- 1Ministry of Education Key Laboratory for Biodiversity Science and Ecological Engineering, Center for Evolutionary Biology, School of Life Sciences, Fudan University, Shanghai, China
- 2Shanghai Key Laboratory of Plant Functional Genomics and Resources, Shanghai Chenshan Botanical Garden, Shanghai, China
Alternanthera philoxeroides is a perennial amphibious weed native to South America but has now spread to diverse parts of the world. A. philoxeroides reproduces both sexually and asexually in its native range, but propagates solely through vegetative means in its introduced range. Traits associated with sexual reproduction become degraded for sexual dysfunction, with flowers possessing either pistillate stamens or male-sterile anthers. Degradations of sexual characters for loss of sexuality commonly take place in clonal plants. The underlying molecular-genetic processes remain largely unknown. We compared the gene expression profiles of abnormal stamens with that of normal stamens by RNA-Seq analysis, and identified a large number of differentially expressed genes between abnormal and normal stamens. In accordance with flower morphology, the expression of B-class MADS-box genes (ApAP3, ApTM6, and ApPI) was markedly reduced in pistillate stamens. However, most of the genes involved in meiosis were expressed normally in stamens with male-sterile anthers. In addition to verifying the expression patterns of genes previously known to be related to stamen and pollen grain development, we also identified previously unknown molecular phenotypes associated with sexual dysfunction in A. philoxeroides, that is helpful for dissecting the molecular mechanisms underpinning various male-sterile phenotypes and the molecular processes underlying the transition from sexuality to asexuality in clonal plants.
Introduction
Alternanthera philoxeroides, commonly known as alligator weed, is a perennial amphibious weed native to South America, but has now spread to diverse parts of the world, showing up in North and South America, France, Italy, Australia, New Zealand, China, and other parts of Asia. A. philoxeroides can grow in a variety of habitats, including open lands, waterway banks, ponds, and lakes. Individuals growing in aquatic and terrestrial habitats showed extensive variations in leaf size and shape, stem diameter and internode length, but exhibited little genetic differentiation within and among populations (Ye et al., 2003; Geng et al., 2007). It has thus been proposed that phenotypic plasticity, rather than locally adapted ecotypes, allows A. philoxeroides to colonize a wide range of habitats (Geng et al., 2006, 2007; Li and Ye, 2006).
Alternanthera philoxeroides reproduces both sexually and asexually in its native range, but propagates mainly through vegetative means via storage root and stem fragmentation in its introduced range and does not produce viable seeds (Julien and Stanley, 1999; Sosa et al., 2007). Extensive field survey of the introduced A. philoxeroides in China revealed various patterns of anomalous floral development (Chen, 1964). The most striking aberration is the homeotic transformation of stamens into pistils or pistil-like structures (Figure 1). The complete pistillate flowers do not have stamens but five pistil-like structures and one normal pistil. Unlike the normal pistil, the ‘pistils’ transformed from stamens often develop ovary-like structures but contain no ovule inside (Chen, 1964; Hu et al., 2011). Monoclinous flowers possessing both stamens and pistils are common in natural populations. However, the anthers of these flowers are often shriveled bearing no or few non-viable pollen grains (Hu et al., 2011; Wang et al., 2011). There also exist some incomplete pistillate flowers with intermediate phenotypes between monoclinous and complete pistillate plants.
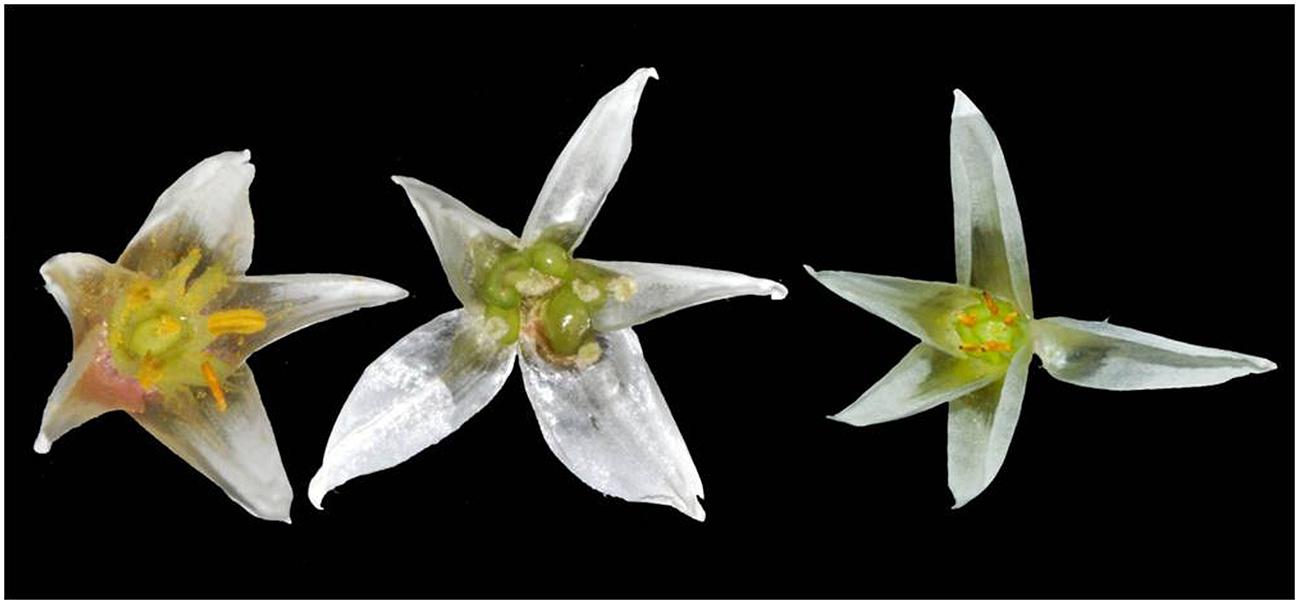
FIGURE 1. Images of representative Alternanthera philoxeroides flowers: normal flowers (left), pistillate flowers (middle), and male-sterile flowers (right).
The ABC(DE) model is now widely used as a framework for understanding the molecular mechanisms controlling floral organ identity (Coen and Meyerowitz, 1991; Theißen, 2001). According to the model, the differentiation of floral organs is controlled by the differential expression of several subsets of homeotic genes belonging to the MADS-box gene family, except for the A-class gene APETALA2 (Riechmann and Meyerowitz, 1997; Vandenbussche et al., 2003). The co-expression of B- and C-class MADS-box genes establishes the identity of the stamens (Coen and Meyerowitz, 1991; Jack et al., 1992). B-function mutants produce homeotic transformation of stamens into carpels (Jack et al., 1992; Goto and Meyerowitz, 1994). It is unclear whether pistillody in A. philoxeroides was caused by altering the expression pattern of the B-class MADS-box genes. Additionally, the cytotype of A. philoxeroides found in China is a hexaploid (Cai et al., 2009). Sosa et al. (2007) suggested a hybrid origin of the invasive hexaploid of A. philoxeroides, and that meiotic abnormalities due to the formation of univalents/multivalents led to microspore degeneration which resulted in anthers bearing no pollen grains. Hu et al. (2011) found, however, that the microspore tetrads were formed and separated normally in the anthers of the hexaploid A. philoxeroides, but the protoplasm of most pollen grains disintegrated at the post-maturation stage and pollen grains became empty, with only a few non-viable pollen grains left in the anthers.
Male sterility in plants has received considerable attention because of its potential value in breeding and hybrid seed production. It is also of great importance in evolutionary studies on the origin of dioecy (Sawhney and Shukla, 1994; Singh et al., 2012). The phenotypic manifestations of male sterility are diverse in plants, including the complete absence of male organs, the failure to develop normal sporogenous tissues (no meiosis), the abortion of pollen at any step of its development, and the inability of mature pollen to germinate on compatible stigma (Budar and Pelletier, 2001; Singh et al., 2012). The conversion of stamens to different type of floral organs also represents a male-sterile condition (Sawhney and Shukla, 1994). Although multiple genes and proteins related to microspore and pollen abortion have been characterized (Yang et al., 2003a; Jung et al., 2006; de Azevedo Souza et al., 2009), the genetic and molecular mechanisms underpinning various male-sterile phenotypes are still poorly understood. Furthermore, loss of sexuality is common in invasive clonal plants (Eckert and Barrett, 1993; Eckert, 2002; Barrett et al., 2008). Degeneration of sex can be caused by environmental and/or genetic factors. It is possible that the sexual infertility in sterile polyploids is due to polyploidy per se (Eckert, 2002). Traits associated with sexual reproduction may become degraded for sexual dysfunction, especially in plants that are sexually infertile and reproduction is solely clonal. However, there seems to have been a general lack of interest in dissecting the molecular-genetic processes associated with sexual infertility and degradation of sexual characters in clonal plants, even though they have arisen repeatedly in many groups of plants (Eckert, 2002). Investigation of the genetic architecture and molecular mechanisms underlying the transition from sexuality to asexuality in clonal plants will not only extend our understanding of the genetic control of reproductive organ development, but may also provide insights into the mechanisms and evolutionary pathways of sexual sterility in clonal plants.
Molecular phenotypes are important links between genomic information and organismic functions, fitness, and evolution (Held et al., 2014). In this study, we compared the gene expression profiles of abnormal stamens with that of normal stamens by RNA-Seq analysis. A large number of differentially expressed genes between abnormal and normal stamens were captured. The pistillate stamens exhibited a molecular phenotype distinct from that of the stamens with male-sterile anthers. In addition to verifying the expression patterns of genes previously known to be related to stamen and pollen grain development, we identified molecular phenotypes previously unknown to be associated with sexual dysfunction in A. philoxeroides that will be helpful in future analyses.
Materials and Methods
Plant Materials
Plants producing normal fertile flowers were collected from Argentina and maintained in the botanical garden of Yunnan University (E102°42′, N25°03′, Kunming, China). Pistillate flowers and male-sterile flowers were collected from plants growing in natural habitats close to the botanical garden. Flower heads containing flowers at different developmental stages were collected and preserved in RNAlater solution (Life Technologies, Gaithersburg, MD, USA). Five individuals were sampled from each type of flowers.
RNA Extraction, cDNA Library Construction and Illumina Sequencing
RNAs of normal flowers, male-sterile flowers, and pistillate flowers were extracted using the RNeasy Plant Mini Kit (Qiagen, Valencia, CA, USA) and purified with the on-column DNase I digestion (Qiagen) following the manufacturer’s instructions. RNA quality was visually checked on a 1% agarose gel and by a Nanodrop 2000c Spectrophotometer (NanoDrop Technologies, Wilmington, DE, USA). RNA integrity was further verified by an Agilent 2100 Bioanalyzer (Agilent Technologies, Santa Clara, CA, USA).
cDNA libraries were constructed following the High-Throughput Illumina Strand-Specific RNA Sequencing Library protocol (Zhong et al., 2011). Briefly, poly A containing mRNA was purified from total RNA and then fragmented into small pieces. Double-stranded cDNA was synthesized from the fragmented cDNA, and Illumina sequencing adapters were ligated to the ends of the fragments. Libraries were sequenced using the HiSeq 2000 System (Illumina, San Diego, CA, USA).
De novo Transcriptome Assembly and Gene Annotation
Raw reads generated by the sequencing machine were filtered to obtain high-quality reads. Reads containing adaptor sequences were discarded. Read with a PHRED quality score below 20 were also removed. De novo assembly was carried out using the Trinity software with default settings and a minimum contig length of 200 bp (Grabherr et al., 2011). Assembled contigs were used as input for a second assembly made with CAP3 (Huang and Madan, 1999). Redundancy was reduced using CD-HIT with a sequence similarity threshold of 95% (Li and Godzik, 2006). De novo assembled sequences were annotated using BLASTX against the Arabidopsis thaliana protein database1 (TAIR10_peptide), with an e-value cut-off of 10-10. BLAST searches against the Phytozome database2 were then done for unannotated sequences.
Clean reads from each sample were mapped back to the de novo assembled reference transcriptome. Gene expression levels were calculated from the number of uniquely aligned clean reads and then normalized into units of Reads Per Kilobase per Million reads mapped (RPKM; Mortazavi et al., 2008). Differentially expressed transcripts were detected using an False Discovery Rate (FDR) value cut-off ≤0.001 and the absolute value of log2 ratio ≥1. GO enrichment analysis for biological processes was carried out utilizing Fisher’s exact test with default parameters (p < 0.01) by the R package topGO (Alexa and Rahnenfuhrer, 2010). The REViGO web server3 was used to reduce the redundancy and visualize the overrepresented GO terms based on semantic similarity (Supek et al., 2011).
Identification and Cloning of B-class MADS-Box Genes and Meiotic Genes in A. philoxeroides
Putative A. philoxeroides AP3, TM6, and PI sequences were used as queries to conduct BLAST searches against the NCBI databases4 to find homologous sequences. Multiple alignments of the retrieved sequences were constructed using ClustalW 2.0 (Larkin et al., 2007). A neighbor-joining tree was reconstructed by MEGA 6.0 (Tamura et al., 2013) using the Jones–Taylor–Thornton (JTT) model. Support for each node was tested using bootstrap method with 1000 replicates. Gene-specific primers were designed for amplifying conserved motif of each gene. PCR products were cloned into pMD 19-T vector (TaKaRa, Dalian, China) and confirmed by Sanger sequencing. Based on conserved motif sequences, gene-specific primers (Supplementary Table S1) were designed for conducting RACE-PCR to amplify target 5′and 3′cDNA ends, using SMARTer RACE cDNA amplification kit (Clontech, Mountain View, CA, USA). Amplification products of 5′and 3′ RACE were then cloned and sequenced to get full-length cDNAs. Four meiotic genes identified from A. philoxeroides, ApASY1, ApMLH3, ApMPK4, and ApMMD1, were also cloned and sequenced. They are responsible for homologous chromosome synapsis (Armstrong et al., 2002), crossover formation (Jackson et al., 2006), male-specific meiotic cytokinesis (Zeng et al., 2011) and general meiotic cell cycle progression (Yang et al., 2003b), respectively.
Quantitative Real-Time PCR (qRT-PCR) Analysis
The expression patterns of B-class MADS-box genes and genes involved in meiosis were analyzed by quantitative real-time PCR (qRT-PCR). For B-class gene analysis, total RNAs were isolated from leaves, sepals, stamens, and carpels of normal and pistillate flowers, respectively. For meiotic gene analysis, total RNAs were isolated from the stamens at early developmental stages of normal and sterile flowers. The first-strand cDNA was made from 2 μg of total RNA using PrimeScriptTM RT Master Mix Perfect Real Time (TaKaRa, Dalian, China) following the manufacturer’s recommendations. The gene-specific primers used for qRT-PCR (Supplementary Table S1) were designed using PRIMERS35. Real-time PCR was performed on a Roche LightCycler®2.0 machine (Roche diagnostics, Mannheim, Germany) using SYBR® Premix Ex TaqTM II (TliRNaseH Plus; TaKaRa, Dalian, China). The cycling parameters are as follows: initial denaturation (95°C for 30 s), 40 amplification cycles (95°C for 5 s and 60°C for 20 s), and followed by a melt cycle (60°C for 15 s). All reactions were run with three biological replicates and each with three technical replicates. UBC10 was used as the reference gene to normalize the gene expression level. Quantification of the relative changes in gene expression was performed using the 2-ΔΔCT method (Livak and Schmittgen, 2001). Data represented three biological replicates with three technical replicates and were shown as average, with error bars representing standard deviations. Duncan’s test was used to determine the statistical significance of differences.
Results
De novo Transcriptome Assembly and Annotation
cDNA libraries were constructed and sequenced for the normal flower, male-sterile flower, and pistillate flower, respectively. A total of 107,160,189 raw reads accounting for 21.6 Gb of raw data were generated for the three libraries. After filtering, 104,487,087 clean reads (20.1 Gb) were retained and used for de novo assembly (Table 1). Each library was assembled independently, and then merged to generate the final assembly. After redundancy removal, a final set of 208,082 transcripts (≥200 bp) were obtained, with a mean length of 870 bp and N50 of 1,514 bp (Table 2). Of the transcripts retained, 169,183 (81.3%) transcripts were expressed in all three samples and 4.3% only in normal flowers.
A total of 83,878 (40.3%) transcripts were matched to 15,273 A. thaliana genes, covering 56.4% of A. thaliana genome. 3,980 (1.9%) transcripts were further identified by BLAST searches against the Phytozome database. Among the annotated transcripts, 56 were associated with A-, B-, C-, and E-class MADS-box genes (Supplementary Table S2), and 168 associated with 31 know meiotic genes responsible for homologous chromosome synapsis (Armstrong et al., 2002), male-specific meiotic cytokinesis (Zeng et al., 2011), general meiotic cell cycle progression (Yang et al., 2003b), and meiotic recombination (Osman et al., 2011), respectively (Supplementary Table S3).
Detection of Differentially Expressed Genes
To identify molecular phenotypes associated with different patterns of anomalous stamen development in A. philoxeroides, expression patterns of annotated transcripts were compared between different types of flowers. Comparison between normal and pistillate flowers revealed 11,015 up-regulated and 8,591 down-regulated transcripts in the pistillate flower, using a FDR of 0.1% (Figure 2). 12,761 and 12,364 transcripts were up- and down-regulated, respectively, in the male-sterile flower compared to the normal flower. Transcripts associated with B-class MADS-box genes exhibited lower expression in the pistillate flower, while transcripts associated with A-, C-, and E-class genes showing no significant differences between normal and pistillate flowers (Supplementary Table S2). Transcripts associated with meiotic genes did not show significant decreases in male-sterile flowers compared with normal flowers, with the exception of transcripts associated with AtMSH5 that showed decreased expression in the male-sterile flower (Supplementary Table S3).
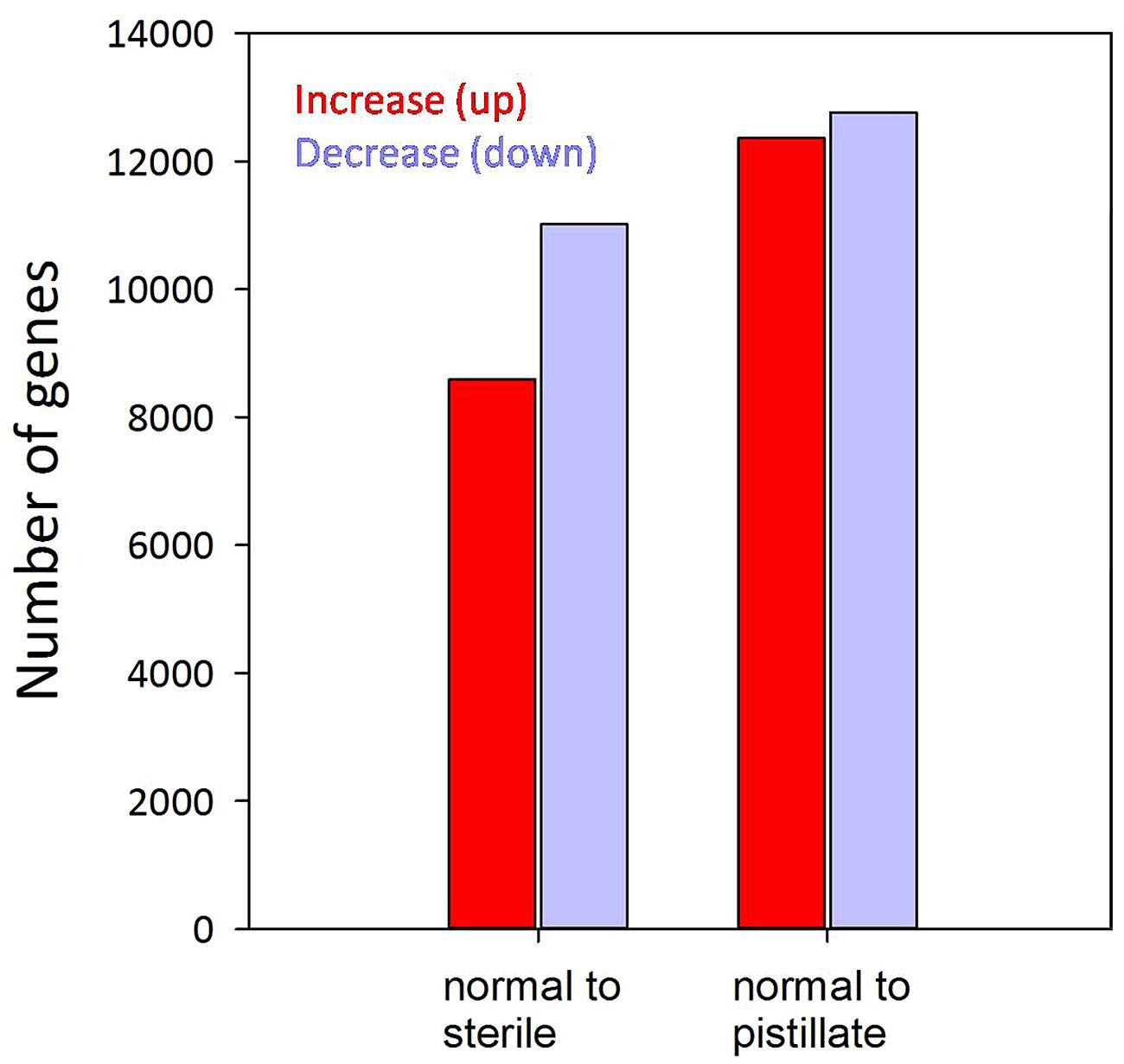
FIGURE 2. Overview of differentially (FDR ≤0.001) expressed genes. Red bars present the number of genes that increased expression while blue bars present the number of genes that decreased expression in the corresponding interval.
GO term enrichment analysis of differentially expressed genes revealed additional enriched functional categories. Genes involved in polyketide biosynthesis, oligopeptide transport, anther wall tapetum development, pectin catabolism, and negative regulation of endopeptidase activity, showed decreased expressions in the pistillate flower (Figure 3). GO terms associated with the response to red or far red light, negative regulation of circadian rhythm, ATP-dependent chromatin remodeling and protein acetylation were also enriched in the down-regulated genes of the pistillate flower. In addition, GO term annotation highlighted that genes involved in the jasmonic acid (JA) mediated signaling pathway were strongly overrepresented among the differentially expressed genes between normal and male-sterile flowers (Figure 4), and most of these genes were expressed decreasely in the male-sterile flower. Genes involved in the biosynthesis of constituents required for pollen wall development and pollen maturation, such as sporopollenin, xanthophyll, cellulose, pectin, lipid, sugar, and various pollen proteins, were included in the supercluster of JA mediated signaling pathway (Figure 4).
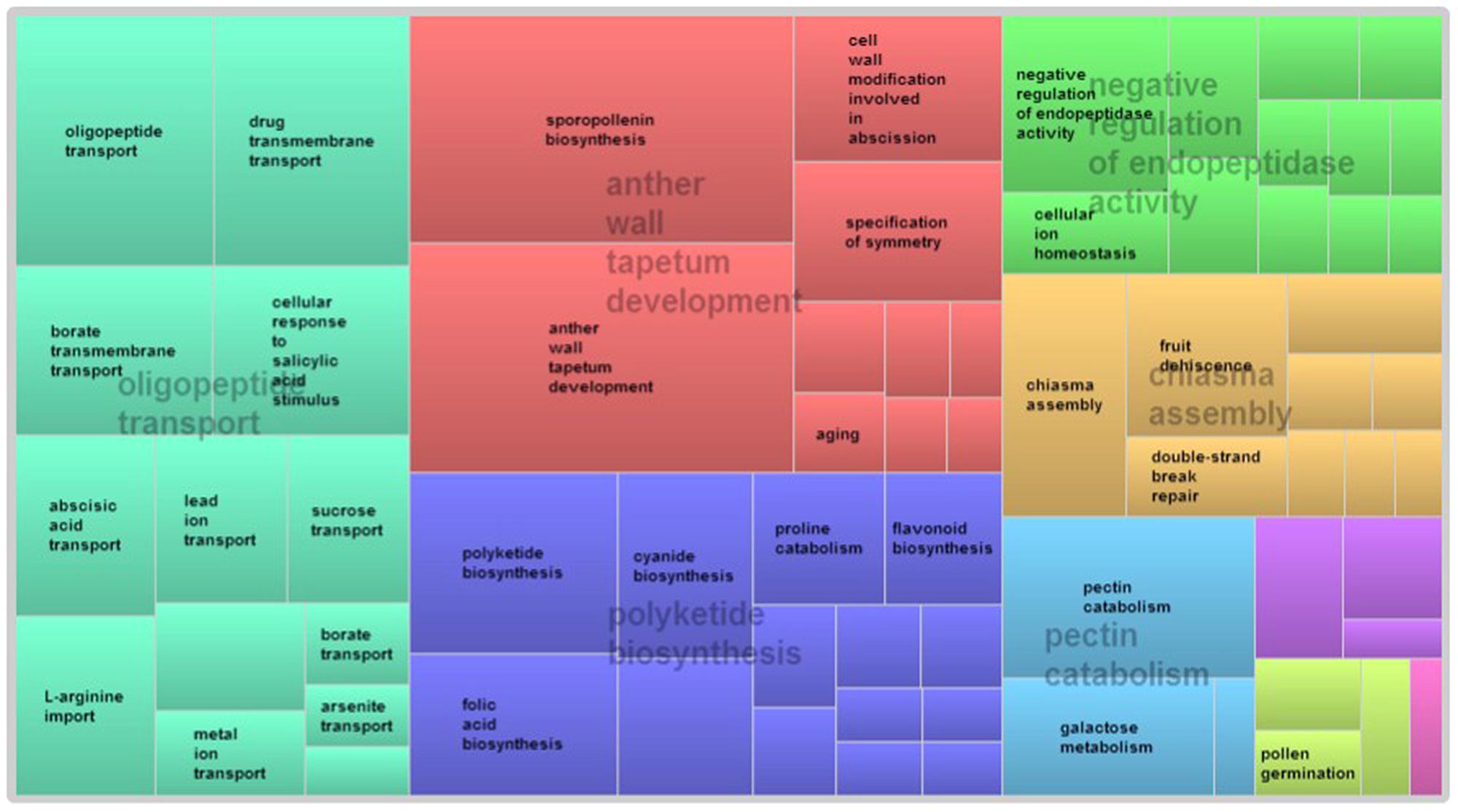
FIGURE 3. REVIGO-summarized enriched GO terms among down-regulated genes in pistillated flowers. Similar colors denote semantic similarity in the supercluster and the area of the rectangles is proportional to the significance of the over-representation of the GO term (-log10 p-value).
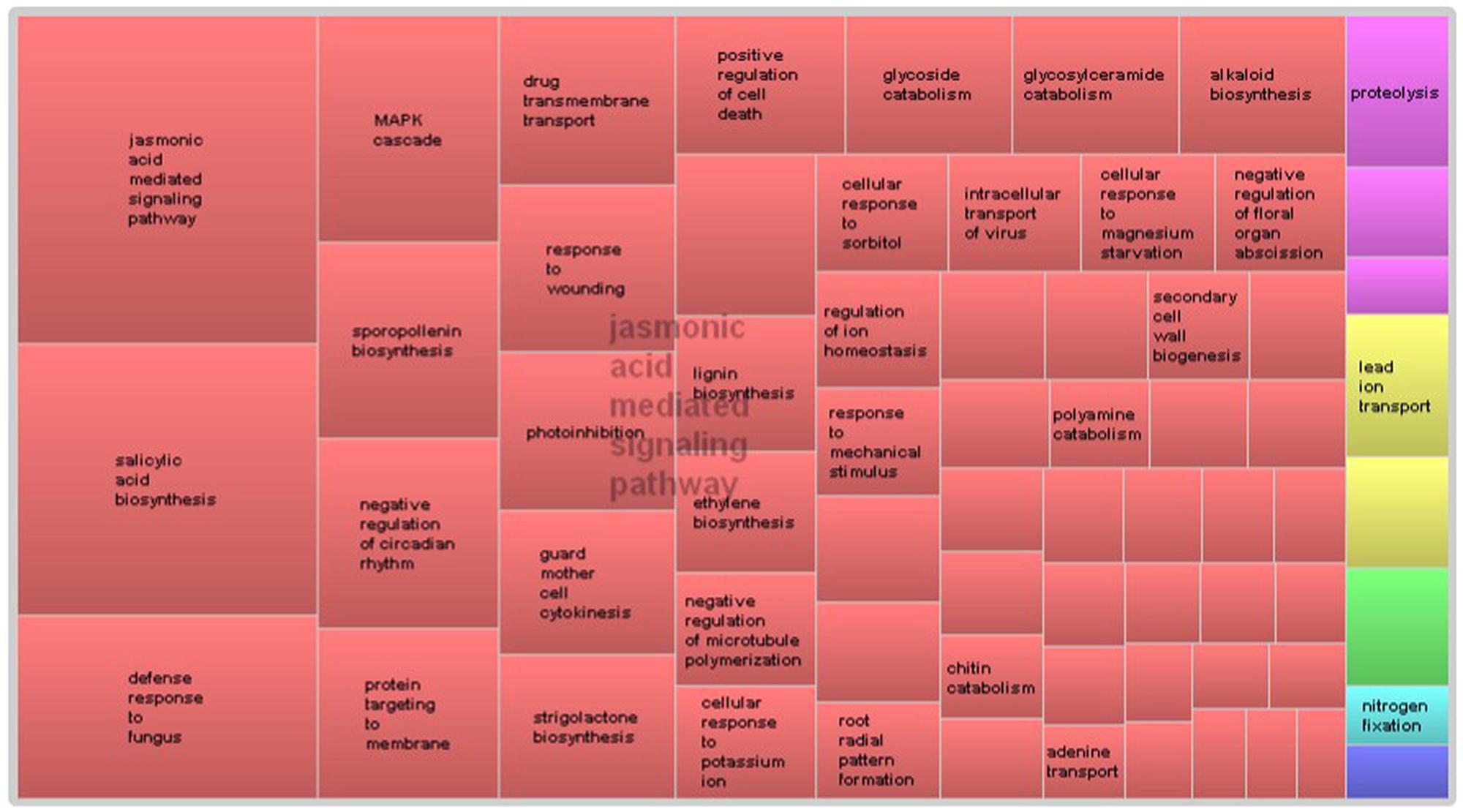
FIGURE 4. REVIGO-summarized enriched GO terms among down-regulated genes in male-sterile flowers. Similar colors denote semantic similarity in the supercluster and the area of the rectangles is proportional to the significance of the over-representation of the GO term (-log10 p-value).
Validation the Expression of B-Function and Meiotic Genes in Anomalous Stamens
Full-length cDNAs of three B-class MADS-box genes were obtained from A. philoxeroides. They were clustered, respectively, with the AP3, TM6, and PI orthologs from other plant species in the phylogenetic tree (Supplementary Figure S1), and were thus designated, respectively, as ApAP3, ApTM6, and ApPI. ApAP3 was 675 bp in length with an open reading frame corresponding to 224 deduced amino acid residues. ApTM6 was 717 bp long, encoding a 238 amino acid protein, while ApPI containing a 654 bp open reading frame. The expression patterns of ApAP3, ApTM6, and ApPI in normal and pistillate flowers were validated by qRT-PCR. The results showed that three B-class genes were all expressed in the sepals of both flowers. However, the expression levels of ApTM6 and ApPI were very low, and there were no significant differences in expression levels of three genes between two types of flowers (Figure 5). Three B-class genes were all highly expressed in the stamens of normal flowers, but the expression levels decreased by 73.2, 70.1, and 54.2%, respectively, in the stamens of pistillate flowers. Expressions of ApAP3 and ApTM6 were also detected in the carpels of both flowers but not for ApPI.
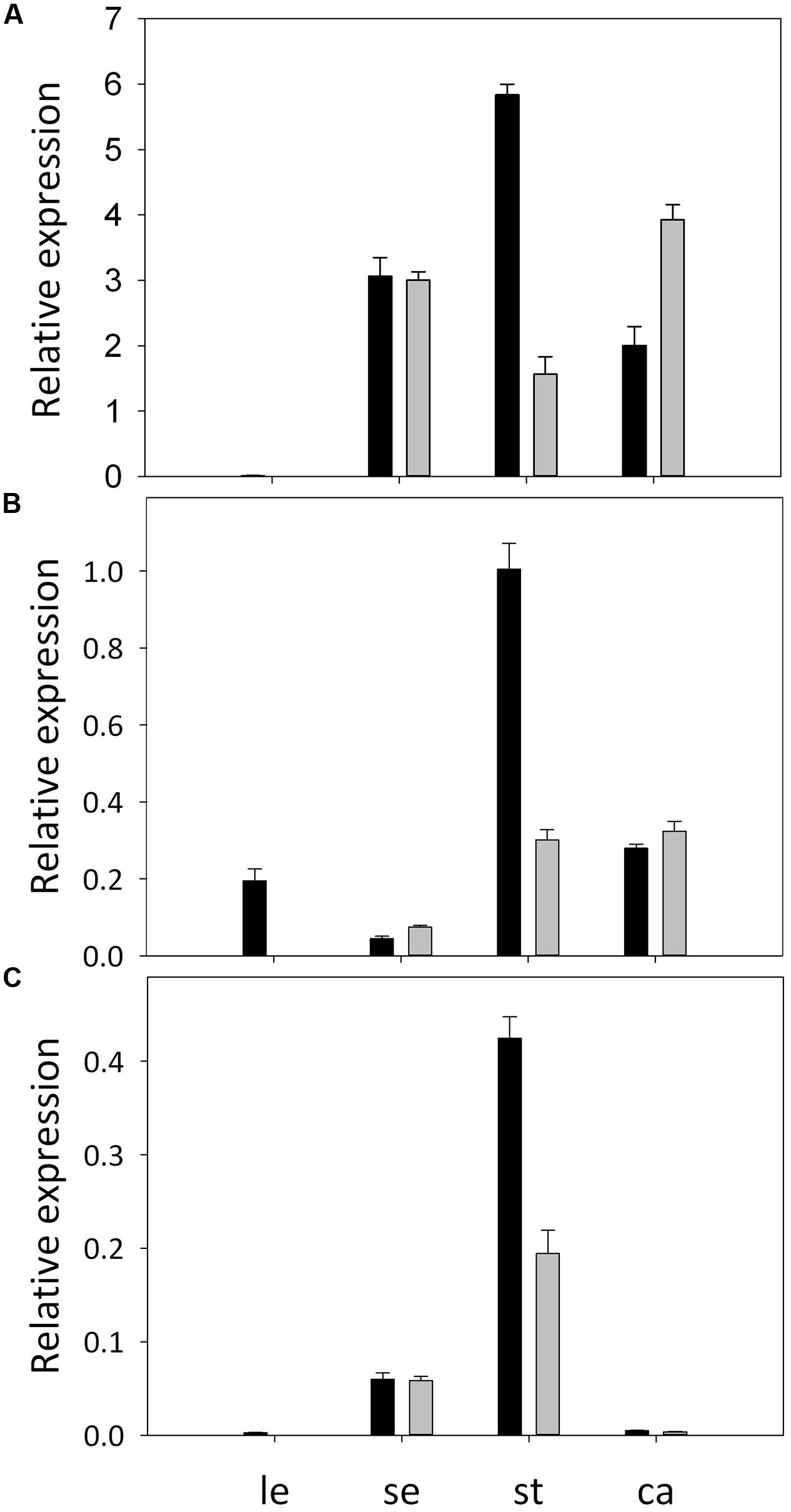
FIGURE 5. Expression patterns of B-function genes in A. philoxeroides. Relative expression of ApAP3 (A), ApTM6 (B), and ApPI (C) in tissues from normal flowers (black bars) and pistillate flowers (gray bars), as revealed by qRT-PCR. Tissues assayed are leaf (le), sepal (se), stamen (st), and carpel (ca). Error bars represent standard deviations of three replicates.
The expression patterns of four meiotic genes, ApASY1, ApMLH3, ApMPK4, and ApMMD1 involved in different processes of meiosis, were also validated by qRT-PCR. They were all expressed in the stamens of normal and male-sterile flowers, and did not show significant differences in expression levels between normal and male-sterile flowers (Figure 6).
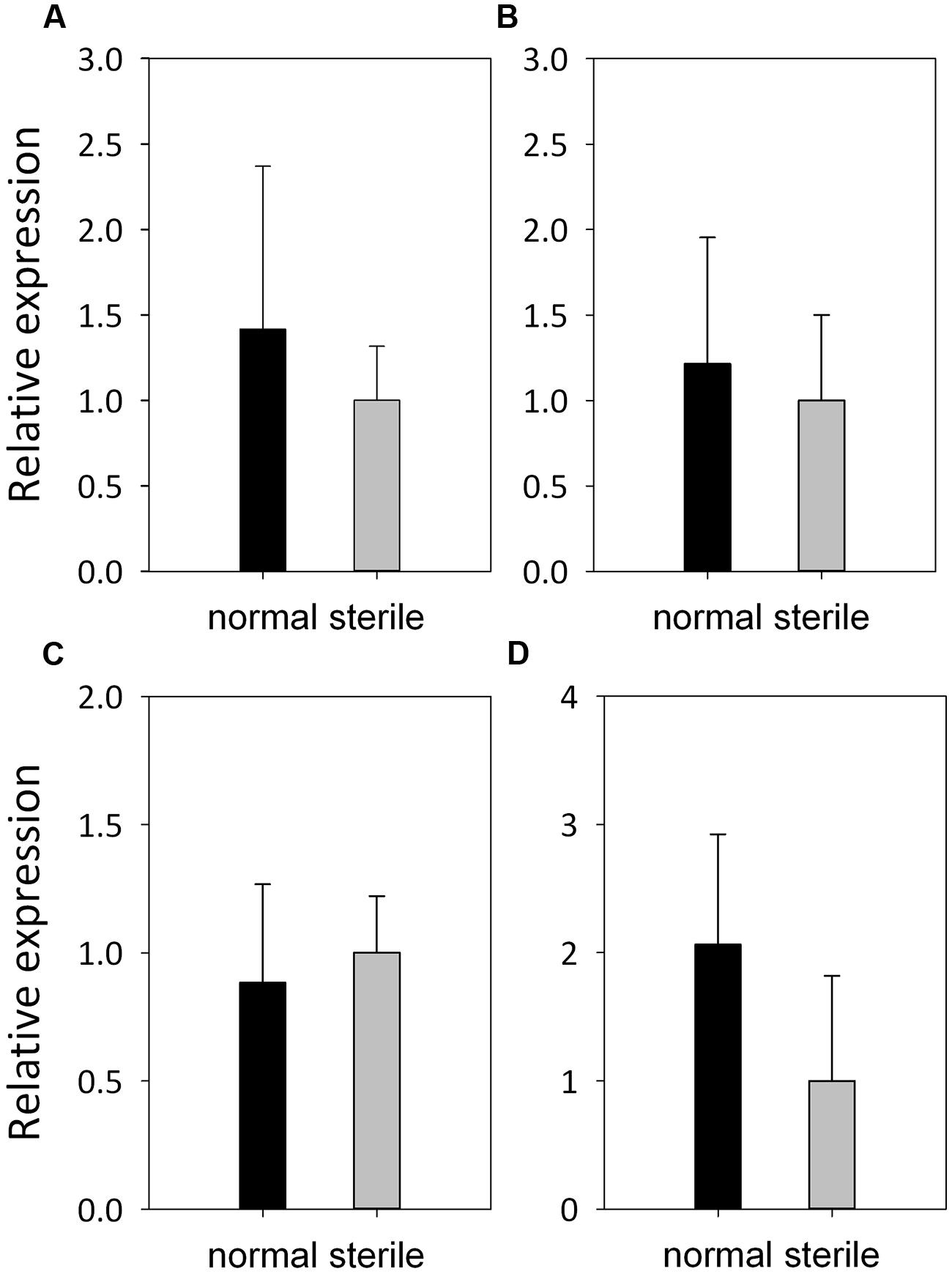
FIGURE 6. Expression patterns of meiotic genes in A. philoxeroides. Relative expression of ApASY1 (A), ApMLH3 (B), ApMPK4 (C), and ApMMD1 (D) in anthers from normal flowers (black bars) and male-sterile flowers (gray bars), as revealed by qRT-PCR. Error bars represent standard deviations of three replicates.
Discussion
Comparative transcriptome analysis revealed overall differences in gene expression between normal and anomalous flowers. Altered expressions of genes associated with stamen development were confirmed with qRT-PCR analyses. As revealed in other plants (de Martino et al., 2006), the expression levels of B-function MADS-box genes were significantly decreased in the stamens of A. philoxeroides pistillate flowers. B-function genes physically interact with C- and E-function genes to form quaternary complexes to specify stamen development (Airoldi, 2010). Because the expression levels of C- and E-function genes remained in A. philoxeroides pistillate flowers, the decreased expression of B-class genes was evidently responsible for the homeotic transformation of stamens into carpels in A. philoxeroides. Our results are in agreement with previous studies on homeotic variation in flowers. de Martino et al. (2006) showed that decreased expression of only one B-class gene could result in a complete transformation of the stamens into carpel-like organs in tomato. The deficiency of B-class MADS-box genes also caused homeotic conversions of stamens into carpels in Arabidopsis (Jack et al., 1992; Goto and Meyerowitz, 1994), Antirrhinum (Schwarz-Sommer et al., 1992; Tröbner et al., 1992), tomato (Rasmussen and Green, 1993; Olimpieri and Mazzucato, 2008) and wheat (Hama et al., 2004; Yamada et al., 2009). The reduced expression of B-function genes in A. philoxeroides seems not to result from the loss-of-function mutation in B-class genes because, by cloning and sequencing ApAP3, ApTM6, and ApPI from different plants, we did not find significant sequence variation between normal and pistillate flowers. Deng et al. (2011) and Liu et al. (2011) showed that environmental variation, especially soil nutrient heterogeneity, can induce floral gender transformation in A. philoxeroides, with the stamens of monoclinous flowers being completely or partially transformed into carpels (Deng et al., 2011; Liu et al., 2011). It is unclear, however, by which mechanisms the change in environment is sensed, transduced, and finally elicits modifications to the selective expression of B-class genes in different habitats. In addition to B-class genes, transcriptome analysis also revealed other genes that were differentially expressed between normal and pistillate flowers, and were enriched for a wide range of molecular function categories. The differential expression of genes involved in GA signaling and epigenetic regulation is of special interest. It has been revealed that floral homeotic genes (AP3 and PI) were targets of GA signaling in flower development (Yu et al., 2004). GA probably promoted stamen development by upregulating expression of the floral meristem identity gene LEAFY (LFY), which in turn upregulates expression of the B-class MADS-box gene AP3 (Plackett et al., 2011). Reduction in GA synthesis might lead to a reduced expression of AP3, and thereby produces abnormal flowers with carpelloid stamens (Kamata et al., 2013). Studies on the stamenless mutant also showed evidences that stamen identity in tomato depended on gene–hormone interactions (Quinet et al., 2014). Additionally, it has been shown that epigenetically regulated ectopic expression of flower homeotic genes may alter floral organ identity (Kapoor et al., 2005; Pu et al., 2013). Histone modification and ATP-dependent chromatin remodeling are also involved in the regulation of spatiotemporal-specific expression of genes that lead to patterning, specification, and morphogenesis of flowers (Gan et al., 2013). Mutation in the chromatin-remodeling ATPases BRAHMA led to the occurrence of carpelloid structures in the third whorl of Arabidopsis flowers (Hurtado et al., 2006; Wu et al., 2012). It has been suggested that MADS-domain proteins may closely interact with chromatin remodeling factors to facilitate chromatin opening and transcription initiation (Smaczniak et al., 2012; Guo et al., 2015).
Most of the meiotic genes investigated in this study were normally expressed in the male-sterile flower of A. philoxeroides. This result was inconsistent with our original hypothesis. The cytotype of A. philoxeroides found in China is a hexaploid, with approximately 100 chromosomes. Abnormal male meiosis is often used as a cytological explanation for pollen sterility in polyploidy plants. The most common meiotic abnormalities were those related to irregular chromosome segregation due to polyploidy, leading to the formation of chromosomally imbalanced gametes and aneuploidy. To date, little cytogenetic work has been done on the meiotic process of the invasive A. philoxeroides, due to the small size and apparent similarity of the chromosomes. It is unclear whether meiosis proceeds normally in the A. philoxeroides male-sterile flower. The normal expression of meiotic genes in the male-sterile flower seems to suggest that the meiotic abnormality is unlikely responsible for the pollen sterility observed in A. philoxeroides, or segregation defects sometimes occur during meiosis II after meiosis I has proceeded normally. Other anther developmental defects may also generate male-sterile phenotypes (Sanders et al., 1999; Sakata and Higashitani, 2008). In consistent with this prediction, we found that many genes involved in the JA mediated signaling pathway were strongly down-regulated in the male-sterile flower. JA is critical for late stages of stamen development, regulating filament elongation, anther opening, and pollen maturation. (Turner et al., 2002; Song et al., 2013; Wasternack and Hause, 2013). Arabidopsis mutants impaired in JA biosynthesis exhibited non-viable pollen and delayed anther dehiscence (Wasternack and Hause, 2013). JA signaling also played crucial roles in a variety of biosynthetic pathways for the components of pollen intine and exine, and various storage materials accumulated during pollen maturation (Mandaokar et al., 2003, 2006; Wasternack and Hause, 2013). As a result of defects in the JA signaling pathway, a lot of genes involved in the biosynthesis of constituents required for pollen wall development and pollen maturation were also down-regulated in the male-sterile flower of A. philoxeroides. Thus, defects in JA synthesis and/or JA signaling, as well as subsequent physiological disorders, might be potential causes for male sterility in A. philoxeroides.
Overall, the invasive A. philoxeroides exhibited a high level of plasticity in stamen development. This high level of plasticity is clearly resulted from relaxed selective constraints on sexual reproduction. After being introduced into China, A. philoxeroides spreads mainly by vegetative (clonal) propagules, though it retains the principal ability to reproduce both sexually and asexually in its native range. Although genetic factors, such as changes in ploidy, may play a role in causing reduced sexual fertility, the shift toward asexual reproduction is more likely promoted by biotic and/or abiotic limiting factors of the environment in exotic A. philoxeroides populations. Clonal reproduction probably helps the plants of A. philoxeroides to overcome the negative effects associated with low population densities during colonization and enhances exploitation of ubiquitous environmental heterogeneity, facilitating range expansion. Asexual reproduction is particularly common among introduced species (Kronauer et al., 2012), and shifts from sexual to asexual reproduction in the exotic range have been observed in several clonal invaders (Sculthorpe, 1967; Ornduff, 1987; Hollingsworth and Bailey, 2000). Repeated cycles of colonization and low-density may favor uniparental reproduction because selfing and asexuality provide plants with reproductive assurance (Eckert et al., 2006; Barrett et al., 2008). Meanwhile, genetic sterility may be induced by environmental suppression of sexual recruitment because natural selection no longer strongly maintains the traits involved in sex (Eckert, 2002). As a result, ‘neutral’ sterility mutations and developmental abnormalities accumulate in highly clonal populations, as shown in exotic A. philoxeroides plants. Thus, the occurrence of various types of stamen abnormalities could be explained by the hypothesis that sex were degraded for they no longer increase fitness (Larkin et al., 2007; Tamura et al., 2013). Sexual sterility may be first induced by ecological factors, the resulting genetic sterility may, in turn, further hamper sexual recruitment in clonal populations, facilitating the evolution of asexual reproduction in clonal plants.
Author Contributions
ZZ collaborated in the design of the research, collected plant materials, prepared RNA samples for high-throughput sequencing, performed the bioinformatics analyses, interpreted the results and wrote the first draft of the manuscript. CZ helped in the design of the experiments as well as RNA samples preparation, performed the experiments with B-class MADS-box genes, including plant materials collection, cDNA full-length cloning, phylogenetic analysis and qRT-PCR. Moreover, she made graphs and wrote the draft about B-class MADS-box genes. JY made substantial contributions to the design of the research, analysis of next-generation sequencing data and manuscript revision. All authors have read the final version of the manuscript and agree with its content.
Conflict of Interest Statement
The authors declare that the research was conducted in the absence of any commercial or financial relationships that could be construed as a potential conflict of interest.
Acknowledgments
This work was supported by the Ministry of Science and Technology of China (Grant No.2011CB944604), National Natural Science Foundation of China (Grant No. 31070201), and the Shanghai Municipal Science and Technology Commission (Grant No. 14DZ 2260400). We are grateful to Ms. Luoyan Zhang for assistance in bioinfomatic data analysis.
Supplementary Material
The Supplementary Material for this article can be found online at: http://www.frontiersin.org/journal/10.3389/fpls.2015.00242/abstract
Footnotes
- ^http://www.arabidopsis.org/
- ^http://www.phytozome.net/
- ^http://revigo.irb.hr/
- ^http://www.ncbi.nlm.nih.gov/
- ^http://bioinfo.ut.ee/primer3/
References
Airoldi, C. A. (2010). Determination of sexual organ development. Sex. Plant Reprod. 23, 53–62. doi: 10.1007/s00497-009-0126-z
PubMed Abstract | Full Text | CrossRef Full Text | Google Scholar
Alexa, A., and Rahnenfuhrer, J. (2010). topGO: Enrichment Analysis for Gene Ontology. R Package Version 2.8.
Armstrong, S. J., Caryl, A. P., Jones, G. H., and Franklin, F. C. H. (2002). Asy1, a protein required for meiotic chromosome synapsis, localizes to axis-associated chromatin in Arabidopsis and Brassica. J. Cell Sci. 115, 3645–3655. doi: 10.1242/jcs.00048
PubMed Abstract | Full Text | CrossRef Full Text | Google Scholar
Barrett, S. C., Colautti, R. I., and Eckert, C. G. (2008). Plant reproductive systems and evolution during biological invasion. Mol. Ecol. 17, 373–383. doi: 10.1111/j.1365-294X.2007.03503.x
PubMed Abstract | Full Text | CrossRef Full Text | Google Scholar
Budar, F., and Pelletier, G. (2001). Male sterility in plants: occurrence, determinism, significance and use. C. R. Acad. Sci. Paris. Sciences de la vie 324, 543–550.
Cai, H., Wei, C. L., and Chen, N. (2009). Chromosome karyotype characters of biological invasion in Alternanthera philoxeroides. Chinese J. Trop. Crop. 30, 530–534.
Chen, Z. (1964). Feminization of stamens of Altemanthera philoxeroides Grisebich. Acta Bot. Sinica 12, 133–137.
Coen, E. S., and Meyerowitz, E. M. (1991). The war of the whorls: genetic interactions controlling flower development. Nature 353, 31–37. doi: 10.1038/353031a0
PubMed Abstract | Full Text | CrossRef Full Text | Google Scholar
de Azevedo Souza, C., Kim, S. S., Koch, S., Kienow, L., Schneider, K., Mckim, S. M.,et al. (2009). A novel fatty acyl-CoA synthetase is required for pollen development and sporopollenin biosynthesis in Arabidopsis. Plant Cell 21, 507–525. doi: 10.1105/tpc.108.062513
PubMed Abstract | Full Text | CrossRef Full Text | Google Scholar
de Martino, G., Pan, I., Emmanuel, E., Levy, A., and Irish, V. F. (2006). Functional analyses of two tomato APETALA3 genes demonstrate diversification in their roles in regulating floral development. Plant Cell 18, 1833–1845. doi: 10.1105/tpc.106.042978
PubMed Abstract | Full Text | CrossRef Full Text | Google Scholar
Deng, R. F., Liu, W. P., and Liu, W. (2011). Research on the environmental factors regulating the phenotypic states of gender plasticity in Alternanthera philoxeroides. Guihaia 31, 485–489.
Eckert, C. G. (2002). The loss of sex in clonal plants. Evol. Ecol. 15, 501–520. doi: 10.1023/A:1016005519651
Eckert, C. G., and Barrett, S. C. (1993). Clonal reproduction and patterns of genotypic diversity in Decodon verticillatus (Lythraceae). Am. J. Bot. 80, 1175–1182. doi: 10.2307/2445546
Eckert, C. G., Samis, K. E., and Dart, S. (2006). “Reproductive assurance and the evolution of uniparental reproduction in flowering plants,” in Ecology and Evolution of Flowers, eds L. D. Harder and S. C. Barrett (Oxford, UK: Oxford University Press), 183–203.
Gan, E.-S., Huang, J., and Ito, T. (2013). Functional roles of histone modification, chromatin remodeling and microRNAs in Arabidopsis flower development. Int. Rev. Cell Mol. Biol. 305, 115–161. doi: 10.1016/B978-0-12-407695-2.00003-2
PubMed Abstract | Full Text | CrossRef Full Text | Google Scholar
Geng, Y. P., Pan, X. Y., Xu, C. Y., Zhang, W. J., Li, B., and Chen, J. K. (2006). Phenotypic plasticity of invasive Alternanthera philoxeroides in relation to different water availability, compared to its native congener. Acta Oecol. 30, 380–385. doi: 10.1016/j.actao.2006.07.002
Geng, Y. P., Pan, X. Y., Xu, C. Y., Zhang, W. J., Li, B., Chen, J. K.,et al. (2007). Phenotypic plasticity rather than locally adapted ecotypes allows the invasive alligator weed to colonize a wide range of habitats. Biol. Invasions 9, 245–256. doi: 10.1007/s10530-006-9029–9021
Goto, K., and Meyerowitz, E. M. (1994). Function and regulation of the Arabidopsis floral homeotic gene PISTILLATA. Genes Dev. 8, 1548–1560. doi: 10.1101/gad.8.13.1548
PubMed Abstract | Full Text | CrossRef Full Text | Google Scholar
Grabherr, M. G., Haas, B. J., Yassour, M., Levin, J. Z., Thompson, D. A., Amit, I.,et al. (2011). Full-length transcriptome assembly from RNA-Seq data without a reference genome. Nat. Biotechnol. 29, 644–652. doi: 10.1038/nbt.1883
PubMed Abstract | Full Text | CrossRef Full Text | Google Scholar
Guo, S., Sun, B., Looi, L.-S., Xu, Y., Gan, E.-S., Huang, J.,et al. (2015). Coordination of flower development through epigenetic regulation in two model species: rice and Arabidopsis. Plant Cell Physiol. doi: 10.1093/pcp/pcv037 [Epub ahead of print].
PubMed Abstract | Full Text | CrossRef Full Text | Google Scholar
Hama, E., Takumi, S., Ogihara, Y., and Murai, K. (2004). Pistillody is caused by alterations to the class-B MADS-box gene expression pattern in alloplasmic wheats. Planta 218, 712–720. doi: 10.1007/s00425-003-1157-6
PubMed Abstract | Full Text | CrossRef Full Text | Google Scholar
Held, T., Nourmohammad, A., and Laessig, M. (2014). Adaptive evolution of molecular phenotypes. J. Stat. Mech.-Theory E. doi: 10.1088/1742–5468/2014/09/p09029
Hollingsworth, M. L., and Bailey, J. P. (2000). Evidence for massive clonal growth in the invasive weed Fallopia japonica (Japanese Knotweed). Bot. J. Linn. Soc. 133, 463–472. doi: 10.1006/bojl.2000.0359
Hu, F. Y., Chang, Q. S., Zhang, L. X., Qiu, M., and Wang, Q. Y. (2011). Comparative study on the development of stamen and feminisation stamens of Alternanthera philoxeroides. Guihaia 31, 444–450.
Huang, X. Q., and Madan, A. (1999). CAP3: a DNA sequence assembly program. Genome Res. 9, 868–877. doi: 10.1101/gr.9.9.868
PubMed Abstract | Full Text | CrossRef Full Text | Google Scholar
Hurtado, L., Farrona, S., and Reyes, J. C. (2006). The putative SWI/SNF complex subunit BRAHMA activates flower homeotic genes in Arabidopsis thaliana. Plant Mol. Biol. 62, 291–304. doi: 10.1007/s11103-006-9021-2
PubMed Abstract | Full Text | CrossRef Full Text | Google Scholar
Jack, T., Brockman, L. L., and Meyerowitz, E. M. (1992). The homeotic gene APETALA3 of Arabidopsis thaliana encodes a MADS box and is expressed in petals and stamens. Cell 68, 683–697. doi: 10.1016/0092-8674(92)90144–90142
PubMed Abstract | Full Text | CrossRef Full Text | Google Scholar
Jackson, N., Sanchez-Moran, E., Buckling, E., Armstrong, S. J., Jones, G. H., and Franklin, F. C. H. (2006). Reduced meiotic crossovers and delayed prophase I progression in AtMLH3-deficient Arabidopsis. EMBO J. 25, 1315–1323. doi: 10.1038/sj.emboj.7600992
PubMed Abstract | Full Text | CrossRef Full Text | Google Scholar
Julien, M. H., and Stanley, J. N. (1999). “The management of alligator weed, a challenge for the new millennium,” in Biennial Noxious Weeds Conference, eds R. Ensbey, P. Blackmore, and A. Simpson (Ballina, AT: NSW Agriculture).
Jung, K.-H., Han, M.-J., Lee, D.-Y., Lee, Y.-S., Schreiber, L., Franke, R.,et al. (2006). Wax-deficient anther1 is involved in cuticle and wax production in rice anther walls and is required for pollen development. Plant Cell 18, 3015–3032. doi: 10.1105/tpc.106.042044
PubMed Abstract | Full Text | CrossRef Full Text | Google Scholar
Kamata, N., Okada, H., Komeda, Y., and Takahashi, T. (2013). Mutations in epidermis-specific HD-ZIP IV genes affect floral organ identity in Arabidopsis thaliana. Plant J. 75, 430–440. doi: 10.1111/tpj.12211
PubMed Abstract | Full Text | CrossRef Full Text | Google Scholar
Kapoor, M., Baba, A., Kubo, K. I., Shibuya, K., Matsui, K., Tanaka, Y.,et al. (2005). Transgene-triggered, epigenetically regulated ectopic expression of a flower homeotic gene pMADS3 in Petunia. Plant J. 43, 649–661. doi: 10.1111/j.1365-313X.2005.02481.x
PubMed Abstract | Full Text | CrossRef Full Text | Google Scholar
Kronauer, D. J., Pierce, N. E., and Keller, L. (2012). Asexual reproduction in introduced and native populations of the ant Cerapachys biroi. Mol. Ecol. 21, 5221–5235. doi: 10.1111/mec.12041
PubMed Abstract | Full Text | CrossRef Full Text | Google Scholar
Larkin, M. A., Blackshields, G., Brown, N., Chenna, R., Mcgettigan, P. A., Mcwilliam, H.,et al. (2007). Clustal W and Clustal X version 2.0. Bioinformatics 23, 2947–2948. doi: 10.1093/bioinformatics/btm404
PubMed Abstract | Full Text | CrossRef Full Text | Google Scholar
Li, J., and Ye, W. H. (2006). Genetic diversity of alligator weed ecotypes is not the reason for their different responses to biological control. Aquat. Bot. 85, 155–158. doi: 10.1016/j.aquabot.2006.02.006
Li, W. Z., and Godzik, A. (2006). Cd-hit: a fast program for clustering and comparing large sets of protein or nucleotide sequences. Bioinformatics 22, 1658–1659. doi: 10.1093/bioinformatics/btl158
PubMed Abstract | Full Text | CrossRef Full Text | Google Scholar
Liu, W., Deng, R. F., Liu, W. P., Wang, Z. M., Ye, W. H., Wang, L. Y.,et al. (2011). Phenotypic differentiation is associated with gender plasticity and its responsive delay to environmental changes in Alternanthera philoxeroides – phenotypic differentiation in alligator weed. PLoS ONE 6:e27238. doi: 10.1371/journal.pone.0027238
PubMed Abstract | Full Text | CrossRef Full Text | Google Scholar
Livak, K. J., and Schmittgen, T. D. (2001). Analysis of relative gene expression data using real-time quantitative PCR and the 2- ΔΔCT method. Methods 25, 402–408. doi: 10.1006/meth.2001.1262
PubMed Abstract | Full Text | CrossRef Full Text | Google Scholar
Mandaokar, A., Kumar, V. D., and Amway, M. (2003). Microarray and differential display identify genes involved in jasmonate-dependent anther development. Plant Mol. Biol. 52, 775–786. doi: 10.1023/A:1025045217859
PubMed Abstract | Full Text | CrossRef Full Text | Google Scholar
Mandaokar, A., Thines, B., Shin, B., Markus Lange, B., Choi, G., Koo, Y. J.,et al. (2006). Transcriptional regulators of stamen development in Arabidopsis identified by transcriptional profiling. Plant J. 46, 984–1008. doi: 10.1111/j.1365-313X.2006.02756.x
PubMed Abstract | Full Text | CrossRef Full Text | Google Scholar
Mortazavi, A., Williams, B. A., Mccue, K., Schaeffer, L., and Wold, B. (2008). Mapping and quantifying mammalian transcriptomes by RNA-Seq. Nat. Methods 5, 621–628. doi: 10.1038/nmeth.1226
PubMed Abstract | Full Text | CrossRef Full Text | Google Scholar
Olimpieri, I., and Mazzucato, A. (2008). Phenotypic and genetic characterization of the pistillate mutation in tomato. Theor. Appl. Genet. 118, 151–163. doi: 10.1007/s00122-008-0884-2
PubMed Abstract | Full Text | CrossRef Full Text | Google Scholar
Ornduff, R. (1987). Reproductive systems and chromosome races of Oxalis pes-caprae L. and their bearing on the genesis of a noxious weed. Ann. Mo. Bot. Gard. 74, 79–84. doi: 10.2307/2399264
Osman, K., Higgins, J. D., Sanchez-Moran, E., Armstrong, S. J., and Franklin, F. C. H. (2011). Pathways to meiotic recombination in Arabidopsis thaliana. New Phytol. 190, 523–544. doi: 10.1111/j.1469-8137.2011.03665.x
PubMed Abstract | Full Text | CrossRef Full Text | Google Scholar
Plackett, A. R., Thomas, S. G., Wilson, Z. A., and Hedden, P. (2011). Gibberellin control of stamen development: a fertile field. Trends Plant Sci. 16, 568–578. doi: 10.1016/j.tplants.2011.06.007
PubMed Abstract | Full Text | CrossRef Full Text | Google Scholar
Pu, L., Liu, M. S., Kim, S. Y., Chen, L. F. O., Fletcher, J. C., and Sung, Z. R. (2013). EMBRYONIC FLOWER1 and ULTRAPETALA1 act antagonistically on Arabidopsis development and stress response. Plant Physiol. 162, 812–830. doi: 10.1104/pp.112.213223
PubMed Abstract | Full Text | CrossRef Full Text | Google Scholar
Quinet, M., Bataille, G., Dobrev, P. I., Capel, C., Gómez, P., Capel, J.,et al. (2014). Transcriptional and hormonal regulation of petal and stamen development by STAMENLESS, the tomato (Solanum lycopersicum L.) orthologue to the B-class APETALA3 gene. J. Exp. Bot. 65, 2243–2256. doi: 10.1093/jxb/eru089
PubMed Abstract | Full Text | CrossRef Full Text | Google Scholar
Rasmussen, N., and Green, P. B. (1993). Organogenesis in flowers of the homeotic green pistillate mutant of tomato (Lycopersicon esculentum). Am. J. Bot. 80, 805–813. doi: 10.2307/2445600
Riechmann, J. L., and Meyerowitz, E. M. (1997). MADS domain proteins in plant development. Biol. Chem. 378, 1079–1102.
Sakata, T., and Higashitani, A. (2008). Male sterility accompanied with abnormal anther development in plants–genes and environmental stresses with special reference to high temperature injury. Int. J. Plant Dev. Biol. 2, 42–51.
Sanders, P. M., Bui, A. Q., Weterings, K., Mcintire, K. N., Hsu, Y. C., Lee, P. Y.,et al. (1999). Anther developmental defects in Arabidopsis thaliana male-sterile mutants. Sex. Plant Reprod. 11, 297–322. doi: 10.1007/s004970050158
Sawhney, V. K., and Shukla, A. (1994). Male sterility in flowering plants: are plant growth substances involved? Am. J. Bot. 81, 1640–1647. doi: 10.2307/2445343
PubMed Abstract | Full Text | CrossRef Full Text | Google Scholar
Schwarz-Sommer, Z., Hue, I., Huijser, P., Flor, P., Hansen, R., Tetens, F.,et al. (1992). Characterization of the Antirrhinum floral homeotic MADS-box gene deficiens: evidence for DNA binding and autoregulation of its persistent expression throughout flower development. EMBO J. 11, 251–263.
Singh, S. P., Roy, J. K., Kumar, D., and Sawant, S. V. (2012). “Tools for generating male sterile plants,” in Frontiers on Recent Developments in Plant Science, eds A. Goyal and P. Maheshwari (Sharjah: Bentham Science Publishers), 67–85.
Smaczniak, C., Immink, R. G., Muiño, J. M., Blanvillain, R., Busscher, M., Busscher-Lange, J.,et al. (2012). Characterization of MADS-domain transcription factor complexes in Arabidopsis flower development. Proc. Natl. Acad. Sci. U.S.A. 109, 1560–1565. doi: 10.1073/pnas.1112871109
PubMed Abstract | Full Text | CrossRef Full Text | Google Scholar
Song, S., Qi, T., Huang, H., and Xie, D. (2013). Regulation of stamen development by coordinated actions of jasmonate, auxin, and gibberellin in Arabidopsis. Mol. Plant 6, 1065–1073. doi: 10.1093/mp/sst054
PubMed Abstract | Full Text | CrossRef Full Text | Google Scholar
Sosa, A. J., Greizerstein, E., Cardo, M. V., Telesnicki, M. C., and Julien, M. H. (2007). “The evolutionary history of an invasive species: alligator weed, Alternanthera philoxeroides”, in Proceedings of the International Symposium on Biological Control of Weeds (La Grande-Motte: Año)
Supek, F., Bošnjak, M., Škunca, N., and Šmuc, T. (2011). REVIGO summarizes and visualizes long lists of gene ontology terms. PLoS ONE 6:e21800 doi: 10.1371/journal.pone.0021800
PubMed Abstract | Full Text | CrossRef Full Text | Google Scholar
Tamura, K., Stecher, G., Peterson, D., Filipski, A., and Kumar, S. (2013). MEGA6: Molecular evolutionary genetics analysis version 6.0. Mol. Biol. Evol. 30, 2725–2729. doi: 10.1093/molbev/mst197
PubMed Abstract | Full Text | CrossRef Full Text | Google Scholar
Theißen, G. (2001). Development of floral organ identity: stories from the MADS house. Curr. Opin. Plant Biol. 4, 75–85. doi: 10.1016/S1369-5266(00)00139-4
PubMed Abstract | Full Text | CrossRef Full Text | Google Scholar
Tröbner, W., Ramirez, L., Motte, P., Hue, I., Huijser, P., Lonnig, W. E.,et al. (1992). GLOBOSA: a homeotic gene which interacts with DEFICIENS in the control of Antirrhinum floral organogenesis. EMBO J. 11, 4693–4704.
Turner, J. G., Ellis, C., and Devoto, A. (2002). The jasmonate signal pathway. Plant Cell 14, S153–S164. doi: 10.1105/tpc.000679
Vandenbussche, M., Theissen, G., Van De Peer, Y., and Gerats, T. (2003). Structural diversification and neo-functionalization during floral MADS-box gene evolution by C-terminal frameshift mutations. Nucleic Acids Res. 31, 4401–4409. doi: 10.1093/nar/gkg642
PubMed Abstract | Full Text | CrossRef Full Text | Google Scholar
Wang, G. Q., Gao, R. R., Wang, Y. L., and Chai, R. J. (2011). Structure foundation and ecological adaptability of Alternathera philoxeroides in heterogeneous habitats. Acta Prataculturae Sin. 20, 143–152.
Wasternack, C., and Hause, B. (2013). Jasmonates: biosynthesis, perception, signal transduction and action in plant stress response, growth and development. An update to the 2007 review in Annals of Botany. Ann. Bot. 111, 1021–1058. doi: 10.1093/aob/mct067
PubMed Abstract | Full Text | CrossRef Full Text | Google Scholar
Wu, M. F., Sang, Y., Bezhani, S., Yamaguchi, N., Han, S. K., Li, Z. T.,et al. (2012). SWI2/SNF2 chromatin remodeling ATPases overcome polycomb repression and control floral organ identity with the LEAFY and SEPALLATA3 transcription factors. Proc. Natl. Acad. Sci. U.S.A. 109, 3576–3581. doi: 10.1073/pnas.1113409109
PubMed Abstract | Full Text | CrossRef Full Text | Google Scholar
Yamada, K., Saraike, T., Shitsukawa, N., Hirabayashi, C., Takumi, S., and Murai, K. (2009). Class D and Bsister MADS-box genes are associated with ectopic ovule formation in the pistil-like stamens of alloplasmic wheat (Triticum aestivum L.) . Plant Mol. Biol. 71, 1–14. doi: 10.1007/s11103-009-9504-z
PubMed Abstract | Full Text | CrossRef Full Text | Google Scholar
Yang, S. L., Xie, L. F., Mao, H. -Z., San Puah, C., Yang, W. C., Jiang, L.,et al. (2003a). Tapetum determinant1 is required for cell specialization in the Arabidopsis anther. Plant Cell 15, 2792–2804. doi: 10.1105/tpc.016618
PubMed Abstract | Full Text | CrossRef Full Text | Google Scholar
Yang, X., Makaroff, C. A., and Ma, H. (2003b). The Arabidopsis MALE MEIOCYTE DEATH1 gene encodes a PHD-finger protein that is required for male meiosis. Plant Cell 15, 1281–1295. doi: 10.1105/tpc.010447
PubMed Abstract | Full Text | CrossRef Full Text | Google Scholar
Ye, W. H., Li, J., Cao, H. L., and Ge, X. J. (2003). Genetic uniformity of Alternanthera philoxeroides in South China. Weed Res. 43, 297–302. doi: 10.1046/j.1365–3180.2003.00346.x
Yu, H., Ito, T., Zhao, Y., Peng, J., Kumar, P., and Meyerowitz, E. M. (2004). Floral homeotic genes are targets of gibberellin signaling in flower development. Proc. Natl. Acad. Sci. U.S.A. 101, 7827–7832. doi: 10.1073/pnas.0402377101
PubMed Abstract | Full Text | CrossRef Full Text | Google Scholar
Zeng, Q., Chen, J. G., and Ellis, B. E. (2011). AtMPK4 is required for male-specific meiotic cytokinesis in Arabidopsis. Plant J. 67, 895–906. doi: 10.1111/j.1365-313X.2011.04642.x
PubMed Abstract | Full Text | CrossRef Full Text | Google Scholar
Zhong, S., Joung, J. G., Zheng, Y., Chen, Y. R., Liu, B., Shao, Y.,et al. (2011). High-throughput illumina strand-specific RNA sequencing library preparation. Cold Spring Harb. Protoc. 2011, 940–949. doi: 10.1101/pdb.prot5652
PubMed Abstract | Full Text | CrossRef Full Text | Google Scholar
Keywords: Alternanthera philoxeroide, sexual dysfunction, aberrant stamen development, male sterility, molecular phenotypes
Citation: Zhu Z, Zhou C and Yang J (2015) Molecular phenotypes associated with anomalous stamen development in Alternanthera philoxeroides. Front. Plant Sci. 6:242. doi: 10.3389/fpls.2015.00242
Received: 30 December 2014; Accepted: 26 March 2015;
Published online: 14 April 2015
Edited by:
Kang Chong, Institue of Botany – Chinese Academy of Sciences, ChinaReviewed by:
Hongzhi Kong, Institute of Botany – Chinese Academy of Sciences, ChinaPingfang Yang, Wuhan Botanical Garden – Chinese Academy of Sciences, China
Copyright © 2015 Zhu, Zhou and Yang. This is an open-access article distributed under the terms of the Creative Commons Attribution License (CC BY). The use, distribution or reproduction in other forums is permitted, provided the original author(s) or licensor are credited and that the original publication in this journal is cited, in accordance with accepted academic practice. No use, distribution or reproduction is permitted which does not comply with these terms.
*Correspondence: Ji Yang, Ministry of Education Key Laboratory for Biodiversity Science and Ecological Engineering, Center for Evolutionary Biology, School of Life Sciences, Fudan University, 2005 Songhu Road, Shanghai 200438, Chinaaml5YW5nQGZ1ZGFuLmVkdS5jbg==