- 1College of Food Science and Technology, Nanjing Agricultural University, Nanjing, China
- 2College of Biological and Environmental Sciences, Zhejiang Wanli University, Ningbo, China
Proanthocyanidins (PAs) are distributed widely in Chinese bayberry fruit and have been associated with human health benefits, but molecular and biochemical characterization of PA biosynthesis remains unclear. Here, two genes encoding key PA biosynthetic enzymes, anthocyanidin reductase (ANR) and leucoanthocyanidin reductase (LAR) were isolated in bayberry fruit. MrANR was highly expressed at the early stage of fruit development when soluble PAs accumulated at high levels. Meanwhile, the transcript abundance of both MrANR and MrLAR observed at the late stage was paralleled with the high amounts of insoluble PAs. LC-MS/MS showed that PAs in developing Chinese bayberry fruits were comprised predominantly of epigallocatechin-3-O-gallate terminal subunits, while the extension subunits were a mixture of epigallocatechin-3-O-gallate, epigallocatechin and catechin. Recombinant MrANR protein converted cyanidin to a mixture of epicatechin and catechin, and delphinidin to a mixture of epigallocatechin and gallocatechin in vitro. Recombinant MrLAR was active with leucocyanidin as substrate to produce catechin. Ectopic expression of MrANR in tobacco reduced anthocyanin levels but increased PA accumulation. The catechin and epicatechin contents in transgenic flowers overexpressed MrANR were significantly higher than those of wild-type. However, overexpression of MrLAR in tobacco led to an increase in catechin levels but had no impact on PA contents. Quantitative real time PCR revealed that the loss of anthocyanin in transgenic flowers overexpressed MrANR or MrLAR is probably attributed to decreased expression of tobacco chalcone isomerase (CHI) gene. Our results not only reveal in vivo and in vitro functions for ANR and LAR but also provide a resource for understanding the mechanism of PA biosynthesis in Chinese bayberry fruit.
Introduction
Several different classes of metabolites are synthesized via the flavonoid pathway, including anthocyanins, proanthocyanidins (PAs) and flavonols, which occur in a wide range of plants and play important roles during plant development (Winkel-Shirley, 2001). PAs, also known as condensed tannins, are involved in plant defenses against pathogens and herbivores due to their ability to bind proteins and metal ions (de Colmenares et al., 1998; Dixon et al., 2005). It is well documented that PAs can affect flavor and astringency in fruits, fruit juices, tea, or wine and protect ruminants from potentially pasture bloat (McMahon et al., 2000; Aron and Kennedy, 2008). Furthermore, PAs are beneficial to human health by protecting against cardiovascular disease, cancer establishment and progression, and bacterial infections (Bagchi et al., 2000; Cos et al., 2004; Dixon et al., 2005).
PAs are colorless flavonoid polymers formed by polymerization of flavan-3-ol units and are synthesized via the pathway leading to the synthesis of anthocyanins (Figure 1, Lepiniec et al., 2006). The key enzymes involved in PAs biosynthesis are leucoanthocyanidin reductase (LAR) and anthocyanidin reductase (ANR), which act in the production of 2,3-trans-flavan-3-ols [(+)-afzelechin, (+)-catechin, and (+)-gallocatechin] and 2,3-cis-flavan-3-ols [(−)-epiafzelechin, (−)-epicatechin, and (−)-epigallocatechin], respectively (Tanner et al., 2003; Xie et al., 2003). Although 2,3-trans- and 2,3-cis-flavan-3-ols only differ by the stereochemical configuration at the C2 and C3 positions, they can be identified by HPLC and LC-MS/MS (Zifkin et al., 2012; Ferraro et al., 2014). Quite commonly, these flavan-3-ol monomers can be esterified with gallic acid. Some gallate ester flavan-3-ols, such as (−)-epicatechin-gallate and (−)-epigallocatechin-gallate have been shown to be major phenolic compounds in persimmon (Gu et al., 2008), tea (Molan et al., 2009), and grape (Xie and Dixon, 2005). Both ANR and LAR genes have been characterized from several plant species, except for Arabidopsis, which lacks an intact LAR orthologue. In Arabidopsis, expression of the ANR gene is limited to the seed coat (Devic et al., 1999), while in many other plants such as grape (Bogs et al., 2005), Medicago (Pang et al., 2007), and cacao (Liu et al., 2013), expression of both ANR and LAR genes was observed in a range of different tissues including seeds, leaves, and flowers. In addition, it was reported that overexpression of cacao TcANR in Arabidopsis banyuls mutants which accumulate anthocyanins, instead of colorless proanthocyanidin precursors complemented the PA deficient phenotype of the seed coat (Liu et al., 2013). Ectopic expression of LAR genes from Medicago (Pang et al., 2007) or cacao (Liu et al., 2013) and ANR genes from apple (Han et al., 2012) or grape (Bogs et al., 2005) in tobacco petals resulted in accumulation of PAs but reduced anthocyanidin levels. However, a loss of PA accumulation was also observed in flowers of transgenic tobacco lines overexpressing the apple MdLAR1 gene (Liao et al., 2015).
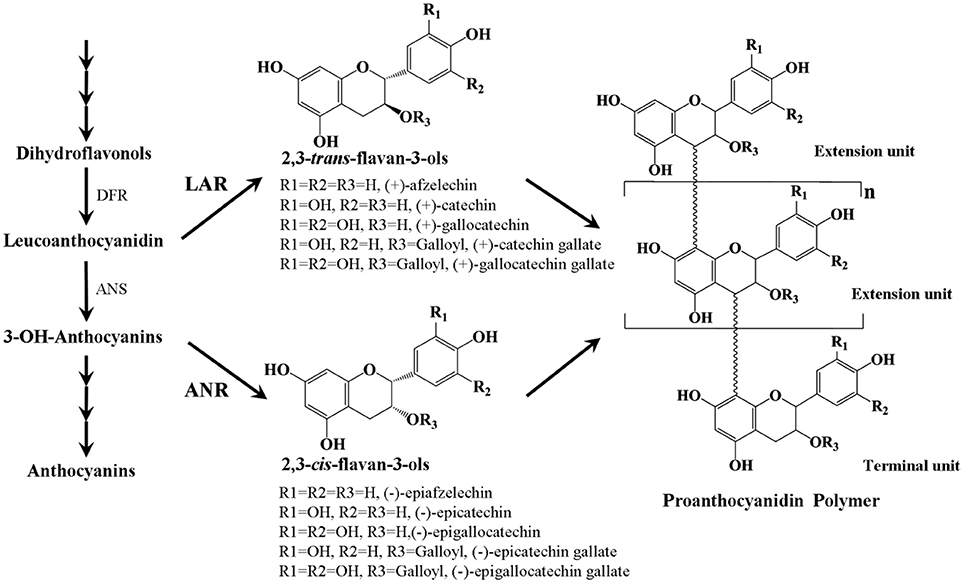
Figure 1. Scheme of the proanthocyanidin synthesis pathway. DFR, dihydroflavonal 4-reductase; ANS, anthocyanidin synthase; LAR, leucoanthocyanidin reductase; ANR, anthocyanidin reductase.
Chinese bayberry (Myrica rubra Sieb. et Zucc.) is an important subtropical fruit crop, which is originated in eastern Asia, mainly in China. Recently, it has been received much attention due to its high levels of flavonoids, which is believed to provide benefits to human health (Bao et al., 2005). Since fruits are important edible source of PAs, many studies have focused on the PA synthesis of fruits, such as grape (Bogs et al., 2005), apple (Han et al., 2012; Liao et al., 2015), persimmon (Akagi et al., 2009), blueberry (Zifkin et al., 2012), and strawberry (Almeida et al., 2007). In these fruits, both ANR and LAR genes contribute to PA synthesis and the accumulation of PAs varies in different species and at different developmental stages of the fruit. Recently, PAs in Chinese bayberry leaves were first qualitatively analyzed by Fu et al. (2014). However, the PAs accumulation and biosynthesis in Chinese bayberry fruit is still unknown.
In this study, we measured PA content and chemically characterized the PAs in Chinese bayberry fruit during ripening. The expression profiles and functional characterization of MrANR and MrLAR in relation to PA biosynthesis were investigated. The results presented here further our understanding of the molecular mechanism of the PA biosynthesis in Chinese bayberry.
Materials and Methods
Plant Material
Bayberries (Myrica rubra Sieb. and Zucc. cv. Biqi) used in this study were obtained from five adult trees cultivated in a homogeneous orchard in Cixi, Zhejiang Province. Fruit were hand-harvested at five developmental stages from 57 to 113 days after flower bloom (DAFB) with an interval of 2 weeks. At each stage, 15 fruit picked from each bayberry trees were collected. All samples were transported to the laboratory within 1 h and the fruit flesh of each whole fruit were immediately frozen in liquid nitrogen, ground to a fine powder and then stored at −81°C until analyzed.
Tobacco plants (Nicotiana tabacum var. Samsun) were cultured in a greenhouse at 25°C under 16 h/8 h light-dark illumination.
Analysis for PA Contents and Composition
For extraction of PAs, approximately 0.2–0.5 g of grounded tissues (bayberry fruit flesh or tobacco petals) were extracted with 5 mL of extraction solution (70% acetone: 29.5% water: 0.5% acetic acid) by vortexing for 30 s followed by sonication at 30°C for 15 min. After centrifuging at 13,000 g for 10 min, the pellet was re-extracted twice as above. Pooled supernatants were then extracted twice with chloroform and three times with hexane.
The PA subunit composition was analyzed using LC-MS/MS following acid-catalyzed cleavage in the presence of excess phloroglucinol. For phloroglucinol degradation of the PAs, the method of Cavallini et al. (2015) was used with some modifications. In brief, a 400 μL aliquot of supernatant was freeze-dried overnight and dissolved in 100 μL of reagent solution [0.25 g of phloroglucinol, 0.05 g of ascorbic acid, and 5 mL of acidified methanol (0.2 N HCl)]. The mixture was heated at 50°C for 100 min and then 100 μL of sodium acetate buffer (200 mM; pH 7.5) was added to quench the reaction. After centrifugation for 15 min at 13,000 g, 20 μL of supernatant was run on a Waters ACQUITY UPLC system (Milford, MA, USA) equipped with an ACQUITY UPLC photodiode array detector (Waters). Molecules were separated on a Sunfire C18 column (4.6 × 150 mm, 5 μm particle size) with a pre-column of the same material (4.6 × 10 mm, 4 μm particle size) (Waters) at 25°C and at a flow rate of 0.7 mL min−1. Elution was performed with 0.2% (v/v) aqueous formic acid (solvent A) and methanol with 0.2% formic acid (v/v; solvent B), using the following elution program: initially 5% B for 4 min; 67% B at 25 min; 90% B at 25.5 min; and isocratic at 90% B from 25.5 to 30 min. All phloroglucinol adducts of flavan-3-ols and flavan-3-ol terminal units were monitored at 280 nm. Mass spectrometry was carried out using a Waters Q-TOF Synapt G2 mass spectrometer. The mass spectrometer conditions used were as follows: capillary voltage, 2200 V; cone voltage, 40 V; extractor voltage, 4 V; source temperature, 105°C; desolvation temperature 350°C; cone gas flow (N2), 50 L h−1 and desolvation gas flow (N2), 800 L h−1. The spectra ranging from m/z 50 to 1,200 were taken in the negative mode. The energies for collision-induced dissociation were 20–40 V for fragmentation. The data were accumulated for 0.2 s per cycle and calculated using the MassLynx 4.1 software (Waters). PA terminal subunits levels were calculated by comparison with commercial standards. The concentration of extension subunit-phloroglucinol adducts was determined using published molar extinction coefficients (Kennedy and Jones, 2001; Zhang et al., 2016). All compounds were expressed as catechin equivalents. The mean degree of polymerization (mDP) was calculated from the molar ratio of all subunits (terminal and extension subunits) to terminal units.
Total soluble PA content was determined spectrophoto-metrically after reaction with dimethylaminocinnamaldehyde (DMACA) reagent (0.2% [w/v] DMACA in methanol-3 N HCl [1:1]) at 640 nm (Pang et al., 2007). The residues from the above tissue extractions were used for insoluble PAs analysis using the method of Pang et al. (2007). Total soluble PA or insoluble PA levels were calculated as procyanidin B2 equivalents.
Anthocyanin Analysis
The total anthocyanin content of tobacco petals was determined according to the method described by our group (Shi et al., 2014), which involves measuring the absorbance (510 and 700 nm) of extracts that have been diluted with pH 1.0 and 4.5 buffers.
RNA Extraction and cDNA Preparation
Total RNA was isolated from bayberry fruit flesh and tobacco petals using a Plant RNA Kit (Omega, Norcross, GA) according to the manufacturer's instructions. DNA contamination was removed from RNA preparations using RNase-free DNase I (Omega, Norcross, GA). Approximately 2 μg of total RNA was reverse-transcribed with the SuperRT First Strand cDNA Synthesis Kit (CWBIO, Beijing, China), following the manufacturer's instructions.
Real-Time Quantitative RT-PCR
Real-time Quantitative RT-PCR (qRT-PCR) analysis was performed on a Bio-Rad CFX96 Real-Time PCR System (Bio-Rad, Hercules, CA, USA) using LightCycler 480 SYBR Green Master (Roche, Shanghai, China) following the manufacturer's instructions. Each reaction contained 0.5 μL of template cDNA, 0.5 μL of 10 mM forward and reverse primers, 6.25 μL of SYBR Green PCR Master Mix, and 4.75 μL of RNase-free water in a total volume of 12.5 μL. The transcript levels were normalized to MrACT (GenBank accession no. GQ340770) to minimize variation in cDNA template levels. All gene expression analyses were performed with three independent biological replicates, and primer sequences used for qRT-PCR are listed in Table S1.
Cloning of MrANR and MrLAR
Partial sequences of MrANR and MrLAR were isolated using degenerate primers (Table S1) designed from conserved regions of corresponding genes reported from other species by the CODEHOP strategy (Rose et al., 1998). The 5′ and 3′ ends of the coding sequence were obtained by rapid amplification of cDNA ends (RACE) using the SMART RACE cDNA amplification kit (Clontech, Mountain View, CA, USA). PCR fragments were subsequently cloned into pMD18-T vector (Takara, Japan) and sequenced. The complete open reading frames (ORFs) of MrANR and MrLAR were amplified from bayberry (Biqi) cDNA derived from fruit sampled at 113 DAFB PCR products were then ligated into the pMD18-T vector (Takara, Japan) and subjected to DNA sequencing. The primers used are shown in Table S1.
Expression and Purification of Recombinant MrANR and MrLAR
The ORFs of MrANR and MrLAR were amplified from Biqi fruit (113 DAFB). The PCR product was purified and subcloned into the BamHI and SalI sites of the pET32a expression vector (Novagen, Gibbstown, NJ, USA). After confirmation by sequencing, the recombinant vectors were introduced into Escherichia coli BL21 (TransGen, Beijing, China). The bacteria were grown in Luria-Bertani ampicillin (100 μg mL−1) medium at 37°C overnight until the OD600 reached 0.6, at which point 1 mM IPTG (isopropyl β-D-1-thiogalactopyranoside) was added to induce protein expression at 120 rpm for 48 h at 16°C. The recombinant proteins with a 6 × His tag at the N terminus were purified with the His-tagged protein purification kit (CWBIO, Beijing, China), according to the manufacturer's instructions. Purity was then checked by SDS-PAGE.
Assay of ANR and LAR Activities
3,4-cis-Leucocyanidin was prepared by reduction of (+)-dihydroquercetin with sodium borohydride, modified from the method of Tanner and Kristiansen (1993). Briefly, a solution containing 2 mg (+)-dihydroquercetin in 250 μL of dry ethanol was added to 0.2 mg of solid sodium borohydride and incubated at 20°C for 2 h. After that, 5 ml of 0.1% (v/v) acetic acid was added into the mixture and incubation for 4 h at 40°C. The epimerization was halted by freezing in liquid nitrogen after 3 h and lyophilization. LAR activity for production of catechin from 3,4-cis-leucocyanidin was determined in a final volume of 200 μL containing 100 mM Tris-HCl (pH 7.5), 1 mM NADPH, 0.1 mM 3,4-cis-leucocyanidin, and 1 mg of recombinant MrLAR protein. For assay of recombinant MrANR with cyanidin or delphinidin as substrate, reaction mixtures (200 μL) contained 100 mM Tris-HCl (pH 6.0), 1 mM NADPH, 0.1 mM cyanidin or delphinidin, and 1 mg of recombinant MrANR protein. After incubation at 30°C for 30 min, the reaction was terminated by extracting twice with 400 μL ethyl acetate, vortexing for 1 min, and centrifuging for 1 min. The solvent was evaporated, and the organic fraction was resuspended in 200 μL of methanol and then subjected to LC-MS/MS analysis. Crude protein extract from induced BL21 containing empty vector was used as a control.
LC-MS/MS analysis of enzymatic products was performed using a Waters ACQUITY UPLC system (Milford, MA, USA) equipped with an ACQUITY UPLC HSS T3 column (2.1 × 100 mm, 1.8 μm particle size; Waters) at a flow rate of 0.2 mL min−1 and a column temperature of 25°C. The mobile phase consisted of 0.2% acetic acid (solvent A) and methanol (solvent B). The gradient conditions were: 0 min, 5% B; 15 min, 70% B; 15.2 min, 95% B; 16.2 min, 95% B and 16.4 min, 5% B. The mass spectrometer conditions were the same as for the PA subunit composition analysis.
Transformation of Tobacco
The coding sequences of MrANR and MrLAR were amplified by PCR, and cloned into the pCAMBIA-1300 binary vector (CAMBIA, Canberra, Australia) using the BamHI and SalI sites. The resulting binary vectors were transferred into Agrobacterium tumefaciens strain EHA105 by electroporation. Agrobacterium-mediated transformation in tobacco was carried out using the leaf disk method (Horsch et al., 1989). Transgenic plants were selected on Murashige and Skoog medium supplemented with 25 mg /L hygromycin.
Results
Molecular Cloning and Sequence Analysis of MrANR and MrLAR Genes
A full-length cDNA of MrANR was isolated by 5′- and 3′-RACE; the cDNA was 1355 bp long and had an ORF of 1014 bp encoding a 337-amino acids protein. A BLASTP search revealed that the deduced amino acid sequence was 82% identical with ANRs from Vitis vinifera (VvANR), Theobroma cacao (TcANR), or Malus domestica (MdANR). Multiple sequence alignment (Figure S1) showed that MrANR contained a Rossmann dinucleotide-binding domain (motif GXGXXA) (Bottoms et al., 2002), which is conserved among ANR proteins (Greco et al., 2012). The calculated pI and molecular mass of the deduced MrANR protein were 6.36 and 36 kDa, respectively.
The MrLAR cDNA was 1531 bp in length and contained an ORF of 1083 bp encoding a protein of 360 amino acids with a predicted pI of 6.06 and a molecular mass of 39 kDa. MrLAR at the amino acid level showed 77% identity to CsLAR (Camellia sinensis), 72% to TcLAR (Theobroma cacao), and 70% to VvLAR (Vitis vinifera). The MrLAR protein contained the LAR characteristic amino acid motifs such as RFLP, ICCN, and THD (Tanner et al., 2003; Bogs et al., 2005; Figure S2).
Expression of MrANR and MrLAR During Fruit Development
The transcript abundance of MrANR was at a high level at 57 DAFB, then declined at 71 DAFB followed by an increase toward to maturity (Figure 2A). Expression of MrLAR remained relatively low from 57 to 99 DAFB, but a significant increase was observed at 113 DAFB (Figure 2A).
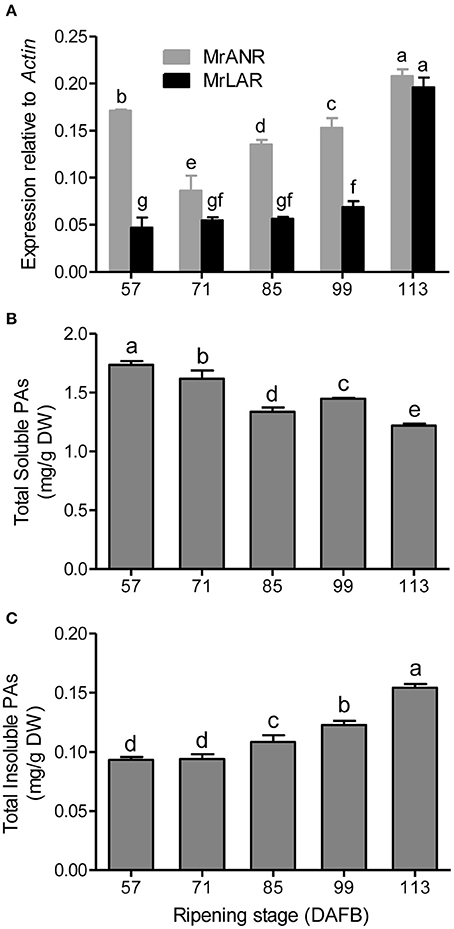
Figure 2. PA accumulation and gene expression of MrANR and MrLAR during ripening in Chinese bayberry fruit. (A) Relative transcript levels of of MrANR and MrLAR. Levels of soluble PAs (B) and insoluble PAs (C). Different letters above the bars indicate significant differences among different stages (P < 0.05 by Duncan's multiple range test).
PA Accumulation in Developing Bayberry Fruit
To understand temporal PA accumulation in Chinese bayberry fruit, levels of total soluble and insoluble PAs at different developmental stages were measured (Figures 2B,C). The concentration of soluble PAs was highest in fruit at 57 DAFB, and declined thereafter. No significant changes in the levels of insoluble PAs were observed at the early developmental stages (57 to 71 DAFB) but the content increased to a maximum at 113 DAFB.
To further analysis the structure of Chinese bayberry fruit PAs, the soluble PA oligomers was subsequently subjected to acid-catalyzed cleavage in the presence of phloroglucinol. The released phloroglucinol adducts (extension units) and terminal flavan-3-ol units were then identified and quantified by LC-MS/MS (Table S2 and Figures 3A,B). The PA extension subunits were consisted mostly of epigallocatechin-3-O-gallate (EGCG), epigallocatechin (EGC) and catechin (C), with much lower levels of epicatechin-3-O-gallate (ECG) units. The concentration of EGCG, EGC and ECG extension subunits steadily decreased until fruit maturation, while a small increase in catechin occurred at 99 DAFB (Figure 3A). For flavan-3-ols, EGCG was the predominant terminal subunit detected in fruit at all stages (78–88%) and displayed a decline in content over development (Figure 3B). Minor amounts of ECG, EGC and epicatechin (EC) were also detected in the PA terminal subunits, but only the EGC content decreased during ripening (Figure 3B). In developing bayberries, the mDP of the PA polymers was around 4.5 (Figure 3C).
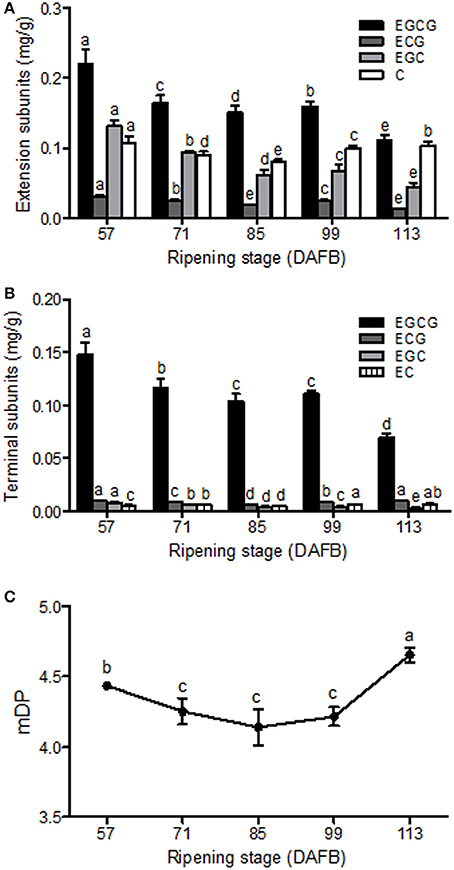
Figure 3. PA composition in developing Chinese bayberry fruit. Accumulation and composition of PA extension subunits (A) and terminal subunits (B). EGCG, epigallocatechin-3-O-gallate; ECG, epicatechin-3-O-gallate; EGC, epigallocatechin; EC, epicatechin; C, catechin. (C) Mean degree of polymerization of PAs in developing bayberry fruit from 57 to 113 DAFB. Different letters above the bars indicate significant differences among different stages (P < 0.05 by Duncan's multiple range test).
Functional Analysis of Recombinant MrANR and MrLAR in vitro
To determine the catalytic activity of MrANR, the MrANR ORF was subcloned into the bacterial expression vector pET32a, expressed in Escherichia coli and purified. Denaturing gel electrophoresis revealed that the recombinant MrANR protein had a molecular mass of approximately 56 kDa, which most likely corresponded to the 20 kDa of Trx.Tag-His.Tag-S.Tag sequences (Kurup et al., 2000) fused to the expected 36 kDa MrANR gene product (Figure 4A). Based on the bayberry PA subunit composition data, cyanidin and delphinidin were the primary in planta substrates for formation of EC and EGC as well as their gallate esters, ECG and EGCG, respectively. However, no epiafzelechin (EAZ; with pelargonidin as a substrate) was detected in Chinese bayberry fruit. Therefore, recombinant MrANR enzyme was assayed using cyanidin and delphinidin as substrates. When the enzymatic products were analyzed by LC-MS/MS, their chromatograms and ions spectra were identical with those of the corresponding authentic cis-flavan-3-ol standards, EC and EGC (Figures 4B,C, 5A,B and Figure S3A). Interestingly, the trans-flavan-3-ol products with the same chromatographic and MS/MS patterns as C and gallocatechin (GC) were also detected when MrANR protein was incubated with cyanidin and delphinidin, respectively. No products were generated in incubations with protein from the vector control (Figures 4D, 5C).
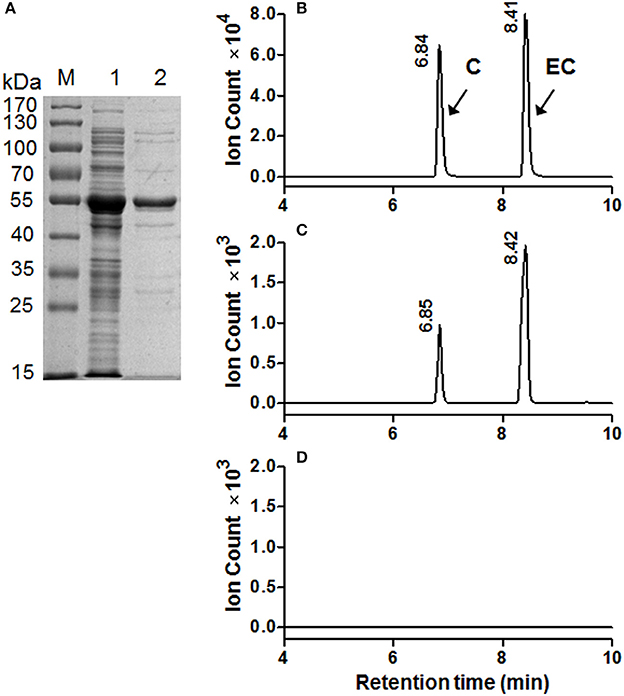
Figure 4. Enzymatic assay of recombinant MrANR using cyanidin as a substrate. (A) Analysis of MrANR protein on a 12.5% SDS-PAGE gel. M, Molecular weight marker; 1, crude protein from E. coli BL21 (DE3) harboring pET32α-MrANR induced by IPTG; 2, purified recombinant MrANR protein. (B–D): LC-MS [M-H]− extracted ion chromatographs (m/z = 289) of authentic (+)-catechin (C) and (−)-epicatechin (EC) (B) along with the enzymatic products of purified recombinant MrANR protein (C) or protein extract from vector control (D).
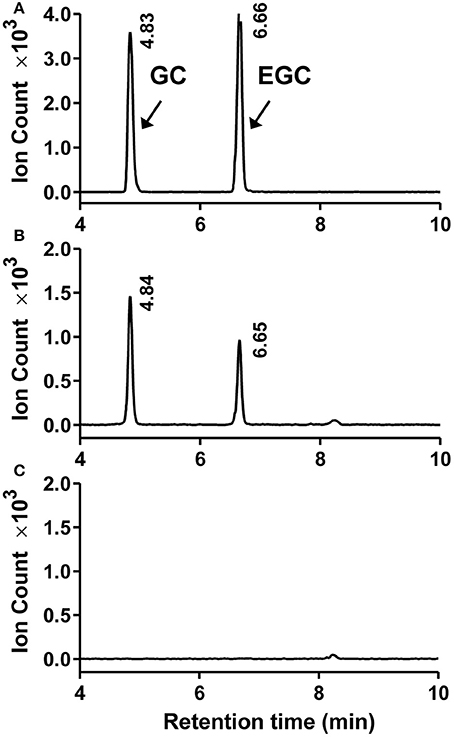
Figure 5. Enzymatic assay of recombinant MrANR using delphinidin as a substrate. (A–C). LC-MS [M-H]− extracted ion chromatographs (m/z = 305) of authentic (+)-gallocatechin (GC) and (−)-epigallocatechin (EGC) (A) along with the enzymatic products of purified recombinant MrANR protein (B) or protein extract from vector control (C).
The MrLAR ORF was expressed as an N-terminal 6 × His tagged recombinant protein in Escherichia coli. The purified recombinant protein showed a single protein band of around 59 kDa, higher than its predicted molecular mass (39 kDa), which can be ascribed to its N-terminal fusion of Trx.Tag-His.Tag-S.Tag sequences (about 20 kDa) (Figure 6A). Because C was the predominant trans-flavan-3-ol in Chinese bayberry fruits, the MrLAR catalytic activity for the conversion of leucocyanidin to C was analyzed by LC-MS/MS in comparison to the authentic standards. The LC-MS/MS chromatograms and ions spectra confirmed the formation of C, whereas no EC product was detected (the retention time of 8.41 min) (Figures 6B,C and Figure S3B). Control incubations with protein from the vector control gave no conversion of leucocyanidin (Figure 6D).
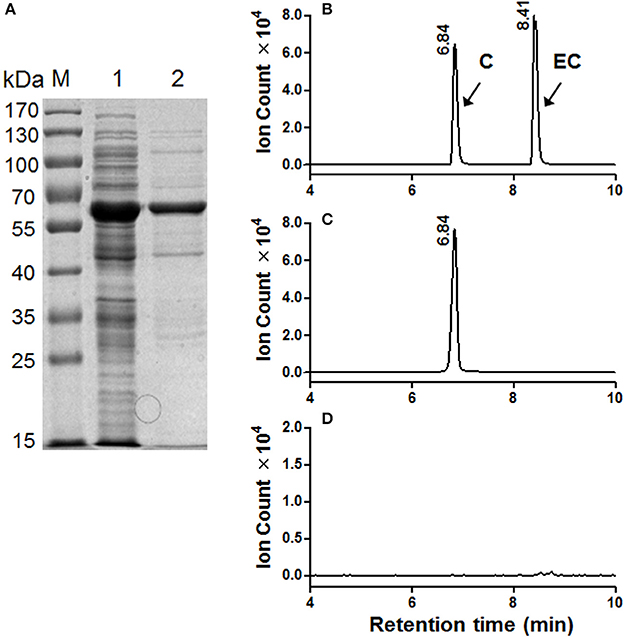
Figure 6. Enzymatic assay of recombinant MrLAR using leucocyanidin as a substrate. (A) Analysis of MrLAR protein on a 12.5% SDS-PAGE gel. M, Molecular weight marker; 1, crude protein from E. coli BL21 (DE3) harboring pET32α-MrLAR induced by IPTG; 2, purified recombinant MrLAR protein. (B–D). LC-MS [M-H]− extracted ion chromatographs (m/z = 289) of authentic (+)-catechin (C) and (−)-epicatechin (EC) (B) along with the enzymatic products of purified recombinant MrLAR protein (C) or protein extract from vector control (D).
Ectopic Expression of MrANR in Tobacco
To characterize the in vivo function of MrANR, its ORF was ectopically expressed in tobacco (cv. Samsun) driven by the cauliflower mosaic virus (CaMV) 35S promoter. From 25 independent hygromycin-resistant transgenic tobacco lines, nine plants exhibited a decrease in pink color intensity in flower petals. Three independent lines (Lines A2, A3, and A11) displaying virtually white petals were selected for further analysis (Figure 7A and Figure S4A). The qRT-PCR analysis confirmed extremely high levels of MrANR transcripts in these three lines (Figure 7B). Quantification of anthocyanin and soluble PA levels indicated that anthocyanin levels of all three lines were significantly lower than those of wild-type (WT) flowers. On the contrary, their soluble PA contents were significantly higher (Figures 7C,D). Insoluble PA levels were also determined, but with no significant difference between WT and transgenic plants (Figure S5A). Thus, in these lines, an inverse relationship between the level of anthocyanins and PAs was detected. The structure of PAs in the transgenic tobacco lines was further confirmed by using phloroglucinolysis and LC-MS/MS analyses (Table 1 and Figure S6A). Compared with the WT, overexpression of MrANR in tobacco resulted in a significant increase in the concentration of all PA subunits, including catechin-terminal units and epicatechin-terminal and extension units. Moreover, an increased mDP ranging from 13.2 to 16.4 subunits was also observed in the overexpressing lines (Table 1).
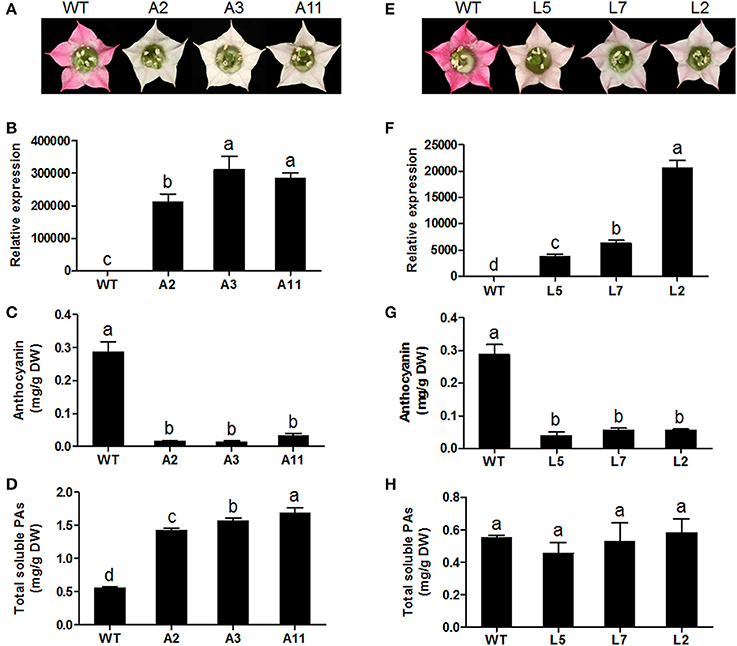
Figure 7. Overexpression of MrANR and MrLAR in tobacco. (A) Flower color of wild type (WT) and three independent lines of MrANR transgenic (A2, A3 and A11,) tobacco plants. (B) Expression profiles of MrANR in transgenic flowers. (C) Anthocyanin levels in flowers of WT and MrANR transgenic plants. (D) Total soluble PAs levels in flowers of WT and MrANR transgenic plants. (E) Flower color of wild type (WT) and three independent lines of MrLAR transgenic (L5, L7 and L2) tobacco plants. (F) Expression proflles of MrLAR in flowers of transgenic tobacco lines. (G) Anthocyanin levels in flowers of plants shown in (E). (H), Total soluble PAs levels in flowers of of plants shown in (E). All data are presented as mean ± SD of three replicate reactions. Different letters above the bars indicate significant difference between wild-type and transgenic plants (P < 0.05 by Duncan's multiple range test).
QRT-PCR analysis was conducted to elucidate the effect of ectopic expression of MrANR on flavonoids pathway in tobacco flowers. The results showed that overexpressing MrANR strongly affected expression of flavonoid structural genes in transgenic tobacco flowers (Figure 8A). Most of the structural genes, including NtLAR, NtANR2, NtF3H, NtF3'H, NtDFR, NtANS, NtUFGT, and NtFLS, were up-regulated in flowers of all three MrANR overexpression lines. However, transcripts of NtCHI in the three lines were significantly lower than in WT.
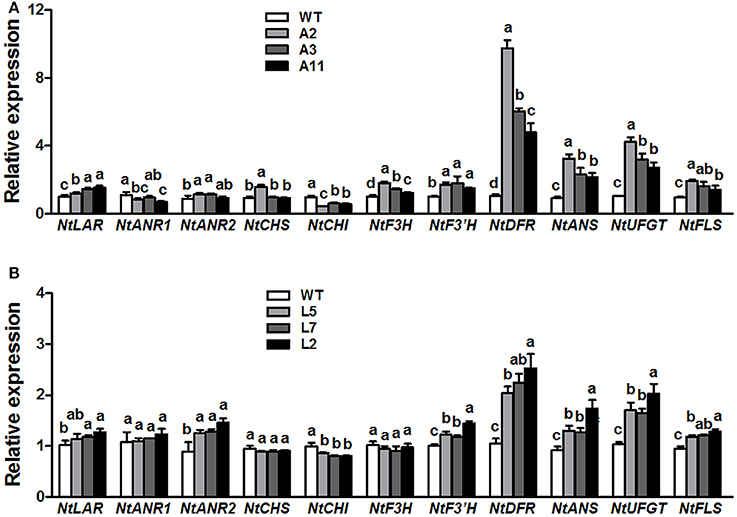
Figure 8. Expression profiles of flavonoid-related structural biosynthetic genes in flowers of MrANR (A) and MrLAR (B) transgenic tobacco lines. All data are presented as mean ± SD of three replicate reactions. Different letters above the bars indicate significant difference between wild-type and transgenic plants (P < 0.05 by Duncan's multiple range test).
Ectopic Expression of MrLAR in Tobacco
Thirty independent hygromycin-resistant transgenic tobacco lines were generated after introduced with the ORF of MrLAR. Among 10 plants with visibly decreased color, three lines (Lines L5, L7 and L2) with the greatest decrease in petal color (Figure 7E and Figure S4B) and high levels of MrLAR transcripts (Figure 7F) were selected for further analysis. Compared with WT, these three lines exhibited a strong reduction in anthocyanin content (Figure 7G). Surprisingly, no significant difference in soluble nor insoluble PA levels was observed between MrLAR transgenic plants and WT flowers (Figure 7H and Figure S5B). However, all of L5, L7 and L2 lines accumulated higher levels of catechin-terminal units known as the predicted product of MrLAR, and epicatechin-terminal units but lower epicatechin-extension units than WT (Table 2 and Figure S6B). Moreover, the mDP was about 2 to 5 times lower in these transgenic lines than that in WT (Table 2). All three transgenic plants displayed significantly higher transcript abundance of most flavonoid structural genes, including NtANR2, NtF3'H, NtDFR, NtANS, NtUFGT, and NtFLS but lower NtCHI as compared to WT (Figure 8B). In vivo genetic analysis also showed that overexpression of MrANR and MrLAR led to accumulation of PAs in tobacco leaves, but changes in total soluble PAs and expression of PA-related genes in leaves of transgenic lines compared to wild type were much less marked than those in flowers (Figure S7).
Discussion
PAs, the products of the flavonoid pathway, are abundant in many fruits, with a strong impact on their taste and health benefits. PA concentration has been found to decrease in the progression of ripening in a variety of fruits, such as bilberry (Jaakola et al., 2002), grape (Bogs et al., 2005), and strawberry (Carbone et al., 2009). Interestingly, soluble PAs in Chinese bayberry fruit also declined as the fruit matured while an increase in insoluble PAs levels was observed in the late stages of fruit development (Figures 2B,C), which suggested that the PAs in bayberries are initially soluble (potentially in the cell vacuole) and are subsequently insolubilized (presumably in the cell wall) (Pang et al., 2007). ANR and LAR genes are known to encode the key enzymes involved in PA biosynthesis, and both exhibit down-regulation pattern during ripening in many fruits, which is consistent with the decrease in soluble PA levels (Jaakola et al., 2002; Bogs et al., 2005; Carbone et al., 2009). In present study, high transcript level of MrANR was observed at early stages of fruit development, which was correlated with the early accumulation of soluble PAs, indicating the possibility of the early involvement of MrANR in PA accumulation in bayberries. In contrast, the up-regulated expression of both MrANR and MrLAR genes during the later stages of ripening was well associated with the increase in insoluble PA content. Therefore, these results suggested that both MrANR and MrLAR may play an important role in PA synthesis with a temporal-specific manner in Chinese bayberry fruit.
The soluble PA oligomers in Chinese bayberry fruit were demonstrated to contain EGCG as the predominant terminal subunit, while EGCG, EGC and C were the major components of the extension subunit (Figures 3A,B). This result is agreeable with previous studies showing that PAs in Chinese bayberry leaves were composed of EGCG as their terminal subunit and most of their extension subunits (Yang et al., 2011; Fu et al., 2014). In contrast, the PAs of grapes, blueberry, strawberry, and other berries contain a high proportion of EGC, whereas galloylated EGC (EGCG) are undetectable (Cohen et al., 2012; Huang et al., 2012). The presence of galloyl groups was reported to potentially enhance the antioxidative activity of PAs (Aron and Kennedy, 2008). ANR has been characterized as producing cis-flavan-3-ols (e.g., EGCG or EGC) in a number of species (Xie et al., 2003; Bogs et al., 2005; Akagi et al., 2009), while LAR producing trans-flavan-3-ols (e.g., C) (Tanner et al., 2003). Our enzymatic analysis showed that recombinant MrANR protein use cyanidin and delphinidin as substrates to produce corresponding EC and EGC. For LAR activity, in vitro experiments suggested that the LAR enzymes characterized from Chinese bayberry efficiently catalyze the production of C from leucocyanidin (Figure 6). Therefore, in this study, the reduction of soluble PA in bayberries was due to the decline in all cis-flavan-3-ol terminal and extension subunits during the earlier stages of bayberry development, which was closely related to the expression pattern of MrANR. On the contrary, the increase in C extension subunits in late stages was correlated with the up-regulated expression of MrLAR. Intriguingly, in addition to EC and EGC, the MrANR protein in our study also produced C and GC from the substrates of cyanidin and delphinidin, respectively, suggesting that the MrANR protein may have an epimerase activity in vitro. Such epimerase activity inherently associated with ANR has been reported in Gossypium hirsutum (Zhu et al., 2015), Vitis bellula (Zhu et al., 2014), Vitis vinifere (Gargouri et al., 2010) and Camellia sinensis (Pang et al., 2013), producing cis-flavan-3-ols in vitro as well as trans-flavan-3-ols.
The functionality of MrANR and MrLAR was further investigated by ectopic expression in tobacco. Over-expression of MrANR in tobacco led to a reduction of anthocyanidin level but an increase in C-terminal unit including EC-terminal and extension unit. Our results were similar to previous reports that over-expression of the ANR gene in tobacco diverted the metabolic flow from anthocyanin to PA synthesis (Xie et al., 2003; Liu et al., 2013; Pang et al., 2013). Likewise, ectopic expression of MrLAR resulted in a decrease in the levels of anthocyanin pigments in tobacco flowers as well. Surprisingly, no significant difference in PA levels was observed between MrLAR transgenic plants and WT flowers, which were probably associated with the elevated levels of both C and EC terminal units together with a corresponding decrease of EC-extension unit in transgenic tobacco flowers compared to WT (Table 2). Similar results were also found in PAP1-expressing tobacco when introduced with tea LAR gene (Pang et al., 2013). PA accumulation was even reduced in transgenic tobacco overexpressed the MdLAR1 gene (Liao et al., 2015). Furthermore, the opposite effects of MrANR and MrLAR overexpression on EC-extension unit accumulation seemed to cause the adverse change in mDP between transgenic and WT plants, with mDP being increased in MrANR transgenic tobacco but decreased in MrLAR transgenic tobacco. Taken together, these results suggested that the ectopic expression of MrANR or MrLAR could affect the PA properties in tobacco including PA concentration, composition and polymerization.
CHI is a key enzyme involved in anthocyanin metabolism and silencing of CHI gene in tobacco is highly effective in reduction of anthocyanin accumulation (Nishihara et al., 2005). It was reported that overexpression of CsANR, MdANR or MdLAR1 in tobacco suppressed expression of CHI gene in flowers, resulting in a loss of anthocyanin (Han et al., 2012; Kumar and Yadav, 2012; Liao et al., 2015). Consistently, ectopic expression of MrANR and MrLAR in present study also inhibited expression of CHI gene in tobacco flowers, which were related to the decrease in anthocyanin accumulation. In contrast, the expression of multiple other flavonoid structural genes, such as NtF3'H, NtDFR, NtANS, NtUFGT, and NtFLS, was up-regulated in flowers of transgenic plants carrying either MrANR or MrLAR (Figure 8). The mechanism by which these genes regulated the changes of anthocyanin and PA accumulation in transgenic tobacco is still unclear, but similar results were also reported in previous findings. For example, the increased transcript levels of NtF3'H and NtUFGT genes were observed in flowers of transgenic tobacco plants overexpressed MdANR1 or MdANR2a (Han et al., 2012). Overexpression of CsANR enhanced the expression levels of NtFLS (Kumar and Yadav, 2012).
In summary, we successfully isolated two genes encoding key PA biosynthetic enzymes in Chinese bayberry fruit, MrANR and MrLAR. Their expression pattern coincided with the change of soluble and insoluble PA accumulation in developing bayberry fruit. In vitro enzyme assays of MrANR and MrLAR recombinant protein verified their predicted function in PA biosynthesis. Moreover, in vivo genetic analysis of MrANR in tobacco demonstrated its role in promoting the PA biosynthesis pathway. Although overexpression of MrLAR in tobacco was unable to affect the total PA contents, an increase in catechin levels was observed in flowers of transgenic plants. Therefore, we concluded that MrANR and MrLAR were responsible for the biosynthesis of flavan-3-ol monomers in Chinese bayberry fruit to some extent. In plants, PAs are colorless flavonoid polymers formed by polymerization of flavan-3-ol units with sequential addition of extension subunits to a flavan-3-ol terminal unit. It has been reported recently that excessive 4β-(S-cysteinyl)-epicatechin, which is a carbocation form, played an important role in non-enzymatic polymerization and served as a PA extension unit (Liu et al., 2016). LARs could catalyze it to form EC in vitro and consequently inhibited the polymerization of PAs (Liu et al., 2016; Wang et al., 2018). Thus, the biosynthesis of carbocation and its effect on PA polymerization in bayberries should be investigated further to reveal the true role of MrLAR.
Author Contributions
ZY and YZ conceived and designed the study. LS, SC, XC, and WC performed experiments, analyzed and interpreted the data, and wrote the manuscript. LS, SC, ZY, and YZ revised the manuscript critically. All authors read and approved the final manuscript.
Conflict of Interest Statement
The authors declare that the research was conducted in the absence of any commercial or financial relationships that could be construed as a potential conflict of interest.
Acknowledgments
This study was supported by the National Natural Science Foundation of China (31671898 and 31471632) and the Innovation Research Foundation from Zhejiang Top Key Discipline of Bioengineering (BK2012306).
Supplementary Material
The Supplementary Material for this article can be found online at: https://www.frontiersin.org/articles/10.3389/fpls.2018.00212/full#supplementary-material
References
Akagi, T., Ikegami, A., Suzuki, Y., Yoshida, J., Yamada, M., Sato, A., et al. (2009). Expression balances of structural genes in shikimate and flavonoid biosynthesis cause a difference in proanthocyanidin accumulation in persimmon (Diospyros kaki Thunb.) fruit. Planta 230, 899–915. doi: 10.1007/s00425-009-0991-6
Almeida, J. R., D'amico, E., Preuss, A., Carbone, F., De Vos, C. H., Deiml, B., et al. (2007). Characterization of major enzymes and genes involved in flavonoid and proanthocyanidin biosynthesis during fruit development in strawberry (Fragaria × ananassa). Arch. Biochem. Biophys. 465, 61–71. doi: 10.1016/j.abb.2007.04.040
Aron, P. M., and Kennedy, J. A. (2008). Flavan-3-ols: nature, occurrence and biological activity. Mol. Nutr. Food Res. 52, 79–104. doi: 10.1002/mnfr.200700137
Bagchi, D., Bagchi, M., Stohs, S. J., Das, D. K., Ray, S. D., Kuszynski, C. A., et al. (2000). Free radicals and grape seed proanthocyanidin extract: importance in human health and disease prevention. Toxicology 148, 187–197. doi: 10.1016/S0300-483X(00)00210-9
Bao, J., Cai, Y., Sun, M., Wang, G., and Corke, H. (2005). Anthocyanins, flavonols, and free radical scavenging activity of Chinese bayberry (Myrica rubra) extracts and their color properties and stability. J. Agric. Food Chem. 53, 2327–2332. doi: 10.1021/jf048312z
Bogs, J., Downey, M. O., Harvey, J. S., Ashton, A. R., Tanner, G. J., and Robinson, S. P. (2005). Proanthocyanidin synthesis and expression of genes encoding leucoanthocyanidin reductase and anthocyanidin reductase in developing grape berries and grapevine leaves. Plant Physiol. 139, 652–663. doi: 10.1104/pp.105.064238
Bottoms, C. A., Smith, P. E., and Tanner, J. J. (2002). A structurally conserved water molecule in Rossmann dinucleotide-binding domains. Protein Sci. 11, 2125–2137. doi: 10.1110/ps.0213502
Carbone, F., Preuss, A., De Vos, R. C., D'Amico, E., Perrotta, G., Bovy, A. G., et al. (2009). Developmental, genetic and environmental factors affect the expression of flavonoid genes, enzymes and metabolites in strawberry fruits. Plant Cell Environ. 32, 1117–1131. doi: 10.1111/j.1365-3040.2009.01994.x
Cavallini, E., Matus, J. T., Finezzo, L., Zenoni, S., Loyola, R., Guzzo, F., et al. (2015). The phenylpropanoid pathway is controlled at different branches by a set of R2R3-MYB C2 repressors in grapevine. Plant Physiol. 167, 1448–1470. doi: 10.1104/pp.114.256172
Cohen, S. D., Tarara, J. M., Gambetta, G. A., Matthews, M. A., and Kennedy, J. A. (2012). Impact of diurnal temperature variation on grape berry development, proanthocyanidin accumulation, and the expression of flavonoid pathway genes. J. Exp. Bot. 63, 2655–2665. doi: 10.1093/jxb/err449
Cos, P., De Bruyne, T., Hermans, N., Apers, S., Berghe, D. V., and Vlietinck, A. J. (2004). Proanthocyanidins in health care: current and new trends. Curr. Med. Chem. 11, 1345–1359. doi: 10.2174/0929867043365288
de Colmenares, N. G., Ramírez-Martínez, J. R., Aldana, J. O., Ramos-Ni-o, M. E., Clifford, M. N., Pékerar, S., et al. (1998). Isolation, characterisation and determination of biological activity of coffee proanthocyanidins. J. Sci. Food Agric. 77, 368–372. doi: 10.1002/(SICI)1097-0010(199807)77:3<368::AID-JSFA52>3.0.CO;2-V
Devic, M., Guilleminot, J., Debeaujon, I., Bechtold, N., Bensaude, E., Koornneef, M., et al. (1999). The BANYULS gene encodes a DFR-like protein and is a marker of early seed coat development. Plant J. 19, 387–398. doi: 10.1046/j.1365-313X.1999.00529.x
Dixon, R. A., Xie, D. Y., and Sharma, S. B. (2005). Proanthocyanidins–a final frontier in flavonoid research? New Phytol. 165, 9–28. doi: 10.1111/j.1469-8137.2004.01217.x
Ferraro, K., Jin, A. L., Nguyen, T. D., Reinecke, D. M., Ozga, J. A., and Ro, D. K. (2014). Characterization of proanthocyanidin metabolism in pea (Pisum sativum) seeds. BMC Plant Biol. 14:238. doi: 10.1186/s12870-014-0238-y
Fu, Y., Qiao, L., Cao, Y., Zhou, X., Liu, Y., and Ye, X. (2014). Structural elucidation and antioxidant activities of proanthocyanidins from Chinese bayberry (Myrica rubra Sieb. et Zucc.) leaves. PLoS ONE 9:e96162. doi: 10.1371/journal.pone.0096162
Gargouri, M., Chaudière, J., Manigand, C., Maugé, C., Bathany, K., Schmitter, J. M., et al. (2010). The epimerase activity of anthocyanidin reductase from Vitis vinifera and its regiospecific hydride transfers. Biol. Chem. 391, 219–227. doi: 10.1515/bc.2010.015
Greco, M., Chiappetta, A., Bruno, L., and Bitonti, M. B. (2012). In Posidonia oceanica cadmium induces changes in DNA methylation and chromatin patterning. J. Exp. Bot. 63, 695–709. doi: 10.1093/jxb/err313
Gu, H. F., Li, C. M., Xu, Y. J., Hu, W. F., Chen, M. H., and Wan, Q. H. (2008). Structural features and antioxidant activity of tannin from persimmon pulp. Food Res. Int. 41, 208–217. doi: 10.1016/j.foodres.2007.11.011
Han, Y., Vimolmangkang, S., Soria-Guerra, R. E., and Korban, S. S. (2012). Introduction of apple ANR genes into tobacco inhibits expression of both CHI and DFR genes in flowers, leading to loss of anthocyanin. J. Exp. Bot. 63, 2437–2447. doi: 10.1093/jxb/err415
Horsch, R. B., Fry, J., Hoffmann, N., Neidermeyer, J., Rogers, S. G., and Fraley, R. T. (1989). “Leaf disc transformation” in Plant Molecular Biology Manual, eds S. B. Gelvin, R. A Schilperoort, and D. P. S. Verma (Dordrecht: Kluwer Academic Press), 1–9.
Huang, W. Y., Zhang, H. C., Liu, W. X., and Li, C. Y. (2012). Survey of antioxidant capacity and phenolic composition of blueberry, blackberry, and strawberry in Nanjing. J. Zhejiang Univ. Sci. B 13, 94–102. doi: 10.1631/jzus.B1100137
Jaakola, L., Määtta, K., Pirttilä, A. M., Törrönen, R., Kärenlampi, S., and Hohtola, A. (2002). Expression of genes involved in anthocyanin biosynthesis in relation to anthocyanin, proanthocyanidin, and flavonol levels during bilberry fruit development. Plant Physiol. 130, 729–739. doi: 10.1104/pp.006957
Kennedy, J. A., and Jones, G. P. (2001). Analysis of proanthocyanidin cleavage products following acid-catalysis in the presence of excess phloroglucinol. J. Agr. Food Chem. 49, 1740–1746. doi: 10.1021/jf001030o
Kumar, V., and Yadav, S. K. (2012). Overexpression of CsANR increased flavan-3-ols and decreased anthocyanins in transgenic tobacco. Mol. Biotechnol. 54, 426–435. doi: 10.1007/s12033-012-9580-1
Kurup, S., Jones, H. D., and Holdsworth, M. J. (2000). Interactions of the developmental regulator ABI3 with proteins identified from developing Arabidopsis seeds. Plant J. 21, 143–155. doi: 10.1046/j.1365-313x.2000.00663.x
Lepiniec, L., Debeaujon, I., Routaboul, J. M., Baudry, A., Pourcel, L., Nesi, N., et al. (2006). Genetics and biochemistry of seed Xavonids. Annu. Rev. Plant Biol. 57, 405–430. doi: 10.1146/annurev.arplant.57.032905.105252
Liao, L., Vimolmangkang, S., Wei, G., Zhou, H., Korban, S. S., and Han, Y. (2015). Molecular characterization of genes encoding leucoanthocyanidin reductase involved in proanthocyanidin biosynthesis in apple. Front. Plant Sci. 6:243. doi: 10.3389/fpls.2015.00243
Liu, C., Wang, X., Shulaev, V., and Dixon, R. A. (2016). A role for leucoanthocyanidin reductase in the extension of proanthocyanidins. Nat. Plants 2:16182. doi: 10.1038/nplants.2016.182
Liu, Y., Shi, Z., Maximova, S., Payne, M. J., and Guiltinan, M. J. (2013). Proanthocyanidin synthesis in theobroma cacao: genes encoding anthocyanidin synthase, anthocyanidin reductase, and leucoanthocyanidin reductase. BMC Plant Biol. 13:202. doi: 10.1186/1471-2229-13-202
McMahon, L. R., McAllister, T. A., Berg, B. P., Majak, W., Acharya, S. N., Popp, J. D., et al. (2000). A review of the effects of forage condensed tannins on ruminal fermentation and bloat in grazing cattle. Can. J. Plant Sci. 80, 469–485. doi: 10.4141/P99-050
Molan, A. L., De, S., and Meagher, L. (2009). Antioxidant activity and polyphenol content of green tea flavan-3-ols and oligomeric proanthocyanidins. Int. J. Food Sci. Nutr. 60, 497–506. doi: 10.1080/09637480701781490
Nishihara, M., Nakatsuka, T., and Yamamura, S. (2005). Flavonoid components and flower color change in transgenic tobacco plants by suppression of chalcone isomerase gene. FEBS Lett. 579, 6074–6078. doi: 10.1016/j.febslet.2005.09.073
Pang, Y., Abeysinghe, I. S., He, J., He, X., Huhman, D., Mewan, K. M., et al. (2013). Functional characterization of proanthocyanidin pathway enzymes from tea and their application for metabolic engineering. Plant Physiol. 161, 1103–1116. doi: 10.1104/pp.112.212050
Pang, Y., Peel, G. J., Wright, E., Wang, Z., and Dixon, R. A. (2007). Early steps in proanthocyanidin biosynthesis in the model legume Medicago truncatula. Plant Physiol. 145, 601–615. doi: 10.1104/pp.107.107326
Rose, T. M., Schultz, E. R., Henikoff, J. G., Pietrokovski, S., McCallum, C. M., and Henikoff, S. (1998). Consensus-degenerate hybrid oligonucleotide primers for amplification of distantly related sequences. Nucleic Acids Res. 26, 1628–1635. doi: 10.1093/nar/26.7.1628
Shi, L., Cao, S., Shao, J., Chen, W., Zheng, Y., Jiang, Y., et al. (2014). Relationship between sucrose metabolism and anthocyanin biosynthesis during ripening in Chinese bayberry fruit. J. Agric. Food Chem. 62, 10522–10528. doi: 10.1021/jf503317k
Tanner, G. J., Francki, K. T., Abrahams, S., Watson, J. M., Larkin, P. J., and Ashton, A. R. (2003). Proanthocyanidin biosynthesis in plants purification of legume leucoanthocyanidin reductase and molecular cloning of its cDNA. J. Biol. Chem. 278, 31647–31656. doi: 10.1074/jbc.M302783200
Tanner, G. J., and Kristiansen, K. N. (1993). Synthesis of 3, 4-cis-[3H] leucocyanidin and enzymatic reduction to catechin. Anal. Biochem. 209, 274–277. doi: 10.1006/abio.1993.1119
Wang, P., Zhang, L., Jiang, X., Dai, X., Xu, L., Li, T., et al. (2018). Evolutionary and functional characterization of leucoanthocyanidin reductases from Camellia sinensis. Planta 247, 139–154. doi: 10.1007/s00425-017-2771-z
Winkel-Shirley, B. (2001). Flavonoid biosynthesis. A colorful model for genetics, biochemistry, cell biology, and biotechnology. Plant Physiol. 126, 485–493. doi: 10.1104/pp.126.2.485
Xie, D. Y., and Dixon, R. A. (2005). Proanthocyanidin biosynthesis–still more questions than answers? Phytochemistry 66, 2127–2144. doi: 10.1016/j.phytochem.2005.01.008
Xie, D. Y., Sharma, S. B., Paiva, N. L., Ferreira, D., and Dixon, R. A. (2003). Role of anthocyanidin reductase, encoded by BANYULS in plant flavonoid biosynthesis. Science 299, 396–399. doi: 10.1126/science.1078540
Yang, H., Ye, X., Liu, D., Chen, J., Zhang, J., Shen, Y., et al. (2011). Characterization of unusual proanthocyanidins in leaves of Bayberry (Myrica rubra Sieb. et Zucc.). J. Agric. Food Chem. 59, 1622–1629. doi: 10.1021/jf103918v
Zhang, Y., Zhou, X., Tao, W., Li, L., Wei, C., Duan, J., et al. (2016). Antioxidant and antiproliferative activities of proanthocyanidins from Chinese bayberry (Myrica rubra Sieb. et Zucc.) leaves. J. Funct. Foods 27, 645–654. doi: 10.1016/j.jff.2016.10.004
Zhu, Y., Peng, Q. Z., Li, K. G., and Xie, D. Y. (2014). Molecular cloning and functional characterization of the anthocyanidin reductase gene from Vitis bellula. Planta 240, 381–398. doi: 10.1007/s00425-014-2094-2
Zhu, Y., Wang, H., Peng, Q., Tang, Y., Xia, G., Wu, J., et al. (2015). Functional characterization of an anthocyanidin reductase gene from the fibers of upland cotton (Gossypium hirsutum). Planta 241, 1075–1089. doi: 10.1007/s00425-014-2238-4
Zifkin, M., Jin, A., Ozga, J. A., Zaharia, L. I., Schernthaner, J. P., Gesell, A., et al. (2012). Gene expression and metabolite profiling of developing highbush blueberry fruit indicates transcriptional regulation of flavonoid metabolism and activation of abscisic acid metabolism. Plant Physiol. 158, 200–224. doi: 10.1104/pp.111.180950
Keywords: Chinese bayberry, proanthocyanidin, flavan-3-ols, anthocyanidin reductase, leucoanthocyanidin reductase
Citation: Shi L, Cao S, Chen X, Chen W, Zheng Y and Yang Z (2018) Proanthocyanidin Synthesis in Chinese Bayberry (Myrica rubra Sieb. et Zucc.) Fruits. Front. Plant Sci. 9:212. doi: 10.3389/fpls.2018.00212
Received: 04 November 2017; Accepted: 05 February 2018;
Published: 28 February 2018.
Edited by:
Kevin Davies, The New Zealand Institute for Plant & Food Research Ltd, New ZealandReviewed by:
Yongzhen Pang, Institute of Botany (CAS), ChinaVinay Kumar, Central University of Punjab, India
Copyright © 2018 Shi, Cao, Chen, Chen, Zheng and Yang. This is an open-access article distributed under the terms of the Creative Commons Attribution License (CC BY). The use, distribution or reproduction in other forums is permitted, provided the original author(s) and the copyright owner are credited and that the original publication in this journal is cited, in accordance with accepted academic practice. No use, distribution or reproduction is permitted which does not comply with these terms.
*Correspondence: Yonghua Zheng, emhlbmd5aEBuamF1LmVkdS5jbg==
Zhenfeng Yang, eWFuZ3pmQHp3dS5lZHUuY24=