- 1College of Biological Sciences and Biotechnology, Beijing Forestry University, Beijing, China
- 2Institute of Cellular and Molecular Botany, University of Bonn, Bonn, Germany
- 3Department of Biological Sciences, Tokyo Metropolitan University, Tokyo, Japan
Light can penetrate several centimeters below the soil surface. Growth, development and behavior of plant roots are markedly affected by light despite their underground lifestyle. Early studies provided contrasting information on the spatial and temporal distribution of light-sensing cells in the apical region of root apex and discussed the physiological roles of plant hormones in root responses to light. Recent biological and microscopic advances have improved our understanding of the processes involved in the sensing and transduction of light signals, resulting in subsequent physiological and behavioral responses in growing root apices. Here, we review current knowledge of cellular distributions of photoreceptors and their signal transduction pathways in diverse root tissues and root apex zones. We are discussing also the roles of auxin transporters in roots exposed to light, as well as interactions of light signal perceptions with sensing of other environmental factors relevant to plant roots.
Introduction
Roots, the underground organ of all terrestrial plants, do not grow in a completely dark environment. Actually, sunlight can penetrate several millimeters beneath the soil surface, affecting the development of root architecture and guiding the growth direction of roots (Woolley and Stoller, 1978; Tester and Morris, 1987). When sunlight strikes the ground, the spectral characters of light are altered with depth under the soil surface (Figure 1A; Kasperbauer and Hunt, 1988; Mandoli et al., 1990). Photons in the red and far-red part of the spectrum can penetrate deeper than blue light photons. Furthermore, vascular tissue can conduct light to the roots over several centimeters and, again, red to far-red light reaches deeper than blue light (Briggs and Mandoli, 1984; Sun et al., 2003, 2005). Plants have evolved complex and extremely sensitive light sensing systems to react properly to light of different spectra. Plants have several classes of sensory photoreceptors, including the UV-B photoreceptor, UV-A/blue (B) light receptors and red (R)/far-red (FR) receptors (Briggs and Lin, 2012). Most members of these photoreceptors can be expressed in plant roots, giving roots the ability to sense light at wavelengths from the spectral UV-B to FR regions. For laboratory maintained Arabidopsis seedlings, when shoots and cotyledons are exposed to light and roots are grown in shadowed conditions, the root growth and the root-shoot ratio change prominently (Xu et al., 2013; Yokawa et al., 2013, 2014; Novák et al., 2015). Young seedlings with illuminated roots have shorter hypocotyls and longer roots (Novák et al., 2015). The shading roots condition was applied via new method “GLO-Roots” to analyze the root system architecture, showing that light changes the root architecture (Rellán-Álvarez et al., 2015). Importantly, the phot1 mutant is not affected by light exposure (Rellán-Álvarez et al., 2015). Arabidopsis roots exposed to continuous light generate immediate burst of reactive oxygen species (ROS) and show significantly altered responses to salt stresses (Yokawa et al., 2011, 2014). Moreover, Gundel et al. (2014) have hypothesized that the light perceived by the shoots and canopy cover could affect the root architecture. Glucose, synthesized by the photosynthetic process, influences root growth direction and root architecture by adjusting transport and response of phytohormones, for instance, brassinosteroids, auxin, cytokinin, and ethylene (Kircher and Schopfer, 2012; Roycewicz and Malamy, 2012; Singh et al., 2014a,b). Light, directly or/and indirectly, affects root growth, lateral root initiation, root hair formation and root gravitropic and phototropic bending (Lake and Slack, 1961; Klemmer and Schneider, 1979; Burbach et al., 2012; Hopkins and Kiss, 2012; Wan et al., 2012). Another newly proposed system for cultivating young Arabidopsis seedlings with shaded roots is a D–root system (Silva-Navas et al., 2015). In the D-Root system, the light comes from the top and shoots perceive the same amount and intensity of light whereas roots do not get any light. Only in the modified D-Root system, used to analyze specific wavelengths, the light is provided frontally (Silva-Navas et al., 2015).
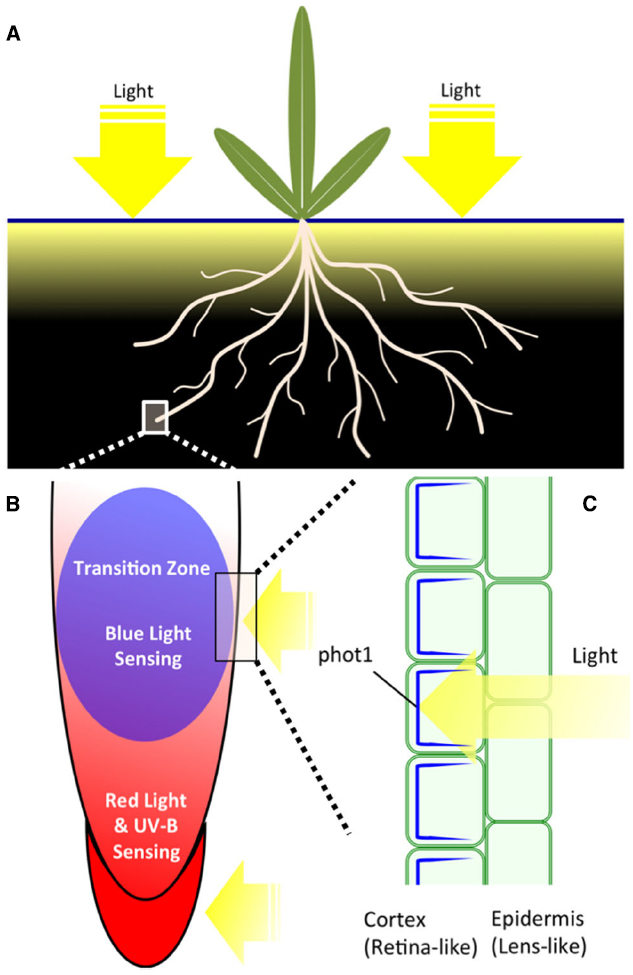
Figure 1. (A) Plant organs and their light environment. Shoot part of plants is fully exposed to light during a day. Root part is exposed only to limited amounts of light which penetrates into the soil during a day. Actual light mosaics in the soil depend on numerous factors and it changes with the depth (Woolley and Stoller, 1978; Tester and Morris, 1987; Kasperbauer and Hunt, 1988). (B) Root apex zonation with respect of light-sensitivity. Root cap, meristem and transition zone are expressing phytochromes (Adam et al., 1994; Somers and Quail, 1995a,b; Goosey et al., 1997) whereas only the transition zone is abundantly expressing phototropin phot1 (Wan et al., 2008, 2012). UVR8 is expressed, similarly as phytochromes, in all zones of Arabidopsis root apex (Rizzini et al., 2011; Yokawa et al., 2014). RUS1 and RUS2 are also expressed preferentially in cells of the transition zone (Leasure et al., 2009; Yu et al., 2013). (C) Tissue-specific and polar distribution of phot1 in cells of the transition zone. While epidermis cells do not express phot1, this blue light photoreceptor essential for negative phototropism of roots is abundant and polarly distributed (shown in blue) in underlying cortex cells (Wan et al., 2008) and controls PIN2 distribution and recycling (Wan et al., 2012). This tissue-specific expression and polarity of phot1 fits nicely to the plant “ocelli” concept (the epidermis act as lens-like tissue and the sub-epidermis as retina-like tissue) as proposed by Haberland for shoots (Haberlandt, 1904; Darwin, 1907; von Guttenberg, 1955).
Plants and their roots actively sense light and analyze both the spectrum and intensity of light using several photoreceptors, to integrate the development of organs growing aboveground and underground. In this review, we will discuss the spatial distribution of these photoreceptors and the physiological responses of roots to different light signals. It complements the more general reviews published recently (Kutschera and Briggs, 2012; Goyal et al., 2013; Briggs, 2014; Liscum et al., 2014; Fankhauser and Christie, 2015).
Phytochromes
The plant sensory photoreceptor, phytochrome, was first discovered in the 1960s (Siegelman and Hendricks, 1964). Five members of this red/far-red photoreceptor family, phyA-phyE, are encoded by the nuclear genome in Arabidopsis thaliana (Briggs and Olney, 2001). All phytochromes use a single chromophore, phytochromobilin, to sense light signals (Lamparter, 2004). Phytochromes have two spectrally distinct conformations. The Pr form has a maximum absorption wavelength in the red spectrum (max = 660 nm), while Pfr absorbs far-red light (max = 730 nm) and these two conformations are photoconvertible. For example, quantification of seed germination rate under special light conditions revealed that Pfr is the active form initiating the germination of plants (Shinomura et al., 1994).
Schwarz and Schneider (1987) reported that phytochromes accumulated in the coleoptile tip, shoot apex and the root cap of Zea mays, while other root regions almost lack expression of phytochromes. Seven years later, expression of reporter genes driven by endogenous promoters was reported for phytochromes in different model plants. Adam et al. (1994) reported that the PHYA gene was mainly expressed in the root meristem and root cap in both light- and dark-grown Nicotiana tabacum seedlings. Later, Somers and Quail (1995a,b) agreed with this expression pattern of PHYA in A. thaliana, with additional PHYA expression in initiation sites of lateral roots. They further found that the expression of PHYB genes in meristem and root cap was induced by light illumination (Somers and Quail, 1995a,b). In addition, light stimulation of the dark-grown Arabidopsis seedlings induced the PHYD expression in whole roots with high expression rate in the root elongation and transition zones, but not in the root apical meristem and root cap (Goosey et al., 1997).
Phytochromes mediate variable physiological processes in roots of Arabidopsis seedlings, including promotion of root elongation, formation of lateral roots and mediation of root phototropic responses (Takano et al., 2001; Kiss et al., 2003a; Costigan et al., 2011; Raya-Gonzalez et al., 2014). PhyA and phyB act as active photoreceptors leading to red light-induced positive root phototropism (Kiss et al., 2003a). PhyA inhibits the blue light-induced negative root phototropism in Arabidopsis (Kiss et al., 2003b). Later, Correll and Kiss (2005) reported that both phyA and phyB have roles in light-stimulated root elongation, which is related to the root gravitropism. Hopkins and Kiss (2012) used mutant lines lacking PHY chromophore in shoots (CAB3::pBVR) and roots (M0062/UASBVR) to determine that the root growth was directly affected by the light sensing in roots. The signaling systems mediated by phys provide a mechanism to balance and integrate the development of shoots and roots (Salisbury et al., 2007). Interestingly, light signals sensed by the root apices influence also the shoot gravitropic bending (Hopkins and Kiss, 2012).
Cryptochromes
Cryptochromes (CRYs) were discovered as a blue light photoreceptor in plants in the 1990s. CRYs are nuclear flavoproteins, with homology to photolyases, which exist in almost all organisms (Thompson and Sancar, 2002; Chaves et al., 2006; Ikeda et al., 2011; Christie et al., 2015). The model plant Arabidopsis has two members, cry1 and cry2 (Christie et al., 2015), which mediate inhibition of hypocotyl elongation under blue light, floral initiation controlled by circadian rhythms and other blue light-induced processes (Li and Yang, 2007; Yu et al., 2010). Crys have similar structures with two functional domains, the N-terminal PHR (photolyase-homologous region) domain that binds the chromophore FAD (flavin adenine dinucleotide) and a CCE (CRY C-terminal extension) domain at the C-terminal (Yu et al., 2010). Blue light changes the protein conformation by altering phosphorylation status, adjusting the interaction with protein partners, such as CIB1 (CRYPTOCHROME-INTERACTING bHLH1), COP1 (CONSTITUTIVELY PHOTOMORPHOGENIC 1), and SPAs (SUPPRESSOR OF PHYA), to activate the cry signaling pathways (Yu et al., 2007, 2010). cry3, or CRY-dash, is another putative member of the cry family, but its physiological roles are still unclear (Brudler et al., 2003; Huang et al., 2006).
Expression of CRY1 and CRY2 were detected in Arabidopsis roots at the transcriptional and post-transcriptional levels. It appears that CRYs affect root elongation via indirect pathways. The perception site of blue light is within the shoot, affecting root elongation by inhibiting rootward auxin transport (Canamero et al., 2006; Mao et al., 2014). However, more direct impacts of CRYs on root growth were also reported (Zeng et al., 2010a,b). Both cry1 and cry2 inhibit root growth, lower levels of free auxin and PIN1 amount, and increase flavonoids (Zeng et al., 2010b). Over-expression of CRY1 and CRY2 inhibit root growth and make it less sensitive toward auxin transport inhibitor NPA (Zeng et al., 2010a). In addition, cry1 restrains lateral root formation via inhibiting polar auxin transport (Zeng et al., 2010b). Interestingly, the blue light induced development of chloroplasts in root is synergistically mediated by crys and phys (Usami et al., 2004). Light signals are transduced via auxin/cytokinin signaling pathways to modify transcriptional factors LONG HYPOCOTYL5 (HY5) and GOLDEN2-LIKE2 (GLK2), initiating root greening processes (Kobayashi et al., 2012).
Phototropins
Phototropins are blue light photoreceptors mediating dynamic plant behaviors, including shoot and root phototropism, chloroplast relocalization, adjustment of stomatal opening and expansion of cotyledons (Christie, 2007). The phototropin family has two members, phot1 and phot2. Both phots have similar structures and molecular mechanisms that sense blue light. They have two N-terminal LOV (light, oxygen, voltage) domains, which bind to flavin mononucleotide (FMN; Christie et al., 1999). The LOV domains are activated under illumination by forming a covalent bond between the cysteine residue and FMNs (Harper et al., 2003). The C-terminal kinase activities are released by activation of the LOV2 domain, causing self-phosphorylation or/and cross-phosphorylation of phototropins (Kaiserli et al., 2009). In addition to phototropin itself, several substrates of phot1-kinase were published in recent years. Among them, the ATP-BINDING CASSETTE B19 (ABCB19) is an auxin efflux transporter that can adjust the phototropic responses by maintaining the polar auxin transport in hypocotyls (Christie et al., 2011). The PHYTOCHROME KINASE SUBSTRATE 4 (PKS4) may have roles in adjusting phototropin- and phytochrome-mediated responses (Demarsy et al., 2012). However, PKS4 has only limited roles in the phototropic signaling process. BLUE LIGHT SIGNALING1 (BLUS1) is another known phosphorylation substrate of phots, mediating blue light-induced stomata opening of Arabidopsis (Takemiya et al., 2013). Besides these signaling proteins, phots need variable interaction proteins to mediate blue light signaling, including the NPH3 protein family (NPH3/RPT2/CPT1; Motchoulski and Liscum, 1999; Inada et al., 2004; Haga et al., 2005; Pedmale and Liscum, 2007), 14-3-3 proteins and small G-protein ARF proteins (Sullivan et al., 2009; Tseng et al., 2012). Tissue specific expression of phots provides another mechanism (see Figure 1C, for the root apex) to mediate a wide range of physiological processes (Sakamoto and Briggs, 2002; Wan et al., 2008; Preuten et al., 2013).
In mature Arabidopsis root system, phot1 is strongly expressed in the upper (closer to the soil surface) roots, where blue light reaches (Figure 1A), to increase drought tolerance in roots (Galen et al., 2007a,b). Moreover, mutant roots lacking phot1 showed random growth whereas control roots with active phot1 enjoyed directional and efficient growth (Galen et al., 2007a). In Arabidopsis root apices, phot1 accumulates in the apical region of primary and lateral roots, mediating blue light induced negative bending in primary roots and suppressing lateral root elongation (Sakamoto and Briggs, 2002; Wan et al., 2008; Zhang et al., 2013; Moni et al., 2015). Compared to the important roles of phot1 in roots, phot2 has only very weak distribution in root tissues (Kong et al., 2006; Wan et al., 2008). Interestingly, Arabidopsis root caps (Figures 1B,C) do not express phot1 (Sakamoto and Briggs, 2002; Wan et al., 2008), implying that the site of blue light perception is the root transition zone, adjusting root bending by controlling polar auxin transport (Figure 1: Wan et al., 2012; Zhang et al., 2013).
Root Phototropism via Light-Activated Root Photoreceptors: Root Cap Versus Transition Zone
Both root and light tropisms have attracted the attention of researchers since a long time (Darwin, 1907; Kutschera and Briggs, 2012; Briggs, 2014). According to a classic study to quantify root phototropic behaviors in 166 plant species under unilateral white light illumination, 88 species showed no phototropic bending, while 72 had negative and 8 had positive phototropic bending responses (Schaefer, 1911). Naundorf (1940) reported that the root caps of sunflower seedlings are responsible for negative phototropic bending in roots. However, Schneider suggested that removing the apical 1 mm of the roots of maize plants did not result in altered phototropic bending behavior (Schneider, 1964). Mullen et al. (2002) designed a computer feedback system to maintain the root tip in a vertical position, and a fine optic fiber to control the exact light perception site of maize. They confirmed that the root cap is the organ of blue light perception in maize (Mullen et al., 2002).
Interestingly, the phot1-GFP protein driven by an endogenous promoter is not expressed in the root cap in Arabidopsis, but has a high expression level in the apical part of the transition zone (Figure 1C; Sakamoto and Briggs, 2002; Wan et al., 2008). The root cap, a site of perception of gravity signals (Swarup et al., 2005; Leitz et al., 2009), is rather a red-light-sensing organ as PHYA and PHYB are expressed there (Figures 1B,C and 2; Adam et al., 1994; Somers and Quail, 1995a,b; Goosey et al., 1997). Therefore, the critical question is this: is the root cap also a blue light-sensing organ? Is the root cap an organ for interaction between gravity and light signals to determine tropic bending?
The classic Cholodny–Went theory postulates that the asymmetric distribution of auxin determines both gravitropic and phototropic bending (Went and Thimann, 1937). It is logical to presume that phototropic and gravitropic signaling interacts via polar auxin transport in roots. The sensing of and response to tropic signals are spatially separated. In the maize root apex, the bending position responses to light and gravity signals have been determined, and gravitropic bending occurs at the transition zone (Baluška et al., 1996, 2010; Verbelen et al., 2006), also known as the distal elongation zone or the oscillatory zone (Baluška and Mancuso, 2013); whereas phototropic bending occurs above it, at the central elongation zone (Ishikawa and Evans, 1993; Mullen et al., 2002). The sensing of gravity occurs in the root caps. Re-orientation of roots causes sedimentation statoliths in the cortical endoplasmic reticulum to reorient to the new bottom in central S2 columella cells (Leitz et al., 2009). The dynamic force triggers release of Ca2+ from the endoplasmic reticulum, resulting in redistribution of PIN3 protein, an auxin efflux carrier, in these columella cells (Friml et al., 2002). However, the critical photoreceptor for blue light-induced root phototropism, phot1, is not expressed in root cap cells. Lateral blue light affects PIN3 localization in root cap columella cells, while the phot1 mutant lacks this response (Zhang et al., 2013). How the phot1 sensing of blue light adjusts localization of PIN3 in columella cells is still unclear.
Furthermore, Wan et al. (2012) reported that activated phot1 determines the cellular behavior of PIN2 via the NPH3-based signal transduction process, affecting polar auxin transport in cortical cells of the root apex transition zone. Interestingly, PIN2 and phot1 are expressed preferentially in the transition zone (Figure 2), special root apex zone in which sensory-response integration is accomplished for root gravitropism and phototropism (Baluška et al., 2001, 2010; Wan et al., 2012; Baluška and Mancuso, 2013). Phot1-mediated signaling determines polar localization of PIN2 and PIN3, resulting in asymmetric auxin distribution on the shaded and lighted sides, leading to negative root phototropism. When roots were illuminated by symmetric blue light illumination, root gravitropic bending was reduced (Wan et al., 2012). Adjustment of the polar localization of PINs is a crucial process in polar auxin transport and PINOID (PID) kinase is one of the key regulators (Kleine-Vehn et al., 2009; Sukumar et al., 2009). Phot1 and PID belong to the same AGC kinase group, with a close evolutionary relationship (Galván-Ampudia and Offringa, 2007; Robert and Offringa, 2008). PID acts as a negative regulator of root apex phototropism and is expressed in the transition zone (Haga and Sakai, 2015). Intriguingly, blue light irradiation changed the symmetric PID distribution into an asymmetric one, with reduced PID on the shaded root apex side (Haga and Sakai, 2015). Thus, these results suggest that phototropic and gravitropic signaling may share a similar regulatory mechanism via a PIN-based auxin transport through the root apical zones.
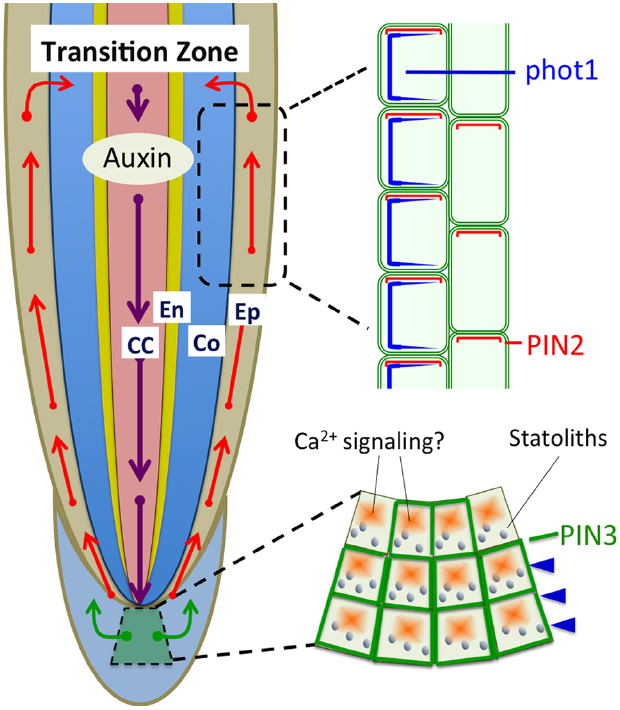
Figure 2. Polar auxin transport based on PIN1, PIN2, and PIN3 is light sensitive and involved in the light-induced negative phototropism of roots (Friml et al., 2002; Wan et al., 2012; Zhang et al., 2014). PIN1 is involved in the acropetal (rootward) auxin transport, PIN3 in the lateral auxin transport in statocytes, and PIN2 in the basipetal (shootward) auxin transport in epidermis and cortex cells. CC, central cylinder; En, endodermis; Co, cortex; Ep, epidermis.
Recently, PIN1 has also been shown to be needed for the root negative phototropism (Zhang et al., 2014). Blue light induced redistribution of both PIN2 (Wan et al., 2012), and PIN1 (Zhang et al., 2014) is mediated via BFA and GNOM-dependent endosomal trafficking pathways. Moreover, PINOID and PP2A are involved in the blue light induced redistribution of PIN1. Since PINOID is not expressed in stele cells expressing PIN1 (Dhonukshe et al., 2010; Zhang et al., 2014), it remains a mystery how PINOID controls the status of PIN1 protein.
Phys, the red light receptors, affect tropic bending via different mechanisms. Lariguet and Fankhauser (2004) revealed that top blue light illumination inhibited hypocotyl gravitropism through phyA-mediated pathways, while phot1 rescued upward growth by promoting phototropism. PhyA and phyB were found to have roles in adjusting root elongation and root gravitropism (Correll and Kiss, 2005). The phytochrome interaction protein, PKS1 acts as a signal transducer to inhibit gravitropic bending and adjust blue light-induced phototropism in Arabidopsis roots (Boccalandro et al., 2007). PhyA may mediate blue light responses by forming a signal complex with phot1 (Jaedicke et al., 2012). Interestingly, PKS1 is an interaction partner with phot1 and PKS4 is the substrate of phot1 kinase (Lariguet et al., 2006; Demarsy et al., 2012). Current structure analysis of the signaling protein complex may reveal the molecular mechanisms of phots, phys and PKSs interactions to reveal the mechanism of root phototropism and gravitropism.
Light Spectra and Root Apex Functional Zones
Sunlight can penetrate the soil for centimeters. The spectrum and intensity of light underneath the soil surface can be sensed by roots, with diverse photoreceptors distributed specifically in different root zones. In addition to the phytochromes, CRYs and phototropin photoreceptors, studies showed that UV-B light affects root growth and development. Root development of UV-B sensitive1 (rus1, rus2) mutants of Arabidopsis is blocked under weak UV-B illumination (Tong et al., 2008; Leasure et al., 2009). The RUS1 protein is an essential factor for polar auxin transport in Arabidopsis (Yu et al., 2013). A UV-B receptor, UVR8, was identified and its crystal structure characterized (Christie et al., 2012). UVR8 is expressed in root apices of Arabidopsis (Rizzini et al., 2011; Yokawa et al., 2014) and its over-expression reduces growth of illuminated roots (Fasano et al., 2014).
Another protein, the F-box protein ZEITLUPE, uses the LOV domain to sense light, modulating circadian rhythms and mediating hypocotyl elongation under light conditions (Kim et al., 2007). However, we have little knowledge of these photoreceptors and their sensing mechanisms, especially in roots. The root apical region may act as a site to sense and respond properly to light signals of different intensities and wavelengths. Root caps express both phyA and phyB, allowing them to act as a sensing organ for red and blue light (Figure 1B). The root apex transition zone acts as a command center for interactions between sensory and endogenous signals (Baluška et al., 2010; Baluška and Mancuso, 2013). Polar localization of phot1 in the transition zone (Figure 1C) provides a fine adjustment mechanism for auxin polar transport in the root apex, influencing phototropism and gravitropism at the root apex. Since blue light cannot penetrate deep beneath the soil surface (Figure 1A), blue and red light receptors are more highly expressed in the upper portion of roots, adjusting formation and initiation of lateral roots to better cope with drought stress, and initiation development of chloroplast in roots.
In conclusion, plant roots can sense light and respond to a colorful underground world via complex signaling networks constructed from interwoven signaling pathways based on plant-specific photoreceptors. It is important to maintain the roots of laboratory-grown Arabidopsis seedlings in darkened Petri dishes (Yokawa et al., 2011, 2014; Xu et al., 2013: Novák et al., 2015). Illumination of roots affects not only roots but changes the whole seedlings, their metabolism, physiology and perhaps also their circadian rhythms.
Conflict of Interest Statement
The authors declare that the research was conducted in the absence of any commercial or financial relationships that could be construed as a potential conflict of interest.
Acknowledgments
This project was supported by the Fundamental Research Funds for the Central Universities (JC2013-2), the 111 Project (B13007), Program for Changjiang Scholars and Innovative Research Team in University (IRT13047), Program for New Century Excellent Talents in University (NCET-12-0785), and the National Natural Science Foundation of China (31271433). KY was supported by the JSPS (Japanese Society for the Promotion of Science) Postdoctoral Fellowship. This work was supported in part by JSPS KAKENHI, Grant-in-Aid for JSPS fellows, No. 261654.
References
Adam, E., Szell, M., Szekeres, M., Schaefer, E., and Nagy, F. (1994). The developmental and tissue-specific expression of tobacco phytochrome-A genes. Plant J. 6, 283–293.
Baluška, F., Volkmann, D., and Barlow, P. W. (1996). Specialized zones of development in roots: view from the cellular level. Plant Physiol. 112, 3–4.
Baluška, F., Volkmann, D., and Barlow, P. W. (2001). A polarity crossroad in the transition growth zone of maize root apices: cytoskeletal and developmental implications. J. Plant Growth Regul. 20, 170–181. doi: 10.1007/s003440010013
Baluška, F., and Mancuso, S. (2013). Root apex transition zone as oscillatory zone. Front. Plant Sci. 4:354. doi: 10.3389/fpls.2013.00354
Baluška, F., Mancuso, S., Volkmann, D., and Barlow, P. W. (2010). Root apex transition zone: a signalling-response nexus in the root. Trends Plant Sci. 15, 402–408. doi: 10.3389/fpls.2013.00354
Boccalandro, H. E., De Simone, S. N., Bergmann-Honsberger, A., Schepens, I., Fankhauser, C., and Casal, J. J. (2007). PHYTOCHROME KINASE SUBSTRATE1 regulates root phototropism and gravitropism. Plant Physiol. 146, 108–115. doi: 10.1104/pp.107.106468
Briggs, W. R. (2014). Phototropism: some history, some puzzles, and a look ahead. Plant Physiol. 164, 13–23. doi: 10.1104/pp.113.230573
Briggs, W. R., and Lin, C. T. (2012). Photomorphogenesis—from one photoreceptor to 14: 40 years of progress. Mol. Plant 5, 531–532. doi: 10.1093/mp/sss059
Briggs, W. R., and Mandoli, D. F. (1984). Fiber optics in plants. Sci. Am. 251, 90–98. doi: 10.1038/scientificamerican0884-90
Briggs, W. R., and Olney, M. A. (2001). Photoreceptors in plant photomorphogenesis to date. Five phytochromes, two cryptochromes, one phototropin, and one superchrome. Plant Physiol. 125, 85–88. doi: 10.1104/pp.125.1.85
Brudler, R., Hitomi, K., Daiyasu, H., Toh, H., Kucho, K., Ishiura, M., et al. (2003). Identification of a new cryptochrome class. structure, function, and evolution. Mol. Cell 11, 59–67. doi: 10.1016/S1097-2765(03)00008-X
Burbach, C., Markus, K., Zhang, Y., Schlicht, M., and Baluška, F. (2012). Photophobic behavior of maize roots. Plant Signal. Behav. 7, 874–878. doi: 10.4161/psb.21012
Canamero, R. C., Bakrim, N., Bouly, J. P., Garay, A., Dudkin, E. E., Habricot, Y., et al. (2006). Cryptochrome photoreceptors cry1 and cry2 antagonistically regulate primary root elongation in Arabidopsis thaliana. Planta 224, 995–1003. doi: 10.1007/s00425-006-0280-6
Chaves, I., Yagita, K., Barnhoorn, S., Okamura, H., van der Horst, G. T., and Tamanini, F. (2006). Functional evolution of the photolyase/cryptochrome protein family: importance of the C terminus of mammalian CRY1 for circadian core oscillator performance. Mol. Cell. Biol. 26, 1743–1753. doi: 10.1128/MCB.26.5.1743-1753.2006
Christie, J. M. (2007). Phototropin blue-light receptors. Annu. Rev. Plant Biol. 58, 21–45. doi: 10.1146/annurev.arplant.58.032806.103951
Christie, J. M., Arvai, A. S., Baxter, K. J., Heilmann, M., Pratt, A. J., O’Hara, A., et al. (2012). Plant UVR8 photoreceptor senses UV-B by tryptophan-mediated disruption of cross-dimer salt bridges. Science 335, 1492–1496. doi: 10.1126/science.1218091
Christie, J. M., Blackwood, L., Petersen, J., and Sullivan, S. (2015). Plant flavoprotein photoreceptors. Plant Cell Physiol. 56, 401–413. doi: 10.1093/pcp/pcu196
Christie, J. M., Salomon, M., Nozue, K., Wada, M., and Briggs, W. R. (1999). LOV (light, oxygen, or voltage) domains of the blue-light photoreceptor phototropin (nph1): binding sites for the chromophore flavin mononucleotide. Proc. Natl. Acad. Sci. U.S.A. 96, 8779–8783. doi: 10.1073/pnas.96.15.8779
Christie, J. M., Yang, H., Richter, G. L., Sullivan, S., Thomson, C. E., Lin, J., et al. (2011). phot1 inhibition of ABCB19 primes lateral auxin fluxes in the shoot apex required for phototropism. PLoS Biol. 9:e1001076. doi: 10.1371/journal.pbio.1001076
Correll, M. J., and Kiss, J. Z. (2005). The roles of phytochromes in elongation and gravitropism of roots. Plant Cell Physiol. 46, 317–323. doi: 10.1093/pcp/pci038
Costigan, S. E., Warnasooriya, S. N., Humphries, B. A., and Montgomery, B. L. (2011). Root-localized phytochrome chromophore synthesis is required for photoregulation of root elongation and impacts root sensitivity to jasmonic acid in Arabidopsis. Plant Physiol. 157, 1138–1150. doi: 10.1104/pp.111.184689
Darwin, F. (1907). Lectures on the physiology of movement in plants. V. The sense-organs for gravity and light. New Phytol. 6, 69–76. doi: 10.1111/j.1469-8137.1907.tb06045.x
Demarsy, E., Schepens, I., Okajima, K., Hersch, M., Bergmann, S., Christie, J., et al. (2012). Phytochrome kinase substrate 4 is phosphorylated by the phototropin 1 photoreceptor. EMBO J. 31, 3457–3467. doi: 10.1038/emboj.2012.186
Dhonukshe, P., Huang, F., Galvan-Ampudia, C. S., Mähönen, A. P., Kleine-Vehn, J., Xu, J., et al. (2010). Plasma membrane-bound AGC3 kinases phosphorylate PIN auxin carriers at TPRXS(N/S) motifs to direct apical PIN recycling. Development 137, 3245–3255. doi: 10.1242/dev.052456
Fankhauser, C., and Christie, J. M. (2015). Plant phototropic growth. Curr. Biol. 25, R384–R389. doi: 10.1016/j.cub.2015.03.020
Fasano, R., Gonzalez, N., Tosco, A., Piaz, F. D., Docimo, T., Serrano, R., et al. (2014). Role of Arabidopsis–UV RESISTANCE LOCUS 8 in plant growth reduction under osmotic stress and low levels of UV-B. Mol. Plant 5, 773–791. doi: 10.1093/mp/ssu002
Friml, J., Wisniewska, J., Benkova, E., Mendgen, K., and Palme, K. (2002). Lateral relocation of auxin efflux regulator PIN3 mediates tropism in Arabidopsis. Nature 415, 806–809. doi: 10.1038/415806a
Galen, C., Rabenold, J. J., and Liscum, E. (2007a). Functional ecology of a blue light photoreceptor: effects of phototropin-1 on root growth enhance drought tolerance in Arabidopsis thaliana. New Phytol. 173, 91–99. doi: 10.1111/j.1469-8137.2006.01893.x
Galen, C., Rabenold, J. J., and Liscum, E. (2007b). Light-sensing in roots. Plant Signal. Behav. 2, 106–108. doi: 10.4161/psb.2.2.3638
Galván-Ampudia, C. S., and Offringa, R. (2007). Plant evolution: AGC kinases tell the auxin tale. Trends Plant Sci. 12, 541–547. doi: 10.1016/j.tplants.2007.10.004
Goosey, L., Palecanda, L., and Sharrock, R. A. (1997). Differential patterns of expression of the Arabidopsis PHYB, PHYD, and PHYE phytochrome genes. Plant Physiol. 115, 959–969. doi: 10.1104/pp.115.3.959
Goyal, A., Szarzynska, B., and Fankhauser, C. (2013). Phototropism: at the crossroads of light-signaling pathways. Trends Plant Sci. 18, 393–401. doi: 10.1016/j.tplants.2013.03.002
Gundel, P. E., Pierik, R., Mommer, L., and Ballare, C. L. (2014). Competing neighbors: light perception and root function. Oecologia 176, 1–10. doi: 10.1007/s00442-014-2983-x
Haberlandt, G. (1904). Die Perception des Lichtreizes durch das Laubblatt. Ber. Deutsch. Bbot. Gesell. 22, 105–119.
Haga, K., and Sakai, T. (2015). PINOID functions in root phototropism as a negative regulator. Plant Signal. Behav. 10, e998545. doi: 10.1080/15592324.2014.998545
Haga, K., Takano, M., Neumann, R., and Iino, M. (2005). The Rice COLEOPTILE PHOTOTROPISM1 gene encoding an ortholog of Arabidopsis NPH3 is required for phototropism of coleoptiles and lateral translocation of auxin. Plant Cell 17, 103–115. doi: 10.1105/tpc.104.028357
Harper, S. M., Neil, L. C., and Gardner, K. H. (2003). Structural basis of a phototropin light switch. Science 301, 1541–1544. doi: 10.1126/science.1086810
Hopkins, J. A., and Kiss, J. Z. (2012). Phototropism and gravitropism in transgenic lines of Arabidopsis altered in the phytochrome pathway. Physiol. Plant 145, 461–473. doi: 10.1111/j.1399-3054.2012.01607.x
Huang, Y., Baxter, R., Smith, B. S., Partch, C. L., Colbert, C. L., and Deisenhofer, J. (2006). Crystal structure of cryptochrome 3 from Arabidopsis thaliana and its implications for photolyase activity. Proc. Natl. Acad. Sci. U.S.A. 103, 17701–17706. doi: 10.1073/pnas.0608554103
Ikeda, H., Fujii, N., and Setoguchi, H. (2011). Molecular evolution of cryptochrome genes and the evolutionary manner of photoreceptor genes in Cardamine nipponica (Brassicaceae). J. Plant Res. 124, 85–92. doi: 10.1007/s10265-010-0361-2
Inada, S., Ohgishi, S., Mayama, T., Okada, K., and Sakai, T. (2004). RPT2 Is a signal transducer involved in phototropic response and stomatal opening by association with phototropin 1 in Arabidopsis thaliana. Plant Cell 16, 887–896. doi: 10.1105/tpc.019901
Ishikawa, H., and Evans, M. L. (1993). The role of the distal elongation zone in the response of maize roots to auxin and gravity. Plant Physiol. 102, 1203–1210.
Jaedicke, K., Lichtenthaler, A. L., Meyberg, R., Zeidler, M., and Hughes, J. (2012). A phytochrome-phototropin light signaling complex at the plasma membrane. Proc. Natl. Acad. Sci. U.S.A. 109, 12231–12236. doi: 10.1073/pnas.1120203109
Kaiserli, E., Sullivan, S., Jones, M. A., Feeney, K. A., and Christie, J. M. (2009). Domain swapping to assess the mechanistic basis of Arabidopsis Phototropin 1 receptor kinase activation and endocytosis by blue light. Plant Cell 21, 3226–3244. doi: 10.1105/tpc.109.067876
Kasperbauer, M. J., and Hunt, P. G. (1988). Biological and photometric measurement of light transmission through soils of various colors. Bot. Gaz. 149, 361–364. doi: 10.1086/337726
Kim, W. Y., Fujiwara, S., Suh, S. S., Kim, J., Kim, Y., Han, L., et al. (2007). ZEITLUPE is a circadian photoreceptor stabilized by GIGANTEA in blue light. Nature 449, 356–360. doi: 10.1038/nature06132
Kircher, S., and Schopfer, P. (2012). Photosynthetic sucrose acts as cotyledon-derived long-distance signal to control root growth during early seedling development in Arabidopsis. Proc. Natl. Acad. Sci. U.S.A. 109, 11217–11221. doi: 10.1073/pnas.1203746109
Kiss, J. Z., Mullen, J. L., Correll, M. J., and Hangarter, R. P. (2003a). Phytochromes A and B mediate red-light-induced positive phototropism in roots. Plant Physiol. 131, 1411–1417. doi: 10.1104/pp.013847
Kiss, J. Z., Correll, M. J., Mullen, J. L., Hangarter, R. P., and Edelmann, R. E. (2003b). Root phototropism: how light and gravity interact in shaping plant form. Gravit. Space Biol. Bull. 16, 55–60.
Kleine-Vehn, J., Huang, F., Naramoto, S., Zhang, J., Michniewicz, M., Offringa, R., et al. (2009). PIN auxin efflux carrier polarity is regulated by PINOID kinase-mediated recruitment into GNOM-independent trafficking in Arabidopsis. Plant Cell 21, 3839–3849. doi: 10.1105/tpc.109.071639
Klemmer, R., and Schneider, H. A. W. (1979). On a blue light effect and phytochrome in the stimulation of georesponsiveness of maize roots. Zeitschr. Pflanzenphysiol. 95, 189–197. doi: 10.1016/S0044-328X(79)80233-0
Kobayashi, K., Baba, S., Obayashi, T., Sato, M., Toyooka, K., Keranen, M., et al. (2012). Regulation of root greening by light and auxin/cytokinin signaling in Arabidopsis. Plant Cell 24, 1081–1095. doi: 10.1105/tpc.111.092254
Kong, S. G., Suzuki, T., Tamura, K., Mochizuki, N., Hara-Nishimura, I., and Nagatani, A. (2006). Blue light-induced association of phototropin 2 with the Golgi apparatus. Plant J. 45, 994–1005. doi: 10.1111/j.1365-313X.2006.02667.x
Kutschera, U., and Briggs, W. R. (2012). Root phototropism: from dogma to the mechanism of blue light perception. Planta 235, 443–452. doi: 10.1007/s00425-012-1597-y
Lake, J. V., and Slack, G. (1961). Dependence on light of geotropism in plant roots. Nature 191, 300–302. doi: 10.1038/191300a0
Lamparter, T. (2004). Evolution of cyanobacterial and plant phytochromes. FEBS Lett. 573, 1–5. doi: 10.1016/j.febslet.2004.07.050
Lariguet, P., and Fankhauser, C. (2004). Hypocotyl growth orientation in blue light is determined by phytochrome A inhibition of gravitropism and phototropin promotion of phototropism. Plant J. 40, 826–834. doi: 10.1111/j.1365-313X.2004.02256.x
Lariguet, P., Schepens, I., Hodgson, D., Pedmale, U. V., Trevisan, M., and Kami, C. (2006). PHYTOCHROME KINASE SUBSTRATE 1 is a phototropin 1 binding protein required for phototropism. Proc. Natl. Acad. Sci. U.S.A. 103, 10134–10139. doi: 10.1073/pnas.0603799103
Leasure, C. D., Tong, H., Yuen, G., Hou, X., Sun, X., and He, Z. H. (2009). ROOT UV-B SENSITIVE2 acts with ROOT UV-B SENSITIVE1 in a root ultraviolet B-sensing pathway. Plant Physiol. 150, 1902–1915. doi: 10.1104/pp.109.139253
Leitz, G., Kang, B. H., Schoenwaelder, M. E. A., and Staehelin, L. A. (2009). Statolith sedimentation kinetics and force transduction to the cortical endoplasmic reticulum in gravity-sensing Arabidopsis columella cells. Plant Cell 21, 843–860. doi: 10.1105/tpc.108.065052
Li, Q. H., and Yang, H. Q. (2007). Cryptochrome signaling in plants. Photochem. Photobiol. 83, 94–101. doi: 10.1562/2006-02-28-IR-826
Liscum, E., Askinosie, S. K., Leuchtman, D. L., Morrow, J., Willenburg, K. T., and Coats, D. R. (2014). Phototropism: growing towards an understanding of plant movement. Plant Cell 26, 38–55. doi: 10.1105/tpc.113.119727
Mandoli, D. F., Ford, G. A., Waldron, L. J., Nemson, J. A., and Briggs, W. R. (1990). Some spectral properties of several soil types: implications for photomorphogenesis. Plant Cell Environ. 13, 287–294. doi: 10.1111/j.1365-3040.1990.tb01313.x
Mao, K., Jiang, L., Bo, W., Xu, F., and Wu, R. (2014). Cloning of the cryptochrome-encoding PeCRY1 gene from Populus euphratica and functional analysis in Arabidopsis. PLoS ONE 9:e115201. doi: 10.1371/journal.pone.0115201
Moni, A., Lee, A. Y., Briggs, W. R., Han, I. S., and Adams, W. (2015). The blue light receptor Phototropin 1 suppresses lateral root growth by controlling cell elongation. Plant Biol. 17, 34–40. doi: 10.1111/plb.12187
Motchoulski, A., and Liscum, E. (1999). Arabidopsis NPH3: a NPH1 photoreceptor-interacting protein essential for phototropism. Science 286, 961–964. doi: 10.1126/science.286.5441.961
Mullen, J. L., Wolverton, C., Ishikawa, H., Hangarter, R. P., and Evans, M. L. (2002). Spatial separation of light perception and growth response in maize root phototropism. Plant Cell Environ. 25, 1191–1196. doi: 10.1046/j.1365-3040.2002.00899.x
Naundorf, G. (1940). Untersuchungen über den phototropismus der Keimwurzel von Helianthus annuus. Planta 30, 639–663. doi: 10.1007/BF01917044
Novák, J., Černý, M., Pavlu, J., Zemánková, J., Skalák, J., Plačková, L., et al. (2015). Roles of proteome dynamics and cytokinin signaling in root to hypocotyl ratio changes induced by shading roots of Arabidopsis seedlings. Plant Cell Physiol. 56, 1006–1018. doi: 10.1093/pcp/pcv026
Pedmale, U. V., and Liscum, E. (2007). Regulation of phototropic signaling in Arabidopsis via phosphorylation state changes in the phototropin 1-interacting protein NPH3. J. Biol. Chem. 282, 19992–20001. doi: 10.1074/jbc.M702551200
Preuten, T., Hohm, T., Bergmann, S., and Fankhauser, C. (2013). Defining the site of light perception and initiation of phototropism in Arabidopsis. Curr. Biol. 23, 1934–1938. doi: 10.1016/j.cub.2013.07.079
Raya-Gonzalez, J., Ortiz-Castro, R., Ruiz-Herrera, L. F., Kazan, K., and Lopez-Bucio, J. (2014). PHYTOCHROME AND FLOWERING TIME1/MEDIATOR25 regulates lateral root formation via auxin signaling in Arabidopsis. Plant Physiol. 165, 880–894. doi: 10.1104/pp.114.239806
Rellán-Álvarez, R., Lobet, G., Lindner, H., Pradier, P. M., Sebastian, J., Yee, M. C., et al. (2015). GLO-Roots: an imaging platform enabling multidimensional characterization of soil-grown roots systems. Elife. doi: 10.7554/eLife.07597 [Epub ahead of print].
Rizzini, L., Favory, J. J., Cloix, C., Faggionato, D., O’Hara, A., Kaiserli, E., et al. (2011). Perception of UV-B by the Arabidopsis UVR8 protein. Science 332, 103–106. doi: 10.1126/science.1200660
Robert, H. S., and Offringa, R. (2008). Regulation of auxin transport polarity by AGC kinases. Curr. Opin. Plant Biol. 11, 495–502. doi: 10.1016/j.pbi.2008.06.004
Roycewicz, P., and Malamy, J. E. (2012). Dissecting the effects of nitrate, sucrose and osmotic potential on Arabidopsis root and shoot system growth in laboratory assays. Philos. Trans. R. Soc. Lond. B Biol. Sci. 367, 1489–1500. doi: 10.1098/rstb.2011.0230
Sakamoto, K., and Briggs, W. R. (2002). Cellular and subcellular localization of phototropin 1. Plant Cell 14, 1723–1735. doi: 10.1105/tpc.003293
Salisbury, F. J., Hall, A., Grierson, C. S., and Halliday, K. J. (2007). Phytochrome coordinates Arabidopsis shoot and root development. Plant J. 50, 429–438. doi: 10.1111/j.1365-313X.2007.03059.x
Schneider, H. (1964). Kritische Versuche zum Problem des Phototropismus bei Wurzeln. Z. Bot. 52, 451–499.
Schwarz, H., and Schneider, H. A. (1987). Immunological assay of phytochrome in small sections of roots and other organs of maize (Zea mays L.) seedlings. Planta 170, 152–160. doi: 10.1007/BF00397883
Shinomura, T., Nagatani, A., Chory, J., and Furuya, M. (1994). The induction of seed germination in Arabidopsis thaliana is regulated principally by phytochrome B and secondarily by Phytochrome A. Plant Physiol. 104, 363–371.
Siegelman, H. W., and Hendricks, S. B. (1964). Phytochrome and its control of plant growth and development. Adv. Enzymol. Relat. Areas Mol. Biol. 26, 1–33. doi: 10.1002/9780470122716.ch1
Silva-Navas, J., Moreno-Risueno, M. A., Manzano, C., Pallero-Baena, M., Navarro-Neila, S., Téllez-Robledo, B., et al. (2015). D-Root: a system to cultivate plants with the root in darkness or under different light conditions. Plant J. doi: 10.1111/tpj.12998 [Epub ahead of print].
Singh, M., Gupta, A., and Laxmi, A. (2014a). Glucose control of root growth direction in Arabidopsis thaliana. J. Exp. Bot. 65, 2981–2993. doi: 10.1093/jxb/eru146
Singh, M., Gupta, A., and Laxmi, A. (2014b). Glucose and phytohormone interplay in controlling root directional growth in Arabidopsis. Plant Signal. Behav. 9, e29219. doi: 10.4161/psb.29219
Somers, D. E., and Quail, P. H. (1995a). Phytochrome-mediated light regulation of PHYA- and PHYB-GUS transgenes in Arabidopsis thaliana seedlings. Plant Physiol. 107, 523–534.
Somers, D. E., and Quail, P. H. (1995b). Temporal and spatial expression patterns of PHYA and PHYB genes in Arabidopsis. Plant J. 7, 413–427. doi: 10.1046/j.1365-313X.1995.7030413.x
Sukumar, P., Edwards, K. S., Rahman, A., DeLong, A., and Muday, G. K. (2009). PINOID kinase regulates root gravitropism through modulation of PIN2-dependent basipetal auxin transport in Arabidopsis. Plant Physiol. 150, 722–735. doi: 10.1104/pp.108.131607
Sullivan, S., Thomson, C. E., Kaiserli, E., and Christie, J. M. (2009). Interaction specificity of Arabidopsis 14-3-3 proteins with phototropin receptor kinases. FEBS Lett. 583, 2187–2193. doi: 10.1016/j.febslet.2009.06.011
Sun, Q., Yoda, K., and Suzuki, H. (2005). Internal axial light conduction in the stems and roots of herbaceous plants. J. Exp. Bot. 56, 191–203. doi: 10.1093/jxb/eri019
Sun, Q., Yoda, K., Suzuki, M., and Suzuki, H. (2003). Vascular tissue in the stem and roots of woody plants can conduct light. J. Exp. Bot. 54, 1627–1635. doi: 10.1093/jxb/erg167
Swarup, R., Kramer, E. M., Perry, P., Knox, K., Leyser, H. M. O., Haseloff, J., et al. (2005). Root gravitropism requires lateral root cap and epidermal cells for transport and response to a mobile auxin signal. Nat. Cell Biol. 7, 1057–1065. doi: 10.1038/ncb1316
Takano, M., Kanegae, H., Shinomura, T., Miyao, A., Hirochika, H., and Furuya, M. (2001). Isolation and characterization of rice phytochrome A mutants. Plant Cell 13, 521–534. doi: 10.1105/tpc.13.3.521
Takemiya, A., Sugiyama, N., Fujimoto, H., Tsutsumi, T., Yamauchi, S., Hiyama, A., et al. (2013). Phosphorylation of BLUS1 kinase by phototropins is a primary step in stomatal opening. Nat. Commun. 4, 3094. doi: 10.1038/ncomms3094
Tester, M., and Morris, C. (1987). The penetration of light through soil. Plant Cell Environ. 10, 281–286. doi: 10.1111/j.1365-3040.1987.tb01607.x
Thompson, C. L., and Sancar, A. (2002). Photolyase/cryptochrome blue-light photoreceptors use photon energy to repair DNA and reset the circadian clock. Oncogene 21, 9043–9056. doi: 10.1038/sj.onc.1205958
Tong, H., Leasure, C. D., Hou, X., Yuen, G., Briggs, W. R., and He, Z. H. (2008). Role of root UV-B sensing in Arabidopsis early seedling development. Proc. Natl. Acad. Sci. U.S.A. 106, 21039–21044. doi: 10.1073/pnas.0809942106
Tseng, T. S., Whippo, C., Hangarter, R. P., and Briggs, W. R. (2012). The Role of a 14-3-3 Protein in stomatal opening mediated by PHOT2 in Arabidopsis. Plant Cell 24, 1114–1126. doi: 10.1105/tpc.111.092130
Usami, T., Mochizuki, N., Kondo, M., Nishimura, M., and Nagatani, A. (2004). Cryptochromes and phytochromes synergistically regulate Arabidopsis root greening under blue light. Plant Cell Physiol. 45, 1798–1808. doi: 10.1093/pcp/pch205
Verbelen, J. P., De Cnodder, T., Le, J., Vissenberg, K., and Baluška, F. (2006). The root apex of Arabidopsis thaliana consists of four distinct zones of growth activities: meristematic zone, transition zone, fast elongation zone and growth terminating zone. Plant Signal. Behav. 1, 296–304. doi: 10.4161/psb.1.6.3511
Wan, Y. L., Eisinger, W., Ehrhardt, D., Kubitscheck, U., Baluška, F., and Briggs, W. (2008). The subcellular localization and blue-light-induced movement of phototropin 1-GFP in etiolated seedlings of Arabidopsis thaliana. Mol. Plant 1, 103–117. doi: 10.1093/mp/ssm011
Wan, Y., Jasik, J., Wang, L., Hao, H., Volkmann, D., Menzel, D., et al. (2012). The signal transducer NPH3 integrates the phototropin1 photosensor with PIN2-based polar auxin transport in Arabidopsis root phototropism. Plant Cell 24, 551–565. doi: 10.1105/tpc.111.094284
Woolley, J. T., and Stoller, E. W. (1978). Light penetration and light-induced seed germination in soil. Plant Physiol. 61, 597–600. doi: 10.1104/pp.61.4.597
Xu, W., Ding, G., Yokawa, K., Baluška, F., Li, Q.-F., Liu, Y., et al. (2013). An improved agar-plate method for studying root growth and response of Arabidopsis thaliana. Sci. Rep. 3:1273. doi: 10.1038/srep01273
Yokawa, K., Fasano, R., Kagenishi, T., and Baluška, F. (2014). Light as stress factor to plant roots—case of root halotropism. Front. Plant Sci. 5:718. doi: 10.3389/fpls.2014.00718
Yokawa, K., Kagenishi, T., and Baluška, F. (2013). Root photomorphogenesis in laboratory-maintained Arabidopsis seedlings. Trends Plant Sci. 18, 117–119. doi: 10.1016/j.tplants.2013.01.002
Yokawa, K., Kagenishi, T., Kawano, T., Mancuso, S., and Baluška, F. (2011). Illumination of Arabidopsis roots induces immediate burst of ROS production. Plant Signal. Behav. 6, 1460–1464. doi: 10.4161/psb.6.10.18165
Yu, H., Karampelias, M., Robert, S., Peer, W. A., Swarup, R., Ye, S., et al. (2013). ROOT ULTRAVIOLET B-SENSITIVE1/weak auxin response3 is essential for polar auxin transport in Arabidopsis. Plant Physiol. 162, 965–976. doi: 10.1104/pp.113.217018
Yu, X., Liu, H., Klejnot, J., and Lin, C. (2010). The cryptochrome blue light receptors. Arabidopsis Book 8, e0135. doi: 10.1199/tab.0135
Yu, X., Shalitin, D., Liu, X., Maymon, M., Klejnot, J., Yang, H., et al. (2007). Derepression of the NC80 motif is critical for the photoactivation of Arabidopsis CRY2. Proc. Natl. Acad. Sci. U.S.A. 104, 7289–7294. doi: 10.1073/pnas.0701912104
Zeng, J., Wang, Q., Lin, J., Deng, K., Tang, D., and Zhao, X. (2010a). The effects of cryptochrome photoreceptors on root growth in Arabidopsis. Afr. J. Biotechnol. 8, 3179–3183.
Zeng, J., Wang, Q., Lin, J., Deng, K., Zhao, X., Tang, D., et al. (2010b). Arabidopsis cryptochrome-1 restrains lateral roots growth by inhibiting auxin transport. J. Plant Physiol. 167, 670–673. doi: 10.1016/j.jplph.2009.12.003
Zhang, K. X., Xu, H. H., Gong, W., Jin, Y., Shi, Y. Y., Yuan, T. T., et al. (2014). Proper PIN1 distribution is needed for root negative phototropism in Arabidopsis. PLoS ONE 9:e85720. doi: 10.1371/journal.pone.0085720
Keywords: root, photomorphogenesis, photoreceptors, plant, phytohormones, phototropism, auxin
Citation: Mo M, Yokawa K, Wan Y and Baluška F (2015) How and why do root apices sense light under the soil surface? Front. Plant Sci. 6:775. doi: 10.3389/fpls.2015.00775
Received: 27 June 2015; Accepted: 10 September 2015;
Published: 24 September 2015.
Edited by:
Stefan De Folter, Centro de Investigación y de Estudios Avanzados del Instituto Politécnico Nacional, MexicoReviewed by:
Rubén Rellán-Álvarez, National Laboratory of Genomics for Biodiversity – Center for Research and Advanced Studies of the National Polytechnic Institute, MexicoJohn Christie, University of Glasgow, UK
Copyright © 2015 Mo, Yokawa, Wan and Baluška. This is an open-access article distributed under the terms of the Creative Commons Attribution License (CC BY). The use, distribution or reproduction in other forums is permitted, provided the original author(s) or licensor are credited and that the original publication in this journal is cited, in accordance with accepted academic practice. No use, distribution or reproduction is permitted which does not comply with these terms.
*Correspondence: Yinglang Wan, College of Biological Sciences and Biotechnology, Beijing Forestry University, Qinghua East Road No. 35, 100083 Beijing, China,eWx3YW5AYmpmdS5lZHUuY24=; František Baluška, Institute of Cellular and Molecular Botany, University of Bonn, Kirschallee 1, D-53115 Bonn, Germany,YmFsdXNrYUB1bmktYm9ubi5kZQ==