fixation in photosynthetic organisms,” “Glutathione metabolism,” “Nitrogen metabolism,” and “Tyrosine metabolism” (Figure 7D). Therefore, we speculated that glutathione metabolism might involved in cold tolerance pathway.
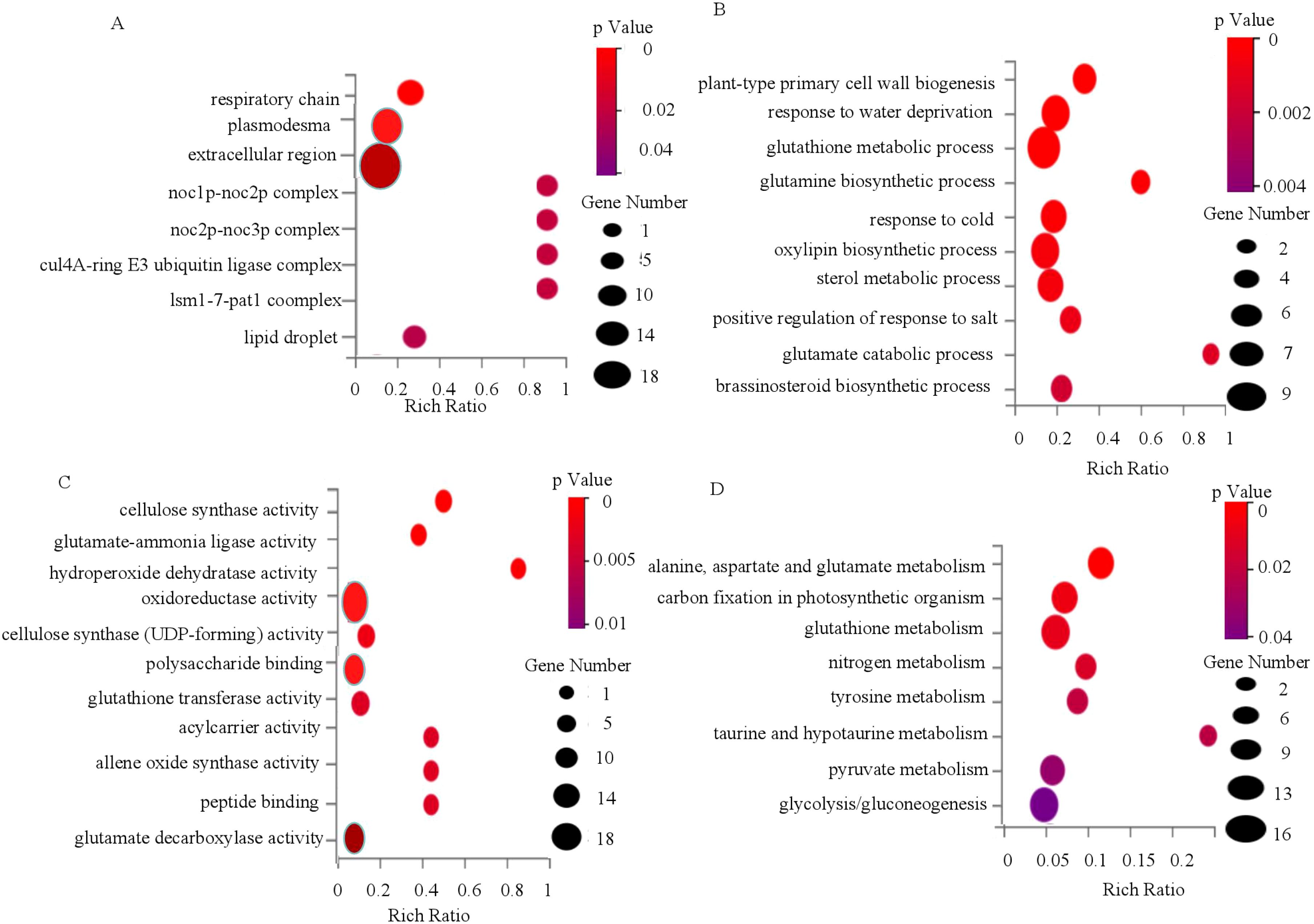
Figure 7. GO and KEGG enrichment analysis of DEGs between XZX45 and IL43 under cold stress. (A) Enrichment analysis of DEGs in the cellular component category. (B) Enrichment analysis of DEGs in the biological process category. (C) Enrichment analysis of DEGs in the molecular function category. (D) KEGG enrichment analysis of DEGs between XZX45 and IL43.
To further validate it, some gultathione-related genes, such as encoded glutathione S-transferase and hydroxymethyl-glutathione synthetase, were investigated for their expression level in IL43 and XZX45 after cold treatment. The results revealed that these genes showed significantly differential expression (Figure 8). Collectively, the transcriptomic data suggests a potential link between OsWRKY71 and glutathione metabolism, but further biochemical validation is required.
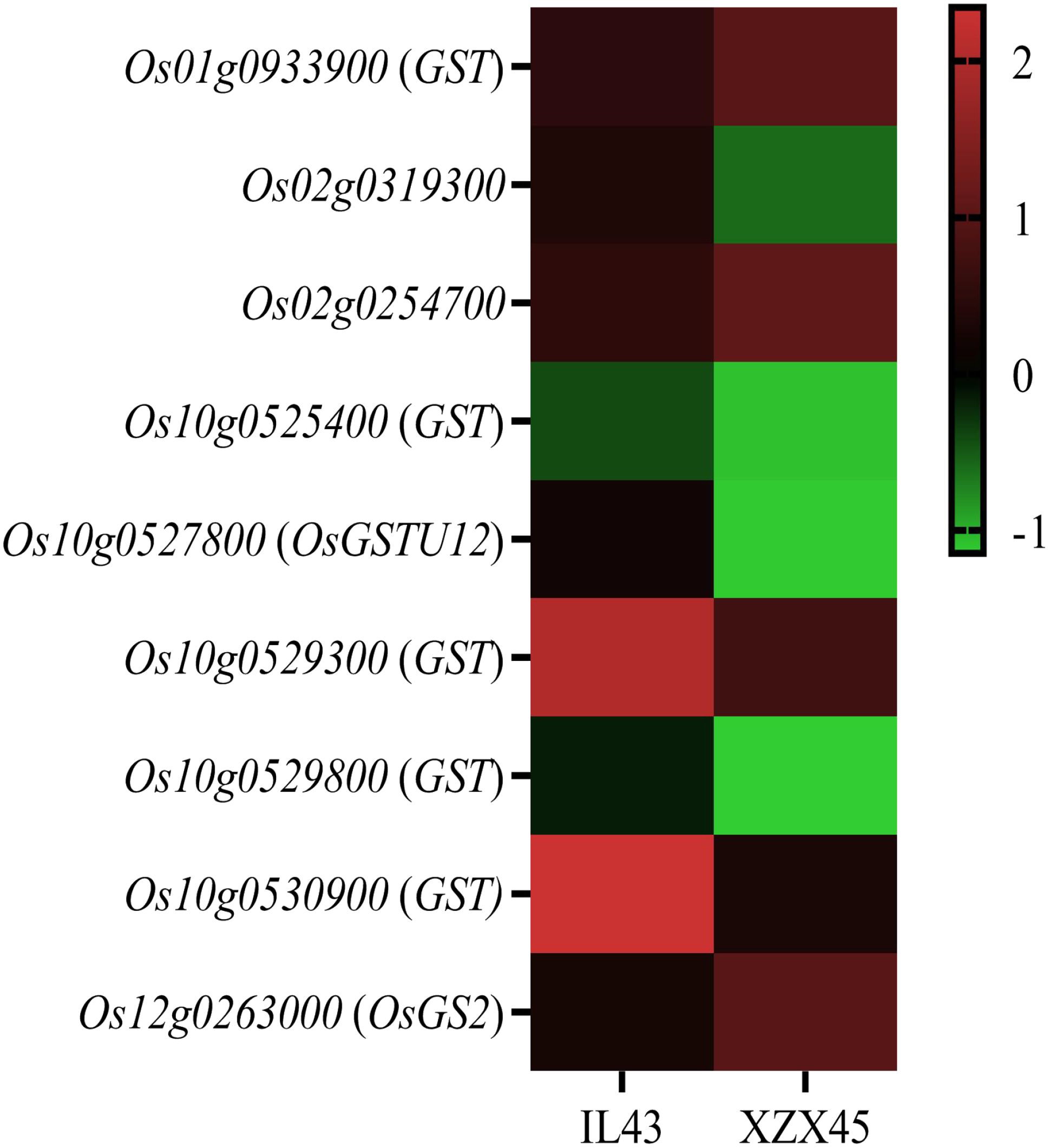
Figure 8. Heat map of glutathione metabolism related genes from buds subject to cold tolerance at 4°C between IL43 and ZXZ45. GST glutathione S-transferase.
Discussion
Genetic identification of quantitative trait loci (QTL) associated with complex traits often involves delimiting QTL into small intervals using molecular markers. The next challenge is determining the target genes within these intervals. RT-qPCR is commonly used to analyze the expression levels of genes within these regions to identify candidate genes. With the advent of high-throughput expression profiling and the construction of RNA-Seq libraries for rice exposed to various stress conditions—such as cold and salt stress—it has become increasingly feasible to identify stress-induced genes within target regions (Liu et al., 2013). When comparing two parents with contrasting genetic backgrounds, a large number of DEGs are typically observed, which can help elucidate the overall mechanism underlying the trait of interest. However, using a pair of NILs that share nearly identical genetic backgrounds, except for a few donor segments, makes it easier to pinpoint candidate genes within target regions based on changes in expression levels. In this study, IL43, an introgression line, was developed through marker-assisted backcrossing using XN1 as the donor parent and XZX45 as the recurrent parent. Genome-wide detection with SSR markers confirmed that IL43 carried only two donor segments. Compared to XZX45, IL43 exhibited significantly higher cold tolerance at the early seedling stage. As expected, a QTL associated with cold tolerance at this stage was detected in the interval of RM1347–RM5356 in the donor segment, using an F2:3 population derived from the cross between IL43 and XZX45. The allele from the donor parent increased cold tolerance. By integrating transcriptomic data, only four genes within the RM1347–RM5356 interval showed differential expression. Among these, OsWRKY71 was validated to be responsible for cold tolerance at the early seedling stage. This study demonstrates that combining RNA-Seq with linkage mapping not only accelerate the identification of candidate genes but also provide a more efficient and precise method for dissecting complex quantitative traits.
Cold tolerance in plant is a complex regulatory network involving multiple genes and pathways. These genes interact in intricate ways to help plants sense, respond to, and survive under cold stress. Currently, most studies have focused on the ICEs (inducer of CBF expression)-CBF/DREB1s (C-repeat binding factor/dehydration-responsive element binding protein 1)-CORs (cold-regulated) signaling pathway (Chinnusamy et al., 2007; Zarka et al., 2003). In this pathway, ICE proteins directly bind the promoters of CBF/DREB1 genes to positively regulate their expression. Subsequently, CBF/DREB1 proteins activate the expression of COR genes, triggering a signaling network that enhances cold tolerance (Guo et al., 2018). In addition to the ICE-CBF/DREB1-COR pathway, other transcription factors have been reported to play roles in cold tolerance. The bZIP73Jap interacts with bZIP71 to regulate the expression of qLTG3-1NIP, overexpression lines of qLTG3-1Nip greatly improved rice tolerance to cold stress (Liu et al., 2019). CTB5, a homeodomain-leucine zipper (HD-Zip) transcription factor, interacts with OsHox12 and targets gibberellin (GA) metabolism genes to promote GA accumulation in anthers, facilitating tapetum development under cold stress (Guo et al., 2025). CTB3, a calmodulin-binding transcriptional activator, activates the expression of trehalose-6-phosphate phosophatase1 (OsTPP1), reducing trehalose 6-phosphate (Tre6P) levels. This leads to increase sugar accumulation in panicles and improved cold tolerance. In this study, Os02g0181300 encodes a WRKY71 transcription factor, a member of the WRKY family. Rice WRKY genes are known to be involved in phytohormone signaling pathways, including abscisic acid (ABA) (Xie et al., 2005) and GA (Zhang et al., 2004). Some WRKY genes, such as OsWRKY45 (Tao et al., 2011), OsWRKY71 (Kim et al., 2016) and OsWRKY24 (Li J. et al., 2023), showed responsive expression in response to cold stress. Although previous studies have demonstrated that OsWRKY71 was responsive to cold stress at the seedling stage (Kim et al., 2016), more validation is lack. Here, OsWRKY71 was identified to be responsible for cold tolerance at the early seedling stage. These results indicate that OsWRKY71 likely plays a role both at the early seedling and at the seedling stage. However, the mechanisms by which OsWRKY71 regulates cold tolerance, including the potential involvement of ABA or GA signaling pathways, remain to be further elucidated.
When plants are exposed to cold stress, cellular homeostasis can be disrupted, leading to the overproduction of reactive oxygen species (ROS). In plants, ROS are highly toxic at elevated concentrations and must be tightly regulated through a balance between production and scavenging systems (Qi et al., 2017). To counteract oxidative damage, plants have evolved a suite of scavenging enzymes, including ascorbate peroxidase, superoxide dismutase, catalase, and glutathione peroxidase (Foyer and Noctor, 2005). Glutathione, a tripeptide composed of glutamic acid, cysteine, and glycine, plays a central role in plant responses to abiotic stresses, including cold tolerance. As a key component of the cellular antioxidant system, glutathione helps mitigate the harmful effects of ROS generated under cold stress. In this study, glutathione and glutathione-related metabolic pathways were frequently enriched in both KEGG and GO analyses. These findings suggest that glutathione and its associated metabolism may play a critical detoxifying role in defending against chilling stress. However, the involvement of glutathione-related genes in cold tolerance regulation still requires further verification.
Owing to the long-term domestication and natural selection process, during which indica and japonica rice have adapted to distinct ecological environments and climatic conditions, most cold-tolerance genes display significant differentiation between the indica and japonica subspecies. For example, genes such as HAN1 (Mao et al., 2019), bZIP73 (Liu et al., 2019), COLD1 (Ma et al., 2015), and CTB4a (Zhang et al., 2017) exhibit favorable haplotypes that are specifically present in japonica rice and significantly enhance cold tolerance. Like other cold-tolerance genes, OsWRKY71 exhibits clear differentiation between indica and japonica subspecies in this paper. The favorable haplotye (Hap1) was present in cold-tolerant japonica rice and showed enhanced cold tolerance at the early seedling stage. This finding offers a valuable genetic resource for enhancing cold tolerance of early-season rice varieties.
Data availability statement
Publicly available datasets were analyzed in this study. This data can be found here: https://rapdb.dna.affrc.go.jp/download/irgsp1.html.
Author contributions
WL: Conceptualization, Data curation, Formal Analysis, Funding acquisition, Writing – original draft. ZC: Investigation, Resources, Validation, Writing – review & editing. LG: Investigation, Resources, Validation, Writing – review & editing. ZD: Formal Analysis, Validation, Writing – review & editing. BY: Methodology, Supervision, Writing – review & editing. LL: Writing – review & editing, Investigation, Validation. SL: Investigation, Software, Writing – review & editing. XP: Writing – review & editing, Conceptualization, Formal Analysis, Funding acquisition, Methodology, Supervision, Visualization.
Funding
The author(s) declare that financial support was received for the research and/or publication of this article. This work was funded by the National Nature Science Foundation (32171998 and 32341026) and Hunan Provincial Science and Technology Innovation Program, China (2023NK1010).
Conflict of interest
The authors declare that the study was carried out without any commercial or financial ties that might be perceived as a potential conflict of interest.
Generative AI statement
The author(s) declare that no Generative AI was used in the creation of this manuscript.
Publisher’s note
All claims expressed in this article are solely those of the authors and do not necessarily represent those of their affiliated organizations, or those of the publisher, the editors and the reviewers. Any product that may be evaluated in this article, or claim that may be made by its manufacturer, is not guaranteed or endorsed by the publisher.
Supplementary material
The Supplementary Material for this article can be found online at: https://www.frontiersin.org/articles/10.3389/fpls.2025.1580022/full#supplementary-material
References
Andaya, V. C., Mackill, D. J. (2003). Mapping of QTLs associated with cold tolerance during the vegetative stage in rice. J. Exp. Bot. 54, 2579–2585. doi: 10.1093/jxb/erg243
Chinnusamy, V., Zhu, J., Zhu, J. K. (2007). Cold stress regulation of gene expression in plants. Trends Plant Sci. 12, 444–451. doi: 10.1016/j.tplants.2007.07.002
Dong, J. F., Zhang, S. H., Hu, H. F., Wang, J., Li, R. S., Wu, J., et al. (2025). Natural variation in CTF1 conferring cold tolerance at the flowering stage in rice. Plant Biotechnol. J. doi: 10.1111/pbi.14600
Foyer, C. H., Noctor, G. (2005). Redox homeostasis and antioxidant signaling: A metabolic interface between stress perception and physiological responses. Plant Cell 17, 1866–1875. doi: 10.1105/tpc.105.033589
Fujino, K., Sekiguchi, H., Matsuda, Y., Suginoto, K., Ono, K., Yano, M. (2008). Molecular identification of a major quantitative trait locus, qLTG3-1, controlling low-temperature germinability in rice. Proc. Nat. Acad. Sci. 105, 12623–12628. doi: 10.1073/pnas.0805303105
Fujino, K., Sekiguchi, H., Sato, T., Kiuchi, H., Nonoue, Y., Takeuchi, Y., et al. (2004). Mapping of quantitative trait loci controlling low-temperature germinability in rice (Oryza sativa L.). Theor. Appl. Genet. 108, 794–799. doi: 10.1007/s00122-003-1509-4
Gao, S. L., Li, J., Zeng, Y. W., Li, H. H., Guo, Z. H., Guo, H. F., et al. (2025). CTB6 confers cold tolerance at the booting stage by maintaining tapetum development in rice. Adv. Sci., 2411357. doi: 10.1002/advs.202411357
Guo, H. F., Gao, S. L., Li, H. H., Yang, J. Z., Jin Li, J., Gu, Y. S., et al. (2025). Natural variation of CTB5 confers cold adaptation in plateau japonica rice. Nat. Commun. 16, 1032. doi: 10.1038/s41467-025-56174-5
Guo, X. Y., Liu, D. F., Chong, K. (2018). Cold signaling in plants:insights into mechanisms and regulation. J. Integr. Plant Biol. 60, 745–756. doi: 10.1111/jipb.12706
Han, L. Z., Qiao, Y. L., Zhang, S. Y., Zhang, Y. Y., Cao, G. L., Kim, J. W., et al. (2007). Identification of quantitative trait loci for cold response of seedling vigor traits in rice. J. Genet. Genomics 34, 239–246. doi: 10.1016/S1673-8527(07)60025-3
Jiang, L., Xun, M. M., Wang, J. K., Wan, J. M. (2008). QTL analysis of cold tolerance at seedling stage in rice (Oryza sativa L.) using recombination inbred lines. J. Cereal Sci. 48, 173–179. doi: 10.1016/j.jcs.2007.08.013
Kim, C. Y., Vo, K. T. X., Nguyen, C. D., Jeong, D. H., Lee, S. K., Kumar, M., et al. (2016). Functional analysis of a cold-responsive rice WRKY gene, OsWRKY71. Plant Biotechnol. Rep. 10, 13–23. doi: 10.1007/s11816-015-0383-2
Li, J. D., Chen, Y. T., Zhang, R., Wu, B., Xiao, G. Q. (2023). Expression identification of three OsWRKY genes in response to abiotic stress and hormone treatments in rice. Plant Signal. Behav. 18. doi: 10.1080/15592324.2023.2292844
Li, J., Guo, H. F., Lou, Q. J., Zeng, Y. W., Guo, Z. H., Xu, P. H., et al. (2025). Natural variation of indels in the CTB3 promoter confers cold tolerance in japonica rice. Nat. Commun. 16, 1613. doi: 10.1038/s41467-025-56992-7
Li, C. J., Liu, J. D., Bian, J. X., Jin, T., Zou, B. L., Liu, S. L., et al. (2021). Identification of cold tolerance QTLs at the bud burst stage in 211 rice landraces by GWAS. BMC Plant Biol. 21, 1–11. doi: 10.1186/s12870-021-03317-7
Li, Z. T., Wang, B., Luo, W., Xu, Y. Y., Wang, J. J., Xue, Z. H., et al. (2023). Natural variation of codon repeats in COLD11 endows rice with chilling resilience. Sci. Adv. 9, 5506. doi: 10.1126/sciadv.abq5506
Lincoln, S. (1992). Constructing genetic maps with MAPMAKER/EXP 3.0 (Cambridge, Massachusetts, USA: Whitehead Institute technical report).
Liu, X. Q., Bai, X. Q., Wang, X. J., Chu, C. C. (2007). OsWRKY71, a rice transcription factor, is involved in rice defense response. J.Plant Physiol. 164, 969–979. doi: 10.1016/j.jplph.2006.07.006
Liu, W. Q., Lu, T. T., Li, Y. C., Pan, X. W., Duan, Y. H., Min, J. J., et al. (2015). Mapping of quantitative trait loci for cold tolerance at the early seedling stage in landrace rice xiang 743. Euphytica 201, 401–409. doi: 10.1007/s10681-014-1227-9
Liu, C. T., Schlppi, M. R., Mao, B. G., Wang, W., Wang, A. J., Chu, C. C. (2019). The bZIP73 transcription factor controls rice cold tolerance at the reproductive stage. Plant Biotechnol. J. 17, 1834–1849. doi: 10.1111/pbi.13104
Liu, F. X., Xu, W. Y., Song, Q., Tan, L. B., Liu, J. Y., Zhu, Z. F., et al. (2013). Microarray-assisted fine-mapping of quantitative trait loci for cold tolerance in rice. Mol. Plant 6, 757–767. doi: 10.1093/mp/sss161
Lou, Q. J., Chen, L., Sun, Z. X., Xing, Y. Z., Li, J., Xu, X. Y., et al. (2007). A major QTL associated with cold tolerance at seedling stage in rice (Oryza sativa L.). Euphytica 158, 87–94. doi: 10.1007/s10681-007-9431-5
Lu, G. W., Wu, F. Q., Wu, W. X., Wang, H. J., Zheng, X. M., Zhang, Y. H., et al. (2014). Rice LTG1 is involved in adaptive growth and fitness under low ambient temperature. Plant J. 78, 468–480. doi: 10.1111/tpj.2014.78.issue-3
Luo, W., Xu, Y. Y., Cao, J., Guo, X. Y., Han, J. D., Zhang, Y. Y. (2024). COLD6-OSM1 module senses chilling for cold tolerance via 2’,3’-cAMP signaling in rice. Mol. Cell 84, 4224–4238. doi: 10.1016/j.molcel.2024.09.031
Ma, Y., Dai, X. Y., Xu, Y. Y., Luo, W., Zheng, X. M., Zeng, D. L., et al. (2015). COLD1 confers chilling tolerance in rice. Cell 160, 1209–1221. doi: 10.1016/j.cell.2015.01.046
Mao, D. H., Xin, Y. Y., Tan, Y. J., Hu, X. J., Bai, J. J., Liu, Z, Y., et al. (2019). Natural variation in the HAN1 gene confers chilling tolerance in rice and allowed adaptation to a temperate climate. Proc. Nat. Acad. Sci. 116, 3494–3501. doi: 10.1073/pnas.1819769116
Miura, K., Lin, S. Y., Yano, M., Nagamine, T. (2002). Mapping quantitative trait loci controlling low temperature germinability in rice (Oryza sativa L.). Breed. Sci. 51, 293–299. doi: 10.1270/jsbbs.51.293
Park, I. K., Oh, C. S., Kim, D. M., Yeo, S. M., Ahn, S. N. (2013). QTL mapping of cold tolerance at the seedling stage using introgression lines derived from an intersubspecific cross in rice. Plant Breed. Biotechnol. 1, 1–8. doi: 10.9787/PBB.2013.1.1.001
Qi, J., Wang, J., Gong, Z., Zhou, J. M. (2017). Apoplastic ROS signaling in plant immunity. Curr. Opin. Plant Biol. 38, 92–100. doi: 10.1016/j.pbi.2017.04.022
Saito, K., Hayano-Saito, Y., Kuroki, M., Sato, Y. (2010). Map-based cloning of the rice cold tolerance gene Ctb1. Plant Sci. 179, 97–102. doi: 10.1016/j.plantsci.2010.04.004
Saito, K., Hayano-Saito, Y., Maruyama-Funatsuki, W., Sato, Y., Kato, A. (2004). Physical mapping and putative candidate gene identification of a quantitative trait locus Ctb1 for cold tolerance at the booting stage of rice. Theor. Appl. Genet. 109, 515–522. doi: 10.1007/s00122-004-1667-z
Saito, K., Miura, K., Nagano, K., Hayano-Saito, Y., Saito, A., Araki, H., et al. (1995). Chromosomal location of quantitative trait loci for cold tolerance at the booting stage in rice variety ‘Norin-PL8’. Breed. Sci. 45, 337–340.
Song, J. Y., Li, J. Q., Sun, J., Hu, T., Wu, A. T., Liu, S. T., et al. (2018). Genome-wide association mapping for cold tolerance in a core collection of rice (Oryza sativa L.) landraces by using high-density single nucleotide polymorphism markers from specific-locus amplified fragment sequencing. Front. Plant Sci. 9. doi: 10.3389/fpls.2018.00875
Suh, J. P., Lee, C. K., Lee, J. H., Kim, J. J., Kim, S. M., Cho, Y. C., et al. (2012). Identification of quantitative trait loci for seedling cold tolerance using RILs derived from a cross between japonica and tropical japonica rice cultivars. Euphytica 184, 101–108. doi: 10.1007/s10681-011-0575-y
Tang, Q. Y., Feng, M. H. (2007). DPS data processing system: experimental design, statistical analysis, and data mining. Version 2.0 (Beijing: Science Press).
Tao, Z., Kou, Y. J., Liu, H. B., Li, X. H., Xiao, J. H., Shiping Wang, S. P. (2011). OsWRKY45 alleles play different roles in abscisic acid signalling and salt stress tolerance but similar roles in drought and cold tolerance in rice. J.Exp.Bot. 62, 4863–4874. doi: 10.1093/jxb/err144
Wang, S., Basten, C., Zeng, Z. (2008). Windows QTL cartographer 2.5 department of statistics (Raleigh, North Carolina, USA: North Carolina State University).
Xie, Z., Zhang, Z. L., Zou, X. L., Huang, J., Ruas, P., Thompson, D., et al. (2005). Annotations and functional analyses of the rice WRKY gene superfamily reveal positive and negative regulators of abscisic acid signaling in aleurone cells. Plant Physiol. 137, 176–189. doi: 10.1104/pp.104.054312
Zarka, D. G., Vogel, J. T., Cook, D., Thomashow, M. F. (2003). Cold induction of Arabidopsis CBF genes involves multiple ICE (inducer of CBF expression) promoter elements and a cold-regulatory circuit that is desensitized by low temperature. Plant Physiol. 133, 910–918. doi: 10.1104/pp.103.027169
Zhang, Z. Y., Li, J. J., Pan, Y. H., Li, J. L., Zhou, L., Shi, H. L., et al. (2017). Natural variation in CTB4a enhances rice adaptation to cold habitats. Nat. Commun. 8, 14788. doi: 10.1038/ncomms14788
Keywords: rice (Oryza sativa L.), cold tolerance, RNA-seq, quantitative trait loci (QTL), haplotype, transcription factor
Citation: Liu W, Chen Z, Guo L, Dong Z, Yang B, Liu L, Liu S and Pan X (2025) Integrating RNA-Seq and linkage mapping to identify and characterize qESCT2, a cold tolerance QTL at the early seedling stage in rice. Front. Plant Sci. 16:1580022. doi: 10.3389/fpls.2025.1580022
Received: 20 February 2025; Accepted: 07 April 2025;
Published: 01 May 2025.
Edited by:
Kai-Hua Jia, Shandong Academy of Agricultural Sciences, ChinaReviewed by:
Ahmed Adel Khatab, Agricultural Research Center, EgyptBiaolin Hu, Jiangxi Acadmy of Agricultural Sciences, China
Ravikiran K T, ICAR-Central Soil Salinity Research Statino, India
Leiyue Geng, Hebei Academy of Agricultural and Forestry Sciences, China
Copyright © 2025 Liu, Chen, Guo, Dong, Yang, Liu, Liu and Pan. This is an open-access article distributed under the terms of the Creative Commons Attribution License (CC BY). The use, distribution or reproduction in other forums is permitted, provided the original author(s) and the copyright owner(s) are credited and that the original publication in this journal is cited, in accordance with accepted academic practice. No use, distribution or reproduction is permitted which does not comply with these terms.
*Correspondence: Xiaowu Pan, cGFueGlhb3d1QGh1bmFhcy5jbg==