- 1Molekularbiologie der Pflanzen, Biozentrum der Ludwig-Maximilians-Universität München, Planegg-Martinsried, Germany
- 2Pflanzliche Entwicklungsbiologie, Biozentrum der Ludwig-Maximilians-Universität München, Planegg-Martinsried, Germany
The assembly and repair of photosystem II (PSII) is facilitated by a variety of assembly factors. Among those, the tetratricopeptide repeat (TPR) protein Slr0151 from Synechocystis sp. PCC 6803 (hereafter Synechocystis) has previously been assigned a repair function under high light conditions (Yang et al., 2014). Here, we show that inactivation of slr0151 affects thylakoid membrane ultrastructure even under normal light conditions. Moreover, the level and localization of Slr0151 are affected in a variety of PSII-related mutants. In particular, the data suggest a close functional relationship between Slr0151 and Sll0933, which interacts with Ycf48 during PSII assembly and is homologous to PAM68 in Arabidopsis thaliana. Immunofluorescence analysis revealed a punctate distribution of Slr0151 within several different membrane types in Synechocystis cells.
Introduction
The ability of plastid-bearing organisms to perform oxygenic photosynthesis was inherited from an ancient cyanobacterium about 2.4 billion years ago. In present-day cyanobacteria, the photosynthetic electron transport chain (PET) is embedded in an internal membrane system made up of thylakoids (Hohmann-Marriott and Blankenship, 2011). The PET is fueled by electrons originating from the water-splitting complex within PSII, which is therefore considered to be the heart of photosynthesis. Recently, the structural analysis of PSII has revealed detailed insights into the architecture and working mode of its Mn4CaO5 cluster, where water is oxidized and molecular oxygen is released (Umena et al., 2011; Kupitz et al., 2014; Suga et al., 2015).
Overall, PSII comprises at least 20 protein subunits as well as numerous organic and inorganic co-factors. All these components have to be assembled in a strictly coordinated manner in both time and space. The emerging picture indicates that the assembly process is initiated at specialized, biogenic thylakoid membrane (TM) regions and proceeds step-wise until the active PSII super-complex is formed as part of photosynthetically active thylakoids (Komenda et al., 2012; Nickelsen and Rengstl, 2013; Nickelsen and Zerges, 2013; Rast et al., 2015).
In the cyanobacterium Synechocystis sp. PCC 6803 (hereafter Synechocystis), the initial steps in de novo PSII assembly have been proposed to take place at biogenesis centers (BC) where the thylakoids converge on the plasma membrane (PM; van de Meene et al., 2006; Schottkowski et al., 2009a; Stengel et al., 2012; Nickelsen and Rengstl, 2013). The precise architecture of BCs is not yet fully understood; however, they are characterized by the accumulation of the PSII assembly factor PratA, which delivers Mn to the precursor of the D1 reaction-center protein (pD1; Stengel et al., 2012). The C-terminal extension of pD1 is then processed by the protease CtpA (Anbudurai et al., 1994; Zak et al., 2001; Komenda et al., 2007). Concomitantly, the first detectable PSII assembly intermediate, i.e., the reaction-center complex (RC), is formed by the attachment of the D2-Cyt b559 module which is aided by the assembly factor Ycf48, a homolog of Hcf136 from Arabidopsis thaliana (Komenda et al., 2004, 2008). Via the interaction of Ycf48 with the PAM68-homolog Sll0933, the inner core antenna proteins CP47 and CP43 bind successively to the RC complex, forming a PSII monomer that still lacks the lumenal subunits of the oxygen-evolving complex (OEC; Komenda et al., 2004; Rengstl et al., 2013). Finally, the OEC is built with the help of the assembly factors CyanoP and Psb27, yielding a fully functional PSII monomer (Nowaczyk et al., 2006; Becker et al., 2011; Komenda et al., 2012; Cormann et al., 2014).
Moreover, due to its susceptibility to photodamage, PSII needs to be repaired about every 30 min (Prasil et al., 1992; Mulo et al., 1998). In this process, PSII is disassembled by removing the PsbO, PsbV, PsbU, and CyanoQ subunits, followed by CP43 (Mulo et al., 2012; Mabbitt et al., 2014). Damaged D1 protein is then degraded by the FtsH2/H3 protease complex (Silva et al., 2003; Komenda et al., 2006; Boehm et al., 2012; Mabbitt et al., 2014) and replaced by newly synthesized D1, which is co-translationally inserted into the complex. Next, CP43 re-attaches and functional PSII is restored (Zhang et al., 1999; Komenda et al., 2008; Mulo et al., 2012; Mabbitt et al., 2014).
As outlined above, recent years have seen the discovery of many accessory factors that are involved in catalyzing distinct PSII assembly/repair steps. Many of these have been found to belong to the so-called family of TPR (tetratricopeptide repeat) proteins (Heinz et al., 2016; Rast et al., 2015). TPR proteins represent solenoid-like, “scaffold” proteins which are distributed throughout all kingdoms of life (for a recent review see Bohne et al., 2016). Typically, a TPR domain consists of multiple copies (3–16) of a degenerate motif which comprises 34 amino acids forming two amphipathic α-helices. The crystal structure of TPR domains revealed that these form right-handed superhelices that serve as a platform for protein–protein interactions (Blatch and Lässle, 1999; D’Andrea and Regan, 2003). TPR proteins have been implicated in a variety of functions during the biogenesis of TMs, including chloroplast protein import, gene expression and chlorophyll (Chl) synthesis, as well as PSII and PSI assembly (Bohne et al., 2016). In total, the Synechocystis genome encodes 29 TPR proteins (Bohne et al., 2016). These include Ycf3 and Ycf37, which have been shown to facilitate PSI assembly. The TPR protein Pitt (light-dependent protochlorophyllide oxidoreductase interacting TPR protein) interacts with POR (light-dependent protochlorophyllide oxidoreductase) and regulates Chl synthesis (Schottkowski et al., 2009b; Rengstl et al., 2011). For cyanobacterial PSII assembly, the above-mentioned TPR protein PratA plays an important role and recently the protein Slr0151 has been shown to be involved in the PSII repair cycle (Yang et al., 2014).
The slr0151 gene is part of the slr0144-slr0151 operon, which codes for eight proteins (Kopf et al., 2014; Yang et al., 2014). This operon was first discovered in the course of a microarray analysis in which expression of the cluster was down-regulated under iron-depleted conditions and during oxidative stress (Singh et al., 2004). The authors hypothesized that the gene cluster is involved in PSI assembly (Singh et al., 2004), and indeed a second study found Slr0151 to be associated with PSI complexes (Kubota et al., 2010). Others, however, have pointed to connections between Slr0151 and PSII (Wegener et al., 2008; Yang et al., 2014). Thus, Wegener et al. (2008) referred to the proteins encoded by the slr0144-slr0151 operon as PSII assembly proteins (Pap) because they stabilize PSII intermediates. Furthermore, these authors showed that the entire pap operon is up-regulated upon loss of any of the lumenal proteins CyanoP, PsbV, and CyanoQ (Wegener et al., 2008). In a slr0151- mutant, however, the expression of the other pap genes was not affected (Yang et al., 2014). In addition, experimental evidence has been provided that links Slr0151 to the PSII repair cycle, and yeast two-hybrid and pulldown analyses have revealed that Slr0151 interacts directly with both CP43 and D1 (Yang et al., 2014). In this study, we further characterize the function and subcellular localization of Slr0151.
Materials and Methods
Construction and Growth of Strains
Synechocystis wild-type and mutant strains were grown on solid or in liquid BG-11 medium at 30°C at a continuous photon irradiance of 30 μmol photons m-2 s-1. The insertion mutant slr0151- was generated by PCR amplification of the wild-type slr0151 gene with the oligonucleotides 0151/5 ATGATGGAAAATCAAGTTAATGA and 0151/3 TTAACCAAATAGGTTAGCTGC as primers, and subsequent cloning of the resulting fragment into the pDrive vector (Qiagen). The fragment was cut from pDrive and inserted into Bluescript pKS vector via the restriction enzymes SalI and PstI of both multiple cloning sites. A kanamycin-resistance cassette was then inserted into its unique HindIII restriction site, and wild-type cells were transformed with the construct as described. For complementation of the slr0151- mutant, the slr0151 gene (including its own promoter) was PCR-amplified with oligonucleotides 0151/5b CTCGAGTGATGAGTTTTTTTAGCTCTA and 0151/3b CTCGAGAACTGGAGTTTTAACCAAA, and cloned into the single XhoI site in the vector pVZ321, which replicates autonomously in Synechocystis 6803 (Zinchenko et al., 1999). Transfer of this construct into slr0151- via conjugation was performed as described (Zinchenko et al., 1999). Construction of the mutant lines psbA- (TD41), (Nixon et al., 1992), ctpA- (Rengstl et al., 2011), pratA- (Klinkert et al., 2004), psbB- (Eaton-Rye and Vermaas, 1991), ycf48- (Komenda et al., 2008), sll0933- (Armbruster et al., 2010), psb27- (Komenda et al., 2012), and pitt- (Schottkowski et al., 2009b) was described previously.
Antibody Production and Western Analysis
For production of the αSlr0151 antibody, the slr0151 reading frame without the N-terminal transmembrane region (amino acid positions 62 to 320) was PCR-amplified using oligonucleotides TH0151a GGATCCGAATTCCATTTGTTTAACCGTAAGCAGTT and TH0151b GTCGACTTAACCAAATAGGTTAGCTGCGGT. The resulting DNA fragment was inserted into the pDrive vector (Qiagen), sequenced and further subcloned into the BamHI and SalI restriction sites of the expression vector pGex-4T-1. Expression of the GST fusion protein in Escherichia coli BL21 and its affinity purification on Glutathione-Sepharose 4B (GE Healthcare) were performed according to the manufacturers’ instructions. Polyclonal antiserum was raised in rabbits (Biogenes). Protein preparation from Synechocystis 6803 and western analyses were carried out as previously reported (Wilde et al., 2001).
Isolation of Total Cell Protein, Membrane Fractionation, and Western Analysis
Isolation of whole-cell protein, two-step membrane fractionation via consecutive sucrose-density gradients, and western analyses were carried out as described previously (Wilde et al., 2001; Schottkowski et al., 2009a,b; Rengstl et al., 2011). Apart from αSlr0151, the primary antibodies used in this study have been described earlier, i.e., αD1 (Schottkowski et al., 2009b), αD2 (Klinkert et al., 2004), αPratA (Klinkert et al., 2004), αYcf48 (Rengstl et al., 2011), αPitt (Schottkowski et al., 2009a), αPOR (Schottkowski et al., 2009a), αYidC (Ossenbühl et al., 2006), αSll0933 (Armbruster et al., 2010), or were purchased from Agrisera (Vännäs, Sweden), i.e., αCP43, αCP47, αRbcL. Western blots were quantified using AIDA software (version 3.52.046). For each experiment, the respective RbcL signal served as internal loading control. Quantifications are based on at least three independent experiments. Mean and standard deviation were calculated for the protein levels and Student’s t-test was performed to verify statistical significant differences between wild-type and slr0151-.
Transmission Electron Microscopy
Synechocystis cells (slr0151- mutant and wild-type) were harvested in mid-log phase by centrifugation at 5000 g and adjusted to an OD750 nm = 3 in BG-11. Aliquots (2 μl) of the cell suspension were high-pressure frozen at 2100 bar (Leica HPM 100) in HPF gold platelets (Leica Microsystems, Vienna, Austria) and stored in liquid nitrogen (Rachel et al., 2010; Klingl et al., 2011). The cryofixed cells were then freeze-substituted (Leica EM AFS2) at -90°C with 2% osmium tetroxide and 0.2% uranyl acetate in pure acetone. Freeze substitution was carried out at -90°C for 20 h, -60°C for 8 h, -30°C for 8 h, with a heating time of 1 h between each step, and then held at 0°C for 3 h. Samples were washed three times with pure, ice-cold acetone followed by infiltration with Epon resin (Fluka, Buchs, Switzerland). After polymerization for 72 h at 63°C, ultrathin sections were cut, and post-stained with lead citrate (Reynolds, 1963). Transmission electron microscopy was carried out at 80 kV either on a Zeiss EM 912 or on a Fei Morgagni 268 electron microscope (FEI). Data analysis was carried out with the Fiji ImageJ software.
Immunofluorescence and Fluorescence Microscopy
Synechocystis cells were harvested in mid-log phase by centrifugation at 5000 g and adjusted to an OD750 nm = 3 in PBS (140 mM NaCl, 2.7 mM KCl, 10 mM Na2HPO4, 1.8 mM KH2PO4; pH 7.4). The cells were fixed with 2% formaldehyde (35%, for Histology, Roth) in PBS for 20 min at 30°C on a shaker, then washed twice with PBS-T (PBS supplemented with 0.05% Tween-20). For permeabilization, the cells were incubated with PBS-T for 2 min × 3 min on an overhead rotor. All subsequent steps were performed in the dark. The cells were applied to poly-L-lysine-coated glass slides (Sigma) and incubated for 30 min to allow them to settle, then incubated with blocking buffer (5% non-fat milk powder in PBS-T) for 20 min. The slides were incubated for 3 h with the first antibody (αSlr0151 and αRbcL, diluted 1:500 in blocking buffer, then washed for 3 min × 3 min by incubating them with PBS-T and rinsing the solution off the slide. The secondary HRP-conjugated goat anti-rabbit antibody (Sigma) was labeled with Alexa 488 (Alexa Fluor Dyes, Life Technologies, ThermoFisher Scientific) according the manufacturer’s instruction. The slides were incubated with the labeled secondary antibody (diluted 1:2000 in blocking buffer) for 1 h. The slides were washed twice with PBS-T and twice with PBS-G (PBS supplemented with 10 mM glycine) to minimize background fluorescence due to non-labeled fluorophores. The slides were then dried and each was covered with a drop of FluorSaveTM Reagent (Calbiochem, Merck Millipore) and a coverslip. Next day, the coverslip was sealed with nail polish. Fluorescence was imaged using a Delta Vision Elite (GE Healthcare, Applied Precision) equipped with Insight SSITM illumination and a CoolSNAP_HQ2 CCD camera. Cells were imaged with a 100× oil PSF U-Plan S-Apo 1.4 objective. The four-color standard set InsightSSI module (code number: 52-852113-003, GE Healthcare, Applied Precision) was used for imaging. Alexa488 was excited with the FITC/GFP excitation filter (461–489 nm) and emission was detected with the FITC/GFP emission filter (501–549 nm). Chlorophyll autofluorescence was excited with the TRITC/Cy3-filter (528–555 nm) and emission was detected with the TRITC/Cy3 filter (574–619 nm). Images were analyzed using the Fiji ImageJ software.
Results
Molecular slr0151- Phenotype
The slr0151 open reading frame in Synechocystis encodes a protein of 320 amino acids, which contains two consecutive TPR domains comprising positions 185–218 and 219–252 (analyzed with TPRpred; Yang et al., 2014; Bohne et al., 2016). It has previously been shown that Slr0151 is an intrinsic membrane protein which forms part of a high-molecular-weight complex (Yang et al., 2014).
To analyze the function of Slr0151, we disrupted its cloned reading frame by inserting a kanamycin-resistance cassette into the unique HindIII site 425 bp downstream of the start codon (Supplementary Figure S1A). After transformation of wild-type (WT) cells with this construct, the transformants were tested for complete segregation by PCR analysis (Supplementary Figure S1B). The complete absence of the Slr0151 protein in the slr0151- mutant was verified by western analysis using an αSlr0151 antibody (Supplementary Figure S1C). Like the previously described slr0151- mutant, the mutant strain described in this study exhibited a high light (800 μmol photons m-2 s-1) sensitive phenotype (Yang et al., 2014). Moreover, and also in agreement with the previous report, less pronounced effects were observed under normal lighting conditions (30 μmol photons m-2 s-1). These effects included moderately reduced photoautotrophic growth and oxygen production rates (Yang et al., 2014). Together, these findings suggest that, like other PSII assembly factors such as Ycf48 or Psb27, Slr0151 might be involved in both PSII assembly and repair (Komenda et al., 2012; Rengstl et al., 2013; Mabbitt et al., 2014; Jackson and Eaton-Rye, 2015).
To explore this possibility further, the levels of various photosynthetic subunits and PSII biogenesis factors accumulated in the slr0151- strain under normal growth conditions were analyzed. Almost all analyzed proteins showed no significant differences relative to WT, except CP43 and Pitt had increased levels in the mutant (Figure 1A; Supplementary Figure S2A). Conversely, however, levels of Slr0151 were significantly altered in different PSII assembly mutants (Figure 1B; Supplementary Figure S2B). In the ΔD1 mutant, in which all three copies of the psbA gene are inactivated, Slr0151 was reduced to only 20% of its wild-type level (Nixon et al., 1992). The ctpA- mutant lacking the C-terminal processing protease for pD1 accumulated only 50% as much Slr0151 as did wild-type cells (Anbudurai et al., 1994). In the PSII assembly factor mutants pratA-, ycf48-, and pitt- (Klinkert et al., 2004; Komenda et al., 2008; Schottkowski et al., 2009b) amounts of Slr0151 reached 52, 53, and 62% of the wild-type level, respectively. In sharp contrast, however, more than twice the WT level of Slr0151 was detected in sll0933-, which lacks the cyanobacterial homolog of the Arabidopsis PSII assembly factor PAM68. This suggests a functional relationship between Slr0151 and Sll0933, despite the fact that levels of the latter were unchanged in the slr0151- mutant (Figure 1; Supplementary Figure S2). To test if this relationship relies on a physical interaction of both factors, we performed co-immunoprecipitation experiments. However, no interaction between Slr0151 and Sll0933 were detected under the applied conditions suggesting that they do not form parts of a stable complex in vivo.
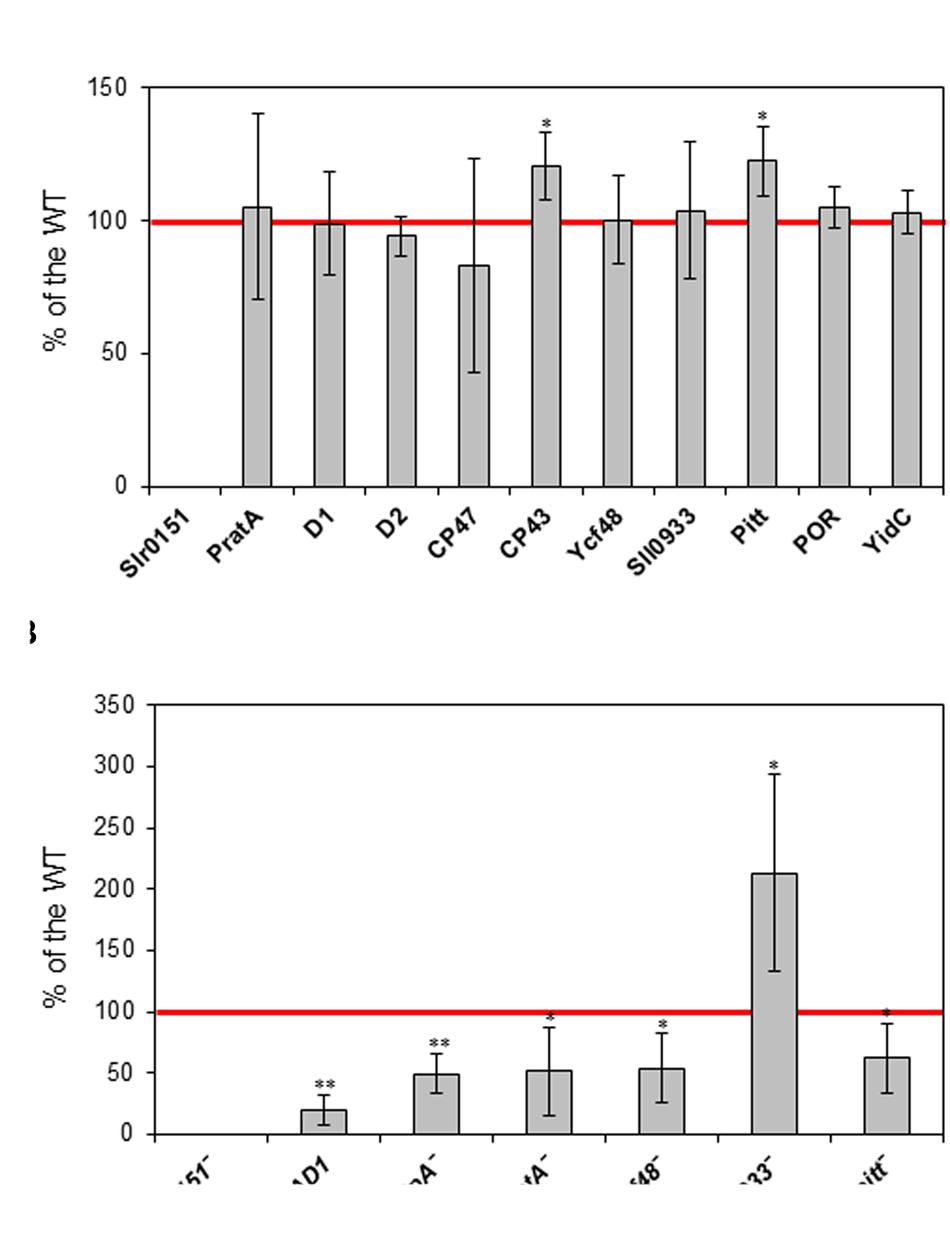
FIGURE 1. Steady-state levels of the indicated PSII-related proteins in the slr0151- mutant (A) and of Slr0151 in the indicated PSII mutants (B). Each value is expressed relative to that in the wild type. Total cell proteins were isolated, fractionated by SDS-PAGE, blotted onto nitrocellulose membranes and detected immunologically. Signals were quantified with AIDA software (version 3.52.046) after densitometrical scanning. Values plotted are means ± SD of at least three independent experiments. Significance according to Student’s t-test with an error probability of 5 and 1% is indicated by one and two asterisks, respectively.
Ultrastructure of slr0151- Cells
In order to gain more insight into the subcellular consequences of slr0151 inactivation, the ultrastructure of the mutant was visualized by transmission electron microscopy (Figure 2). In slr0151- cells grown at normal light intensities, the thylakoids appeared to be less densely packed and thylakoid lumina were swollen when compared to the wild-type (Figure 2). Lumen diameters ranged from 4 to 91 nm in the mutant, whereas the values for wild-type thylakoids fell within the 5- to 9-nm range, as previously observed (Figure 2; van de Meene et al., 2006). Thus, in addition to PSII assembly/repair, Slr0151 deficiency appears to affect TM organization.
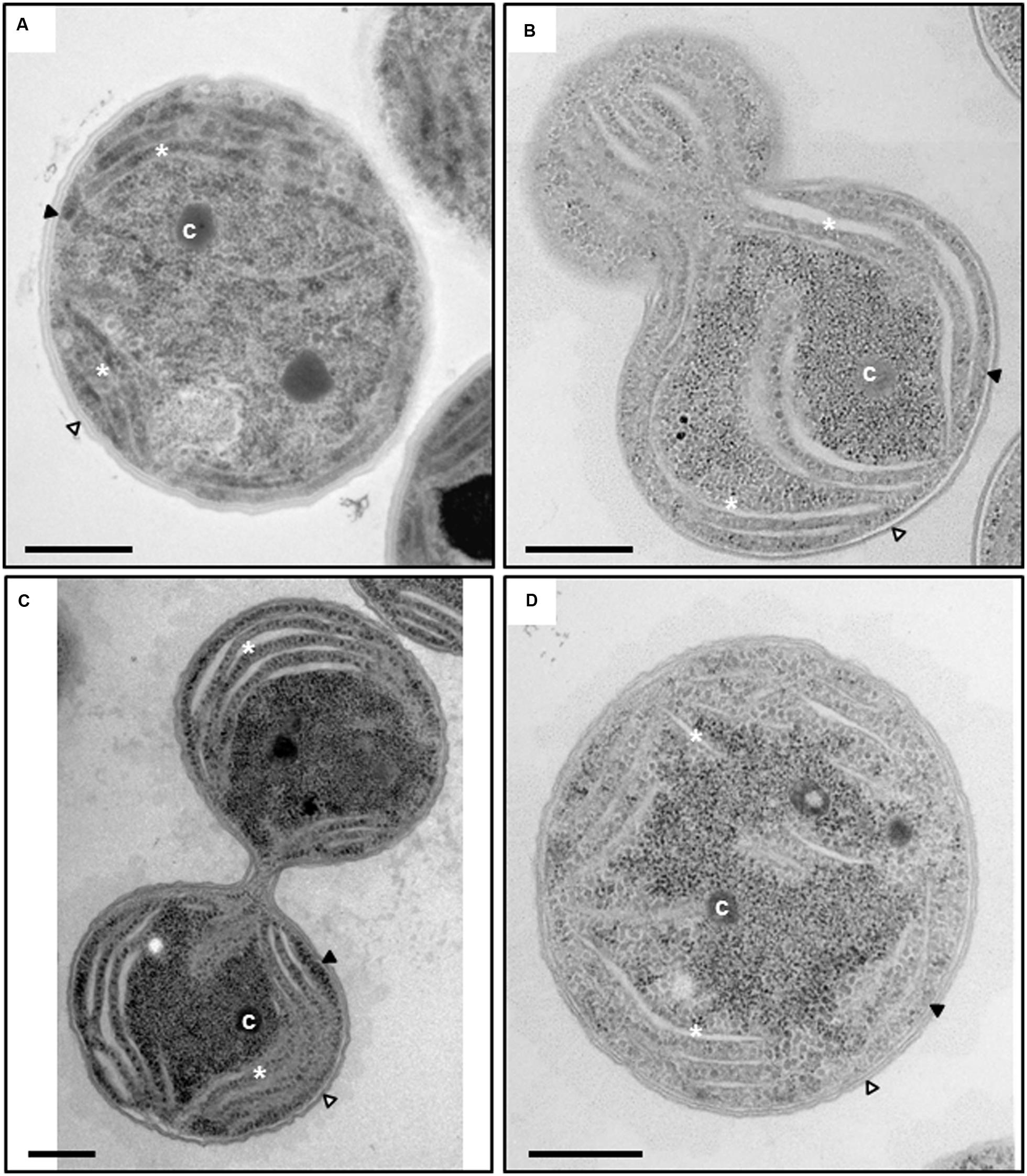
FIGURE 2. Transmission electron microscopy of wild-type Synechocystis (A) and slr0151- mutant cells (B–D). Different stages during the cell division of the slr0151- strain are shown; early stage of the cell division (B), late stage of cell division right before cytokinesis (C) and non-dividing single cell (D). Intracellular components are labeled: Outer membrane (white arrowhead), plasma membrane (black arrowhead), thylakoids (white asterisks) and carboxysome (c). Bars = 500 nm.
Localization of Slr0151 and PSII-Related Factors in Membrane Subfractions
Slr0151 has previously been reported to localize to both the PM and TMs, based on a combined sucrose density/two-phase partitioning approach (Yang et al., 2014). Alternatively, cyanobacterial membranes can be fractionated into PM and TMs via a sucrose step gradient, and the latter can be further fractionated into PratA-defined biogenic membranes (PDMs) and photosynthetically active thylakoids on a second, linear sucrose gradient (Schottkowski et al., 2009a; Heinz et al., 2016). When the distribution of Slr0151 in membrane sub-fractions was followed by applying the latter technique, the protein was accordingly detected in both PM and TM fractions (Figure 3). Further fractionation of thylakoids then revealed that Slr0151 is found in both PDMs and TMs, indicating that the protein is broadly distributed throughout the cell (Figure 4A). Since Slr0151 accumulation was affected in several PSII-related mutants, we next analyzed its TM distribution in the various mutant backgrounds (Figures 2B and 4B). In most cases, Slr0151 distribution followed the wild-type pattern. The only exceptions were the pratA- and sll0933- mutants (Figures 4A,B). In these strains, a shift of Slr0151-containing material toward the less dense PDM fractions was observed (Figures 4A,B). This again suggested a functional relationship between Slr0151 and the PAM68 homolog Sll0933 and, furthermore, a connection to the biogenic PratA-defined region at the periphery of the cell.
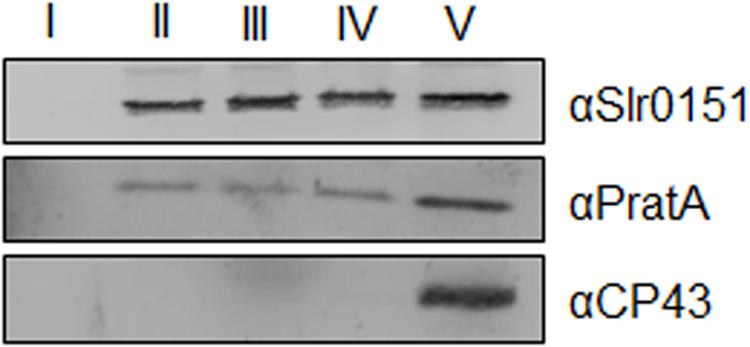
FIGURE 3. Membrane localization of Slr0151. Sucrose step-gradient centrifugation was used to separate membrane types from wild-type Synechocystis cells. Fraction II corresponds to the plasma membrane (PM) and fraction V contains pratA-defined biogenic membranes (PDMs) + thylakoid membrane (TMs). For fractions I–IV 10% of the total volume of each fraction was loaded, while only 0.2% of fraction V was analyzed (Schottkowski et al., 2009a).
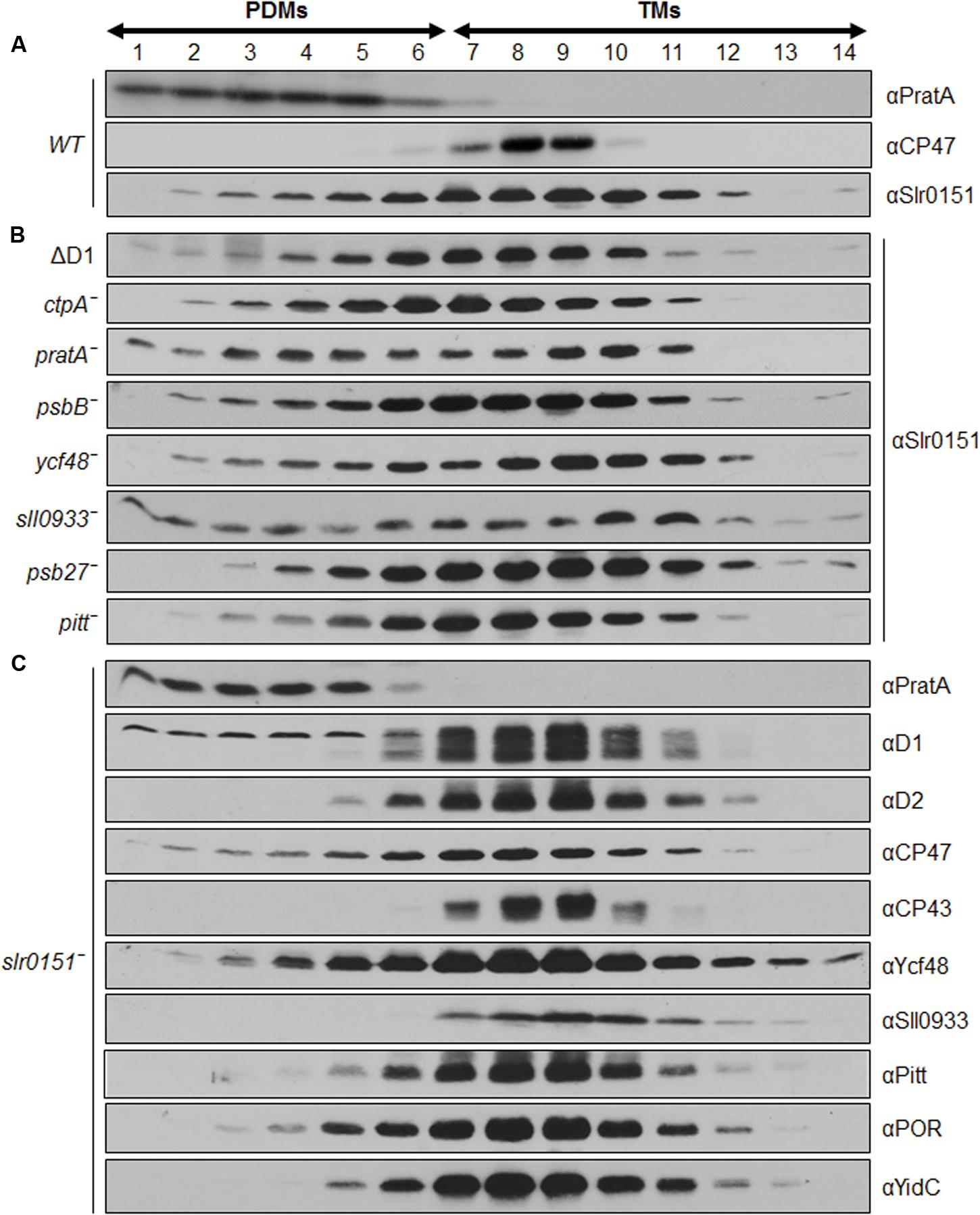
FIGURE 4. Distribution of Slr0151 between PDMs and TMs. (A) Wild-type fraction V (see Figure 3) was centrifuged on linear sucrose gradients in order to separate wild-type PDMs from TMs. (B) Distribution of Slr0151 between PDMs and TMs in the indicated PSII mutants. (C) Distribution of various PSII-related proteins in slr0151- cells. The part of the gradient from 20 to 60% sucrose was apportioned into 14 fractions, which were analyzed by immunoblotting using the antibodies indicated on the right. Fractions 1–6 represent PDMs, and fractions 7–14 represent TMs. To facilitate comparison between gradients, sample volumes were normalized to the volume of fraction 7 that contained 40 μg of protein.
When the distribution of several PSII-related proteins was monitored in a slr0151- background, no significant effects were seen (Figure 4C; Rengstl et al., 2011). The only alteration in membrane distribution concerned CP47. This is usually seen exclusively in TMs, but accumulates to some extent in PDM fractions in the absence of Slr0151 (Figures 4A,C). However, Slr0151 localization was not affected in a psbB- mutant (Figure 4B). Taken together, these data revealed a broad membrane distribution of Slr0151 and further confirmed its relationship to PSII assembly/repair. The distribution Slr0151 in the WT is almost identical to the distribution of Ycf48 which has been shown to be involved in assembly and repair of PSII.
Localization of Slr0151 via Immunofluorescence
To obtain a more comprehensive view of the subcellular localization of Slr0151, we next performed immunofluorescence (IF) analyses with affinity-purified αSlr0151, in combination with an Alexa488-labeled secondary antibody. Fluorescence was recorded by wide-field microscopy followed by deconvolution. In Figure 5, Z-montages from different cells are shown that display sequential slices from the Z-stack to provide a better 3D representation of whole cell volumes. Overall, Slr0151 IF signals in wild-type cells grown under normal light conditions were unevenly distributed, with frequent spot-like concentrations. These partly coincided with the Chl autofluorescence of the thylakoids, but were also detected in regions with low Chl fluorescence, i.e., in the PM at the cell periphery and in thylakoid convergence zones close to the PM. Interestingly, fluorescence signals were also visible in Chl-less central regions of the cells, where fewer thylakoid lamellae tend to traverse the cytoplasm (Figure 5A). Thus, the fluorescence signal is in accordance with the observations from membrane fraction analysis, i.e., that Slr0151 is located in the PM, and in PDMs and TMs. In addition, these data provide evidence that Slr0151 is found in punctate concentrations within the membrane, reminiscent of the previously described distribution of FtsH2-GFP signals, which are thought to label PSII repair zones (Sacharz et al., 2015). Similar to FtsH-GFP signals, Slr0151 IF patterns were unchanged after a 1-h exposure to high light, suggesting that an enhanced requirement for PSII repair does not provoke any substantial reorganization of Slr0151 localization (Figure 5B; Sacharz et al., 2015).
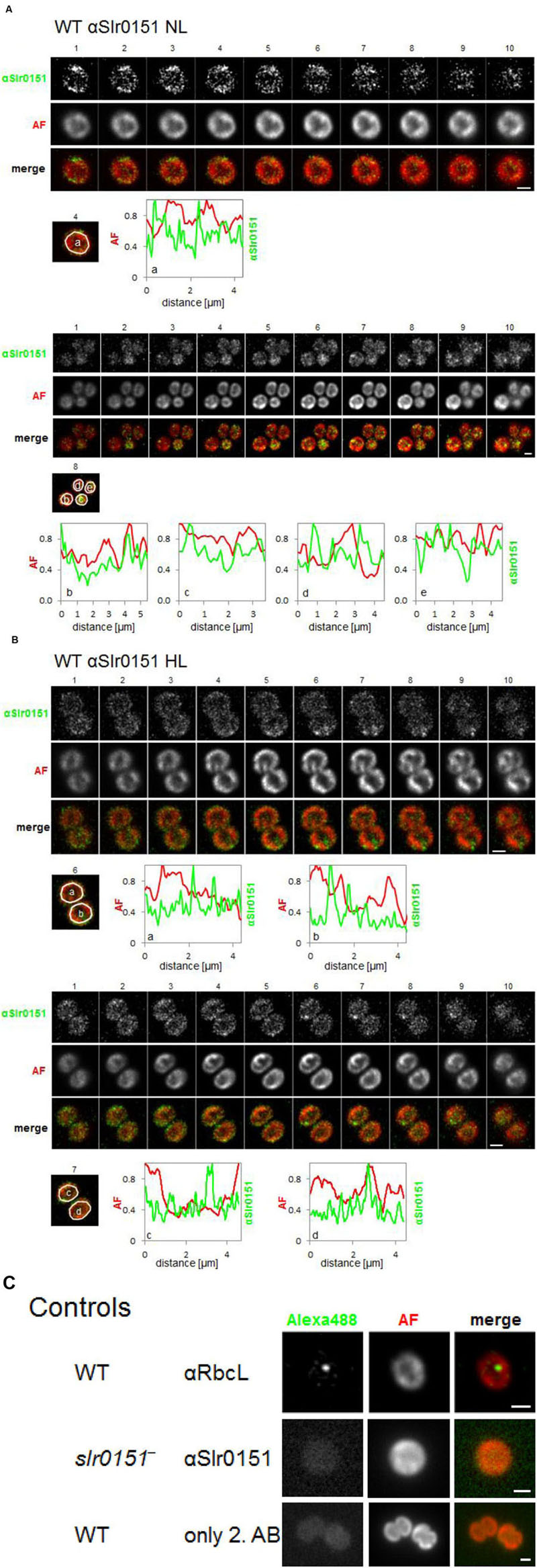
FIGURE 5. Subcellular localization of Slr0151 by immunofluorescence analysis of wild-type Synechocystis cells. Cells grown under normal light conditions (A) and cells that had been exposed to high light for 1 h (B) were treated with αSlr0151 antibody and detected with an Alexa-488-coupled secondary antibody. The constituent subpanels show Z-montages (200 nm spacing) of each separate channel as well as the merged channel. The line plots of relative fluorescence signals are derived from scans of the area defined by the white circle around the indicated cells, and correspond to the Z-slice number of the analyzed slice (given at the top). (C) For controls, cells were grown under normal conditions. In the upper row, Synechocystis wild-type cells were immunostained with αRbcL and visualized with Alexa-488 coupled secondary antibody. In the middle row, slr0151- mutant cells have been treated with the αSlr0151 antibody followed by Alexa-488-coupled secondary antibody. In the bottom row, wild-type cells were probed with Alexa-488-coupled secondary antibody alone. AF, autofluorescence of chlorophyll; scale bar 1 μm.
Control experiments included the omission of the specific αSlr0151 antibody, and analysis of the slr0151- mutant, which displayed at most diffuse background signals (Figure 5C). Moreover, we used an αRbcL antibody as a control for a non-membrane protein that gives rise to fluorescence labeling of carboxysomes from Synechocystis (Cameron et al., 2013).
Discussion
Yang et al. (2014) demonstrated that the TPR protein Slr0151 is involved in the repair of PSII in Synechocystis cultures grown at high light. Prompted by the finding that a milder phenotype can be observed under normal lighting conditions, we have carried out a further investigation of the effects of loss of Slr0151 in that context. In particular, our data suggest a functional relationship between Slr0151 and the PSII assembly factor Sll0933, a homolog of the PAM68 protein from A. thaliana, which has been shown to play a role in the conversion of RC complexes into larger PSII pre-complexes by facilitating attachment of the inner antenna proteins (Figure 1B; Armbruster et al., 2010; Rengstl et al., 2013).
In agreement with the idea that Slr0151 is involved in the transition to larger PSII pre-complexes, reduced amounts of the RC47 complex have previously been detected in slr0151- cells grown under high light (Yang et al., 2014). Moreover, the direct interaction of Slr0151 with CP43 and D1, as well as the unusual membrane distribution of CP47 in PDMs in the slr0151- background, suggest a role for Slr0151 in the transition from RC complexes to PSII monomers (Figure 4C; Yang et al., 2014). Interestingly, PDM-localized CP47 fractions have also been observed in a ctpA- mutant, which further supports the idea that Slr0151 acts during the transition from the RC47 complex to the PSII monomer lacking the OEC (Rengstl et al., 2011). Ycf48 is involved in the formation of the RC complex during assembly and repair of PSII (Komenda et al., 2008; Rengstl et al., 2011). Interestingly, it is distributed like Slr0151 in membrane fractionation experiments (Figure 4). This might suggest that a broad membrane distribution of PSII related proteins is characteristic for factors being involved in both PSII assembly and repair. Taken together, these findings confirm a PSII-related function for Slr0151, and demonstrate that, even under normal lighting conditions, distinct molecular phenotypes are detectable upon inactivation of Slr0151.
This is further underlined by the altered ultrastructure of thylakoids, i.e., looser membrane packing and increased lumen volume, seen in the slr0151- mutant grown in normal light. Swollen lumina of thylakoids have been observed before in WT cells grown at 0.5 μmol photons m-2 s-1 and in WT and mutants with different depletions of carotenoids grown in the dark with 10 min of light per day (Van de Meene et al., 2012; Toth et al., 2015). Recently, a study using inelastic neutron scattering on living Synechocystis WT cells investigated the membrane dynamics of thylakoids during light and dark periods (Stingaciu et al., 2016). The authors showed that the TM in Synechocystis is less flexible in the light as compared to dark conditions due to formation of the photosynthetic proton gradient across the TMs. Therefore, it appears possible that distorted photosynthesis or an absence of the structural function of Slr0151 itself causes swollen thylakoids in the slr0151- mutant. Such a structural role would also be in line with the observed broader membrane distribution of Slr0151. Taken together, we propose that Slr0151 – like other PSII assembly factors such as CtpA, Ycf48 and Psb27 – is involved in both PSII assembly and repair (Nowaczyk et al., 2006; Komenda et al., 2007; Nickelsen and Rengstl, 2013; Jackson et al., 2014; Mabbitt et al., 2014). The suggested involvement of Slr0151 in both processes is also consistent with the fact that the RC47 complex represents the point of convergence between them.
Slr0151 is an intrinsic membrane protein that does not accumulate in the cytoplasm (Yang et al., 2014; data not shown). Indeed, it can be found in a variety of specialized membrane domains. Previously, Slr0151 was detected in the PM as well as in the thylakoids of Synechocystis (Huang et al., 2002; Yang et al., 2014). This distribution was confirmed by our membrane fraction experiments (Figures 3 and 4). In addition, substantial amounts of Slr0151 were observed in PDMs, which are localized at sites where thylakoids converge upon the PM. According to rough estimates based on densitometrical signal analysis, approximately 2% of total cellular Slr0151 is found in PMs and 25 and 70% in PDMs and TMs, respectively. IF analyses confirmed this overall distribution and revealed frequent punctate concentrations of Slr0151 in all membrane types (Figure 5). Intriguingly, a similar localization pattern has been observed for GFP-tagged FtsH2, the protease which degrades damaged D1 protein during PSII repair. The GFP signal co-localized with the Chl autofluorescence and showed patches of increased intensity within as well as between thylakoids at their peripheral convergence sites (Sacharz et al., 2015). The same patterns were maintained under high light conditions for both Slr0151 and FtsH2 (Sacharz et al., 2015). Thus, these data, together with the observation that the synthesis of D1 following photodamage is affected by Slr0151 inactivation (Yang et al., 2014), are consistent with a role of Slr0151 during repair. Furthermore, IF analysis has shown that some Slr0151 is concentrated in thylakoids that traverse the cell center. Whether this reflects any distinct functional role of these regions remains to be discovered.
This work reveals new aspects of the function of the TPR protein Slr0151, i.e., its involvement in PSII assembly in addition to its previously described role in PSII repair. The fact that PSII assembly takes place in BCs does not exclude the possibility that repair and assembly of PSII are co-localized in those regions. Since several steps of assembly and repair involve the same assembly/repair factors as well as some assembly and assembly repair intermediates (Schottkowski et al., 2009a; Rengstl et al., 2011; Stengel et al., 2012). Therefore, the current findings suggest a close relationship between PSII assembly and repair, with regard to the factors involved and their subcellular distribution (Nickelsen and Rengstl, 2013; Mabbitt et al., 2014).
Author Contributions
AR, BR, SH, AK, and JN designed the research. AR, BR, and SH performed the research. AR and JN perpared the article.
Funding
This work was supported by funding from the Deutsche Forschungsgemeinschaft for Research Unit FOR2092 (Ni390/9-1).
Conflict of Interest Statement
The authors declare that the research was conducted in the absence of any commercial or financial relationships that could be construed as a potential conflict of interest.
The reviewer SJ and handling Editor declared their shared affiliation, and the handling Editor states that the process nevertheless met the standards of a fair and objective review.
Acknowledgments
We thank Jürgen Soll for providing αYidC antibody, Marc Bramkamp for help with the Delta Vision and Silvia Dobler for technical assistance. Furthermore, we thank Paul Hardy for critical reading of the manuscript.
Supplementary Material
The Supplementary Material for this article can be found online at: http://journal.frontiersin.org/article/10.3389/fpls.2016.00605
FIGURE S1 | Generation of the Synechocystis slr0151- mutant. (A) Construction of the insertional slr0151- mutant. (B) PCR-based segregation analysis of the Synechocystis slr0151- mutant [primer locations are indicated by black arrows in (A)]. (C) Levels of Slr0151 protein detected with αSlr0151 antiserum in the wild-type (WT), slr0151- mutant and the complemented slr0151- strain (re-slr0151-). RbcL served as the loading control.
FIGURE S2 | Protein levels in the slr0151- mutant and of Slr0151 in various PSII mutants. Representative western blots from the protein level analysis shown in Figure 1. Total proteins were isolated from the respective line and analyzed via SDS-PAGE and western blot. 30 μg were loaded for 100% wild type and each mutant. The quantification of at least three independent experiments is summarized in Figure 1. The RbcL signal served as internal standard for relative quantification. (A) Protein levels of the indicated PSII subunits and PSII-related proteins in the slr0151- mutant. Wild-type and mutant samples were analyzed on the same gel. However, signals from unrelated samples, which were loaded in between, were excised. (B) Representative western analysis of Slr0151 in various PSII mutants.
References
Anbudurai, P. R., Mor, T. S., Ohad, I., Shestakov, S. V., and Pakrasi, H. B. (1994). The ctpA gene encodes the C-terminal processing protease for the D1 protein of the photosystem II reaction center complex. Proc. Natl. Acad. Sci. U.S.A. 91, 8082–8086. doi: 10.1073/pnas.91.17.8082
Armbruster, U., Zuhlke, J., Rengstl, B., Kreller, R., Makarenko, E., Rühle, T., et al. (2010). The Arabidopsis thylakoid protein PAM68 is required for efficient D1 biogenesis and photosystem II assembly. Plant Cell 22, 3439–3460. doi: 10.1105/tpc.110.077453
Becker, K., Cormann, K. U., and Nowaczyk, M. M. (2011). Assembly of the water-oxidizing complex in photosystem II. J. Photochem. Photobiol. B Biol. 104, 204–211. doi: 10.1016/j.jphotobiol.2011.02.005
Blatch, G. L., and Lässle, M. (1999). The tetratricopeptide repeat: a structural motif mediating protein-protein interactions. Bioessays 21, 932–939. doi: 10.1002/(SICI)1521-1878(199911)21:11<932::AID-BIES5>3.3.CO;2-E
Boehm, M., Yu, J., Krynicka, V., Barker, M., Tichy, M., Komenda, J., et al. (2012). Subunit organization of a Synechocystis hetero-oligomeric thylakoid FtsH complex involved in photosystem II repair. Plant Cell 24, 3669–3683. doi: 10.1098/rstb.2012.0066
Bohne, A. V., Schwenkert, S., Grimm, B., and Nickelsen, J. (2016). Roles of tetratricopeptide repeat proteins in piogenesis of the photosynthetic apparatus. Int. Rev. Cell Mol. Biol. 324, 187–227. doi: 10.1016/bs.ircmb.2016.01.005
Cameron, J. C., Wilson, S. C., Bernstein, S. L., and Kerfeld, C. A. (2013). Biogenesis of a bacterial organelle: the carboxysome assembly pathway. Cell 155, 1131–1140. doi: 10.1016/j.cell.2013.10.044
Cormann, K. U., Bartsch, M., Rogner, M., and Nowaczyk, M. M. (2014). Localization of the CyanoP binding site on photosystem II by surface plasmon resonance spectroscopy. Front. Plant Sci. 5:595. doi: 10.3389/fpls.2014.00595
D’Andrea, L. D., and Regan, L. (2003). TPR proteins: the versatile helix. Trends Biochem. Sci. 28, 655–662. doi: 10.1016/j.tibs.2003.10.007
Eaton-Rye, J. J., and Vermaas, W. F. (1991). Oligonucleotide-directed mutagenesis of psbB, the gene encoding CP47, employing a deletion mutant strain of the cyanobacterium Synechocystis sp. PCC 6803. Plant Mol. Biol. 17, 1165–1177. doi: 10.1007/BF00028733
Heinz, S., Liauw, P., Nickelsen, J., and Nowaczyk, M. (2016). Analysis of photosystem II biogenesis in cyanobacteria. Biochim. Biophys. Acta 1857, 274–287. doi: 10.1016/j.bbabio.2015.11.007
Hohmann-Marriott, M. F., and Blankenship, R. E. (2011). Evolution of photosynthesis. Annu. Rev. Plant Biol. 62, 515–548. doi: 10.1146/annurev-arplant-042110-103811
Huang, F., Parmryd, I., Nilsson, F., Persson, A. L., Pakrasi, H. B., Andersson, B., et al. (2002). Proteomics of Synechocystis sp. strain PCC 6803: identification of plasma membrane proteins. Mol. Cell. Proteomics 1, 956–966. doi: 10.1074/mcp.M200043-MCP200
Jackson, S. A., and Eaton-Rye, J. J. (2015). Characterization of a Synechocystis sp. PCC 6803 double mutant lacking the CyanoP and Ycf48 proteins of Photosystem II. Photosynth. Res. 124, 217–229. doi: 10.1007/s11120-015-0122-0
Jackson, S. A., Hervey, J. R., Dale, A. J., and Eaton-Rye, J. J. (2014). Removal of both Ycf48 and Psb27 in Synechocystis sp. PCC 6803 disrupts Photosystem II assembly and alters Q(A)(-) oxidation in the mature complex. FEBS Lett. 588, 3751–3760. doi: 10.1016/j.febslet.2014.08.024
Klingl, A., Moissl-Eichinger, C., Wanner, G., Zweck, J., Huber, H., Thomm, M., et al. (2011). Analysis of the surface proteins of Acidithiobacillus ferrooxidans strain SP5/1 and the new, pyrite-oxidizing Acidithiobacillus isolate HV2/2, and their possible involvement in pyrite oxidation. Arch. Microbiol. 193, 867–882. doi: 10.1007/s00203-011-0720-y
Klinkert, B., Ossenbuhl, F., Sikorski, M., Berry, S., Eichacker, L., and Nickelsen, J. (2004). PratA, a periplasmic tetratricopeptide repeat protein involved in biogenesis of photosystem II in Synechocystis sp. PCC 6803. J. Biol. Chem. 279, 44639–44644. doi: 10.1074/jbc.M405393200
Komenda, J., Barker, M., Kuvikova, S., De Vries, R., Mullineaux, C. W., Tichy, M., et al. (2006). The FtsH protease slr0228 is important for quality control of photosystem II in the thylakoid membrane of Synechocystis sp. PCC 6803. J. Biol. Chem. 281, 1145–1151. doi: 10.1074/jbc.M503852200
Komenda, J., Knoppova, J., Kopecna, J., Sobotka, R., Halada, P., Yu, J., et al. (2012). The Psb27 assembly factor binds to the CP43 complex of photosystem II in the cyanobacterium Synechocystis sp. PCC 6803. Plant Physiol. 158, 476–486. doi: 10.1016/j.pbi.2012.01.017
Komenda, J., Nickelsen, J., Tichy, M., Prasil, O., Eichacker, L. A., and Nixon, P. J. (2008). The cyanobacterial homologue of HCF136/YCF48 is a component of an early photosystem II assembly complex and is important for both the efficient assembly and repair of photosystem II in Synechocystis sp. PCC 6803. J. Biol. Chem. 283, 22390–22399. doi: 10.1074/jbc.M801917200
Komenda, J., Reisinger, V., Muller, B. C., Dobakova, M., Granvogl, B., and Eichacker, L. A. (2004). Accumulation of the D2 protein is a key regulatory step for assembly of the photosystem II reaction center complex in Synechocystis PCC 6803. J. Biol. Chem. 279, 48620–48629. doi: 10.1074/jbc.M405725200
Komenda, J., Tichy, M., Prasil, O., Knoppova, J., Kuvikova, S., De Vries, R., et al. (2007). The exposed N-terminal tail of the D1 subunit is required for rapid D1 degradation during photosystem II repair in Synechocystis sp PCC 6803. Plant Cell 19, 2839–2854. doi: 10.1016/j.bbabio.2007.01.005
Kopf, M., Klahn, S., Scholz, I., Matthiessen, J. K., Hess, W. R., and Voss, B. (2014). Comparative analysis of the primary transcriptome of Synechocystis sp. PCC 6803. DNA Res. 21, 527–539. doi: 10.1093/dnares/dsu018
Kubota, H., Sakurai, I., Katayama, K., Mizusawa, N., Ohashi, S., Kobayashi, M., et al. (2010). Purification and characterization of photosystem I complex from Synechocystis sp. PCC 6803 by expressing histidine-tagged subunits. Biochim. Biophys. Acta 1, 98–105. doi: 10.1016/j.bbabio.2009.09.001
Kupitz, C., Basu, S., Grotjohann, I., Fromme, R., Zatsepin, N. A., Rendek, K. N., et al. (2014). Serial time-resolved crystallography of photosystem II using a femtosecond X-ray laser. Nature 513, 261–265. doi: 10.1038/nature13453
Mabbitt, P. D., Wilbanks, S. M., and Eaton-Rye, J. J. (2014). Structure and function of the hydrophilic Photosystem II assembly proteins: Psb27, Psb28 and Ycf48. Plant Physiol. Biochem. 81, 96–107. doi: 10.1016/j.plaphy.2014.02.013
Mulo, P., Laakso, S., Maenpaa, P., and Aro, E. M. (1998). Stepwise photoinhibition of photosystem II. Studies with Synechocystis species PCC 6803 mutants with a modified D-E loop of the reaction center polypeptide D1. Plant Physiol. 117, 483–490. doi: 10.1104/pp.117.2.483
Mulo, P., Sakurai, I., and Aro, E. M. (2012). Strategies for psbA gene expression in cyanobacteria, green algae and higher plants: from transcription to PSII repair. Biochim. Biophys. Acta 1, 247–257. doi: 10.1016/j.bbabio.2011.04.011
Nickelsen, J., and Rengstl, B. (2013). Photosystem II assembly: from cyanobacteria to plants. Annu. Rev. Plant Biol. 64, 609–635. doi: 10.1146/annurev-arplant-050312-120124
Nickelsen, J., and Zerges, W. (2013). Thylakoid biogenesis has joined the new era of bacterial cell biology. Front. Plant Sci. 4:458. doi: 10.3389/fpls.2013.00458
Nixon, P. J., Trost, J. T., and Diner, B. A. (1992). Role of the carboxy terminus of polypeptide D1 in the assembly of a functional water-oxidizing manganese cluster in photosystem II of the cyanobacterium Synechocystis sp. PCC 6803: assembly requires a free carboxyl group at C-terminal position 344. Biochemistry 31, 10859–10871. doi: 10.1093/aob/mcq059
Nowaczyk, M. M., Hebeler, R., Schlodder, E., Meyer, H. E., Warscheid, B., and Rogner, M. (2006). Psb27, a cyanobacterial lipoprotein, is involved in the repair cycle of photosystem II. Plant Cell 18, 3121–3131. doi: 10.1105/tpc.106.042671
Ossenbühl, F., Inaba-Sulpice, M., Meurer, J., Soll, J., and Eichacker, L. A. (2006). The Synechocystis sp PCC 6803 Oxa1 homolog is essential for membrane integration of reaction center precursor protein pD1. Plant Cell 18, 2236–2246. doi: 10.1105/tpc.106.043646
Prasil, O., Adir, N., and Ohad, I. (1992). Dynamics of photosystem II: mechanism of photoinhibition and recovery process. Top. Photosynth. 11, 295–348.
Rachel, R., Meyer, C., Klingl, A., Gurster, S., Heimerl, T., Wasserburger, N., et al. (2010). Analysis of the ultrastructure of archaea by electron microscopy. Methods Cell Biol. 96, 47–69. doi: 10.1016/S0091-679X(10)96003-2
Rast, A., Heinz, S., and Nickelsen, J. (2015). Biogenesis of thylakoid membranes. Biochim. Biophys. Acta 9, 821–830. doi: 10.1016/j.bbabio.2015.01.007
Rengstl, B., Knoppova, J., Komenda, J., and Nickelsen, J. (2013). Characterization of a Synechocystis double mutant lacking the photosystem II assembly factors YCF48 and Sll0933. Planta 237, 471–480. doi: 10.1007/s00425-012-1720-0
Rengstl, B., Oster, U., Stengel, A., and Nickelsen, J. (2011). An intermediate membrane subfraction in cyanobacteria is involved in an assembly network for photosystem II biogenesis. J. Biol. Chem. 286, 21944–21951. doi: 10.1074/jbc.M111.237867
Reynolds, E. S. (1963). The use of lead citrate at high pH as an electron-opaque stain in electron microscopy. J. Cell Biol. 17, 208–212. doi: 10.1083/jcb.17.1.208
Sacharz, J., Bryan, S. J., Yu, J., Burroughs, N. J., Spence, E. M., Nixon, P. J., et al. (2015). Sub-cellular location of FtsH proteases in the cyanobacterium Synechocystis sp. PCC 6803 suggests localised PSII repair zones in the thylakoid membranes. Mol. Microbiol. 96, 448–462. doi: 10.1111/mmi.12940
Schottkowski, M., Gkalympoudis, S., Tzekova, N., Stelljes, C., Schunemann, D., Ankele, E., et al. (2009a). Interaction of the periplasmic PratA factor and the PsbA (D1) protein during biogenesis of photosystem II in Synechocystis sp. PCC 6803. J. Biol. Chem. 284, 1813–1819. doi: 10.1074/jbc.M806116200
Schottkowski, M., Ratke, J., Oster, U., Nowaczyk, M., and Nickelsen, J. (2009b). Pitt, a novel tetratricopeptide repeat protein involved in light-dependent chlorophyll biosynthesis and thylakoid membrane biogenesis in Synechocystis sp. PCC 6803. Mol. Plant 2, 1289–1297. doi: 10.1093/mp/ssp075
Silva, P., Thompson, E., Bailey, S., Kruse, O., Mullineaux, C. W., Robinson, C., et al. (2003). FtsH is involved in the early stages of repair of photosystem II in Synechocystis sp PCC 6803. Plant Cell 15, 2152–2164. doi: 10.1105/tpc.012609
Singh, A. K., Li, H., and Sherman, L. A. (2004). Microarray analysis and redox control of gene expression in the cyanobacterium Synechocystis sp. PCC 6803. Physiol. Plant. 120, 27–35. doi: 10.1111/j.0031-9317.2004.0232.x
Stengel, A., Gugel, I. L., Hilger, D., Rengstl, B., Jung, H., and Nickelsen, J. (2012). Initial steps of photosystem II de novo assembly and preloading with manganese take place in biogenesis centers in Synechocystis. Plant Cell 24, 660–675. doi: 10.1105/tpc.111.093914
Stingaciu, L. R., O’neill, H., Liberton, M., Urban, V. S., Pakrasi, H. B., and Ohl, M. (2016). Revealing the dynamics of thylakoid membranes in living cyanobacterial cells. Sci. Rep. 6, 19627. doi: 10.1038/srep19627
Suga, M., Akita, F., Hirata, K., Ueno, G., Murakami, H., Nakajima, Y., et al. (2015). Native structure of photosystem II at 1.95 A resolution viewed by femtosecond X-ray pulses. Nature 517, 99–103. doi: 10.1038/nature13991
Toth, T. N., Chukhutsina, V., Domonkos, I., Knoppova, J., Komenda, J., Kis, M., et al. (2015). Carotenoids are essential for the assembly of cyanobacterial photosynthetic complexes. Biochim. Biophys. Acta 1847, 1153–1165. doi: 10.1016/j.bbabio.2015.05.020
Umena, Y., Kawakami, K., Shen, J. R., and Kamiya, N. (2011). Crystal structure of oxygen-evolving photosystem II at a resolution of 1.9 A. Nature 473, 55–60. doi: 10.1038/nature09913
van de Meene, A. M., Hohmann-Marriott, M. F., Vermaas, W. F., and Roberson, R. W. (2006). The three-dimensional structure of the cyanobacterium Synechocystis sp. PCC 6803. Arch. Microbiol. 184, 259–270. doi: 10.1007/s00203-005-0027-y
Van de Meene, A. M., Sharp, W. P., Mcdaniel, J. H., Friedrich, H., Vermaas, W. F., and Roberson, R. W. (2012). Gross morphological changes in thylakoid membrane structure are associated with photosystem I deletion in Synechocystis sp. PCC 6803. Biochim. Biophys. Acta 1818, 1427–1434. doi: 10.1016/j.bbamem.2012.01.019
Wegener, K. M., Welsh, E. A., Thornton, L. E., Keren, N., Jacobs, J. M., Hixson, K. K., et al. (2008). High sensitivity proteomics assisted discovery of a novel operon involved in the assembly of photosystem II, a membrane protein complex. J. Biol. Chem. 283, 27829–27837. doi: 10.1074/jbc.M803918200
Wilde, A., Lunser, K., Ossenbuhl, F., Nickelsen, J., and Borner, T. (2001). Characterization of the cyanobacterial ycf37: mutation decreases the photosystem I content. Biochem. J. 357, 211–216. doi: 10.1042/bj3570211
Yang, H., Liao, L., Bo, T., Zhao, L., Sun, X., Lu, X., et al. (2014). Slr0151 in Synechocystis sp. PCC 6803 is required for efficient repair of photosystem II under high-light condition. J. Integr. Plant Biol. 56, 1136–1150. doi: 10.1111/jipb.12275
Zak, E., Norling, B., Maitra, R., Huang, F., Andersson, B., and Pakrasi, H. B. (2001). The initial steps of biogenesis of cyanobacterial photosystems occur in plasma membranes. Proc. Natl. Acad. Sci. U.S.A. 98, 13443–13448. doi: 10.1073/pnas.241503898
Zhang, L., Paakkarinen, V., Van Wijk, K. J., and Aro, E. M. (1999). Co-translational assembly of the D1 protein into photosystem II. J. Biol. Chem. 274, 16062–16067. doi: 10.1074/jbc.274.23.16062
Keywords: Synechocystis, biogenesis center, TPR protein, photosystem II, thylakoid membrane
Citation: Rast A, Rengstl B, Heinz S, Klingl A and Nickelsen J (2016) The Role of Slr0151, a Tetratricopeptide Repeat Protein from Synechocystis sp. PCC 6803, during Photosystem II Assembly and Repair. Front. Plant Sci. 7:605. doi: 10.3389/fpls.2016.00605
Received: 19 February 2016; Accepted: 19 April 2016;
Published: 03 May 2016.
Edited by:
Julian Eaton-Rye, University of Otago, New ZealandReviewed by:
Yan Lu, Western Michigan University, USASimon Andrew Jackson, University of Otago, New Zealand
Copyright © 2016 Rast, Rengstl, Heinz, Klingl and Nickelsen. This is an open-access article distributed under the terms of the Creative Commons Attribution License (CC BY). The use, distribution or reproduction in other forums is permitted, provided the original author(s) or licensor are credited and that the original publication in this journal is cited, in accordance with accepted academic practice. No use, distribution or reproduction is permitted which does not comply with these terms.
*Correspondence: Jörg Nickelsen, am9lcmcubmlja2Vsc2VuQGxyei51bmktbXVlbmNoZW4uZGU=