- 1National Engineering Laboratory for Resource Development of Endangered Crude Drugs in Northwest China, Key Laboratory of Medicinal Resources and Natural Pharmaceutical Chemistry, Ministry of Education, College of Life Sciences, Shaanxi Normal University, Xi’an, China
- 2Laboratory of Medicinal Plant, Institute of Basic Medical Sciences, School of Basic Medicine, Biomedical Research Institute, Hubei Key Laboratory of Wudang Local Chinese Medicine Research, Hubei University of Medicine, Shiyan, China
- 3Key Laboratory of Plant Development and Environment Adapting Biology, Ministry of Education, School of Life Science, Shandong University, Qingdao, China
- 4Hubei Key Laboratory of Embryonic Stem Cell Research, Hubei University of Medicine, Shiyan, China
Strigolactones (SLs) are known to mediate plant acclimation to environmental stress. We recently reported that SLs acted as prominent regulators in promotion of stomatal closure. However, the detailed mechanism by which SLs induce stomatal closure requires further investigation. Here we studied the essential role of the calcium (Ca2+) signal mediating by the calcium-dependent protein kinase (CPK) in SL-induced stomatal closure. SL-induced stomatal closure was strongly inhibited by a Ca2+ chelator and Ca2+ channel blockers, indicating that Ca2+ functions in SL promotion of stomatal closure. Through examining a collection of cpk mutants, we identified CPK33, potentially acting as a Ca2+ transducer, which is implicated in guard cell SL signaling. SL- and Ca2+-induced stomatal closure were impaired in cpk33 mutants. CPK33 kinase activity is essential for SL induction of stomatal closure as SL-induced stomatal closure is blocked in the dead kinase mutant of CPK33. The cpk33 mutant is impaired in H2O2-induced stomatal closure, but not in SL-mediated H2O2 production. Our study thus uncovers an important player CPK33 which functions as an essential Ca2+ signals mediator in Arabidopsis guard cell SL signaling.
Introduction
Plants are sessile organisms that confront with a wide range of biotic and abiotic stress conditions during their life cycle. As a strategy to cope with environmental stress, plants utilize stomatal pores, each consisting of a pair of guard cells, that open and close to modulate gas exchange for photosynthesis, transpirational water loss, and stomatal immunity, thereby allowing plants to respond properly to diverse environmental stress (Hetherington and Woodward, 2003; Ruszala et al., 2011; Blatt et al., 2017; Melotto et al., 2017). Guard cells have developed sophisticated mechanisms which enable plants to appropriately control of stomatal apertures in mediating response of environmental stimuli such as light, drought, and external calcium ion (Ca2+) (Kim et al., 2010; Murata et al., 2015). Particularly, phytohormones, including abscisic acid (ABA), ethylene, brassinosteroids, strigolactones (SLs), salicylic acid (SA), jasmonic acid (JA), and small signaling peptides have been found to play pivotal roles through their coordination with various key guard cell signaling components to modulate stomatal apertures in response to fluctuating environmental stress (Kim et al., 2010; Daszkowska-Golec and Szarejko, 2013; Munemasa et al., 2015; Murata et al., 2015; Cardinale et al., 2018; Mostofa et al., 2018; Qu et al., 2019; Zhang et al., 2019).
Other than their notable roles in shoot branching (Al-Babili and Bouwmeester, 2015), SLs have been found to be implicated in many plant developmental processes such as primary root development (Kapulnik et al., 2011; Ruyter-Spira et al., 2011), adventitious root formation (Rasmussen et al., 2012; Sun et al., 2015), secondary growth (Agusti et al., 2011), photomorphogenesis (Shen et al., 2007; Shen et al., 2012), flower development (Snowden et al., 2005; Kohlen et al., 2012; Liu et al., 2013), and hypocotyl elongation (Tsuchiya et al., 2010; Jia et al., 2014). Notably, accumulating data indicated that SLs are also involved in mediating plant responses to environmental stress, rendering plants to defend against abiotic stress as well as against specific bacterial and fungal species (Marzec, 2016; Cardinale et al., 2018; Mostofa et al., 2018). Specifically, SL-deficient and SL-signaling mutants exhibited drought hypersensitivity, whereas SLs application rescued drought-sensitive phenotypes of SL-deficient mutants and strengthened drought tolerance of wild-type (WT) plants (Bu et al., 2014; Ha et al., 2014; Liu et al., 2015; Li et al., 2017; Zhang et al., 2018). We recently revealed that SLs could induce stomatal closure through enhancing hydrogen peroxide (H2O2) and nitric oxide production in an ABA-independent manner, possibly preventing water loss and pathogen invasion and thereby resulting in plant acclimation to environmental stress (Lv et al., 2018; Zhang et al., 2018). However, the detail molecular mechanism, especially the intracellular events that are initiated by SLs in guard cells, remains largely unclear. To this end, the potential downstream component(s) that transduces guard cell SL signaling is thus required to be determined.
It has long been known that calcium functions as a secondary messenger in stomatal closure (Blatt, 2000; Bowler and Fluhr, 2000; Kim et al., 2010; Murata et al., 2015; Ray, 2017). For instance, through H2O2 activation of Ca2+-permeable cation channels, ABA triggers an increment of cytosolic Ca2+ [(Ca2+)cyt] that includes Ca2+ influx elevation from extracellular spaces and Ca2+ release from intracellular stores (Pei et al., 2000). In addition, JA-induced stomatal closure is mediated by cytosolic Ca2+ since JA signaling in guard cells is inhibited by Ca2+ channel blockers (Suhita et al., 2003; Suhita et al., 2004). Likewise, Ca2+ signaling is found to be implicated in SA induction of stomatal closure in a mode of action similar to studies of ABA- and JA-mediated stomatal closure (Prodhan et al., 2018). Eventually, the resultant guard cell cytosolic Ca2+ elevation promotes stomatal closure by stimulation of SLOW ANION CHANNEL-ASSOCIATED 1 (SLAC1) anion channels and/or the GATED OUTWARDLY-RECTIFYING K+ (GORK) channel (Kim et al., 2010; Murata et al., 2015; Roux and Leonhardt, 2018).
Calcium-dependent protein kinases (CPKs) function as Ca2+ signal transducers involving in various biological processes including Ca2+-dependent guard cell signaling (Boudsocq and Sheen, 2013; Schulz et al., 2013; Simeunovic et al., 2016). Mechanically, CPKs are known to activate SLAC1 and GORK channels to induce stomatal closure (Kim et al., 2010; Boudsocq and Sheen, 2013; Schulz et al., 2013; Simeunovic et al., 2016; Corratgé-Faillie et al., 2017). To date, a number of CPKs have been identified to be implicated in guard cell signaling to mediate stomatal movement. Several CPKs, including CPK3, CPK4, CPK6, CPK8, CPK9, CPK10, CPK11, and CPK33, are involved in ABA-mediated stomatal closure through distinct modes of action (Boudsocq and Sheen, 2013; Schulz et al., 2013; Li C.L et al., 2016; Chen et al., 2019). For instance, CPK3 and CPK6 activated ABA-induced stomatal closure and slow-type (S-type) anion channel activity (Mori et al., 2006). Disruption of CPK6 impaired JA-mediated stomatal closure and S-type anion channels activation, implying that CPK6 acted as a positive regulator in guard cell MeJA signaling (Munemasa et al., 2011). CPK3 and CPK6 functioned additively in SA-induced stomatal closure and SA activation of S-type anion channels (Prodhan et al., 2018). CPK33 suppressed ABA-induced stomatal closure and S-type anion channel activity (Li C.L et al., 2016; Chen et al., 2019), whereas CPK33 stimulated GORK activity to promote stomatal closure (Corratgé-Faillie et al., 2017). It was reported that CPK10, possibly association with HEAT SHOCK PROTEIN 20-LIKE PROTEIN 1 (HSP1), functioned in ABA- and Ca2+-mediated stomatal closure in response to drought stress (Zou et al., 2010). Altogether, these findings underscore the importance of CPKs in the modulation of stomatal closure. CPKs and Ca2+-independent kinases [e.g. SnRK2-type protein kinase OPEN STOMATA1 (OST1)] have long been recognized to be involved in activating ion channels and stimulating stomatal closure (Geiger et al., 2009; Lee et al., 2009; Geiger et al., 2010; Geiger et al., 2011; Brandt et al., 2012; Brandt et al., 2015).
In this study, the importance of Ca2+ in SL-induced stomatal closure was firstly determined by performing pharmacological studies, which indicate that Ca2+ acts as a prominent mediator functions in SL induction of stomatal closure. Through examining a collection of cpk mutants, we identified CPK33 (and possibly CPK10) which is implicated in guard cell SL signaling. SL activation of stomatal closure, as well as Ca2+-induced stomatal closure, was greatly impaired in cpk33 mutants. Additionally, CPK33 kinase activity is essential for SL induction of stomatal closure. We further found that H2O2-induced stomatal closure was moderately impaired in cpk33 mutants whereas SL-mediated H2O2 production was maintained in cpk33 mutants. Taken together, our study uncovers an important player CPK33 that functions as an important mediator in SL signaling in Arabidopsis guard cells.
Materials and Methods
Plant Growth Conditions and Mutants Isolation
The Arabidopsis ecotype Columbia-0 (Col-0) was used as wild-type (WT) plants in this study. The following mutants have been described previously: max1-1, max2-1, max2-2, max3-9, and max4-1 (Stirnberg et al., 2002; Umehara et al., 2008), d14-5 (Yao et al., 2016), cpk4-1 and cpk11-2 (Zhu et al., 2007), cpk8 (Zou et al., 2015), cpk10 (Zou et al., 2010), cpk23 (Ma and Wu, 2007), cpk33-1, cpk33-2, 35S::CPK33 and 35S::CPK33K102R (Li C.L et al., 2016), cpk3 cpk5 cpk6 cpk11 (Guzel Deger et al., 2015), and cpk5 cpk6 cpk11 cpk23 (Wang et al., 2018).
To obtain the double mutant cpk10 cpk33, the cpk10 mutant and cpk33 mutants (cpk33-1 and cpk33-2) were crossed. Homozygous double mutants were determined by PCRs using a combination of a gene-specific primer and a T-DNA border primer. The primers used were listed in Table S1.
Seeds were sterilized and sown for germination in 1/2 Murashige and Skoog (MS) medium supplemented with 0.8% (w/v) agar and 1% sucrose (w/v). Seedlings were transplanted into pots and subsequently kept in a growth chamber with a 16h/8h (light/darkness) regime at 21°C. Fully expanded rosette leaves detached from 4- to 6-week-old healthy plants were harvested for immediate use.
Stomatal Aperture Bioassay
Stomatal apertures were measured as described previously (Lv et al., 2018; Zhang et al., 2019). In brief, epidermal strips of fully expanded leaves were incubated in the MES-KCl buffer (10 mM MES-KOH/50 mM KCl, pH 6.15) to promote stomatal opening following treatments as described in each experiment. Finally, stomatal apertures on the abaxial epidermis were measured and presented as mean ± SE of three replicates.
Chemicals
The preparation of synthetic SL analog GR24 (Chiralix Nijmegen, the Netherlands) and 2′,7′-dichlorofluorescein diacetate (H2DCF-DA; Biotium Hayward, USA) were performed as described previously (Lv et al., 2018; Zhang et al., 2019). The ethylene glycol-bis(β-aminoethyl ether)-N,N,N′,N′-tetra acetic acid (EGTA; Sigma, USA), lanthanum chloride (LaCl3; Solarbio, China), aluminum chloride (AlCl3, Solarbio, China), trifluoperazine (TFP), and trifluoperazine dihydrochloride (TFP; Santa Cruz, USA) were dissolved appropriately according to the supplier information and prepared stock solutions for further use. Other chemicals used in this study, including H2O2, 2-(N-morpholino) ethanesulphonic acid (MES), and calcium chloride (CaCl2), were purchased from Sigma-Aldrich. All chemicals used are of the highest analytical grade.
H2O2 Content Detection in Guard Cells
H2DCF-DA was utilized to determine H2O2 content in stomata. The measurement of H2O2 content was performed according to Lv et al. (2018) and Zhang et al. (2019). Briefly, epidermal strips with open stomata were incubated in the Tris-KCl solution containing 50 µM H2DCF-DA for 10 min of darkness at 25°C in the absence or presence of GR24. Epidermal strips were washed three times with Tris-KCl buffer to remove excess dye under darkness. The fluorescence in stomata was visualized using a TCS SP2 confocal laser scanning microscope (Leica Lasertechnik GmbH, Germany). The fluorescence intensity representing the endogenous H2O2 content was determined using ImageJ software. Fluorescence intensities were normalized to those of controls. The data for fluorescence intensities represent the mean ± SE of three replicates.
Statistical Analyses
Statistical analyses were performed using a one-way ANOVA to discriminate significant differences followed by the least significant difference test.
Results
Ca2+ Is Required for SL-Induced Stomatal Closure in Arabidopsis
Calcium is known to act as a key secondary messenger in mediating stomatal closure (Blatt, 2000; Bowler and Fluhr, 2000; Pei et al., 2000; Kim et al., 2010; Murata et al., 2015; Ray, 2017). We therefore took a pharmacological approach to determine whether Ca2+ is required for SL-triggered stomatal closure. To this end, we examined the effects of ethylene glycol-bis(β-aminoethyl ether)-N,N,N′,N′-tetra acetic acid (EGTA; a Ca2+ chelator), and LaCl3 and AlCl3 (Ca2+ channel blockers) on SL-induced stomatal closure (Schwartz, 1985; Zhao et al., 2007; Li Y et al., 2016). As expected, the synthetic SL analog GR24 could significantly induce stomatal closure (Figure 1; Lv et al., 2018). However, SL-induced stomatal closure was inhibited by EGTA, LaCl3, and AlCl3, respectively (Figure 1). These results indicate that Ca2+ and Ca2+ channels are involved in SL-induced stomatal closure.
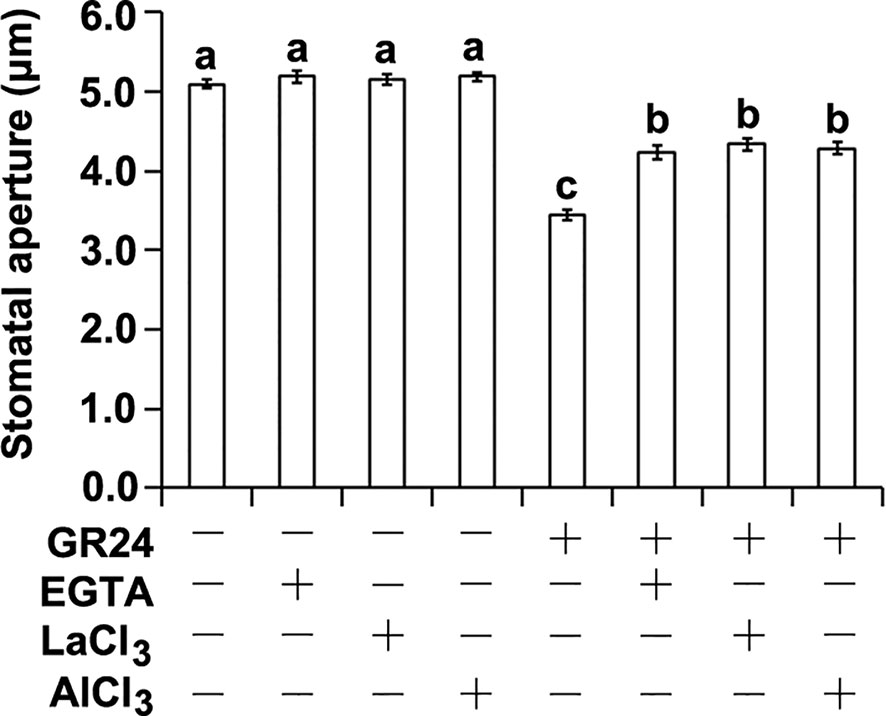
Figure 1 The effects of Ca2+ chelator and channel inhibitors on strigolactone-induced stomatal closure. Leaf epidermal peels of WT plants with open stomata were exposed to either the MES-KCl buffer with (+) or without (–) 1 μM GR24, 2 mM EGTA, 1 mM LaCl3, or 1 μM AlCl3. The stomatal apertures were measured for different treatments. Data are means ± SE of three independent experiments Bars with different letters represent statistically significant differences.
It was reported that exogenous Ca2+ could promote stomatal closure (Blatt, 2000; Ray, 2017). Since we have shown that Ca2+ plays a crucial role in SL-induced stomatal closure, we further examined the stomatal response of SL-related mutants in response to Ca2+. We found that addition of Ca2+ (CaCl2) induced a similar stomatal closure in SL-related mutants as in WT plants, whereas SL-signaling mutants max2 and d14 were insensitive to GR24 as reported previously (Figure S1; Lv et al., 2018). This result indicates that Ca2+ might act as a signaling molecule downstream of SL-signaling and SL-biosynthetic genes.
SL- and Ca2+-Induced Stomatal Closure Were Impaired in the Cpk33 Mutant
Given that Ca2+ is essential for SL-induced stomatal closure (Figure 1), we sought to identify the specific Ca2+ sensor(s) that is involved in guard-cell SL signaling. CPKs, as Ca2+ sensors, are important in the regulation of ABA-mediated and Ca2+-mediated stomatal closure (Kim et al., 2010; Boudsocq and Sheen, 2013; Schulz et al., 2013; Simeunovic et al., 2016). To determine whether any CPK is required for SL signaling in guard cells, we firstly examined SL-induced stomatal closure in the absence and presence of CPK inhibitors TFP and staurosporine (ST) (Li et al., 1998). TFP and ST significantly inhibited GR24-induced stomatal closure (Figure S2), suggesting that CPK(s) is truly indispensable for SL-induced stomatal closure.
Furthermore, to identify CPK(s) that might be involved in SL signaling in guard cells, the effect of GR24 on stomatal aperture was examined in a collection of cpk mutants, including cpk4, cpk8, cpk10, cpk11, cpk23, cpk33-1, cpk33-2, cpk3 cpk5 cpk6 cpk11, and cpk5 cpk6 cpk11 cpk23 (Figure 2). GR24 significantly induced stomatal closure in most of examined cpk mutants. Conversely, SL-induced stomatal closure is greatly impaired in cpk33-1 and cpk33-2 mutants, and marginally impaired in cpk10 mutants (Figure 2). To overcome the potential redundant function between CPK10 and CPK33, we generated double mutants cpk10 cpk33-1 and cpk10 cpk33-2. The double mutants cpk10 cpk33 exhibited insensitivity to SL-induced stomatal closure to a similar extent to that of the cpk33 mutants (Figure 2), suggesting that CPK10 exerted slight effect on SL-induced stomatal closure. Nevertheless, we found that CPK33 is predominantly involved in guard cell SL signaling, and we thus concentrated on CPK33 in subsequent studies.
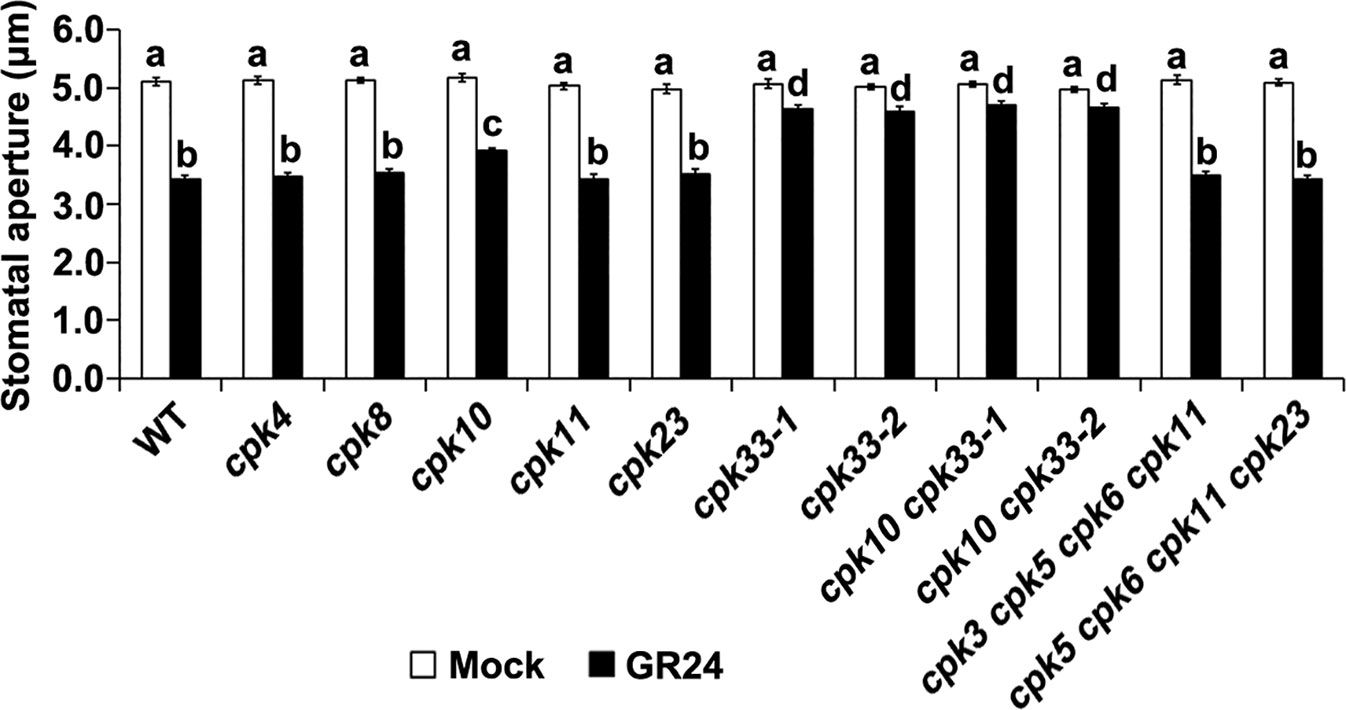
Figure 2 Strigolactone-stimulated stomatal closure is mediated by CPK33. Leaf epidermal peels of WT plants and a collection of cpk mutants were exposed to the MES-KCl buffer in the absence and presence of 1 μM GR24. Stomatal apertures were measured and presented as means ± SE of three independent experiments. Bars with different letters represent statistically significant differences.
Because SL promotion of stomatal closure depends on Ca2+, we hypothesized that disruption of CPK33 would impair Ca2+ signaling transduction. To test this hypothesis, we investigated the stomatal response following exogenous Ca2+ application in cpk33-1 and cpk33-2 mutants. Exogenous Ca2+ stimulated stomatal closure in WT plants, but not in cpk33-1 and cpk33-2 mutants (Figure 3). Taken together, our results indicate that CPK33 acts as an important Ca2+ sensor that is involved in guard-cell SL signaling.
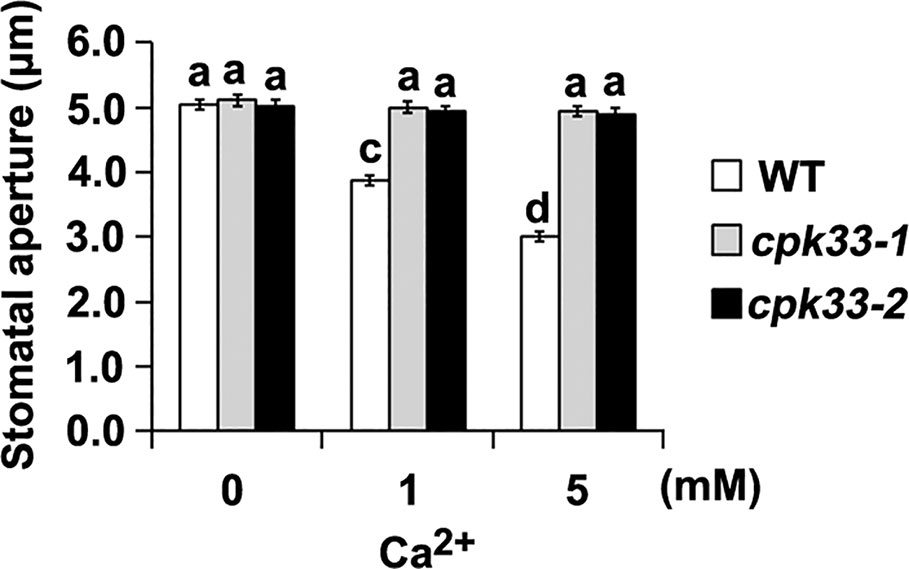
Figure 3 Ca2+-induced stomatal closure is significantly impaired in cpk33 mutants. Leaves of WT plants, cpk33-1, and cpk33-2 mutants with open stomata were exposed to the MES-KCl buffer containing different concentrations of CaCl2. Stomatal apertures were subsequently measured and presented as means ± SE of three independent experiments. Bars with different letters represent statistically significant differences.
CPK33 Kinase Activity Is Essential for SL-Induced Stomatal Closure
Previous studies have shown that the kinase activity of CPK33 is essential for SLAC1 channels and GORK channels activities and ABA-induced stomatal movement (Li C.L et al., 2016; Corratgé-Faillie et al., 2017). To investigate whether the in vivo kinase activity of CPK33 is also required for SL-induced stomatal closure, we performed stomatal bioassay analysis upon GR24 treatment using cpk33-1 and cpk33-2 mutants, two independent cpk33-1 complementation lines (35::CPK33 cpk33 #1 and #2), and two independent lines expressing a kinase-inactive CPK33K102R construct in cpk33-1 (35::CPK33K102R cpk33 #1 and #2). Two 35::CPK33 cpk33 lines exhibited GR24-sensitive phenotype similar to that of WT plants, while we found that 35::CPK33K102R cpk33 plants, as well as cpk33-1 and cpk33-2 mutants, failed to close stomata in response to GR24 (Figure 4). Thus, this result suggests that CPK33 kinase activity is essential for stomatal closure induced by SLs.
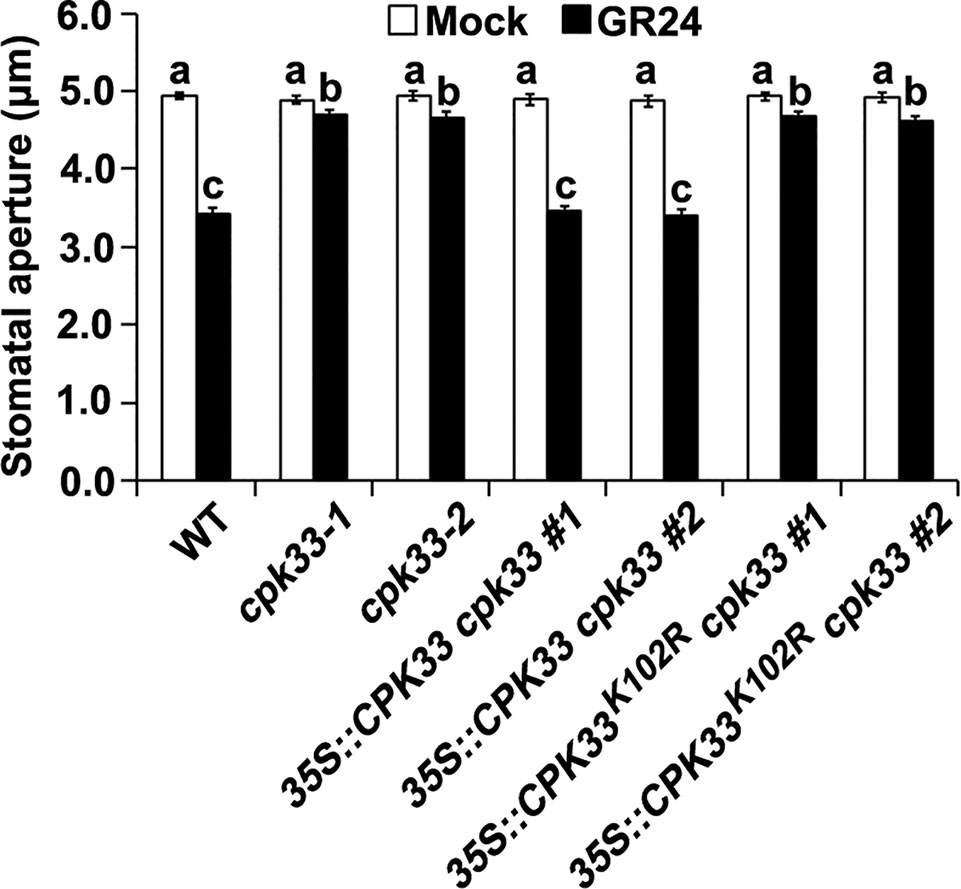
Figure 4 CPK33 kinase activity is essential for strigolactone-induced stomatal closure. Leaves of WT, cpk33-1, cpk33-2, 35::CPK33 cpk33 #1, 35::CPK33 cpk33 #2, 35::CPK33K102R cpk33 #1, and 35::CPK33K102R cpk33 #2 plants with open stomata were exposed to the MES-KCl buffer in the absence or presence of 1 µM GR24. Stomatal apertures were measured and presented as means ± SE of three independent experiments. Bars with different letters represent statistically significant differences.
Effects of the cpk33 Mutation on SL-Induced H2O2 Production and H2O2-Mediated Stomatal Closure in Guard Cells
It was reported previously that SLs stimulate H2O2 accumulation, and the resultant H2O2 acts as an early signal component in the induction of stomatal closure triggered by SLs (Lv et al., 2018). To investigate the genetic relationship between H2O2 and CPK33 in guard cell SL signaling, we examined the SL effect on H2O2 production in cpk33 mutants using the H2O2 fluorescent probe H2DCF-DA. In line with previous data, GR24 stimulated H2O2 production in WT plants. Similarly, GR24 also stimulated H2O2 production in cpk33 mutants (Figure 5A), suggesting that CPK33 disruption did not affect SL-induced H2O2 production.
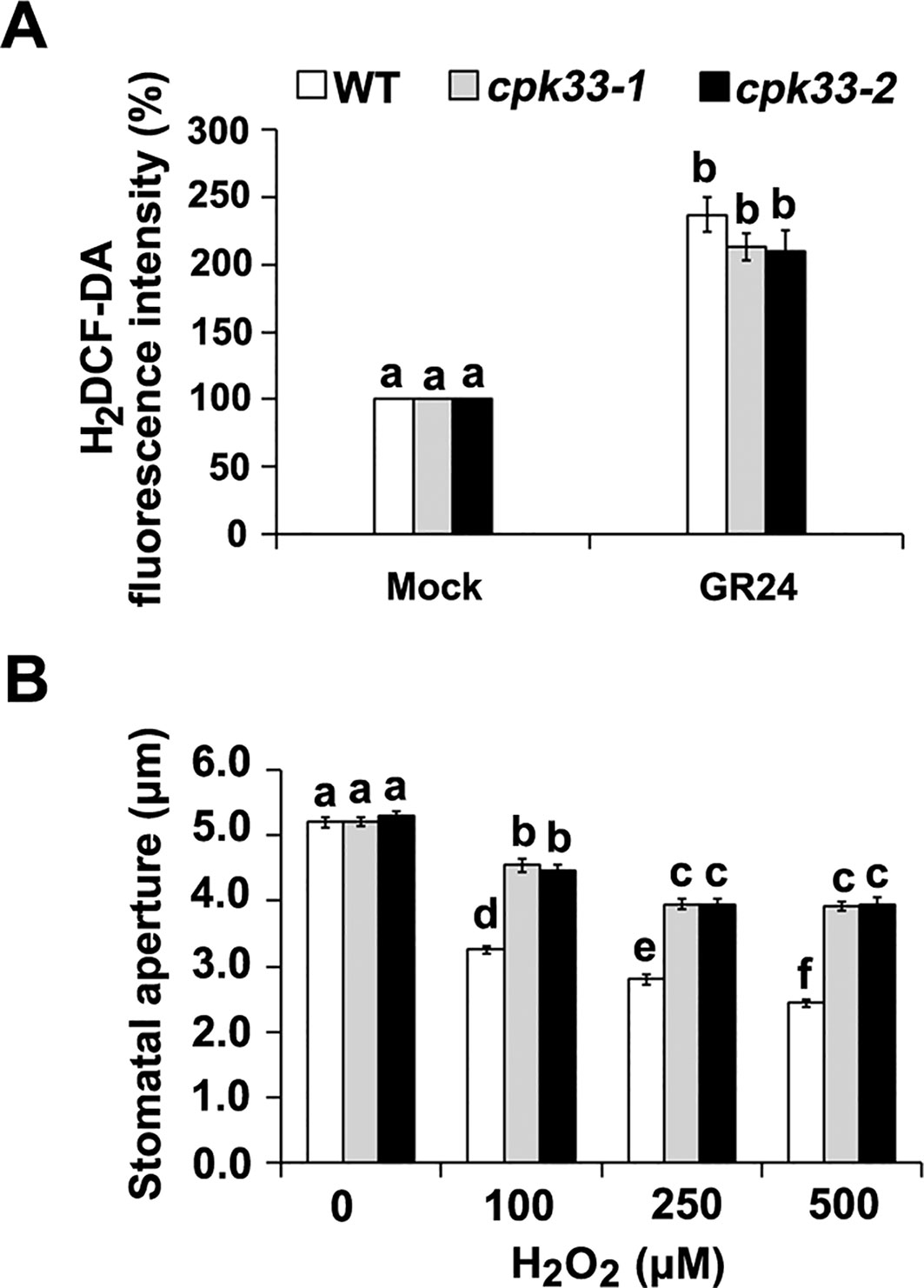
Figure 5 The role of CPK33 in strigolactone-induced H2O2 production and H2O2-mediated stomatal closure in guard cells. (A) Effects of GR24 on H2O2 production in guard cells of WT plants, cpk33-1 and cpk33-2 mutants. Fluorescence intensities were normalized to those of controls that were taken as 100% for the indicated experiments. (B) Epidermal strips of WT plants with open stomata were exposed to the MES-KCl buffer containing different concentrations of H2O2. The stomatal apertures were measured and presented as means ± SE of three independent experiments. Bars with different letters represent statistically significant differences.
To further investigate the position of H2O2 production and CPK33 in guard cell SL signaling pathway, we next examined the stomatal aperture upon exogenous H2O2 in cpk33 mutants. Exogenous application of H2O2 significantly stimulated stomatal closure in WT plants, but H2O2-induced stomatal closure is partially impaired in cpk33 mutants in comparison with WT plants (Figure 5B). Altogether, our results indicate that CPK33 possibly functions downstream of H2O2 production in guard-cell SL signaling. It appears that, as a paradigm, H2O2 activates CPK(s) that function as signal mediator(s) in guard cells. However, it remains to be explored whether and how CPK33 is activated by SL-induced H2O2
Discussion
It has been shown that SLs were implicated in various developmental processes of plants and mediate their responses to environmental stress (Al-Babili and Bouwmeester, 2015; Marzec, 2016; Cardinale et al., 2018; Mostofa et al., 2018). Recently we found that SLs function as common regulators to induce stomatal closure (Lv et al., 2018; Zhang et al., 2018). We further elucidated that SL-induced stomatal closure is accomplished through enhancing production of H2O2 and nitric oxide, eventually promoting plant resilience to environmental stress (Lv et al., 2018; Zhang et al., 2018). However, no significant effect on stomatal aperture was observed when spraying GR24 onto intact plants, although SL-related mutants were genetically confirmed to display higher stomatal conductance (Kalliola et al., 2019). The discrepancy in SL-induced stomatal closure may be due to the different materials used, intact plants and epidermal strips, which possibly results in different efficacy of GR24 such as permeability problem. It has been reported that Ca2+ and its sensors CPKs are crucial for stomatal closing (Blatt, 2000; Bowler and Fluhr, 2000; Pei et al., 2000; Kim et al., 2010; Murata et al., 2015; Ray, 2017). OST1, a Ca2+-independent kinase, was found to be dispensable for SL-induced stomatal closure (Lv et al., 2018). Based on these observations, we thus hypothesize that Ca2+ as well as its transducers CPK(s) is implicated in SL-triggered stomatal closure.
In this study we found that Ca2+ chelator EGTA, and Ca2+ channel blockers LaCl3 and AlCl3 significantly suppressed SL-induced stomatal closure (Figure 1), suggesting the requirement of extracellular free Ca2+ and Ca2+ channels in the modulation of SL-induced stomatal closure. Our pharmacological and further genetic analyses suggest that SL requires cytosolic Ca2+ signals to promote stomatal closure (Figures 1 and 3). It is possible that SLs stimulate either Ca2+ sensitivity priming or cytosolic Ca2+ elevation to perturb Ca2+ signals, thereby resulting in stomatal closure. It is thus demanded to detect the alteration of Ca2+ signals through Ca2+ detection fluorescence dyes and/or different Ca2+ biosensors in the future analyses.
It has long been known that CPKs, acting as Ca2+ sensors, are important mediators of Ca2+-dependent stomatal closure and ion channel activation (Kim et al., 2010; Boudsocq and Sheen, 2013; Schulz et al., 2013; Simeunovic et al., 2016). We identified a potential Ca2+ transducer CPK33 acting as an intermediate component downstream of H2O2 in guard cell SL signaling pathway. SL activation of stomatal closure, as well as Ca2+-induced stomatal closure, were impaired in cpk33 mutants (Figures 2 and 3). Interestingly, CPK10 disruption slightly impaired SL-induced stomatal closure (Figure 2). The double mutant cpk10 cpk33 exhibited insensitivity to SL-induced stomatal closure to a similar extent to that of the cpk33 mutant (Figure 2), suggesting a prime role of CPK33 and a differential contribution of CPK10 and CPK33 in SL-induced stomatal closure. It was found previously that CPK10 was involved in plant responses to drought stress via modulation of ABA- and Ca2+-mediated stomatal closure (Zou et al., 2010). Considering the complexity of crosstalk of distinct signaling pathways, it is plausible that CPK10 might indirectly impact on the SL activation of stomatal closure. Alternatively, given that there are 34 CPKs in Arabidopsis (Boudsocq and Sheen, 2013), a yet unidentified CPK, rather than CPK33, may function redundant with CPK10 in modulation of SL-induced stomatal closure. Nevertheless, the role of CPK10 (possible with another redundant CPK) in SL promotion of stomatal closure needs to be investigated.
Intriguingly, guard cell outward potassium channel GORK is specifically stimulated by CPK33 to active outward potassium ion currents, showing that, unlike its negative regulation of anion channels (Li C.L et al., 2016), CPK33 positively modulates the GORK channel activity to promote stomatal closure (Corratgé-Faillie et al., 2017). Consequently, cpk33 mutants were delayed in stomatal closure under normal conditions (Corratgé-Faillie et al., 2017). In this regard, it is possible that SLs could exploit CPK33-activated GORK channels to promote stomatal closure. It thus remains to be investigated whether SLs could stimulate CPK33-activated GORK channel activity. CPK33 was reported as a negative regulator in ABA-modulated stomatal closure (Li C.L et al., 2016). SL activation of stomatal closure, however, were inhibited in cpk33 mutants (Figure 2), indicating that CPK33 positively functions in SL-induced stomatal closure. Taken together, our results and previous reports suggest that the CPK-dependent Ca2+ recognition conveying by CPK33 could be essential for SL signaling, as well as ABA signaling in guard cells. Nevertheless, the role of opposite effect of CPK33 on anion and potassium channels, as well as the discrepant role of CPK33 in guard cell ABA and SL signaling, is required to be further studied.
Integrated previous studies with our present genetic and physiological analyses, we propose that CPK33, as a Ca2+ transducer, acts downstream of H2O2 and Ca2+ (Figure 6). In guard cells, following perception by D14 and MAX2, SLs stimulate the production of H2O2 that possibly activates CPK33, which likely modulates anion and potassium channels to promote stomatal closure (Li C.L et al., 2016; Corratgé-Faillie et al., 2017; Figure 6). Indeed, it has been reported that CPK33 positively stimulates the GORK channels while negatively regulates SLAC1 channels to promote stomatal closure (Li C.L et al., 2016; Corratgé-Faillie et al., 2017). However, it remains to be examined whether and how CPK33 is activated by SL-induced H2O2. It has been reported that H2O2-stimulated cytosolic Ca2+ elevation is crucial for stomatal closure (Pei et al., 2000). CPK33 was confirmed to be a typical Ca2+-dependent kinase (Corratgé-Faillie et al., 2017), indicating that CPK33 activity requires cytosolic Ca2+ increase. Therefore, it will be intriguing to investigate whether SLs and/or SL-induced H2O2 are able to promote cytosolic Ca2+ which presumably is sensed by CPK33 in SL-mediated guard cell signaling. The finding that the stomata of cpk33 mutants remain considerable response to H2O2 indicates that other factor(s) might also required for SL-triggered stomatal closure. In the meanwhile, our results emphasizes the essential role of H2O2 serving as a hub in the complicated hormone-mediated stomatal closure. Altogether, our study reinforces the understanding of the molecular mechanism by which SLs induce stomatal closure and provides new insights to improve stress acclimatization of plants.
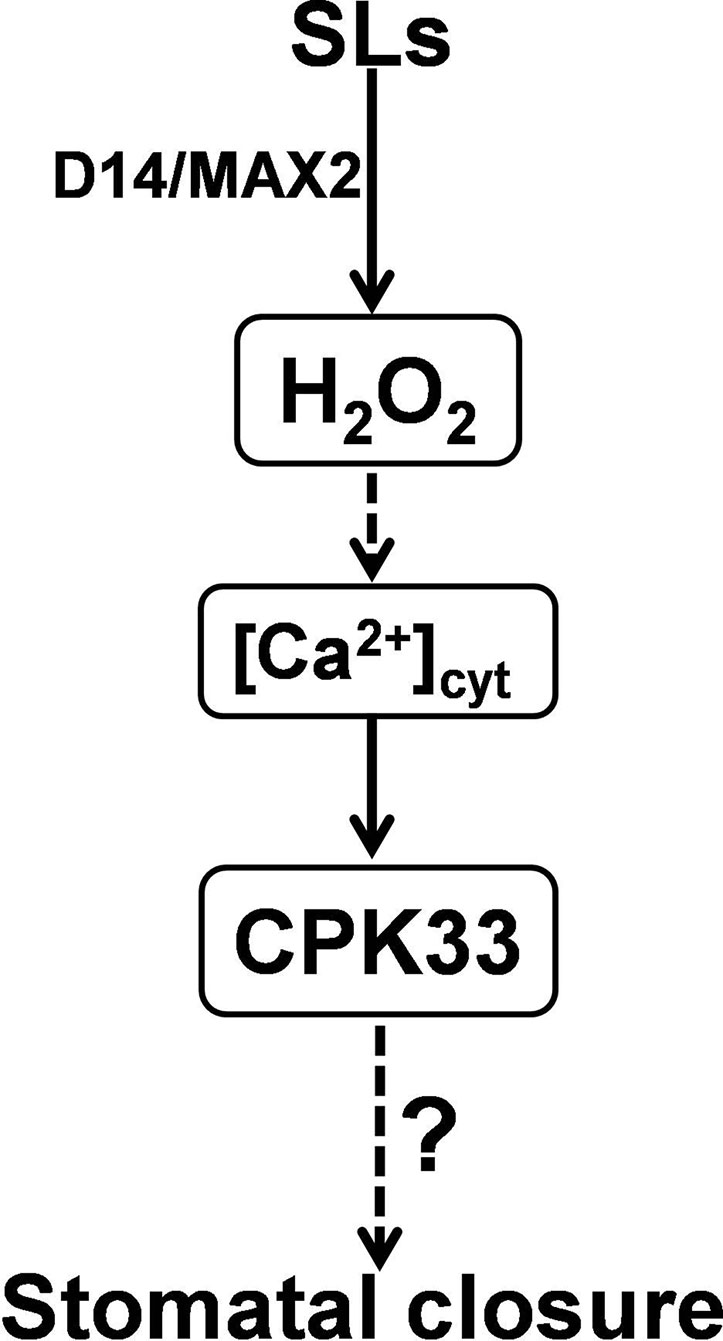
Figure 6 A working model for CPK33-mediated strigolactone (SL) signaling in guard cells. Following perception by D14 and MAX2, SLs stimulate the production of H2O2 that possibly activates the Ca2+ transducer CPK33 which likely modulates anion and potassium channels to promote stomatal closure. The question mark stands for an unknown molecule(s) that is downstream of CPK33 in SL-mediated stomatal closure. It remains to be explored whether and how SLs and/or SL-induced H2O2 could promote cytosolic Ca2+ which is presumably sensed by CPK33 in SL-mediated guard cell signaling.
Data Availability Statement
The datasets generated for this study are available on request to the corresponding author.
Author Contributions
YZ and GW designed the experiments. XW, SL, XH, XG, XS, JK, ZL, and YZ performed the experiments. BC assisted in microscope. WZ contributed materials. YZ, CL, and GW wrote the manuscript. All authors reviewed the manuscript.
Funding
The study is supported by the National Natural Science Foundation of China (31701294 and 31801210), the Fundamental Research Funds for Central Universities (GK201702016), the SNNU Laboratory Technology Research Funds (SYJS201739), the Start-up Foundation of Hubei University of Medicine (2016QDJZR14), and the Natural Science Foundation of Hubei Provincial Department of Education (Q20182104).
Conflict of Interest
The authors declare that the research was conducted in the absence of any commercial or financial relationships that could be construed as a potential conflict of interest.
Acknowledgments
We thank Profs Wei-Hua Wu, Da-Peng Zhang, Rob Roelfsema, Daoxin Xie, Cun Wang, and Shinjiro Yamaguchi for sharing genetic materials.
Supplementary Material
The Supplementary Material for this article can be found online at: https://www.frontiersin.org/articles/10.3389/fpls.2019.01630/full#supplementary-material
References
Agusti, J., Herold, S., Schwarz, M., Sanchez, P., Ljung, K., Dun, E. A., et al. (2011). Strigolactone signaling is required for auxin-dependent stimulation of secondary growth in plants. Proc. Natl. Acad. Sci. U.S.A. 108, 20242–20247. doi: 10.1073/pnas.1111902108
Al-Babili, S., Bouwmeester, H. J. (2015). Strigolactones, a novel carotenoid-derived plant hormone. Annu. Rev. Plant Biol. 66, 161–186. doi: 10.1146/annurev-arplant-043014-114759
Blatt, M. R., Brodribb, T. J., Torii, K. U. (2017). Small pores with a big impact. Plant Physiol. 174, 467–469. doi: 10.1104/pp.17.00642
Blatt, M. R. (2000). Ca2+ signalling and control of guard-cell volume in stomatal movements. Curr. Opin. Plant Biol. 3, 196–204. doi: 10.1016/s1369-5266(00)80065-5
Boudsocq, M., Sheen, J. (2013). CDPKs in immune and stress signaling. Trends Plant Sci. 18, 30–40. doi: 10.1016/j.tplants.2012.08.008
Bowler, C., Fluhr, R. (2000). The role of calcium and activated oxygens as signals for controlling cross-tolerance. Trends Plant Sci. 5, 241–246. doi: 10.1016/s1360-1385(00)01628-9
Brandt, B., Brodsky, D. E., Xue, S., Negi, J., Iba, K., Kangasjärvi, J., et al. (2012). Reconstitution of abscisic acid activation of SLAC1 anion channel by CPK6 and OST1 kinases and branched ABI1 PP2C phosphatase action. Proc. Natl. Acad. Sci. U.S.A. 109, 10593–10598. doi: 10.1073/pnas.1116590109
Brandt, B., Munemasa, S., Wang, C., Nguyen, D., Yong, T., Yang, P. G., et al. (2015). Calcium specificity signaling mechanisms in abscisic acid signal transduction in Arabidopsis guard cells. eLife 4, e03599. doi: 10.7554/eLife.03599
Bu, Q., Lv, T., Shen, H., Luong, P., Wang, J., Wang, Z., et al. (2014). Regulation of drought tolerance by the F-box protein MAX2 in Arabidopsis. Plant Physiol. 164, 424–439. doi: 10.1104/pp.113.226837
Cardinale, F., Krukowski, P. K., Schubert, A., Visentin, I. (2018). Strigolactones: mediators of osmotic stress responses with a potential for agrochemical manipulation of crop resilience. J. Exp. Bot. 69, 2291–2303. doi: 10.1093/jxb/erx494
Chen, D. H., Liu, H. P., Li, C. L. (2019). Calcium-dependent protein kinase CPK9 negatively functions in stomatal abscisic acid signaling by regulating ion channel activity in Arabidopsis. Plant Mol. Biol. 99, 113–122. doi: 10.1007/s11103-018-0805-y
Corratgé-Faillie, C., Ronzier, E., Sanchez, F., Prado, K., Kim, J. H., Lanciano, S., et al. (2017). The Arabidopsis guard cell outward potassium channel GORK is regulated by CPK33. FEBS Lett. 591, 1982–1992. doi: 10.1002/1873-3468.12687
Daszkowska-Golec, A., Szarejko, I. (2013). Open or close the gate-stomata action under the control of phytohormones in drought stress conditions. Front. Plant Sci. 4, 138–138. doi: 10.3389/fpls.2013.00138
Geiger, D., Scherzer, S., Mumm, P., Stange, A., Marten, I., Bauer, H., et al. (2009). Activity of guard cell anion channel SLAC1 is controlled by drought stress signaling kinase-phosphatase pair. Proc. Natl. Acad. Sci. U.S.A. 106, 21425–21430. doi: 10.1073/pnas.0912021106
Geiger, D., Scherzer, S., Mumm, P., Marten, I., Ache, P., Matschi, S., et al. (2010). Guard cell anion channel SLAC1 is regulated by CDPK protein kinases with distinct Ca2+ affinities. Proc. Natl. Acad. Sci. U.S.A. 107, 8023–8028. doi: 10.1073/pnas.0912030107
Geiger, D., Maierhofer, T., Al-Rasheid, K. A., Scherzer, S., Mumm, P., Liese, A., et al. (2011). Stomatal closure by fast abscisic acid signaling is mediated by the guard cell anion channel SLAH3 and the receptor RCAR1. Sci. Signal. 4, ra32. doi: 10.1126/scisignal.2001346
Guzel Deger, A., Scherzer, S., Nuhkat, M., Kedzierska, J., Kollist, H., Brosché, M., et al. (2015). Guard cell SLAC1-type anion channels mediate flagellin-induced stomatal closure. New. Phytol. 208, 162–173. doi: 10.1111/nph.13435
Ha, C. V., Leyva-González, M. A., Osakabe, Y., Tran, U. T., Nishiyama, R., Watanabe, Y., et al. (2014). Positive regulatory role of strigolactone in plant responses to drought and salt stress. Proc. Natl. Acad. Sci. U.S.A. 111, 851–856. doi: 10.1073/pnas.1322135111
Hetherington, A. M., Woodward, F. I. (2003). The role of stomata in sensing and driving environmental change. Nature 424, 901–908. doi: 10.1038/nature01843
Jia, K. P., Luo, Q., He, S. B., Lu, X. D., Yang, H. Q. (2014). Strigolactone-regulated hypocotyl elongation is dependent on cryptochrome and phytochrome signaling pathways in Arabidopsis. Mol. Plant 7, 528–540. doi: 10.1093/mp/sst093
Kalliola, M., Jakobson, L., Davidsson, P., Pennanen, V., Waszczak, C., Yarmolinsky, D., et al. (2019). The role of strigolactones in regulation of stomatal conductance and plant-pathogen interactions in Arabidopsis thaliana. BioRxiv. doi: 10.1101/573873
Kapulnik, Y., Delaux, P. M., Resnick, N., Mayzlish-Gati, E., Wininger, S., Bhattacharya, C., et al. (2011). Strigolactones affect lateral root formation and root-hair elongation in Arabidopsis. Planta 233, 209–216. doi: 10.1007/s00425-010-1310-y
Kim, T. H., Böhmer, M., Hu, H., Nishimura, N., Schroeder, J. I. (2010). Guard cell signal transduction network: advances in understanding abscisic acid, CO2 and Ca2+ signaling. Annu. Rev. Plant Biol. 61, 561–591. doi: 10.1146/annurev-arplant-042809-112226
Kohlen, W., Charnikhova, T., Lammers, M., Pollina, T., Tóth, P., Haider, I., et al. (2012). The tomato CAROTENOID CLEAVAGE DIOXYGENASE8 (SlCCD8) regulates rhizosphere signaling, plant architecture and affects reproductive development through strigolactone biosynthesis. New Phytol. 196, 535–547. doi: 10.1111/j.1469-8137.2012.04265.x
Lee, S. C., Lan, W. Z., Buchanan, B. B., Luan, S. (2009). A protein kinase-phosphatase pair interacts with an ion channel to regulate ABA signaling in plant guard cells. Proc. Natl. Acad. Sci. U.S.A. 106, 21419–21424. doi: 10.1073/pnas.0910601106
Li, J., Lee, Y. R., Assmann, S. M. (1998). Guard cells possess a Calcium-dependent protein kinase that phosphorylates the KAT1 potassium channel. Plant Physiol. 116, 785–795. doi: 10.1104/pp.116.2.785
Li, C. L., Wang, M., Wu, X. M., Chen, D. H., Lv, H. J., Shen, J. L., et al. (2016). THI1, a thiamine thiazole synthase, interacts with Ca2+-dependent protein kinase CPK33 and modulates the S-type anion channels and stomatal closure in Arabidopsis. Plant Physiol. 170, 1090–1104. doi: 10.1104/pp.15.01649
Li, Y., Xu, S., Gao, J., Pan, S., Wang, G. (2016). Glucose- and mannose-induced stomatal closure is mediated by ROS production, Ca2+ and water channel in Vicia faba. Physiol. Plant 156, 252–261. doi: 10.1111/ppl.12353
Li, W., Nguyen, K. H., Chu, H. D., Ha, C. V., Watanabe, Y., Osakabe, Y., et al. (2017). The karrikin receptor KAI2 promotes drought resistance in Arabidopsis thaliana. PloS Genet. 13, e1007076. doi: 10.1371/journal.pgen.1007076
Liu, J., Novero, M., Charnikhova, T., Ferrandino, A., Schubert, A., Ruyter-Spira, C., et al. (2013). CAROTENOID CLEAVAGE DIOXYGENASE 7 modulates plant growth, reproduction, senescence, and determinate nodulation in the model legume Lotus japonicus. J. Exp. Bot. 64, 1967–1981. doi: 10.1093/jxb/ert056
Liu, J., He, H., Vitali, M., Visentin, I., Charnikhova, T., Haider, I., et al. (2015). Osmotic stress represses strigolactone biosynthesis in Lotus japonicus roots: exploring the interaction between strigolactones and ABA under abiotic stress. Planta 241, 1435–1451. doi: 10.1007/s00425-015-2266-8
Lv, S., Zhang, Y., Li, C., Liu, Z., Yang, N., Pan, L., et al. (2018). Strigolactone-triggered stomatal closure requires hydrogen peroxide synthesis and nitric oxide production in an abscisic acid-independent manner. New Phytol. 217, 290–304. doi: 10.1111/nph.14813
Ma, S. Y., Wu, W. H. (2007). AtCPK23 functions in Arabidopsis responses to drought and salt stresses. Plant Mol. Biol. 65, 511–518. doi: 10.1007/s11103-007-9187-2
Marzec, M. (2016). Strigolactones as part of the plant defence system. Trends Plant Sci. 21, 900–903. doi: 10.1016/j.tplants.2016.08.010
Melotto, M., Zhang, L., Oblessuc, P. R., He, S. Y. (2017). Stomatal defense a decade later. Plant Physiol. 174, 561–571. doi: 10.1104/pp.16.01853
Mori, I. C., Murata, Y., Yang, Y., Munemasa, S., Wang, Y. F., Andreoli, S., et al. (2006). CDPKs CPK6 and CPK3 function in ABA regulation of guard cell S-type anion- and Ca2+-permeable channel and stomatal closure. PloS Biol. 4, e327. doi: 10.1371/journal.pbio.0040327
Mostofa, M. G., Li, W., Nguyen, K. H., Fujita, M., Tran, L. P. (2018). Strigolactones in plant adaptation to abiotic stresses: an emerging avenue of plant research. Plant Cell Environ. 41, 2227–2243. doi: 10.1111/pce.13364
Munemasa, S., Hossain, M. A., Nakamura, Y., Mori, I. C., Murata, Y. (2011). The Arabidopsis calcium-dependent protein kinase, CPK6, functions as a positive regulator of methyl jasmonate signaling in guard cells. Plant Physiol. 155, 553–561. doi: 10.1104/pp.110.162750
Munemasa, S., Hauser, F., Park, J., Waadt, R., Brandt, B., Schroeder, J. I. (2015). Mechanisms of abscisic acid-mediated control of stomatal aperture. Curr. Opin. Plant Bio. 28, 154–162. doi: 10.1016/j.pbi.2015.10.010
Murata, Y., Mori, I. C., Munemasa, S. (2015). Diverse stomatal signaling and the signal integration mechanism. Annu. Rev. Plant Biol. 66, 369–392. doi: 10.1146/annurev-arplant-043014-114707
Pei, Z. M., Murata, Y., Benning, G., Thomine, S., Klüsener, B., Allen, G. J., et al. (2000). Calcium channels activated by hydrogen peroxide mediate abscisic acid signalling in guard cells. Nature 406, 731–734. doi: 10.1038/35021067
Prodhan, M. Y., Munemasa, S., Nahar, M. N., Nakamura, Y., Murata, Y. (2018). Guard cell salicylic acid signaling is integrated into abscisic acid signaling via the Ca2+/CPK-dependent pathway. Plant Physiol. 178, 441–450. doi: 10.1104/pp.18.00321
Qu, X., Cao, B., Kang, J., Wang, X., Han, X., Jiang, W., et al. (2019). Fine-tuning stomatal movement through small signaling peptides. Front. Plant Sci. 10, 69. doi: 10.3389/fpls.2019.00069
Rasmussen, A., Mason, M. G., Cuyper, C. D., Brewer, P. B., Herold, S., Agusti, J., et al. (2012). Strigolactones suppress adventitious rooting in Arabidopsis and pea. Plant Physiol. 158, 1976–1987. doi: 10.1104/pp.111.187104
Ray, S. D. (2017). “Ca2+, The miracle molecule in plant hormone signaling during abiotic stress,” in Mechanism of Plant Hormone Signaling under Stress. Eds. Pandey, G. K. (New Jersey, NJ: Wiley Blackwell), 45–90.
Roux, B., Leonhardt, N. (2018). Chapter Seven - The regulation of Ion channels and transporters in the guard cell. Adv. Bot. Res. 87, 171–214. doi: 10.1016/bs.abr.2018.09.013
Ruszala, E. M., Beerling, D. J., Franks, P. J., Chater, C., Casson, S. A., Gray, J. E., et al. (2011). Land plants acquired active stomatal control early in their evolutionary history. Curr. Biol. 21, 1025–1029. doi: 10.1016/j.cub.2011.04.044
Ruyter-Spira, C., Kohlen, W., Charnikhova, T., van Zeijl, A., van Bezouwen, L., de Ruijter, N., et al. (2011). Physiological effects of the synthetic strigolactone analog GR24 on root system architecture in Arabidopsis: another belowground role for strigolactones? Plant Physiol. 155, 721–734. doi: 10.1104/pp.110.166645
Schulz, P., Herde, M., Romeis, T. (2013). Calcium-dependent protein kinases: hubs in plant stress signaling and development. Plant Physiol. 163, 523–530. doi: 10.1104/pp.113.222539
Schwartz, A. (1985). Role of Ca2+ and EGTA on Stomatal Movements in Commelina communis L. Plant Physiol. 79, 1003–1005. doi: 10.1104/pp.79.41003
Shen, H., Luong, P., Huq, E. (2007). The F-box protein MAX2 functions as a positive regulator of photomorphogenesis in Arabidopsis. Plant Physiol. 145, 1471–1483. doi: 10.1104/pp.107.107227
Shen, H., Zhu, L., Bu, Q. Y., Huq, E. (2012). MAX2 affects multiple hormones to promote photomorphogenesis. Mol. Plant 5, 750–762. doi: 10.1093/mp/sss029
Simeunovic, A., Mair, A., Wurzinger, B., Teige, M. (2016). Know where your clients are: subcellular localization and targets of calcium-dependent protein kinases. J. Exp. Bot. 67, 3855–3872. doi: 10.1093/jxb/erw157
Snowden, K. C., Simkin, A. J., Janssen, B. J., Templeton, K. R., Loucas, H. M., Simons, J. L., et al. (2005). The Decreased apical dominance1/Petunia hybrida CAROTENOID CLEAVAGE DIOXYGENASE8 gene affects branch production and plays a role in leaf senescence, root growth, and flower development. Plant Cell 17, 746–759. doi: 10.1105/tpc.104.027714
Stirnberg, P., van De Sande, K., Leyser, H. M. (2002). MAX1 and MAX2 control shoot lateral branching in Arabidopsis. Development 129, 1131–1141.
Suhita, D., Kolla, V. A., Vavasseur, A., Raghavendra, A. S. (2003). Different signaling pathways involved during the suppression of stomatal opening by methyl jasmonate or abscisic acid. Plant Sci. 164, 481–488. doi: 10.1016/s0168-9452(02)00432-6
Suhita, D., Raghavendra, A. S., Kwak, J. M., Vavasseur, A. (2004). Cytoplasmic alkalization precedes reactive oxygen species production during methyl jasmonate- and abscisic acid-induced stomatal closure. Plant Physiol. 134, 1536–1545. doi: 10.1104/pp.103.032250
Sun, H., Tao, J., Hou, M., Huang, S., Chen, S., Liang, Z., et al. (2015). A strigolactone signal is required for adventitious root formation in rice. Ann. Bot. 115, 1155–1162. doi: 10.1093/aob/mcv052
Tsuchiya, Y., Vidaurre, D., Toh, S., Hanada, A., Nambara, E., Kamiya, Y., et al. (2010). A small-molecule screen identifies new functions for the plant hormone strigolactone. Nat. Chem. Biol. 6, 741–749. doi: 10.1038/nchembio.435
Umehara, M., Hanada, A., Yoshida, S., Akiyama, K., Arite, T., Takeda-Kamiya, N., et al. (2008). Inhibition of shoot branching by new terpenoid plant hormones. Nature 455, 195–200. doi: 10.1038/nature07272
Wang, C., Zhang, J., Wu, J., Brodsky, D. E., Schroeder, J. I. (2018). Cytosolic malate and oxaloacetate activate S-type anion channels in Arabidopsis guard cells. New Phytol. 220, 178–186. doi: 10.1111/nph.15292
Yao, R., Ming, Z., Yan, L., Li, S., Wang, F., Ma, S., et al. (2016). DWARF14 is a non-canonical hormone receptor for strigolactone. Nature 536, 469–473. doi: 10.1038/nature19073
Zhang, Y., Lv, S., Wang, G. (2018). Strigolactones are common regulators in induction of stomatal closure in planta. Plant Signal. Behav. 13, 1–12. doi: 10.1080/15592324.2018.1444322
Zhang, L., Shi, X., Zhang, Y., Wang, J., Yang, J., Ishida, T., et al. (2019). CLE9 peptide-induced stomatal closure is mediated by abscisic acid, hydrogen peroxide, and nitric oxide in Arabidopsis thaliana. Plant Cell Environ. 42, 1033–1044. doi: 10.1111/pce.13475
Zhao, M. G., Tian, Q. Y., Zhang, W. H. (2007). Ethylene activates a plasma membrane Ca2+-permeable channel in tobacco suspension cells. New Phytol. 174, 507–515. doi: 10.2307/4640974
Zhu, S. Y., Yu, X. C., Wang, X. J., Zhao, R., Li, Y., Fan, R. C., et al. (2007). Two calcium-dependent protein kinases, CPK4 and CPK11, regulate abscisic acid signal transduction in Arabidopsis. Plant Cell 19, 3019–3036. doi: 10.1105/tpc.107.050666
Zou, J. J., Wei, F. J., Wang, C., Wu, J. J., Ratnasekera, D., Liu, W. X., et al. (2010). Arabidopsis calcium-dependent protein kinase CPK10 functions in abscisic acid- and Ca2+-mediated stomatal regulation in response to drought stress. Plant Physiol. 154, 1232–1243. doi: 10.1104/pp.110.157545
Zou, J. J., Li, X. D., Ratnasekera, D., Wang, C., Liu, W. X., Song, L. F., et al. (2015). Arabidopsis CALCIUM-DEPENDENT PROTEIN KINASE8 and CATALASE3 function in abscisic acid-mediated signaling and H2O2 homeostasis in stomatal guard cells under drought stress. Plant Cell 27, 1445–1460. doi: 10.1105/tpc.15.00144
Keywords: Ca2+, calcium-dependent protein kinase, guard cell, stomatal closure, strigolactones
Citation: Wang X, Lv S, Han X, Guan X, Shi X, Kang J, Zhang L, Cao B, Li C, Zhang W, Wang G and Zhang Y (2019) The Calcium-Dependent Protein Kinase CPK33 Mediates Strigolactone-Induced Stomatal Closure in Arabidopsis thaliana. Front. Plant Sci. 10:1630. doi: 10.3389/fpls.2019.01630
Received: 27 August 2019; Accepted: 19 November 2019;
Published: 17 December 2019.
Edited by:
Kazimierz Trebacz, Maria Curie-Skłodowska University, PolandReviewed by:
Fabien Jammes, Pomona College, United StatesDanilo M. Daloso, Universidade Federal do Ceará, Brazil
Copyright © 2019 Wang, Lv, Han, Guan, Shi, Kang, Zhang, Cao, Li, Zhang, Wang and Zhang. This is an open-access article distributed under the terms of the Creative Commons Attribution License (CC BY). The use, distribution or reproduction in other forums is permitted, provided the original author(s) and the copyright owner(s) are credited and that the original publication in this journal is cited, in accordance with accepted academic practice. No use, distribution or reproduction is permitted which does not comply with these terms.
*Correspondence: Guodong Wang, guodong_wang@snnu.edu.cn; Yonghong Zhang, zhangyonghong52@gmail.com