- Department of Cytogenetics and Genome Analysis, Leibniz Institute of Plant Genetics and Crop Plant Research, Gatersleben, Germany
“Break-induced replication” (BIR) is considered as one way to repair DNA double-strand breaks (DSBs). BIR is defined as replication of the proximal break-ends up to the end of the broken chromosome using an undamaged (homologous) double-stranded template and mimicking a non-reciprocal translocation. This phenomenon was detected by genetic experiments in yeast. BIR is assumed to occur also in mammals, but experimental evidence is not yet at hand. We have studied chromosomes of the field bean, Vicia faba L., with respect to the occurrence of BIR after DSB induction during S and G2 phase. Simultaneous incorporation of the base analog ethynyldeoxyuridine (EdU) revealed no chromosomal replication pattern indicative of BIR. Thus, if occurring at all, BIR does not play a major role in DSB repair in higher plants with large chromosome arms. However, the frequency of interstitial asymmetric EdU incorporation within heterochromatic regions, visible on metaphase chromosomes, increased after chromosome breakage during S and G2 phase. Such asymmetric labeling could be interpreted as conservative replication up to the next replicon, circumventing a DSB, and yielding an interstitial conversion-like event.
Introduction
Double-strand breaks (DSBs) are the most critical DNA lesions. Unrepaired DSBs are usually lethal for dividing cells due to the subsequent loss of the acentric fragment and the unstable break-end of the centric fragment. DSBs can be caused enzymatically, by ionizing irradiation, or by other S phase-independent clastogens. DSBs with only one break-end may appear when a replication fork meets a single-strand break or a repair-mediated parental single-strand discontinuity.
There are several ways to repair DSBs. In case of a restriction endonuclease-mediated break a simple ligation can restore the pre-break situation. Mostly however, the break is extended by a 5′-specific exonuclease activity generating 3′-overhanging ends. As soon as overhanging ends meet at short stretches of complementary bases (single-strand annealing, SSA), the distal free ends are resected, and the break becomes ligated, resulting in a (micro-)deletion (Figure 1A). Alternatively, the break-ends may invade undamaged homologous template strands and a limited DNA synthesis across the break region is followed by re-ligation of both strands (synthesis-dependent strand annealing, SDSA). The result is genetically not detectable when the template was the undamaged sister chromatid; it appears as a gene conversion if an allele of the homologous chromosome served as a template (Figure 1C). If for break-end elongation an ectopic or an extra-chromosomal (partially) homologous sequence is used, the result may be linked with an insertion into the break (Figure 1B and Puchta, 2005). When the Holiday junction (caused by break-end invasion into a template double strand) is resolved in connection with an exchange of the flanking region (Figure 1C), then, depending on the template double helix involved, a sister chromatid exchange, a crossing over, or a reciprocal translocation results.
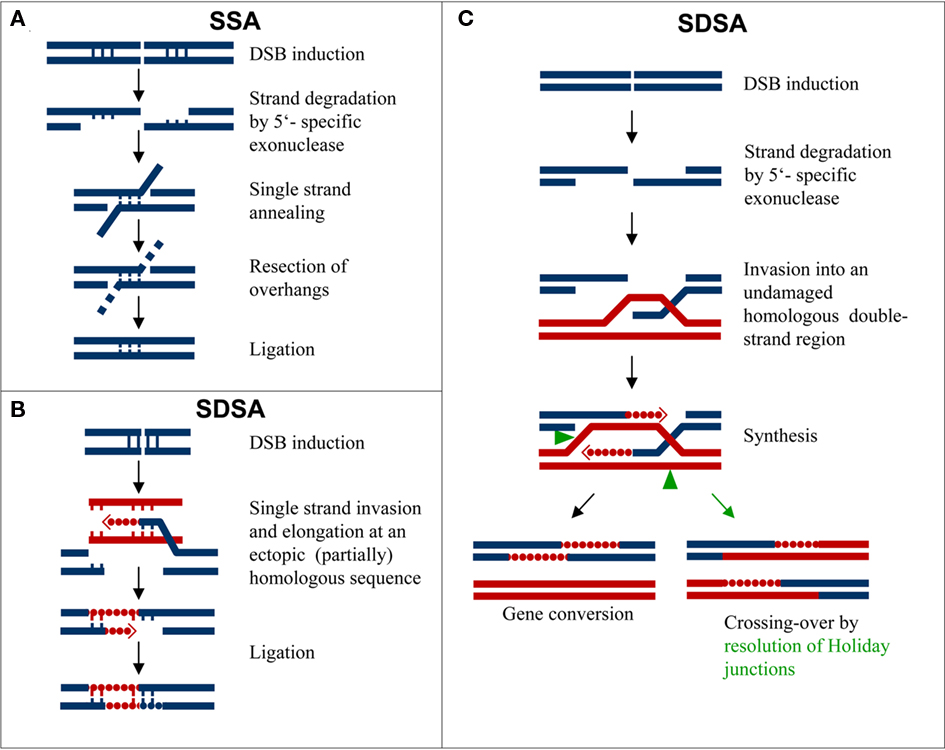
Figure 1. Pathways of DSB misrepair via single-strand annealing(SSA) or via synthesis-dependent strand annealing (SDSA). (A) Deletion via exonucleolytic 5′-end resection, SSA at complementary overhang sequences, resection of the non-aligned ends, and ligation of break-ends. (B) Insertion into a DSB by break-end invasion and elongationalong an ectopic and partially homologous (vertical bars) template.(C) Re-synthesis of break-ends after invasion into a homologous template double-strand without (gene conversion) or with exchange of flanking regions due to appropriate resolution of Holiday junctions (greenarrow heads).
Based on genetic experiments on budding yeast, a further mechanism, “break-induced replication” (BIR; Malkova et al., 1996, 2005; Morrow et al., 1997; Haber, 1999; for review Haber, 2006; McEachern and Haber, 2006; Llorente et al., 2008) has been postulated. With the exception of a direct ligation of “clean” break-ends, all DSB repair events are linked with a limited replication step, BIR is claimed to extend replication from the proximal break-end up to the end of the template chromatid, using a (homologous) undamaged double helix as template. Mechanistically, BIR appears as a non-reciprocal translocation event (Bosco and Haber, 1998) which can be considered as a type of gene conversion extending from the breakpoint up to the telomere. Simultaneously, the original distal fragment (if occurring) of the broken double helix is lost (Figure 2).
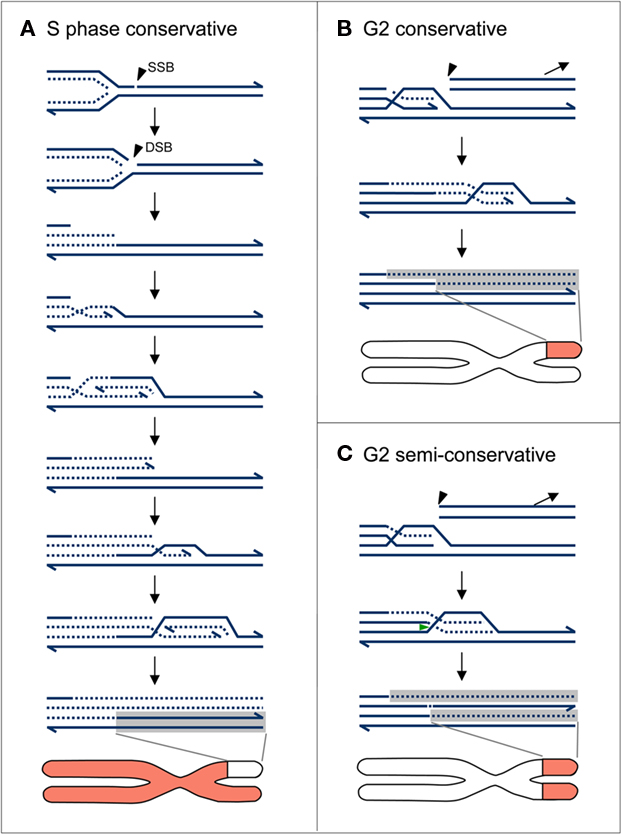
Figure 2. Schematic models of replication and chromosome labeling patterns after BIR at proximal DSB ends in S and G2. (A) BIR through conservative replication of a one ended DSB during S phase. The DSB appears when the replication fork arrives at a single-strand break (arrow head). Conservative replication occurs via recurrent strand invasion (or via unidirectional fork migration) without resolution of the Holiday junction(s) using the parental double strand as a template. The result after EdU incorporation is an asymmetrically unlabeled terminal chromatid region. (B) BIR during G2 phase, through conservative replication at the proximal end of a DSB (arrow head) via recurrent strand invasion and/or via unidirectional fork migration without resolution of the Holiday junction(s) using the undamaged sister double helix as a template. The result after EdU incorporation is an asymmetrically labeled terminal chromatid region. (C) BIR during G2 phase through semiconservative replication achieved by resolution of the Holiday junction (green arrow head) after invasion of the elongating break-end into the template double strand. The result after EdU incorporation is a symmetrically labeled distal chromatid region. Full lines unlabeled; broken lines labeled by EdU. The distal fragment of the broken double helix in (B,C) gets lost.
When a homologous or a heterologous chromatid instead of the sister chromatid is involved in BIR, a non-reciprocal translocation between homologous or heterologous chromosomes is mimicked. BIR with the homologous chromosome as a template results in a loss of heterozygosity. Microhomology-mediated BIR involving heterologous chromosomes leads to duplication of the template region and deletion of the region distal the original DSB. The problem of BIR before or after regular replication in species with large chromosomes is that breakage occurring distantly to the arm end requires replication of the involved region (larger than a chromatin unit of jointly regulated replication comprising ∼1 Mb, Zink et al., 1998) more than once between two nuclear divisions, thus overriding the licensing mechanism which allows only one round of replication between two divisions. BIR occurring during S phase (Figure 2A and Hastings et al., 2009) requires a recurrent 3′-end invasion into a still unreplicated template chromatid (most likely the sister chromatid), or a continuous migration of the replication fork toward the arm end. Resolution of the Holiday junction results in a reciprocal exchange of the distal regions between the chromatids involved. BIR during S phase without resolution of the Holiday junction circumvents the DSB, yielding a conservative replication pattern with regard to the broken chromatid distal to the breakpoint.
To test whether BIR may occur within chromosomes of higher plants after DSB induction, we treated field bean root tip meristems with the S phase-independent clastogen bleomycin during S or G2 phase and checked for the expected chromatid labeling by incorporation of the base analog ethynyldeoxyuridine (EdU, see Kotogany et al., 2010) either during S phase or in G2.
Numerous chromatid aberrations were observed in first post-treatment metaphases. In contrast, no asymmetric terminal chromatid labeling was found. Also semiconservative BIR at terminal chromatid regions (Figure 2C) was not observed after breakage in the presence of the base analog during G2. Our results suggest that BIR either does not occur or is so infrequent that its role within the concert of DSB repair mechanisms can at best be a minor one in organisms with a large DNA content (>1 Mb) per chromosome arm.
Materials and Methods
The field bean, Vicia faba L., karyotype ACB with six large and individually distinguishable chromosome pairs (Döbel et al., 1978) is much more comfortable for aberration scoring than for instance A. thaliana with smaller chromosomes and less mitoses per root meristem.
Seeds were germinated for 3 days on wet filter paper at room temperature in the dark. About 1–2 cm long primary roots were incubated for 18 h in aerated Hoagland solution, pH 5.5, containing 1.25 mM hydroxyurea which synchronizes cells by blocking the cell cycle in early S phase. For mutagen treatment during S phase, the roots were incubated for 1.5 h in Hoagland solution containing 10 μg/ml bleomycin with or without 20 μM EdU (Invitrogen) followed by incubation in Hoagland solution for 4.5 h and then 0.05% colchicine for 2.5 h (to arrest metaphases) before fixation in ethanol:acetic acid (3:1) overnight. For scoring of chromatid aberrations, roots were washed for 10 min in distilled water, hydrolyzed for 11 min in 1 N HCl at 60°C, stained for 30–40 min in Feulgen solution and squashed in a drop of 45% acetic acid. Only complete metaphase cells were scored for chromatid aberrations: chromatid and isochromatid breaks (one or both sister chromatids with terminal deletion), interstitial deletions, duplication deletion (the deleted part of one chromatid is inserted into a break of the sister chromatid), and reciprocal chromatid translocations.
Alternatively, the root tips were fixed in 4% formaldehyde for 20 min for isolation of metaphase chromosomes (Schubert et al., 1993) and subsequent detection of EdU incorporation using the Click.iT EdU Imaging Kit from Invitrogen according to manufacturer’s instruction. Microscopic analysis of chromosomes was performed with an epifluorescence microscope (Zeiss axiophot) using a 100×/1.45 Zeiss alpha-plan-fluar objective and a Sony (DXC-950P) camera. Images of fluorescent chromosomes were captured using filter sets F36-513 for DAPI and F36-750 for Alexa Fluor 594 (AHF Analysentechnik, Germany). DAPI and Alexa Fluor 594 azide images were merged using Adobe Photoshop 6.0 software (Adobe Systems, San Jose, CA, USA).
For mutagen treatment during G2 phase, primary roots were incubated in Hoagland solution with hydroxyurea as above, then in Hoagland solution for 4.5 h and subsequently in Hoagland solution with bleomycin, with EdU, or both for another 1.5 h followed by 2.5 h in colchicine before fixation, staining, and evaluation.
Results
Bleomycin Induces Chromatid Aberrations in S and G2 Phase; Simultaneous Treatment with the Base Analog EdU Does not Further Increase the Aberration Yield
The S phase-independent clastogen bleomycin induces DNA breaks directly. A dose of 10 μg/ml was previously shown to yield chromatid aberrations in up to 30% of the first post-treatment metaphases of field bean root tip meristems (Heindorff et al., 1987). The same bleomycin concentration applied for 1.5 h to (mainly) G2 cells of root tip meristems, in two independent experiments yielded on an average of 14.8% of metaphases with chromatid aberrations. After treatment during S phase 9.2% of aberrant metaphases were found. Treatment with EdU (20 μM, 1.5 h) resulted in 0.8% metaphases with aberrations when applied during G2 and in 3.1% when applied during S phase. Untreated control roots displayed 1% of metaphases with aberrations. Bleomycin and EdU together yielded 12% of metaphases with aberration after exposure in G2 and 6.6% after exposure to S phase cells (Table 1). Although during S phase the base analog EdU caused slightly more aberrations than appear in untreated control cells, no obvious synergistic effect on clastogenicity was observed when applied together with bleomycin.
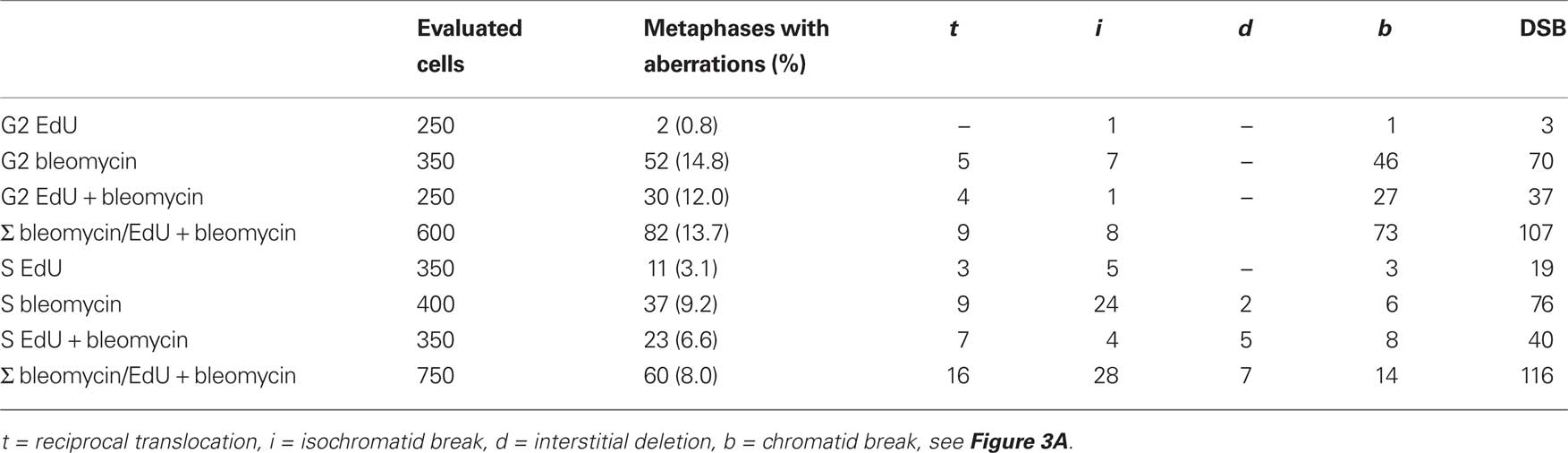
Table 1. Chromatid-type aberrations induced by EdU, bleomycin, or both during G2 or S phase in field bean root tip meristems (summary of two independent experiments).
Chromosomal EdU Incorporation Patterns Do Not Indicate Break-Induced Replication Up to an Arm End
When root tips were incubated in 20 μM EdU 8.5–7 h before fixation, most metaphase chromosomes (87.4%) were completely labeled and some (12.5%) were labeled along their euchromatin regions (Table 2), indicating that the corresponding cells were in S phase during treatment as expected according to the experimental schedule based of the cell cycle duration of field bean meristems (Schubert and Meister, 1977). No chromosome showed asymmetric labeling up to the chromosome end, i.e., an unlabeled terminal region of one of the sister chromatids. Only one out of 1404 chromosomes displayed an unlabeled transversal region within a heterochromatic area of one sister chromatid.
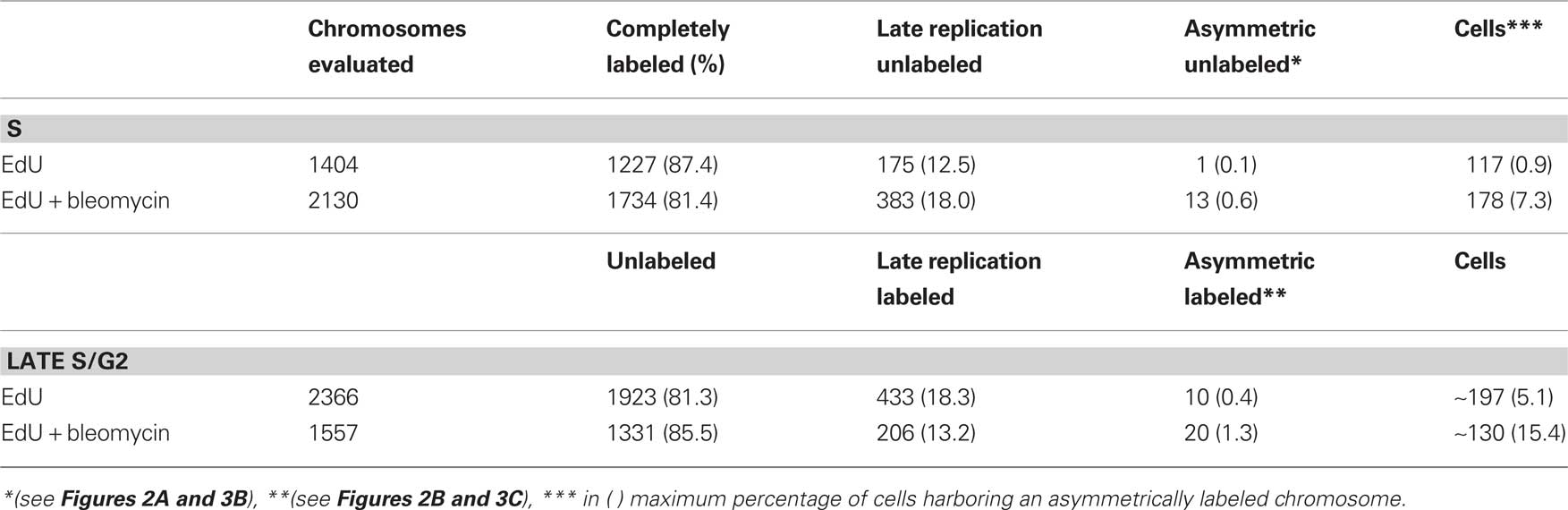
Table 2. Type and frequency of chromosomal labeling patterns after EdU incorporation during S or G2 phase with or without bleomycin treatment (two independent experiments summarized).
When S phase cells were treated simultaneously with EdU and bleomycin, all 2130 observed chromosomes were labeled (81.4% completely and 18% along their euchromatin). None of these chromosomes showed asymmetric labeling up to an arm end as to be expected if the replication fork is stalled at a single-strand break and the 3′-break-end recurrently invaded the unreplicated double helix, or if it replicated till the end of the template by unidirectional fork migration (Figure 2A and Hastings et al., 2009). Thirteen chromosomes showed unlabeled transversal spots within the heterochromatic part of one sister chromatid Figure 3B). Since 2130 chromosomes correspond to 178 cells, in about 7.3% of cells such an asymmetrically unlabeled spot occurred, representing an eightfold increase compared to the variant with EdU treatment alone.
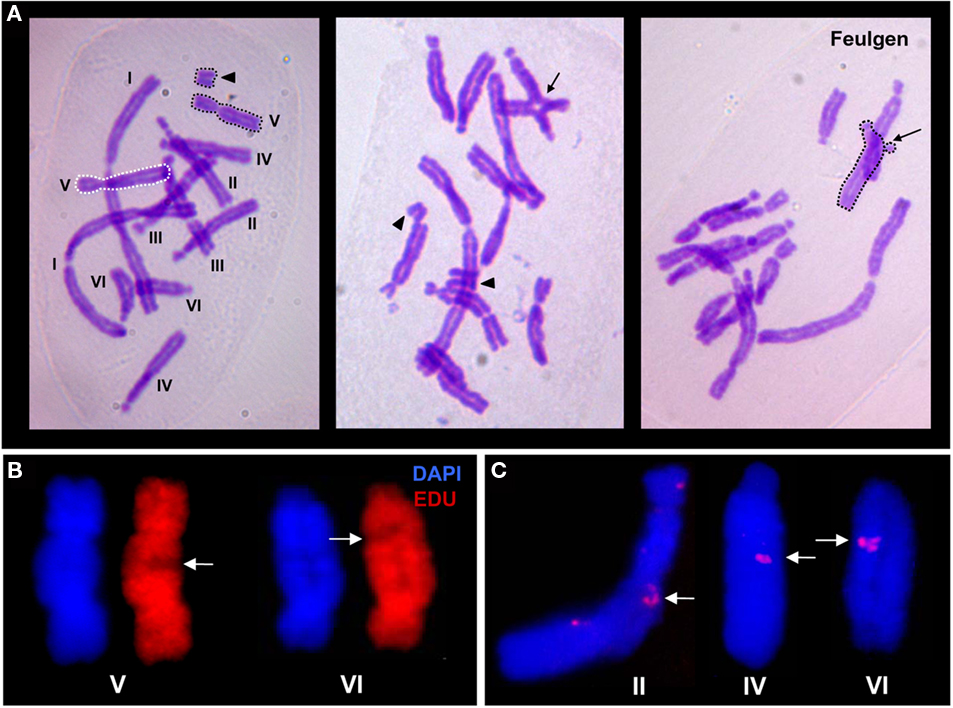
Figure 3. Metaphase chromosomes of the field bean. (A) Chromatid-type aberrations after bleomycin treatment. Left cell: isochromatid break (arrow head), the centric, and the acentric chromatid fragments are surrounded by black dots, the homologous undamaged chromosome is surrounded by white dots. Middle cell: symmetric reciprocal chromatid translocation (arrow) and two terminal chromatid breaks (arrow heads). The latter with the broken fragment either switched to the opposite site of the undamaged sister chromatid (left) or being at least 90° apart from the other break-end as in case of the broken secondary constriction (right). Right cell: interstitial deletion (arrow), the deleted fragment remains attached to the undamaged sister chromatid, the chromosome involved is surrounded by black dots. (B) Interstitial asymmetric chromatid labeling (arrows) after bleomycin treatment in the presence of EdU during S phase. (C) Interstitial asymmetric chromatid labeling (arrows) after bleomycin treatment in the presence of EdU during G2. The asymmetric signals appear on chromosomes II, IV, V, and VI, respectively, at interstitial heterochromatic regions composed of homologous tandem repeats (Fuchs et al., 1994).
When metaphase chromosomes were tested for EdU incorporation after incubation in EdU 4–2.5 h before fixation, the corresponding cells should have been in G2 during EdU treatment. Indeed, most of the chromosomes (81.3%) were unlabeled while 18.3% revealed late replication pattern (symmetric labeling of heterochromatic regions) thus indicating they were in late S phase during EdU treatment. No chromosome showed asymmetric labeling of one chromatid up to the arm end, as expected in the case of a conservative BIR (Figure 2B). Also symmetric terminal labeling of sister chromatids, as expected in the case of semiconservative BIR (Figure 2C) was not observed. Single transversal interstitial fluorescence signals on one of the sister chromatids (Figure 3C) were observed in 10 (0.4%) chromosomes.
After simultaneous exposure to EdU and to bleomycin 4–2.5 h before fixation, again most of the chromosomes (85.5%) were completely unlabeled and thus were in G2 during treatment; 13.2% of chromosomes showed late replication patterns, indicating that the corresponding cells were in late S during treatment. Again no chromosome showed asymmetric or symmetric terminal labeling as predicted for BIR. However, there was a >3-fold increase in chromosomes showing an interstitial transversal and asymmetric fluorescence signal (20 signals per 130 cells), usually in heterochromatic regions.
Discussion
If BIR occurs in higher plants in the same way as postulated for yeast (i.e., as replication extending from the break position up to the corresponding chromosome arm end) conservative replication in S or G2 (Figures 2A,B) or semiconservative replication in G2 (Figure 2C) should be detectable. Of the chromatid aberrations observed after bleomycin and bleomycin plus EdU treatment during S and G2, reciprocal translocations (25), isochromatid breaks (36), and interstitial deletions (7) require two DSBs each, while simple chromatid breaks (87) representing terminal deletions, go back to a single DSB. Therefore, within 600 G2 and 750 S phase cells, bleomycin caused 223 DSBs which underwent misrepair. Thus, on average at least one DSB per six cells was induced. Considering that a fraction of DSBs might have been repaired via pathways not resulting in microscopically detectable chromatid-type aberrations (for instance by sister chromatid exchange, intrachromosomal SSA, or gene conversion), the actual number of DSBs may be higher. However, in a number of chromosomes corresponding to 308 S and G2 cells, treated simultaneously with the chromosome-breaking agent bleomycin and the base analog EdU, not a single chromosome showed a labeling pattern indicative of BIR. In budding yeast, more than half of diploid cells repair HO-endonuclease-induced DSBs by BIR using the homologous chromosome as template (Bosco and Haber, 1998); meaning more than half of the DSBs are repaired via BIR if the endonuclease HO causes one DSB per diploid yeast cell. Had BIR occurred with a similar efficiency in the field bean, we should have observed >223 instead of no chromosomes with asymmetric terminal EdU signal. Our result does not exclude the occurrence of BIR in plants, however, the frequency of such events is below 0.45% (less than 1 out of 223) of the DSBs causing chromosome structural aberrations, i.e., more than 100-times lower than in yeast. Unidirectional replication forks that override distal replication origins have to migrate up to the arm ends, or, alternatively, the requirement of recurrent invasion of 3′-ends into other double helices, are presumptions for which experimental evidence is lacking so far. Long range replication in addition to the regular round of replication between two nuclear divisions, represent a further unproven assumptions. To our knowledge no experimental evidence as to the occurrence of BIR in animals bas been shown (Zhang et al., 2009). Taken together these arguments imply that the existence of a BIR pathway in higher eukaryotes is unlikely. Even when such events occur, their frequency is much lower than in yeast arguing against their importance as a pathway for DSB repair at least in higher plants such as the field bean. Because the 12 megabase pair (Mb) genome size of Saccharomyces cerevisiae is distributed over 32 chromosome arms, the risk that BIR interferes with replication licensing at the replicon level is much smaller than for species with orders of magnitude larger genomes with several Mb per arm. Also complex chromosome rearrangements (e.g., Difilippantonio et al., 2002; Zhang et al., 2009) can more easily be explained by other well-documented pathways (Zhang et al., 2009; Schubert and Lysak, 2011) rather than by BIR via recurrent invasion of a DSB-induced 3′-end into different template double helices. The more so as experimental approaches even in yeast cannot differentiate “between BIR and gene conversion events involving G2 cross over, followed by appropriate chromatid segregation” (Ricchetti et al., 2003).
Nevertheless, the increased frequency of interstitial asymmetric labeling patterns of chromosomes exposed to bleomycin and EdU during S or G2 might indicate that conserved replication, although not reaching arm ends, could be linked with DSB repair to result in microscopically detectable stretches of gene conversion extending hundreds of kilobases (possibly, up to the junction with the next replication unit, but not covering DNA stretches of >1 Mb up to the arm ends). Such extended gene conversion-like repair might be considered as BIR, if the postulation “up to the chromosome terminus” is excluded. Similar events could potentially have been responsible for the “zebra” structure of the long arm of chromosome z5A observed in a backcross progeny between Elymus trachycaulus Gould ex Shinners and Triticum aestivum L. (Zhang et al., 2008). The preferential appearance of local asymmetric labeling within heterochromatic regions of the field bean suggests that for late replicating repetitive DNA the units of replication regulation are larger or more variable than for euchromatin.
Conflict of Interest Statement
The authors declare that the research was conducted in the absence of any commercial or financial relationships that could be construed as a potential conflict of interest.
Acknowledgment
We thank Andrea Kunze and Joachim Bruder for excellent technical assistance, Stefan Heckmann for initial help with the click assay and Holger Puchta and Tim Sharbel for critical reading the manuscript.
References
Bosco, G., and Haber, J. E. (1998). Chromosome break-induced DNA replication leads to nonreciprocal translocations and telomere capture. Genetics 150, 1037–1047.
Difilippantonio, M. J., Petersen, S., Chen, H. T., Johnson, R., Jasin, M., Kanaar, R., Ried, T., and Nussenzweig, A. (2002). Evidence for replicative repair of DNA double-strand breaks leading to oncogenic translocation and gene amplification. J. Exp. Med. 196, 469–480.
Döbel, P., Schubert, I., and Rieger, R. (1978). Distribution of heterochromatin in a reconstructed karyotype of Vicia faba as identified by banding- and DNA-late replication patterns. Chromosoma 69, 193–209.
Fuchs, J., Pich, U., Meister, A., and Schubert, I. (1994). Differentiation of field bean heterochromatin by in situ hybridization with a repeated FokI sequence. Chromosome Res. 2, 25–28.
Haber, J. E. (1999). DNA recombination: the replication connection. Trends Biochem. Sci. 24, 271–275.
Haber, J. E. (2006). Transpositions and translocations induced by site-specific double-strand breaks in budding yeast. DNA Repair 5, 998–1009.
Hastings, P. J., Ira, G., and Lupski, J. R. (2009). A microhomology-mediated break-induced replication model for the origin of human copy number variation. PLoS Genet. 5, e1000327. doi: 10.1371/journal.pgen.1000327
Heindorff, K., Schubert, I., Rieger, R., and Michaelis, A. (1987). Clastogenic adaptation of Vicia faba root-tip meristem cells after consecutive treatments with S-phase dependent and S-phase independent agents. Biol. Zent. Bl. 106, 439–448.
Kotogany, E., Dudits, D., Horvath, G. V., and Ayaydin, F. (2010). A rapid and robust assay for detection of S-phase cell cycle progression in plant cells and tissues by using ethynyl deoxyuridine. Plant Methods 6, 5.
Llorente, B., Smith, C. E., and Symington, L. S. (2008). Break-induced replication: what is it and what is it for? Cell Cycle 7, 859–864.
Malkova, A., Ivanov, E. L., and Haber, J. E. (1996). Double-strand break repair in the absence of RAD51 in yeast: a possible role for break-induced DNA replication. Proc. Natl. Acad. Sci. U.S.A. 93, 7131–7136.
Malkova, A., Naylor, M. L., Yamaguchi, M., Ira, G., and Haber, J. E. (2005). RAD51-dependent break-induced replication differs in kinetics and checkpoint responses from RAD51-mediated gene conversion. Mol. Cell Biol. 25, 933–944.
McEachern, M. J., and Haber, J. E. (2006). Break-induced replication and recombinational telomere elongation in yeast. Annu. Rev. Biochem. 75, 111–135.
Morrow, D. M., Connelly, C., and Hieter, P. (1997). “Break copy” duplication: a model for chromosome fragment formation in Saccharomyces cerevisiae. Genetics 147, 371–382.
Puchta, H. (2005). The repair of double-strand breaks in plants: mechanisms and consequences for genome evolution. J. Exp. Bot. 56, 1–14.
Ricchetti, M., Dujon, B., and Fairhead, C. (2003). Distance from the chromosome end determines the efficiency of double strand break repair in subtelomeres of haploid yeast. J. Mol. Biol. 328, 847–862.
Schubert, I., Dolezel, J., Houben, A., Scherthan, H., and Wanner, G. (1993). Refined examination of plant metaphase chromosome structure at different levels made feasible by new isolation methods. Chromosoma 102, 96–101.
Schubert, I., and Lysak, M. A. (2011). Interpretation of karyotype evolution should consider chromosome structural constraints. Trends in Genet. (in press).
Schubert, I., and Meister, A. (1977). Cell-cycle investigations in root-tip meristems of reconstructed karyotypes from Vicia faba. Biol. Zent. Bl. 96, 183–201.
Zhang, F., Carvalho, C. M., and Lupski, J. R. (2009). Complex human chromosomal and genomic rearrangements. Trends Genet. 25, 298–307.
Zhang, P., Li, W., Friebe, B., and Gill, B. S. (2008). The origin of a “zebra” chromosome in wheat suggests nonhomologous recombination as a novel mechanism for new chromosome evolution and step changes in chromosome number. Genetics 179, 1169–1177.
Keywords: break-induced replication, Vicia faba, chromatid aberrations, cell cycle, base analog, non-conservative replication, chromosome labeling, double-strand break
Citation: Schubert I, Schubert V and Fuchs J (2011) No evidence for “break-induced replication” in a higher plant – but break-induced conversion may occur. Front. Plant Sci. 2:8. doi: 10.3389/fpls.2011.00008
Received: 31 January 2011; Paper pending published: 17 March 2011;
Accepted: 23 March 2011; Published online: 18 April 2011.
Edited by:
Richard Jorgensen, Laboratorio Nacional de Genomica para la Biodiversidad, Mexico.Reviewed by:
Paul Fransz, University of Amsterdam, NetherlandsCharles I White, National Center for Scientific Research, France
Alan Christensen, University of Nebraska, USA
Copyright: © 2011 Schubert, Schubert and Fuchs. This is an open-access article subject to a non-exclusive license between the authors and Frontiers Media SA, which permits use, distribution and reproduction in other forums, provided the original authors and source are credited and other Frontiers conditions are complied with.
*Correspondence: Ingo Schubert, Leibniz Institute of Plant Genetics and Crop Plant Research, Corrensstrasse 3, D-06466 Gatersleben, Germany. e-mail:c2NodWJlcnRAaXBrLWdhdGVyc2xlYmVuLmRl