- 1School of Agriculture, Policy and Development, University of Reading, Reading, UK
- 2Faculty of Forestry and Wood Sciences, Czech University of Life Sciences, Prague, Czechia
- 3Department of Crop Genetics, John Innes Centre, Norwich, UK
- 4Computational and Systems Biology Department, Rothamsted Research, Harpenden, UK
- 5Institute of Biological, Environmental and Rural Sciences, University of Aberystwyth, Aberystwyth, UK
Successive single day transfers of pot-grown wheat to high temperature (35/30°C day/night) replicated controlled environments, from the second node detectable to the milky-ripe growth stages, provides the strongest available evidence that the fertility of wheat can be highly vulnerable to heat stress during two discrete peak periods of susceptibility: early booting [decimal growth stage (GS) 41–45] and early anthesis (GS 61–65). A double Gaussian fitted simultaneously to grain number and weight data from two contrasting elite lines (Renesansa, listed in Serbia, Ppd-D1a, Rht8; Savannah, listed in UK, Ppd-D1b, Rht-D1b) identified peak periods of main stem susceptibility centered on 3 (s.e. = 0.82) and 18 (s.e. = 0.55) days (mean daily temperature = 14.3°C) pre-GS 65 for both cultivars. Severity of effect depended on genotype, growth stage and their interaction: grain set relative to that achieved at 20/15°C dropped below 80% for Savannah at booting and Renesansa at anthesis. Savannah was relatively tolerant to heat stress at anthesis. A further experiment including 62 lines of the mapping, doubled-haploid progeny of Renesansa × Savannah found tolerance at anthesis to be associated with Ppd-D1b, Rht-D1b, and a QTL from Renesansa on chromosome 2A. None of the relevant markers were associated with tolerance during booting. Rht8 was never associated with heat stress tolerance, a lack of effect confirmed in a further experiment where Rht8 was included in a comparison of near isogenic lines in a cv. Paragon background. Some compensatory increases in mean grain weight were observed, but only when stress was applied during booting and only where Ppd-D1a was absent.
Introduction
Improving crop resilience to more frequent extreme weather events is required to maintain or improve crop yields across Europe (Semenov et al., 2014). Wheat, a major contributor to human diet and health (Shewry and Hey, 2015), is particularly susceptible to heat stress around meiosis and anthesis (Barnabas et al., 2008). Yield loss due to heat stress at these growth stages is primarily due to disruption of reproductive processes (Saini and Aspinall, 1982; Saini et al., 1983), as evidenced by a reduction in fertility and grain number (Dolferus et al., 2011). Previous reports on heat stress in wheat usually concern only one of the susceptible timings i.e., meiosis (Saini and Aspinall, 1982; Saini et al., 1984) or anthesis (Tashiro and Wardlaw, 1990; Ferris et al., 1998; Lukac et al., 2012; Pradhan et al., 2012; Steinmeyer et al., 2013; Liu et al., 2016). Fewer studies have attempted to quantify the response to stress at both of these timings: Alghabari et al. (2014) suggest meiosis is the most vulnerable stage, but Prasad and Djanaguiraman (2014) report that it is anthesis that is particularly susceptible. Previous work has often assumed that these growth stages represent two separate, discrete periods of susceptibility but there is currently little evidence to support this. Single experiments on rice and wheat suggest that there may be a period between meiosis and anthesis that is relatively tolerant to heat stress (Satake and Yoshida, 1978; Craufurd et al., 2013), but it is unclear as to the specific growth stages when this tolerance occurs. Genotypic interactions with heat stress timing also require clarification. Although some recent work has compared the heat stress response at anthesis across multiple genotypes (Liu et al., 2016), little work has quantified how genotype influences susceptibility across both stages, even though consecutive exposure of both stages to stress seems likely to occur in field conditions (Wardlaw et al., 1989).
Here, we investigate firstly whether periods of vulnerability to heat stress during reproductive phases can truly be differentiated temporally, in association with growth stage development. Secondly we investigate whether the effect of genotype on heat stress vulnerability interacts with timing of stress. We pay particular attention to the effects of three alleles reported to influence heat stress tolerance and have adaptive significance in wheat grown in European regions with different frequencies and severities of heat stress, namely Rht8, Ppd-D1a, and Rht-D1b (Worland, 1996; Worland et al., 1998; Rebetzke et al., 2007; Gasperini et al., 2012; Alghabari et al., 2014; Barber et al., 2015; Kowalski et al., 2016; Jones et al., 2017). We also assess associations with the 1BL/1RS translocation (Schlegel and Korzun, 1997) which introduced a number of race-specific disease resistance genes (Snape et al., 2007). The translocation has also been variously associated with increased above ground biomass, spikelet fertility, delayed senescence, and drought tolerance (Villareal et al., 1998; Rajaram, 2001), but there is apparently little information with regards to its influence on heat stress tolerance.
This paper describes the use of 1-day transfers of pot-grown wheat to replicated controlled environments to identify and characterize any periods of heat susceptibility during external growth stages extending from the second node detectable growth stage (GS 32; Zadoks et al., 1974) to the grain milky-ripe stage (GS 77) and hence encompassing meiosis and anthesis (Barber et al., 2015). An initial study compared the Southern European wheat Renesansa (Ppd-D1a, Rht-D1a, Rht8) to the UK-adapted wheat Savannah (Ppd-D1b, Rht-D1b, 1BL/1RS). Once susceptible growth stages were identified, further experiments compared the heat stress responses of near isogenic lines (NILs) of a Paragon background varying for presence and absence of Rht8, and also the responses of a mapping population of 62 doubled haploid progeny of Renesansa × Savannah, at appropriate timings.
Materials and Methods
Plant Material
Savannah has a high yield potential in North West Europe with low bread making quality and was recommended in the UK in 1998. Renesansa, a Serbian winter wheat listed in 1995, has high yield potential and high bread making quality in southern Europe. Sixty-two lines were selected from a recombinant doubled haploid (DH) population of Savannah × Renesansa based on their alleles at Ppd-D1, Rht-D1, 1BL/1RS, and Rht8 (Xgwm261; Simmonds et al., 2006; Snape et al., 2007). NILs varying for the presence and absence of Rht8, though both remaining sensitive to photoperiod were developed in a Paragon background (Kowalski et al., 2016). Paragon is a photoperiod sensitive spring wheat that can be also sown in autumn and was first listed in the UK in 1999 with good bread making quality.
Growing Conditions and Post-harvest Analysis
Plants used in these experiments were grown in pots (180 mm diameter) at the Plant Environment Laboratory at the University of Reading, UK (51 27′ N latitude, 00 56′ W longitude). Each pot contained 2.8 kg of growing media comprising 4:2:4:1 of vermiculite: sand: gravel: compost mixed with Osmocote slow release granules (2 kg m−3) containing a ratio of 15:11:13:2 of N:P2O5:K2O:MgO. Seven seeds were sown per pot; thinned to four plants per pot at the two leaf stage. The pots were maintained outside in prevailing conditions (Table 1) under a protective net cage in four randomized blocks with guard pots of wheat placed around the perimeter of experimental blocks. Fungicide was applied as and when required. Pots were watered up to twice daily by an automatic drip irrigation system to maintain field capacity. All treatments consisted of transfers to Saxil growth cabinets, which began between 10:20 and 11:20 h (BST) and remained there for 24 h (16 h day, night time between 22:00 and 06:00 h) before being returned outside to their original randomized block position. Average daily temperature during the treatment period was 14.3°C in 2013/14 and 13.5°C in 2014/15. Two temperature regimes were used in all experiments, day/night temperatures of 20/15 for the control treatment and 35/30°C for the heat stress treatment. Pots were irrigated to field capacity before transfer, but were not irrigated whilst in the cabinets. Eight growth cabinets were used which allowed the two temperature treatments to be replicated for the four blocks. On the day of transfer main stems in each pot were tagged and assessed for growth stage (GS, Zadoks et al., 1974). Pots were weighed immediately before and after transfer to monitor water loss. Main stems and tillers were harvested separately after physiological maturity (GS 89) and dried (48 h at 80°C). Ears and spikelets per ear were counted, after which grain was threshed from ears, then re-dried, weighed, and counted by a Kirby Lester K18 tablet counter.
Experiment 1
Experiment 1, sown on the 16th December 2013, comprised a complete factorial of: the two DH parent winter wheat cultivars, Savannah and Renesansa; day of transfer to Saxil growth cabinets (31 separate timings between May 2nd and June 13th 2014); and the two temperature regimes within growth cabinets. Confounding effects associated with temperature included water loss. The mean weight of pots on entry was 3.40 kg, whilst mean weights of pots on withdrawal were 3.19 and 2.98 kg (SED = 0.016) for the 20/15 and 35/30°C treatments, respectively. More detailed studies on the water relations within this growing medium and system suggests that this degree of water loss would equate to 78 and 56% field capacity (FC; oven dry = 0% FC; Gooding et al., 2003), respectively, and that a FC of <70% maintained for 14 days during grain filling was required to reduce grain yield. A further confounded environmental variate was mean relative humidity [73% for 20/15°C and 47% for 35/30°C (SED = 4.4)] whilst in the cabinets.
Experiment 2
Also sown on the 16th December 2013, the treatment structure comprised a complete factorial design of: three genotypes [Paragon, Rht8 NIL, and Tall NIL (Kowalski et al., 2016)]; day of transfer to Saxil growth cabinets (5 separate days between 19th May and 10th June 2014) and the two temperature regimes within growth cabinets.
Experiment 3
Experiment 3 was sown on 3rd December 2014. The treatment structure comprised a complete factorial of 62 DH Lines, three growth stages at transfer to Saxil growth cabinets, and two temperature regimes within growth cabinets. The three timings targeted specific stages of growth: early booting (GS 39–41); mid booting (GS 43–45); and early anthesis (GS 63–65). Due to variable rates of development within a 24 h period, and differential rates of progression, not all lines were transferred within target. Nonetheless, GS at transfer was always recorded.
Statistical Analysis
The primary statistical approach was an appropriate factorial analysis of variance (ANOVA) with a blocking structure of Block/Cabinet/Pot (GenStat 14th edn., VSN International Ltd.). For Experiments 1 and 2, polynomial regressions were fitted across day of transfer to growth cabinet using orthogonal polynomial contrasts in the ANOVA i.e., treatment structure was pol (Day; n) * Temperature * Genotype, where n was the maximum level of polynomial to be fitted. Where quartic effects or deviations from them were significant in Experiment 1, fits were compared with the double Gaussian model (Equation 1) on an r2 adj basis. The maximal double Gaussian model permits the estimation of two “bell-shaped” curves:
Where: Relative Effect is the result at 35°C (day temperature) expressed as a percentage of that achieved at 20°C; b and c are the size of the two peaks; m and n are when, in time t, they are centered; and s1 and s2 are the Gaussian shape factors (standard deviation) for the two peaks. This double Gaussian approach has previously been used to detect other phenologically-dependent responses in wheat time series data sets (Lu et al., 2014). The FITNONLINEAR routine in GENSTAT 14 was used to compare regressions and allow a parsimonious approach to the inclusion of various parameters in the model fits. Additionally, the routine allowed simultaneous fits to different response variates (weighted for the inverses of their variances). Here, it was used to investigate potential compensation in mean grain weights at the time when grain numbers were reduced by heat stress.
Experiment 3 was analyzed by ANOVA with a treatment structure of Genotype × Target Growth Stage × Temperature. A regression analysis was conducted in an attempt to control the effects of varying growth stages within the target GS cohorts. Main and interacting effects of Rht-D1b, Rht8, Ppd-D1a, and 1BL/1RS were tested for their significance in the model (P < 0.05). In addition, after correcting for the linear effect of GS within target GS cohort, a QTL analysis was conducted from the effects of the high temperature treatment on individual lines within each target GS. A framework genetic map was constructed from 93 lines of the population as previously described by Snape et al. (2007), containing 107 single sequence repeat (SSR) markers and perfect markers for Ppd-D1, Rht-D1, and 1BL/1RS. Linkage map construction was performed using JoinMap® 3.0 (Kyazma BV) with default settings. Linkage groups were determined using a Divergent log-of-odds (LOD) threshold of 3.0 and genetic distances were computed using the Kosambi regression. The genetic map consisted of 25 linkage groups with 45 unlinked markers. QTL Cartographer 2.5 (North Carolina State University) was used for QTL detection using single marker analysis and composite interval mapping (CIM). Estimates of the additive effects and percentage of total variation for identified QTL were calculated using the multiple interval mapping (MIM) function.
Results
Experiment 1
Grain yield per pot indicated a three factor interaction between day of transfer, temperature and cultivar (P = 0.002; deviation from quartic P = 0.007; Figures 1A,B). Most of the interaction was due to changes in grain number per pot (P < 0.001 for the three factor interaction; deviation from quartic P < 0.001), with some modification through partial compensatory increases in mean grain weight, particularly after some of the earlier transfers (e.g., P < 0.001 for cubic.Day × Cultivar). There were no (P > 0.05) main, or interacting effects, of temperature on ear number per pot (mean for Renesansa and Savannah = 9.2 and 9.5, respectively; S.E.D. = 0.12; 345 d.f.) or spikelet number per ear (Renesansa = 20.3, Savannah = 20.0; S.E.D. = 0.09).
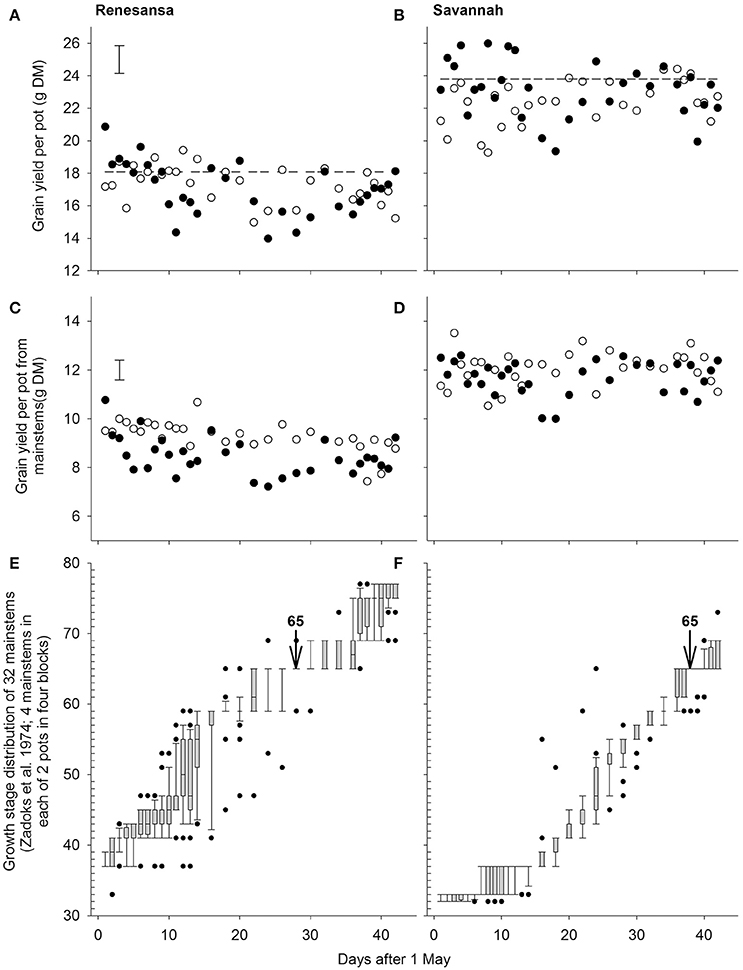
Figure 1. Effects of wheat cultivar and successive 1-day transfers to controlled environment cabinets at 20/15 (◦) and 35/30°C (•) day/night temperature (16 h day) on grain yield per pot from all stems (A,B) or only mainstems (C,D). Panels (E,F) give the growth stage distributions of the mainstems at the time of transfer in to the cabinets (boxes are limited by 25 and 75%, whiskers by 10 and 90%; points are outliers beyond 10 and 90%, and the line within the box is the median where appropriate). S.E.D. (358 d.f.) in (A,C) is for comparing temperatures within day and cultivar for both cultivars. Arrows in (E,F) denote the assumed timing of growth stage (GS) 65 (Zadoks et al., 1974). Dashed lines in (A,B) are the mean yields from eight pots per cultivar left outside.
With regards to timing of susceptibility to heat stress, the grain yields from the main stems provided better clarity than the yields from the whole plot, presumably because of the broader spectrum of the growth stages deriving from the tillers (Jones et al., 2017) and as growth stage assessments focussed primarily on main stems. On the main stems, yields of Renesansa appeared to be repeatedly compromised by day transfers to the higher temperature from 6 to 12 May, and again from 22 to 30 May (Figure 1C). In Savannah there was a significant period of susceptibility from the 17 to 21 May, and possibly a second period from 4 to 9 June (Figure 1D). Variation in growth stage amongst mainstems appeared to be greater for Renesansa (Figure 1E) than for Savannah (Figure 1F). Nonetheless, on average, for much of the period of transfers, the growth stage development of Savannah appeared to be about 10 days later than that for Renesansa. This difference could be identified with accuracy at mid anthesis as over 80% of mainstems were scored as at GS 65 on 28 May for Renesansa and on 7 June for Savannah.
When Day of transfer was expressed as relative to GS 65, there was strong evidence for two peak timings of susceptibility, but there was no evidence that timing of the peaks for susceptibility varied for the two cultivars, or that the standard deviation of the two peaks varied (Gaussian s). With regards to grain numbers on the mainstem (Table 2; Figure 2), a first peak was centered about 18 days before GS 65 when 50% of Renesansa mainstems were at GS 43–45, and 50% of Savannah mainstems were at GS 41–43 (Figure 1). Both cultivars appeared comparatively tolerant of the heat stress during late booting and ear emergence. A second period of susceptibility, however, was detected during late ear emergence and early phases of anthesis, centered on 3 days before GS 65 (Table 2; Figure 2), when most of the ears would have been at GS 61. Grain set in Renesansa appeared equally susceptible to the heat stress during booting and anthesis (Table 2; Figure 2). Grain set in Savannah was significantly more susceptible during booting than at anthesis, but the only time when grain set was significantly compensated by increased mean grain weight was at the earlier timing (Table 2; Figure 2). There was no statistical evidence for compensation for grain set failure through mean grain weight by Renesansa during either period of susceptibility.
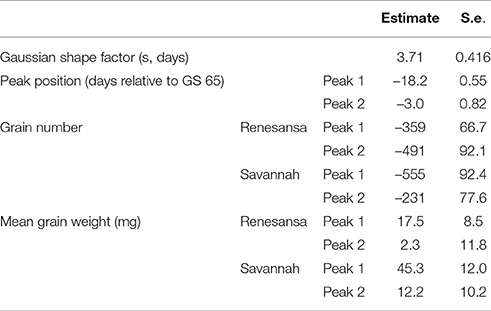
Table 2. Parameter values for simultaneous double Gaussian fit (Figure 2) to the effects of increasing day temperature from 20 to 35°C over successive single days for grain yield components on main stems of two cultivars of winter wheat.
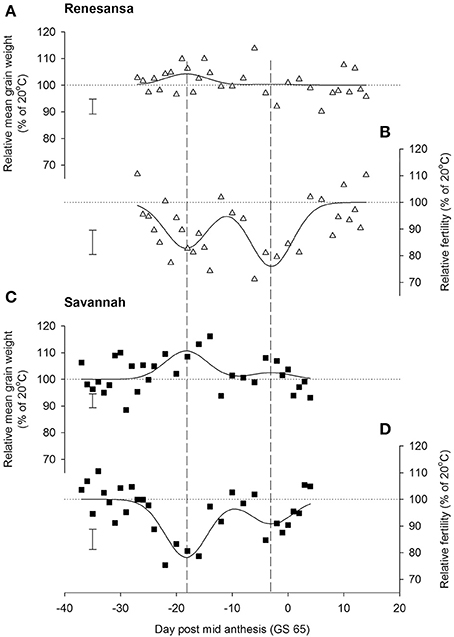
Figure 2. Effects of increasing day temperature from 20 to 35°C in successive 1-day transfers to controlled environment cabinets on mean grain weight (A,C) and grain numbers (B,D) from main stems of winter wheat, cvs Renesansa (triangles) and Savannah (squares). Fits are double Gaussian (Table 1) constrained for peaks to have the same shape (Gaussian S, Equation 1) and timings for the different components and varieties. Error bars are 1 S.E.D. (358 d.f.) for comparison of individual points with the y = 100 line.
Experiment 2
There was a significant interaction between the time of transfer and temperature on mainstem grain number (P = 0.005 for Temperature × quadratic Day). As in Experiment 1, a significant reduction in grain numbers from the main stems resulted from a day transfer to 35/30°C rather than 20/15°C, 18 days before mid anthesis (GS 65; Figure 3), whilst the plants were in the early to mid-stages of booting (c. GS 43). There were smaller reductions in grain numbers following heat stress during late ear-emergence and early anthesis, commensurate with the effects on grain numbers of Savannah at similar timings in Experiment 1. Plants appeared tolerant of the higher temperature at the start of booting (c. GS 40) and by mid anthesis (GS 65). There was no statistical evidence in Experiment 2 that reductions in grain numbers were mitigated by increases in mean grain weight; neither was there any evidence that Rht8 influenced tolerance to heat stress during booting or anthesis (P = 0.997 for Temperature × Day × Genotype on mainstem grain numbers).
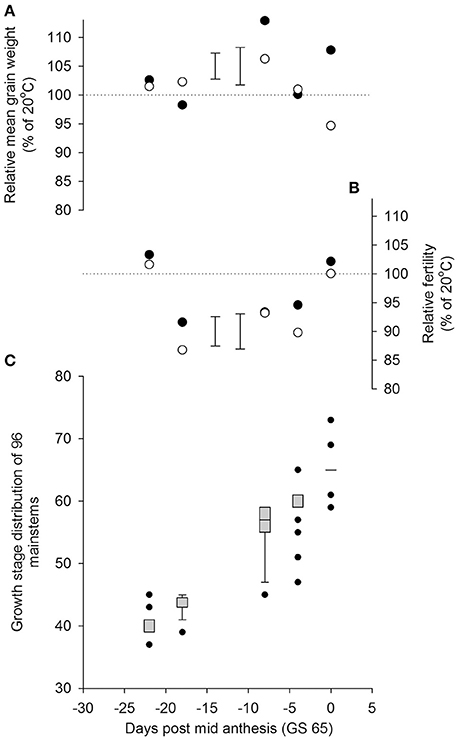
Figure 3. Effects of increasing day temperature from 20 to 35°C in 1-day transfers to controlled environment cabinets on yield components per pot from main stems of near isogenic lines with (•) and without (◦) Rht8 in a Paragon wheat background. Error bars in (A,B) are S.E.D.s for comparing points without (left) and with (right) Rht8 with the 100% line. Box-whisker plots (Figure 1 for description) in (C) show growth stage distributions of mainstems on day of transfer.
Experiment 3
Within the doubled haploid population, when using the “target” growth stages for transfer as a fixed effect there was a very highly significant interaction (P < 0.001) between temperature, growth stage, and DH line for grain number. When making some allowance for actual growth stages within target stress timings, there was evidence of increasing susceptibility from GS 37 to 41 (Figure 4D) and from GS 59 to 65 (Figure 4F). There was wide variation in susceptibility of lines within the doubled-haploid population, particularly at the mid-booting growth stage (Figures 4B,E). None of this variation was significantly associated with the markers for Rht8 or the 1BL/1RS translocation. At anthesis, however, main effect associations with both Rht-D1b (P < 0.001) and Ppd-D1a (P = 0.006) were significant. Rht (tall) and Ppd-D1a were associated with increased susceptibility during anthesis (Figure 4F). The QTL analysis confirmed the protective nature of the Savannah alleles (Rht-D1b and Ppd-D1b), but in addition identified a further, and stronger protective QTL from Renesansa on chromosome 2A (Table 3). None of these alleles could be detected as being protective against heat stress applied during booting. There was however, a weak protective QTL from Renesansa for heat applied during early booting on 2B (nearest marker = Xgwm120; LOD = 1.85; additive effect = −3.75).
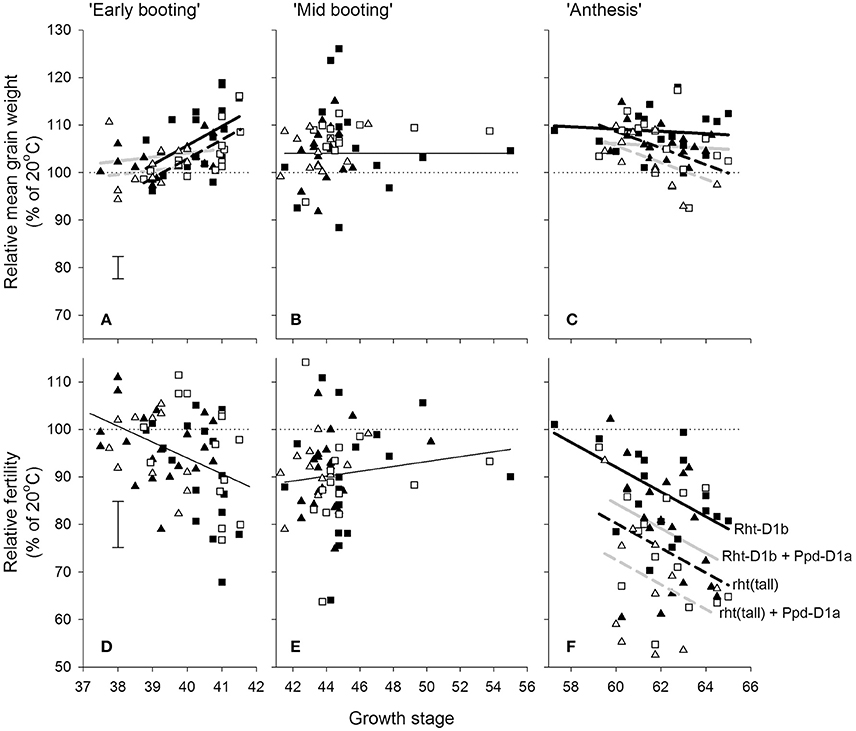
Figure 4. Effects of increasing day temperature from 20 to 35°C in 1-day transfers to controlled environment cabinets and growth stage (A,D = early booting; B,E = mid booting; C,F = Anthesis) on mean grain weight (A–C) and grain numbers (D–F) from main stems of the doubled haploid progeny of Savannah × Renesansa marked for with (solid symbols) and without (open) Rht-D1b and with (triangles) and without (squares) Ppd-D1a. Error bars are S.E.D.s for comparing any point with the 100% line. In A,C,F lines are fits corresponding to markers as described in (F): with (solid) and without (dashed) Rht-D1b; and with (light line) and without (heavy line) Ppd-D1a.
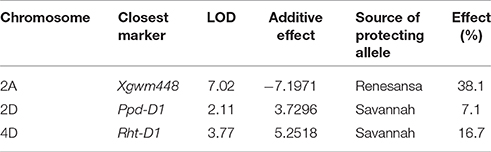
Table 3. Quantitative trait loci for relative fertility (%) in response to heat stress during anthesis (grain numbers following 1 day transfer to 35°C as a percentage of that achieved at 20°C).
In addition to effects on fertility, there was a significant three factor interaction on mean grain weight (P = 0.032). Increased mean grain weight at the higher temperature during the early stages of booting (Figure 4A) occurred in the lines not marked for Ppd-D1a, and was most evident in lines containing Rht-D1b. As anthesis progressed, the higher temperature caused progressively greater reduction in the mean grain weights of lines containing Ppd-D1a (Figure 4C).
Discussion
This study clarifies the effect of heat stress on wheat yield during reproductive development, as well as the influence of growth stage and potentially adaptive genotypic effects. We have identified two discrete periods at which grain set in wheat is susceptible to high temperature: the first in early to mid-booting presumably commensurate with susceptible meiotic stages (Barber et al., 2015) and the second during the early phases of anthesis. We have demonstrated that genotypic effects on tolerance to heat stress vary with the particular period of vulnerability.
Reductions in grain number due to heat stress caused by reduced fertility found across all experiments in this study are in agreement with previous work (Saini and Aspinall, 1982; Ferris et al., 1998; Dolferus et al., 2011; Liu et al., 2016). There is some evidence to suggest that grain size can increase and partially compensate for losses caused by abiotic stresses (Semenov et al., 2014), however this is mostly confined to the booting period of susceptibility and was not consistently observed across genotypes. Grain size increases found at booting but not at anthesis support the lack of grain size compensation found by Liu et al. (2016). This variation in compensatory increases in mean grain weight over genotype and growth stage should be accounted for when attempting to improve the response of crop models to abiotic stress (Stratonovitch and Semenov, 2015; Liu et al., 2016). Consistent with previous literature, the peak periods of susceptibility appear to be early to mid-booting (Saini and Aspinall, 1982; Alghabari et al., 2014) and early flowering (Ferris et al., 1998; Craufurd et al., 2013; Prasad and Djanaguiraman, 2014). There is some evidence to suggest that the period between meiosis and anthesis appears to be relatively tolerant to short durations of heat stress: similar to what has been observed in rice (Satake and Yoshida, 1978, 1981; Craufurd et al., 2013), with indications that this could also be true in wheat (Prasad and Djanaguiraman, 2014). Responses to heat stress are strongly influenced by genotype, as shown by variation within these experiments, especially between Savannah and Renesansa. Genotypic differences, especially at anthesis, as observed here, have been identified previously (Stone and Nicolas, 1994; Alghabari et al., 2014; Lobell et al., 2015; Liu et al., 2016). This suggests that there is potential for identifying heat tolerant traits within the current genetic diversity of wheat, which will be crucial for crop production in future climates (Godfray et al., 2010; Semenov et al., 2014).
It is necessary to acknowledge the possible confounding effects between heat stress tolerance and water deficit (Barnabas et al., 2008; Alghabari et al., 2014) in these experiments. However, the deficits below FC reported here at the end of pot transfer, and the durations over which significant deficits could have occurred, are considered to be relatively minor compared with the results from experiments with longer periods of stress (Gooding et al., 2003; Alghabari et al., 2014). Nonetheless, booting is known to be a period particularly susceptible to drought (Barber et al., 2015) and future work on identifying tolerant traits to abiotic stresses will require consideration of the combination of drought and heat stress.
There has previously been some suggestion that the semi dwarfing allele Rht8, commonly found in southern European genotypes of wheat (Worland, 1996; Gasperini et al., 2012), could also increase tolerance to heat and drought stress compared to other semi dwarfing alleles (Alghabari et al., 2014). However, our study found no effect of Rht8 on susceptibility to heat stress. This suggests that even in future climates, Rht8 would not be of benefit to northern European genotypes due to its lower yield in comparison to other semi dwarfing alleles (Rebetzke et al., 2007). Furthermore, Ppd-D1a, to which Rht8 is closely linked (Gasperini et al., 2012) was shown to increase susceptibility to heat stress. Photoperiod insensitivity caused by the allele Ppd-D1a, a mechanism used to avoid abiotic stress (Gomez et al., 2014), is widely considered to be a beneficial trait in future climates due to reducing thermal time to senescence (Barber et al., 2015), thereby avoiding late season heat and drought stress. It was also suggested by Jones et al. (2017) that the increase in flowering duration associated with Ppd-D1a would add further resilience by increasing diversity of flowering timing within a field. However, the increase in susceptibility to heat stress associated with this allele, as well as lower overall grain yield in non-stressed seasons (Addisu et al., 2010) casts doubt over the benefits that Ppd-D1a might bring under future northern European climates. Although the introduction of Rht-D1b in to Northern European wheats has increased yield through increased harvest index and reduced lodging in fertile conditions (Flintham et al., 1997), it has also been associated with some negative traits, including decreases in fertility (Law et al., 1981). Preliminary work by Law and Worland (1985) suggested that the decrease in GA sensitivity caused by Rht-D1b increases susceptibility to heat stress. This is supported by later work in other cereals, such as barley, which shows that reducing sensitivity to GA increases susceptibility to heat stress (Vettakkorumakankav et al., 1999; summary provided by Maestri et al., 2002). However, our study shows evidence to the contrary. Here, Rht-D1b was associated with greater tolerance of high temperatures at anthesis than the other alleles associated with stature. In particular, the tall allele at the Rht-D1 locus was associated with susceptibility to heat stress at anthesis. This contrasts with the effects of Rht-D1 dwarfing alleles in some, but not all, backgrounds reported by Alghabari et al. (2014). We have found no genetic explanation for the poor performance of the Northern European genotype at booting. However, this can likely be attributed to the lack of selection pressure previously on breeding programmes for this trait.
With respect to the QTL analyses, others have also found regions on chromosomes on 2A and 2B to be associated with differential responses to heat stress (Mason et al., 2010; Talukder et al., 2014). Given the strength of the protective effect associated with the QTL on 2A further investigation is warranted for alleles in the relevant region from Renesansa. What is very clear from this study is that alleles and QTL detected as being associated with heat stress tolerance is highly dependent on the precise growth stage of the plant when excessive heat is experienced.
Conclusions
In conclusion, this paper provides the strongest existing evidence that the key phases susceptible to heat stress at booting and anthesis in wheat are discrete and that genotypes vary with regards to the most susceptible growth stage. Periods of susceptibility are repeatedly observed during GS 41–45 and again from GS 61–65. In the prevailing conditions (mean daily temperature 14.3°C) periods of peak susceptibility could be separated by 15 days. We found no evidence that the southern European semi dwarfing allele Rht8 adds tolerance to heat stress within NILs or a DH population. In contrast, the north European allele Rht-D1b was associated with increased tolerance to heat stress at anthesis. The photoperiod insensitivity allele Ppd-D1a was also found to be linked to increased susceptibility to heat stress.
Author Contributions
HB, MG, and MS contributed to experimental design, HB and MG conducted analysis on the data with assistance from ML on interpretation of the data, whilst JS conducted QTL and genetic analysis. HB and MG drafted the work with revisions from MS, ML, and JS. HB, MG, MS, ML, and JS approve of the final version of the manuscript and all agree to be accountable for all aspects of the work.
Conflict of Interest Statement
The authors declare that the research was conducted in the absence of any commercial or financial relationships that could be construed as a potential conflict of interest.
Acknowledgments
John Innes Centre, Institute of Biological Environmental and Rural Sciences, and Rothamsted Research receive strategic funding from the Biotechnology and Biological Sciences Research Council (BBSRC) of the UK. Henry Barber acknowledges financial support from BBSRC DTP Grant BB/J014451/1. The authors are grateful to Mr. J. L. Hansen, Mr. L. G. Doherty, and Ms. C. J. Hadley for technical assistance with the controlled environment experiments, and to Dr. Simon Griffiths for supplying the near isogenic lines.
References
Addisu, M., Snape, J. W., Simmonds, J. R., and Gooding, M. J. (2010). Effects of reduced height (Rht) and photoperiod insensitivity (Ppd) alleles on yield of wheat in contrasting production systems. Euphytica 172, 169–181. doi: 10.1007/s10681-009-0025-2
Alghabari, F., Lukac, M., Jones, H. E., and Gooding, M. J. (2014). Effect of Rht alleles on the tolerance of wheat grain set to high temperature and drought stress during booting and anthesis. J. Agron. Crop Sci. 200, 36–45. doi: 10.1111/jac.12038
Barber, H. M., Carney, J., Alghabari, F., and Gooding, M. J. (2015). Decimal growth stages for precision wheat production in changing environments? Ann. Appl. Biol. 166, 355–371. doi: 10.1111/aab.12207
Barnabas, B., Jaeger, K., and Feher, A. (2008). The effect of drought and heat stress on reproductive processes in cereals. Plant Cell Environ. 31, 11–38. doi: 10.1111/j.1365-3040.2007.01727.x
Craufurd, P. Q., Vadez, V., Jagadish, S. V. K., Prasad, P. V. V., and Zaman-Allah, M. (2013). Crop science experiments designed to inform crop modeling. Agric. For. Meteorol. 170, 8–18. doi: 10.1016/j.agrformet.2011.09.003
Dolferus, R., Ji, X., and Richards, R. A. (2011). Abiotic stress and control of grain number in cereals. Plant Sci. 181, 331–341. doi: 10.1016/j.plantsci.2011.05.015
Ferris, R., Ellis, R. H., Wheeler, T. R., and Hadley, P. (1998). Effect of high temperature stress at anthesis on grain yield and biomass of field-grown crops of wheat. Ann. Bot. 82, 631–639. doi: 10.1006/anbo.1998.0740
Flintham, J. E., Borner, A., Worland, A. J., and Gale, M. D. (1997). Optimizing wheat grain yield: effects of Rht (giberellin-insensitive) dwarfing genes. J. Agri. Sci. Camb. 128, 11–25. doi: 10.1017/S0021859696003942
Gasperini, D., Greenland, A., Hedden, P., Dreos, R., Harwood, W., and Griffiths, S. (2012). Genetic and physiological analysis of Rht8 in bread wheat: an alternative source of semi-dwarfism with a reduced sensitivity to brassinosteroids. J. Exp. Bot. 63, 4419–4436. doi: 10.1093/jxb/ers138
Godfray, H. C. J., Beddington, J. R., Crute, I. R., Haddad, L., Lawrence, D., Muir, J. F., et al. (2010). Food security: the challenge of feeding 9 billion people. Science 327, 812–818. doi: 10.1126/science.1185383
Gomez, D., Vanzetti, L., Helguera, M., Lombardo, L., Fraschina, J., and Miralles, D. J. (2014). Effect of Vrn-1, Ppd-1 genes and earliness per se on heading time in Argentinean bread wheat cultivars. Field Crop. Res. 158, 73–81. doi: 10.1016/j.fcr.2013.12.023
Gooding, M. J., Ellis, R. H., Shewry, P. R., and Schofield, J. D. (2003). Effects of restricted water availability and increased temperature on the grain filling, drying and quality of winter wheat. J. Cereal Sci. 37, 295–309. doi: 10.1006/jcrs.2002.0501
Jones, H. E., Lukac, M., Brak, B., Martinez-Eixarch, M., Alhomedhi, A., Gooding, M. J., et al. (2017). Photoperiod sensitivity affects flowering duration in wheat. J. Agri. Sci. 155, 32–43. doi: 10.1017/S0021859616000125
Kowalski, A. M., Gooding, M., Ferrante, A., Slafer, G. A., Orford, S., Gasperini, D., et al. (2016). Agronomic assessment of the wheat semi-dwarfing gene Rht8 in contrasting nitrogen treatments and water regimes. Field Crops. Res. 191, 150–160. doi: 10.1016/j.fcr.2016.02.026
Law, C. N., Snape, J. W., and Worland, A. J. (1981). Reduced Fertility of Wheat Associated with Rht3. Plant Breeding Institute Annual Report 1980, Plant Breeding Institute, Cambridge.
Law, C. N., and Worland, A. J. (1985). An Effect of Temperature on the Fertility of Wheats Containing the Dwarfing Genes Rht1, Rht2 and Rht3. Cambridge: Plant Breeding Institute.
Liu, B., Asseng, S., Liu, L., Tang, L., Cao, W., and Zhu, Y. (2016). Testing the responses of four wheat crop models to heat stress at anthesis and grain filling. Glob. Change Biol. 22, 1890–1903. doi: 10.1111/gcb.13212
Lobell, D. B., Hammer, G. L., Chenu, K., Zheng, B., Mclean, G., and Chapman, S. C. (2015). The shifting influence of drought and heat stress for crops in northeast Australia. Glob. Change Biol. 21, 4115–4127. doi: 10.1111/gcb.13022
Lu, L. L., Wang, C. Z., Guo, H. D., and Li, Q. T. (2014). Detecting winter wheat phenology with SPOT-VEGETATION data in the North China Plain. Geocarto Int. 29, 244–255. doi: 10.1080/10106049.2012.760004
Lukac, M., Gooding, M. J., Griffiths, S., and Jones, H. E. (2012). Asynchronous flowering and within-plant flowering diversity in wheat and the implications for crop resilience to heat. Ann. Bot. 109, 843–850. doi: 10.1093/aob/mcr308
Maestri, E., Klueva, N., Perrotta, C., Gulli, M., Nguyen, H. T., and Marmiroli, N., (2002). Molecular genetics of heat tolerance and heat shock proteins in cereals. Plant Mol. Biol. 48, 667–681. doi: 10.1023/A:1014826730024
Mason, R. E., Mondal, S., Beecher, F. W., Pacheco, A., Jampala, B., Ibrahim, A. M. H., et al. (2010). QTL associated with heat susceptibility index in wheat (Triticum aestivum L.) under short-term reproductive stage heat stress. Euphytica 174, 423–436. doi: 10.1007/s10681-010-0151-x
Pradhan, G. P., Prasad, P. V. V., Fritz, A. K., Kirkham, M. B., and Gill, B. S. (2012). High temperature tolerance in aegilops species and its potential transfer to wheat. Crop Sci. 52, 292–304. doi: 10.2135/cropsci2011.04.0186
Prasad, P. V. V., and Djanaguiraman, M. (2014). Response of floret fertility and individual grain weight of wheat to high temperature stress: sensitive stages and thresholds for temperature and duration. Funct. Plant Biol. 41, 1261–1269. doi: 10.1071/FP14061
Rajaram, S. (2001). Prospects and promise of wheat breeding in the 21st century. Euphytica 119, 3–15. doi: 10.1023/A:1017538304429
Rebetzke, G. J., Richards, R. A., Fettell, N. A., Long, M., Condon, A. G., Forrester, R. I., et al. (2007). Genotypic increases in coleoptile length improves stand establishment, vigour and grain yield of deep-sown wheat. Field Crop. Res. 100, 10–23. doi: 10.1016/j.fcr.2006.05.001
Saini, H. S., and Aspinall, D. (1982). Abnormal sporogenesis in wheat (Triticum-Aestivum L) induced by short periods of high-temperature. Ann. Bot. 49, 835–846.
Saini, H. S., Sedgley, M., and Aspinall, D. (1983). Effect of heat-stress during floral development on pollen-tube growth and ovary anatomy in wheat (Triticum-Aestivum-L). Aust. J. Plant Physiol. 10, 137–144. doi: 10.1071/PP9830137
Saini, H. S., Sedgley, M., and Aspinall, D. (1984). Developmental anatomy in wheat of male-sterility induced by heat-stress, water deficit or abscisic-acid. Aust. J. Plant Physiol. 11, 243–253. doi: 10.1071/PP9840243
Satake, T., and Yoshida, H. (1978). High temperature-induced sterility in indica rices at flowering. Jpn. J. Crop Sci. 47, 6–17. doi: 10.1626/jcs.47.6
Schlegel, R., and Korzun, V. (1997). About the origin of 1RS.1BL wheat-rye chromosome translocations from Germany. Plant Breed. 116, 537–540. doi: 10.1111/j.1439-0523.1997.tb02186.x
Semenov, M. A., Stratonovitch, P., Alghabari, F., and Gooding, M. J. (2014). Adapting wheat in Europe for climate change. J. Cereal Sci. 59, 245–256. doi: 10.1016/j.jcs.2014.01.006
Shewry, P. R., and Hey, S. J. (2015). The contribution of wheat to human diet and health. Food Energy Secur. 4, 178–202. doi: 10.1002/fes3.64
Simmonds, J. R., Leverington-Waite, M., Wang, Y., Greenland, A., and Snape, J. W. (2006). Discovering QTL Controlling Yield and Yield Components in Wheat., John Innes Centre. Norwich: European Cereals Genetics Cooperative.
Snape, J. W., Foulkes, M. J., Simmonds, J., Leverington, M., Fish, L. J., Wang, Y., et al. (2007). Dissecting gene × environmental effects on wheat yields via QTL and physiological analysis. Euphytica 154, 401–408. doi: 10.1007/s10681-006-9208-2
Steinmeyer, F. T., Lukac, M., Reynolds, M. P., and Jones, H. E. (2013). Quantifying the relationship between temperature regulation in the ear and floret development stage in wheat (Triticum aestivum L.) under heat and drought stress. Funct. Plant Biol. 40, 700–707. doi: 10.1071/FP12362
Stone, P. J., and Nicolas, M. E. (1994). Wheat cultivars vary widely in their responses of grain yield and quality to short periods of post-anthesis heat stess. Aust. J. Plant Physiol. 21, 887–900. doi: 10.1071/PP9940887
Stratonovitch, P., and Semenov, M. A. (2015). Heat tolerance around flowering in wheat identified as a key trait for increased yield potential in Europe under climate change. J. Exp. Bot. 66, 3599–3609. doi: 10.1093/jxb/erv070
Talukder, S. K., Babar, M. A., Vijayalakshmi, K., Poland, J., Prasad, P. V. V., Bowden, R., et al. (2014). Mapping QTL for the traits associated with heat tolerance in wheat (Triticum aestivum.L.). BMC Genet. 15:97 doi: 10.1186/s12863-014-0097-4
Tashiro, T., and Wardlaw, I. F. (1990). The response to high-temperature shock and humidity changes prior to and during the early stages of grain development in wheat. Aust. J. Plant Physiol. 17, 551–561. doi: 10.1071/PP9900551
Vettakkorumakankav, N. N., Falk, D., Saxena, P., and Fletcher, R. A. (1999). A crucial role for gibberellins in stress protection of plants. Plant Cell Physiol. 40, 542–548. doi: 10.1093/oxfordjournals.pcp.a029575
Villareal, R. L., Banuelos, O., Mujeeb-Kazi, A., and Rajaram, S. (1998). Agronomic performance of chromosomes 1B and T1BL.1RS near-isolines in the spring bread wheat Seri M82. Euphytica 103, 195–202. doi: 10.1023/A:1018392002909
Wardlaw, I. F., Dawson, I. A., Munibi, P., and Fewster, R. (1989). The tolerance of wheat to high temperatures during reproductive growth. 1 survey procedures and general response patterns. Aust. J. Agric. Res. 1, 1–13. doi: 10.1071/AR9890001
Worland, A. J. (1996). The influence of flowering time genes on environmental adaptability in European wheats. Euphytica 89, 49–57. doi: 10.1007/BF00015718
Worland, A. J., Borner, A., Korzun, V., Li, W. M., Petrovic, S., and Sayers, E. J. (1998). The influence of photoperiod genes on the adaptability of European winter wheats (Reprinted from Wheat: prospects for global improvement, 1998). Euphytica 100, 385–394. doi: 10.1023/A:1018327700985
Keywords: heat stress, meiosis, anthesis, Ppd-D1, Rht, wheat
Citation: Barber HM, Lukac M, Simmonds J, Semenov MA and Gooding MJ (2017) Temporally and Genetically Discrete Periods of Wheat Sensitivity to High Temperature. Front. Plant Sci. 8:51. doi: 10.3389/fpls.2017.00051
Received: 10 May 2016; Accepted: 10 January 2017;
Published: 25 January 2017.
Edited by:
Soren K. Rasmussen, University of Copenhagen, DenmarkReviewed by:
Robert John French, Department of Agriculture and Food, AustraliaMuhammad Zahid Ihsan, King Abdulaziz University, Saudi Arabia
Copyright © 2017 Barber, Lukac, Simmonds, Semenov and Gooding. This is an open-access article distributed under the terms of the Creative Commons Attribution License (CC BY). The use, distribution or reproduction in other forums is permitted, provided the original author(s) or licensor are credited and that the original publication in this journal is cited, in accordance with accepted academic practice. No use, distribution or reproduction is permitted which does not comply with these terms.
*Correspondence: Henry M. Barber, aG1iYXJiZXIyOEBnbWFpbC5jb20=