- Institute of Plant Biochemistry, Cluster of Excellence on Plant Sciences, Center for Synthetic Life Sciences, Heinrich Heine University Düsseldorf, Düsseldorf, Germany
The CRISPR/Cas9 system has emerged as a powerful tool for targeted genome editing in plants and beyond. Double-strand breaks induced by the Cas9 enzyme are repaired by the cell’s own repair machinery either by the non-homologous end joining pathway or by homologous recombination (HR). While the first repair mechanism results in random mutations at the double-strand break site, HR uses the genetic information from a highly homologous repair template as blueprint for repair of the break. By offering an artificial repair template, this pathway can be exploited to introduce specific changes at a site of choice in the genome. However, frequencies of double-strand break repair by HR are very low. In this study, we compared two methods that have been reported to enhance frequencies of HR in plants. The first method boosts the repair template availability through the formation of viral replicons, the second method makes use of an in planta gene targeting (IPGT) approach. Additionally, we comparatively applied a nickase instead of a nuclease for target strand priming. To allow easy, visual detection of HR events, we aimed at restoring trichome formation in a glabrous Arabidopsis mutant by repairing a defective glabrous1 gene. Using this efficient visual marker, we were able to regenerate plants repaired by HR at frequencies of 0.12% using the IPGT approach, while both approaches using viral replicons did not yield any trichome-bearing plants.
Introduction
Gene targeting (GT) means integration of foreign DNA into a cell’s genome by homologous recombination (HR) (Paszkowski et al., 1988). GT has been a long-term goal for plant scientists since it allows modifying an endogenous gene in planta or integrating a transgene at a specific position in the genome (Puchta, 2002). GT is easily achieved in lower eukaryotes, such as yeast, or the moss Physcomitrella patens, because they include foreign DNA predominantly through highly efficient HR pathways. However, GT is not easily achieved in higher plants, since they integrate foreign DNA via illegitimate recombination through the non-homologous end joining (NHEJ) pathway (Puchta, 2005). A simple introduction of a DNA donor molecule with homology arms only led to frequencies of 10-4 to 10-6 GT events per transformation event in higher plants, such as tobacco and Arabidopsis (Lee et al., 1990; Offringa et al., 1990; Miao and Lam, 1995; Risseeuw et al., 1995), making GT impractical for most applications. Nevertheless, GT frequencies in higher plants can be increased by two orders of magnitude by inducing a double strand break (DSB) in the target locus with sequence specific nuclease (SSN) (Puchta et al., 1996). SSN are proteins that can cleave DNA at predefined sequence motives. The first generation of SSN, including meganucleases, zinc finger nuclease (ZFN), and transcription activator-like effector nucleases (TALENs) recognized DNA by protein-DNA interactions, which required rational protein design for every target sequence (Voytas, 2013). The most recent addition to the SSN has been the CRISPR/Cas9 system (Cas9 system).
Cas9 is an endonuclease of the bacterium Streptococcus pyogenes that generates DSB in DNA (Doudna and Charpentier, 2014) and that recently has become the SSN of choice for genome editing and GT. Cas9 became so popular because, in contrast to other SSN that recognize DNA sequences through protein-DNA interactions, Cas9 is guided to its DNA target site by complementarity matching of a single guide RNA (sgRNA) (Jinek et al., 2012). The RNA-based targeting system makes Cas9 easily programmable toward a selected target. Therefore, the Cas9 system has been used extensively by numerous laboratories to edit plant genomes (Zhang et al., 2017). Additionally, mutation of one of the two endonuclease sites of Cas9 resulted in a Cas9 nickase, which introduces only single-strand nicks in the plant genome (Fauser et al., 2014).
Double-strand breaks can be lethal for the cell and must be repaired by dedicated protein machineries. Two major repair pathways can be distinguished: NHEJ and HR (Puchta, 2005). Most DSB in higher eukaryotes are repaired by NHEJ (Sargent et al., 1997), a pathway that involves direct re-ligation of the DSB ends (Gorbunova and Levy, 1997; Dueva and Iliakis, 2013), making it an error-prone process. This process can be exploited to introduce random small indel mutations at a DSB site and has been used extensively to knock-out gene functions with SSN (Bortesi and Fischer, 2015). In contrast, HR repair pathways use a homology template to repair a DSB and allow therefore the introduction of designated sequence information. In the conservative synthesis-dependent strand annealing pathway, which is the most prominent HR pathway in plant somatic tissues (Puchta, 1998), DNA ends are resected to create 3′ overhangs. One 3′-end can invade into a homologous sequence forming a loop-structure and copy the sequence from the homology template DNA. After release from the loop structure, the single strand can reanneal with homologous sequences on the opposite side of the DSB, leading to conservative repair without sequence information loss (Steinert et al., 2016). Artificial homology templates with homology arms have already been used for GT experiments to target foreign DNA sequences into a DSB generated by SSN (Puchta et al., 1996; Baltes et al., 2014; Schiml et al., 2014; Li et al., 2015).
While GT frequencies were successfully enhanced by cutting the target site with SSN, this is not sufficient for streamlined application of GT in plants. GT frequencies can be further enhanced up to two orders of magnitudes (compared to conventional T-DNA delivery) by mobilizing the homology template after T-DNA transformation (Baltes et al., 2014). This can be achieved with an in planta gene targeting (IPGT) approach and with a viral replicon approach.
The IPGT approach (Fauser et al., 2012) employs SSN not only to cut the target locus but also to excise the homology template from the chromosomal T-DNA backbone. The liberation from the genomic context of the T-DNA allows the activated homology template to be available at the site of the DSB where it can function as repair template. This method has been used successfully using various SSN in Arabidopsis (Fauser et al., 2012; Schiml et al., 2014; Zhao et al., 2016), maize (Ayar et al., 2013; Kumar et al., 2016), and rice (Sun et al., 2016). While the term IPGT could be used as general term for all GT approaches in plants, we use it to describe the approach to release a homology template by SSN in the context of this publication.
An alternative approach has been developed by Baltes et al. (2014) in tobacco. It activates the homology template by including it in a Geminivirus based replicon (Baltes et al., 2014). Therefore, the homology template is flanked with three viral elements from the bean yellow dwarf virus (BeYDV) that are required to establish the replicon. These regions are known as the cis-acting long intergenic region (LIR), the short intergenic region (SIR) and the gene for the trans-acting viral replicase Rep/RepA. The Rep protein initiates a rolling circle endoreplication at the LIR site, leading to a replicational release of the homology template in form of up to several thousand circular replicons (Chen et al., 2011). This method has been successfully applied using components from various virus strains and with different SSN for GT in wheat protoplast (Gil-Humanes et al., 2017), tomato (Čermák et al., 2015), potato (Butler et al., 2016), and rice (Wang et al., 2017). While IPGT has already been shown to induce stable GT events in Arabidopsis (Fauser et al., 2012; Schiml et al., 2014), reports about successful applications of viral replicons based GT approaches in Arabidopsis have not been published so far.
In this report, we aimed to evaluate and compare the efficiencies of the viral replicon system and the IPGT system to enhance GT frequencies in the model plant Arabidopsis thaliana. Therefore, we made use of the R2R3-MYB transcriptional master regulator of trichome formation GLABROUS1 (GL1), which we established previously as non-invasive, endogenous visual marker for Cas9 activity in Arabidopsis (Hahn et al., 2017). Null-mutations of the GL1 gene lead to loss of trichome formation (Koornneef et al., 1982) on stems and leaves as GL1 is part of the signaling cascade which allows epidermal cells to differentiate into trichomes (Pattanaik et al., 2014). Using the IPGT approach and the viral replicon-based approach, we aimed at repairing a non-functional gl1 gene with a 10 bp (base pair) deletion in the coding region (Hahn et al., 2017) by HR and by this, restoring trichome formation. Using IPGT, we achieved successful GT and regenerated three stable non-glabrous plants out of ∼2,500 plants screened over three generations. However, we were not able to regenerate non-glabrous plants using viral replicons. The gl1 phenotype provided a simple and efficient visual marker for tracking mutagenic activity in planta.
Materials and Methods
Plant Growth Conditions
Surface-sterilized seeds of an Arabidopsis gl1 mutant with a 10 bp deletion in the GL1 gene (Hahn et al., 2017) were stratified at 4°C for 3 days. Seeds were germinated on 0.8% (w/v) agar-solidified half-strength Murashige and Skoog medium (Duchefa) in growth chambers with a light intensity of 100 μmol photons m-2 s-1 under long day conditions (16-h-light/8-h-dark cycle, 22/18°C). After 14 days, seedlings were transferred to soil and further grown under the same conditions. For large-scale screening of plants in the T2 generation, seeds were directly sowed on soil and the plants were grown in the green house after 3 days of stratification at 4°C.
Generation of a Homology Repair Template for Reparation of the 10 Nucleotides Deletion in the gl1 Gene
We designed a homology repair template containing a codon-modified version of the missing 10 bp. The 10 bp (CTGCCGTTTA) were flanked by homology arms complementary to the genomic regions adjacent to the cutting site of Cas9 in the gl1 gene. The homology arms consisted of 813 and 830 bp, respectively. Both homology arms contained a single nucleotide exchange within the homology region to distinguish two-sided true GT events from one-sided GT events combined with NHEJ mediated repair pathways (Schiml et al., 2014). After a short linker sequence, the sequence of the sgRNA recognition site including the protospacer adjacent motif (PAM) sequence followed. The homology template was synthesized at GeneART (Thermo Fisher Scientific) and integrated in the vector backbone pMA-RQ (GeneART). The resulting vector was named pFH95.
Cloning of a Binary T-DNA Repair Vector for In Planta Gene Targeting Repair Approach
We made use of the binary T-DNA vector pDE-Cas9, containing an Arabidopsis-codon-optimized Cas9 controlled by the constitutive Ubiquitin promoter (PcUBp) from Petroselinum crispum (Fauser et al., 2014) and the sgRNA subcloning vector pEN-C.1.1 containing the Arabidopsis U6-26 promoter for sgRNA expression, the sgRNA scaffold and BbsI-sites for subcloning of the protospacer sequence (Schiml et al., 2014). After digestion of pEN-C1.1 with BbsI-HF (New England Biolabs), we inserted the target sequence (AAGAGATGTGGGAAAAGAGA plus an additional guanine as first bp for transcription by the U6-26 promoter) using two annealed primers FH210/FH211 (Supplementary Table S1) resulting in vector pFH89. We then digested pFH89 with MluI-HF (New England Biolabs) to extract the sgRNA cassette and ligated it into MluI-HF-digested pDE-Cas9, thereby creating the vector pFH99. We amplified the homology template from pFH95 using primers FH278/FH279 (Supplementary Table S1) and performed Gateway® BP-reaction with pDONR 207 (Invitrogen) resulting in vector pFH122. LR reaction (Invitrogen) of pFH99 with pFH122 led to the assembly of the IPGT repair vector pIPGT-Nuc.
Generation of Binary T-DNA Repair Vectors for Viral Replicon Repair Approach
For the viral replicon repair approach, we amplified the viral components from BeYDV necessary for replicon formation from the vector pZLSLD.R (Baltes et al., 2014) using primers FH217/FH218 (Supplementary Table S1) and cloned the PCR product into pDONR 207 (Invitrogen) via BP reaction. This vector (pFH83) was then digested with PmII (New England Biolabs) and BsaXI (New England Biolabs) to remove the original homology template from Baltes et al. (2014). We PCR-amplified the GL1 homology template from pFH95 using primers FH240/FH241 (Supplementary Table S1) and inserted it into the digested pFH83® vector by Gibson cloning (New England Biolabs), resulting in the vector pFH84. The final viral replicon nuclease repair construct pVIR-Nuc containing Cas9 nuclease, the sgRNA cassette directed against the gl1 gene and the homology template was then cloned by Gateway® LR reaction (Invitrogen) of pFH99 with pFH84.
For the viral replicon repair approach using the Cas9 nickase, we used the MluI-HF-extracted sgRNA cassette from pFH89 and ligated it into MluI-HF-digested pDE-Cas9-D10A (Fauser et al., 2014) resulting in the vector pFH103. LR reaction of pFH103 with pFH84 led to the final viral replicon nickase repair construct pVIR-Nick.
The sequences of the vectors pIPGT-Nuc (MG760355), pVIR-Nuc (MG760356) and pVIR-Nick (MG760357) are accessible on GenBank.
Plant Transformation
Agrobacterium tumefaciens, strain GV3101::pMP90 was transformed with the vectors pIPGT-Nuc, pVIR-Nuc, and pVIR-Nick. Floral dipping (Clough and Bent, 1998) was used to transform Arabidopsis gl1 plants with the three constructs. Kanamycin (pVIR-Nick) and glufosinate (pIPGT-Nuc, pVIR-Nuc) were used for selection of primary transformants. Furthermore, leaf genomic DNA was isolated from surviving plants via isopropanol precipitation (Weigel and Glazebrook, 2009). The presence of T-DNA insertion was verified by PCR using primer pairs FH190/FH223 (pIPGT-Nuc) or FH223/FH265 (pVIR-Nuc, pVIR-Nick, Supplementary Table S1).
Detection of the Cas9 Gene in Plants
Presence of the Cas9 gene in plant genomes was verified by PCR on leaf genomic DNA using primer pairs amplifying specifically the Cas9 gene (FH313/FH314, Supplementary Table S1).
Verification of Viral Replicon Formation
Formation of circular viral replicons was verified in T1 generation plants by PCR using leaf genomic DNA and the primers FH266/FH271 (Supplementary Table S1).
Detection of Cas9-Mediated Gene Repair
For verification of HDR events on genomic level, leaf genomic DNA was isolated via isopropanol precipitation (Weigel and Glazebrook, 2009) and the region of interest in the gl1 gene was PCR-amplified with proofreading Q5© polymerase (New England Biolabs) using primers FH214/FH215 (Supplementary Table S1). The obtained PCR amplicons were analyzed directly by Sanger sequencing (Macrogen) or subcloned into pJET1.2 (Thermo Fisher Scientific) and then sequenced. 4Peaks software (Nucleobytes) and SnapGene Viewer (GSL Biotech) were used to analyze sequencing histograms.
Results
Selection of a Visual Marker for Testing Gene Targeting Events
In a previous study, we established trichome formation as efficient visual marker to monitor Cas9 mutagenic activity in Arabidopsis (Hahn et al., 2017). We used a CRISPR/Cas9 approach to generate a T-DNA free glabrous Arabidopsis mutant that contains a deletion of 10 bp in the master regulator gene for trichome formation, GL1. This 10 bp deletion disrupts the reading frame of GL1 and leads to a premature stop codon. Homozygous mutant plants show almost no leaf and stem trichomes (Supplementary Figure S1). We decided to use this mutant line as a model system to test Cas9-based GT in Arabidopsis. Cas9-induced cleavage in combination with homology directed repair can be used to integrate sequences of choice into a genomic region. If we successfully reintegrated the missing 10 bp into this mutant line’s genome, we would expect restoration of leaf trichome formation. Since the mutant line was created by CRISPR/Cas9, a PAM sequence was already present at the site of mutation and the predicted Cas9 cleavage site (3 bp upstream of the PAM sequence) matched the desired integration site for the missing 10 bp (Supplementary Figure S1).
Selection of a Gene Targeting System and Design of Related Constructs
Homologous recombination is more efficient in plants if the homology template is available at the site of the DSB (Baltes et al., 2014). If the homology template is delivered as T-DNA, which integrates randomly into the genome, it might not be available at the DSB site for the DNA repair machinery. Thus, we comparatively analyzed two methods to enhance the availability of the homology template at the DSB site.
The first method was developed by Baltes et al. (2014) in tobacco calli. This method increases the availability of the homology template by including it in small circular geminiviral replicons.
For the repair of the gl1 gene, we accordingly created a single T-DNA vector, (Figure 1A), that contained an Arabidopsis codon-optimized Cas9 gene under the control of the constitutive PcUB promoter (Fauser et al., 2014), a sgRNA cassette targeting the gl1 gene, and the homology template with ∼800 bp of homology arms on both sides, flanked by LIRs, SIR, and a Replicase (Rep/RepA) gene. For an easy nomenclature of the plasmids, we decided to include the name of the homology template activation method (viral replicons = VIR; in planta gene targeting = IPGT) and the used Cas9 variant (nuclease = Nuc; nickase = Nick) in the plasmid name and therefore named this construct pVIR-Nuc.
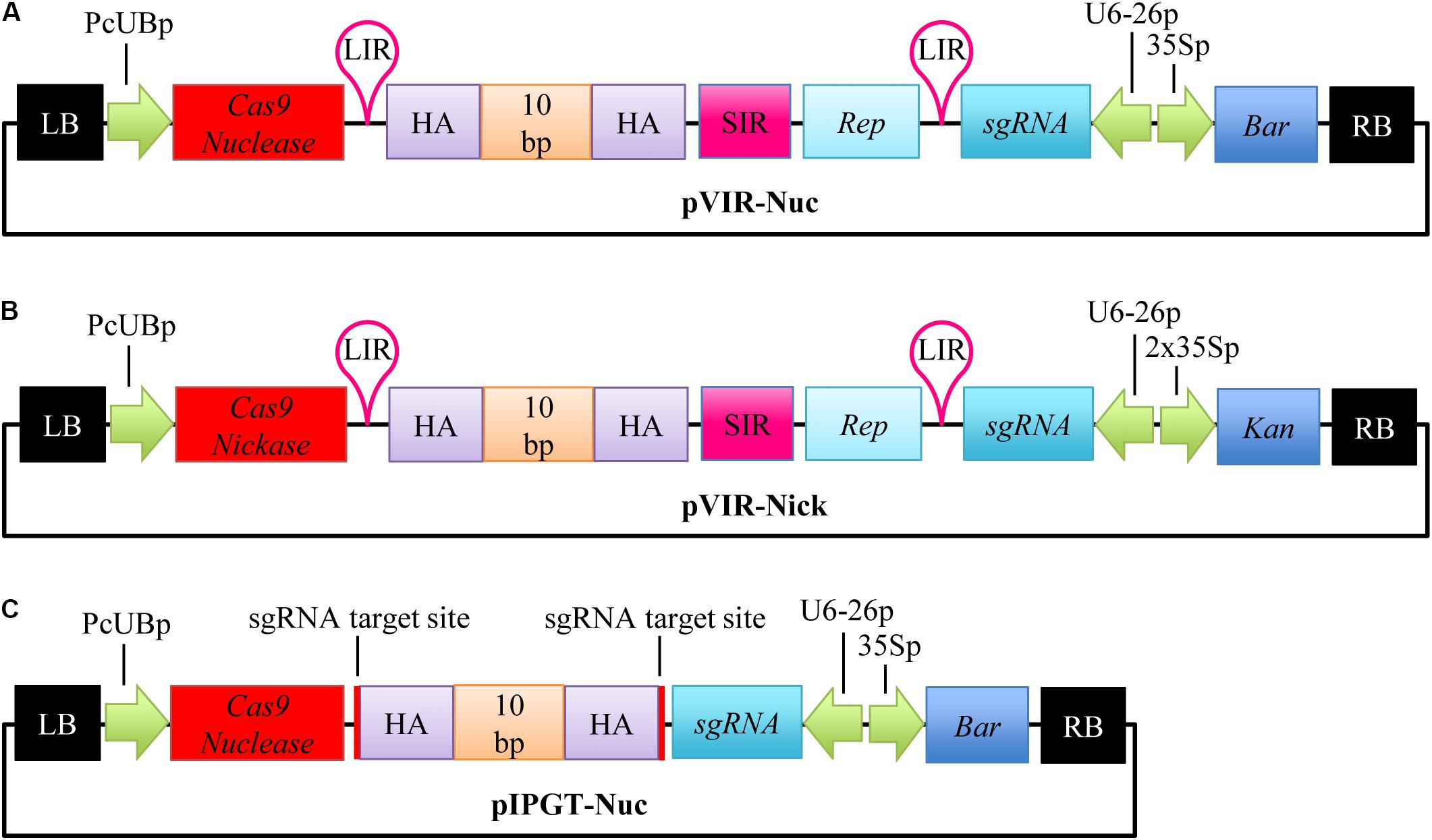
FIGURE 1. Vector design for repair of the gl1 gene. Single T-DNA vectors were designed to repair the dysfunctional gl1 gene. (A) pVIR-Nuc contains the Cas9 Nuclease gene (red box) under a constitutive Ubiquitin4-2 promoter from parsley (PcUBp) and the sgRNA cassette (petrol box) controlled by the U6-26 promoter (U6-26p). A Basta resistance cassette (Bar, dark blue box) is included as selection marker. The repair template itself consists of 10 nucleotides (10 bp, CTGCCGTTTA, orange box), which should restore the reading frame of the gl1 gene, flanked by homology arms (HA, purple box). Rolling circle replication of the homology template is ensured by the flanking long intergenic region (LIR, pink hairpin), short intergenic region (pink box, SIR), and the gene encoding the replicase Rep/RepA (light blue box). (B) pVIR-Nick contains similar features but harbors the gene for the Cas9 nickase instead of the nuclease and a kanamycin resistance cassette (Kan, dark blue box) as selection marker. (C) pIPGT-Nuc contains the gene for the Cas9 nuclease, the sgRNA cassette, the Basta resistance cassette and the repair template with homology arms flanked by target sites for the Cas9 nuclease (red lines). RB, right T-DNA border; 35Sp, Cauliflower Mosaic Virus 35S promoter; LB, left T-DNA border. Size not to scale.
After expression, Rep binds to the LIR sites and initiates rolling circle replication, leading to the formation of up to several thousand copies of the homology template in the nucleus (Timmermans et al., 1992; Baltes et al., 2014), which can then be used as repair template for the DSB created by Cas9 nuclease (Figure 2A).
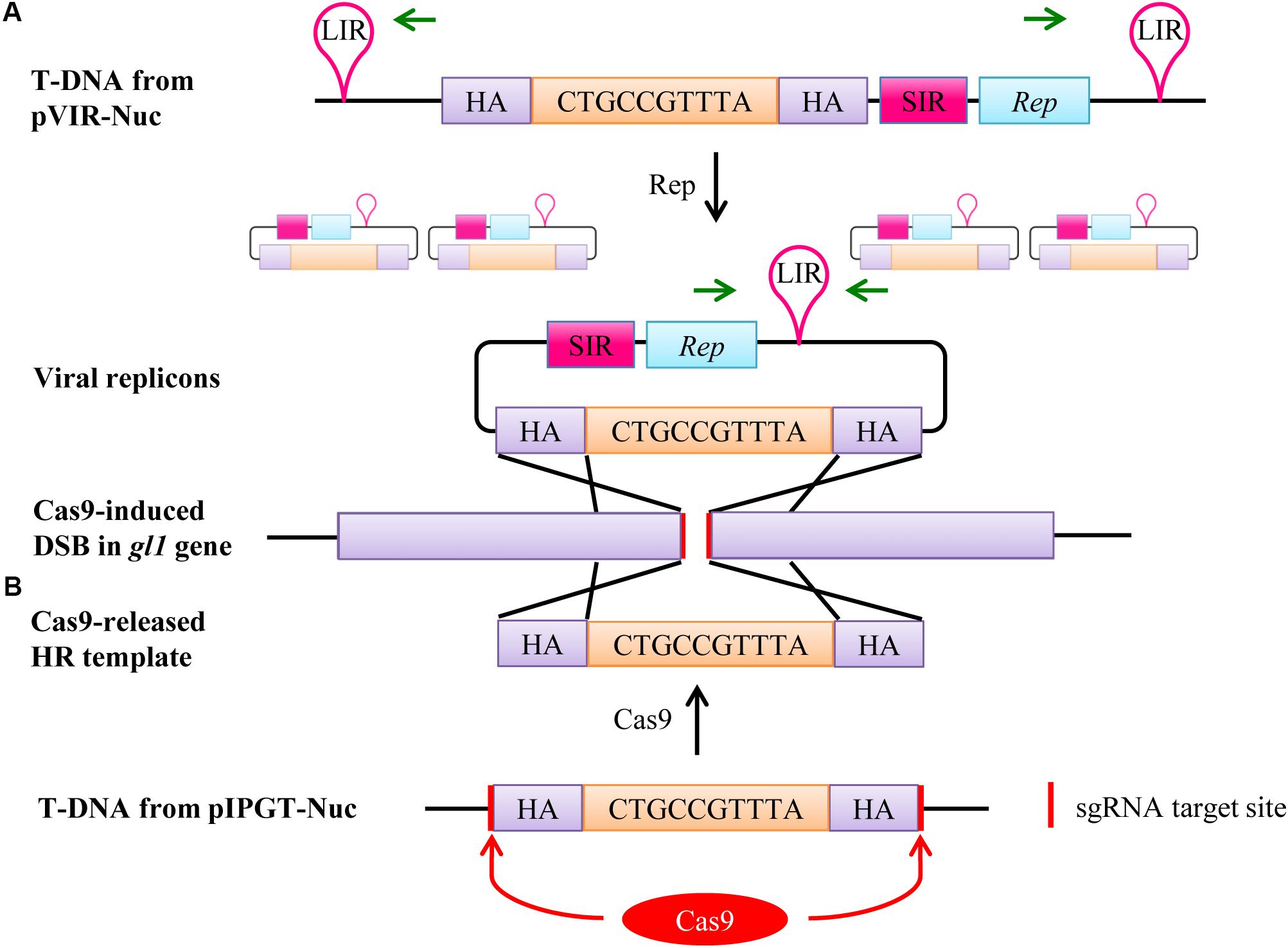
FIGURE 2. Two different mechanisms enhance the availability of the homology template in the plant cell nucleus. (A) If the T-DNA from pVIR-Nuc is integrated into the plant genome, Rep is expressed and initiates rolling circle endoreplication of the homology template leading to thousands of viral replicons. The presence of viral replicons can be detected by PCR using primers that only generate an amplicon on the circular replicons but not on the T-DNA (green arrows). The homology template can then attach to the DSB site in the gl1 gene induced by Cas9 due to homology between the homology arms (HA) and the genomic regions next to the DSB (purple). Repair of the DSB by HR will then lead to integration of the 10 bp, which restores the ORF. pVIR-Nick functions accordingly, except that Cas9 nickase only induces a DNA nick in the gl1 gene. (B) The homology template in the T-DNA of pIPGT-Nuc is flanked by the same sgRNA target site that is present in the gl1 gene. Expression of Cas9 will therefore simultaneously target the gl1 gene and release the homology template, which can then attach to the DSB and function as repair template.
To further explore the potential of this method, we additionally generated and tested a complementary vector pVIR-Nick in which the Cas9 nuclease gene was replaced by a nickase gene (Figure 1B). DNA nicks trigger HR without triggering the competing NHEJ repair (Fauser et al., 2014). NHEJ repair interferes with HR because it induces random indel mutations that destroy the sgRNA target site and make it unsuitable for homology directed repair. By using the Cas9 nickase, we expected to increase HR efficiency.
The second method, developed by Fauser et al. (2012), is called IPGT. This method increases the availability of the homology template by releasing it from the T-DNA backbone using the same SSN that creates the DSB in the target region. Therefore, we designed the vector pIPGT-Nuc (Figure 1C), in which the homology template is flanked by the same sgRNA target site that is used to create the DSB in the gl1 gene (Figure 2B).
Plants Transformed With pVIR-Constructs Form Circular Viral Replicons
We transformed Arabidopsis gl1 plants with either pVIR-Nuc, pVIR-Nick, or pIPGT-Nuc and selected three independent T1 lines per construct. Though we did not detect trichomes on the leaf surface of any T1 plant, we wanted to verify that the viral components were indeed producing circular homology templates in the T1 plants. Thus, we performed a PCR using primers that only yield a product in the circularized version of the homology template but not in the linear T-DNA (Figure 2A). We detected PCR products in the plants transformed with pVIR-Nuc and pVIR-Nick. PCR fragments for plants transformed with pIPGT-Nuc, which do not generate replicons (Supplementary Figure S2), were not obtained.
T2 Generation Plants Show Spots of Trichomes on Leaves
We analyzed several hundred plants from the progeny of each T1 plant for trichome reappearance. While we could not detect plants that fully recovered trichome production on leaves, we found several plants from the IPGT approach that harbored spots of trichomes (Table 1). These spots ranged from two trichomes on a single leaf of the plant to large spots on several leaves (Figure 3A). In contrast, using the viral replicon method, we only detected one plant with a single trichome in plants transformed with pVIR-Nuc, which implements a full Cas9 nuclease to trigger HR. Plants transformed with pVIR-Nick (Figure 3A), which implements the Cas9 nickase, did not form any trichomes on their leaves.
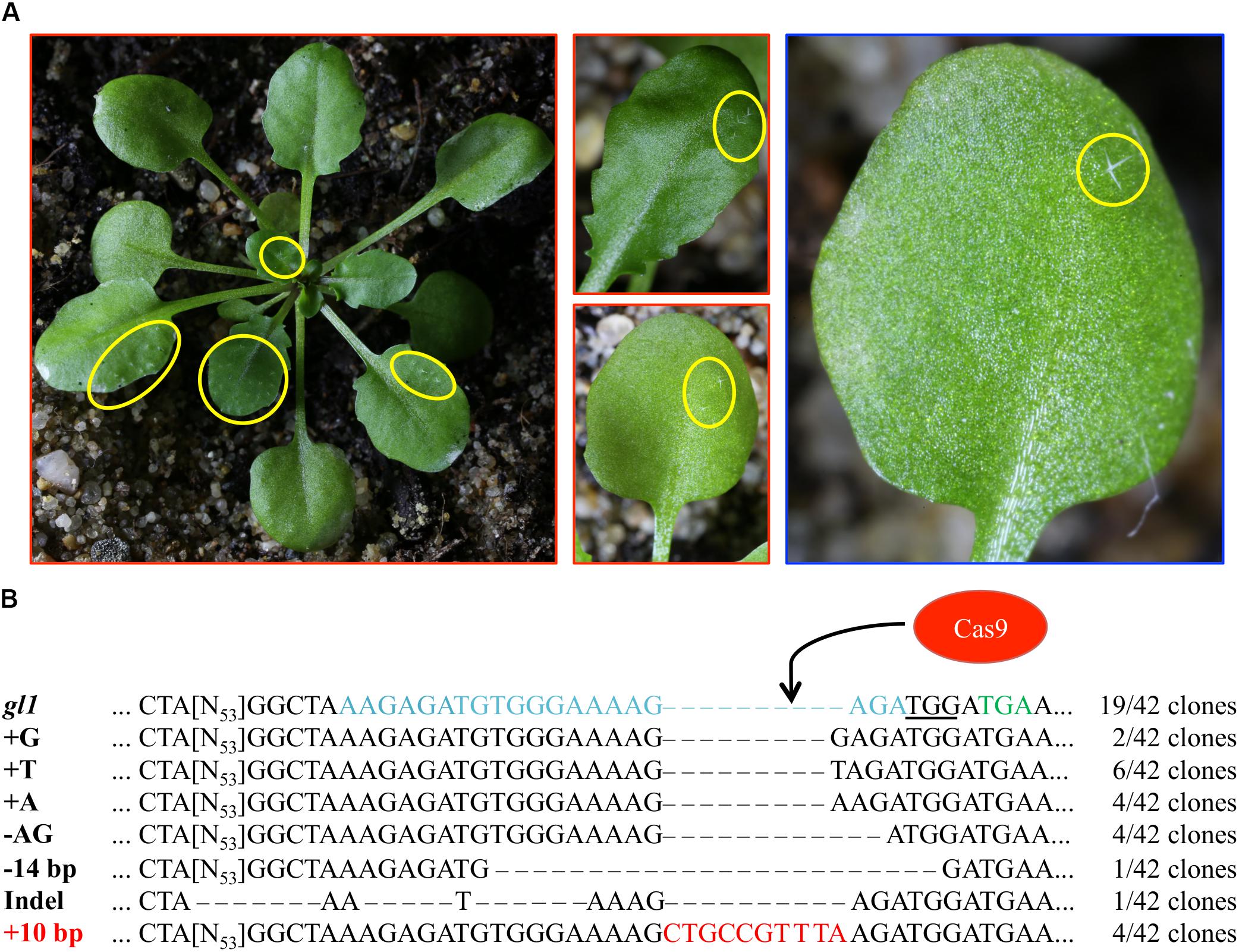
FIGURE 3. T2 generation plants show spots of trichomes. (A) Representative images of T2 plants transformed with pIPGT-Nuc (red frame) or pVIR-Nuc (blue frame) showing spots of trichomes (yellow circles) ranging from one single trichome to fully covered leaves. (B) The gl1 gene was amplified from leaves with trichomes, subcloned, and sequenced. Sequence analysis revealed clones with various indel mutations next to clones containing the desired 10 bp insertion (red letters). PAM sequence is underlined, STOP codon in gl1 line is marked in green letters, Cas9 target site is marked in blue letters.
We isolated gDNA from leaf trichome spots of three IPGT plants (the single trichome on the pVIR-Nuc plant provided not enough plant material for gDNA isolation), amplified the gl1 gene, subcloned the PCR product and sequenced 42 clones. While most clones still contained the gl1 sequence with the 10 bp deletion, we detected various indel mutations but also the integration of the codon-modified 10 bp sequence, which restored the wild-type amino acid sequence of the GL1 gene (Figure 3B). This chimerism on genotype level was in line with the highly chimeric phenotype of the leaves.
Fully Regenerated Trichomes Were Detected in Plants of the T3 Generation
We harvested seeds from the chimeric T2 plants and analyzed ∼200 progeny T3 plants of each T2 plant. In most cases, the progeny plants were glabrous or just contained single trichome spots. However, we detected three plants (frequency: 1.5%) in the progeny of the highly chimeric IPGT plant (Figure 3A, left) with fully regenerated trichomes (Figure 4A). We amplified the gl1 gene from those plants and sequenced the PCR product. Two of the three plants showed clear peaks corresponding to the 10 bp insertion but also peaks corresponding to small indel mutations (Figure 4B and Supplementary Figure S3), indicating that only one of the two alleles was repaired by HR. In the third plant, we detected the expected 10 bp insertion not in the sequencing histogram but in 5 out of 15 sequenced clones of subcloned PCR product (Supplementary Figure S3D).
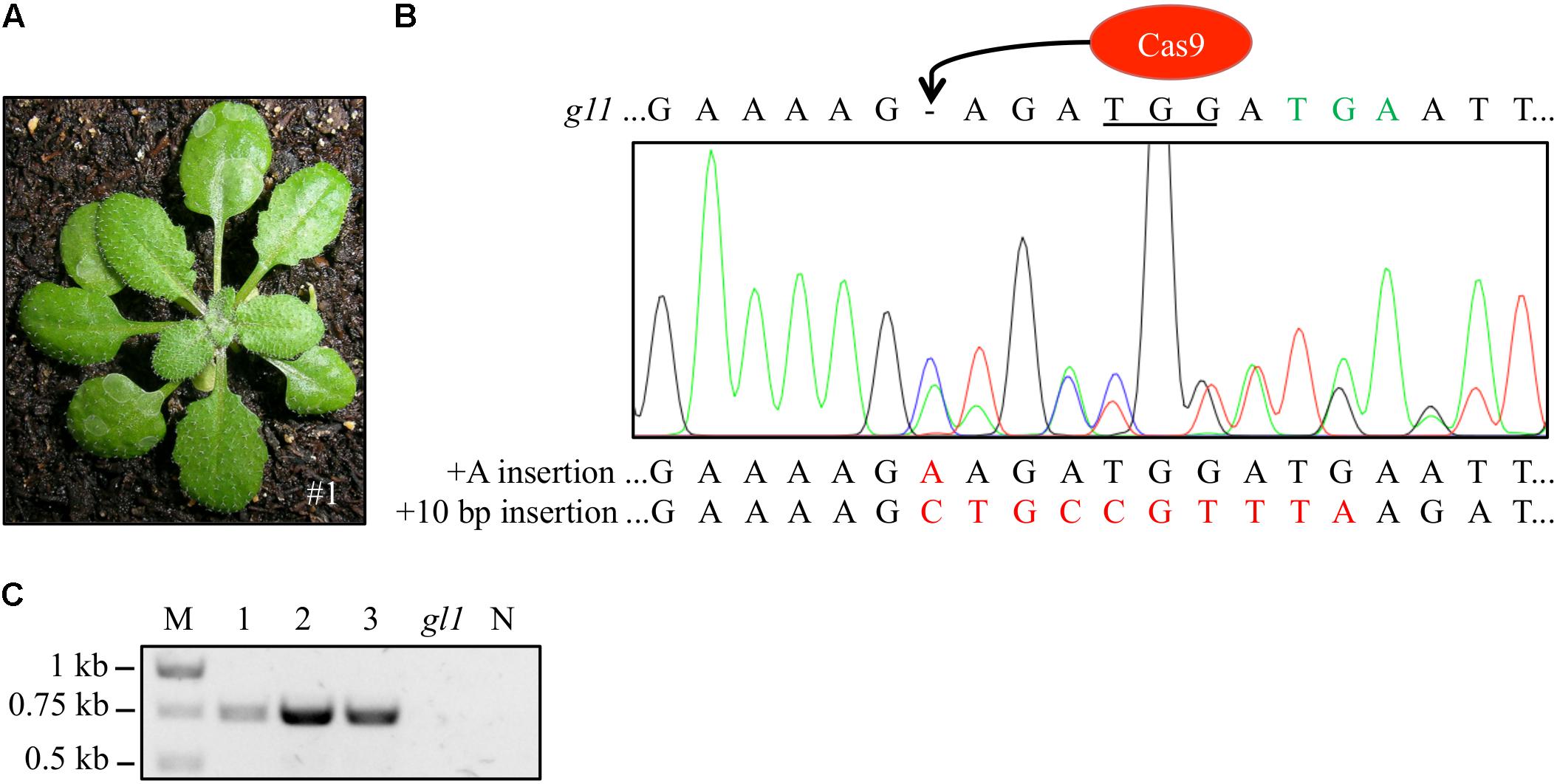
FIGURE 4. Wild-type-like trichome patterning was detected in three T3 generation plants. (A) Exemplary picture of one of the three T3 plants (#1) with full trichome covering. (B) We verified the repair of the gl1 gene by amplifying the gl1 gene and sequencing the PCR amplicon. Double peaks appeared at the site of Cas9 cleavage hinting at a chimeric or biallelic mutation, the peaks corresponded to an insertion of the ten bp (red) from the homology template and to an adenine insertion. Sequence of the dysfunctional gl1 gene is given on top of the sequencing histogram for comparison. Peak colors: Adenine = green, cytosine = blue, guanine = black, thymine = red; PAM sequence underlined, premature STOP codon marked in green letters. (C) Presence of the Cas9 gene in the three non-glabrous plants was detected by PCR using Cas9-specific primers. All three plants revealed PCR amplicons at the expected size of 749 bp. In contrast, DNA from the gl1 background line did not yield a PCR Cas9 signal. Image of agarose gel was color inverted for better visibility. M, marker; N, water control.
The Repaired GL1 Allele Segregates in a Mendelian Way
In genome edited plants, while the editing nuclease (Cas9) is active, it is hard to distinguish between somatic and heritable mutations. The three plants that fully recovered trichome production were still carrying the Cas9 gene (Figure 4C), which was likely still active. Thus, we confirmed in those plants that the 10 bp insertion is indeed heritable and not somatic by Mendelian segregation analysis. We analyzed the offspring of the three T3 plants displaying trichomes and counted the amounts of trichome-bearing and glabrous plants in the T4 generation. If one allele was indeed repaired by HR in the whole plant and the other one not, we would expect a Mendelian distribution of the trichome phenotype with a ratio of non-glabrous:glabrous plants of 3:1. Indeed, the progeny of all three plants did not deviate significantly from the expected 3:1 ratio as calculated by chi-squared test (χ2; P > 0.5, Table 2).

TABLE 2. Segregation pattern of trichome phenotype in T4 generation plants transformed with pIPGT-Nuc.
Sequencing of 109 progeny plants from all three T3 plants (82 plants with trichomes, 27 plants without trichomes) revealed that the trichome phenotype was always associated with at least one repaired GL1 allele, while the glabrous plants mostly contained various small indel mutations (Supplementary Figure S3 and Supplementary Table S2). Among the hairy plants, sequencing indicated that 31 plants appeared to be homozygous for the 10 bp insertion (Figure 5 and Supplementary Table S2). This result is consistent with GT, rather than an ectopic recombination event, in which a mutant allele would still be present. The small indel mutations found in glabrous plants or in heterozygous non-glabrous plants were in many cases not consistent with the indel alleles found in the progenitor plants, indicating that only the repaired GL1 allele was already fixed in the T3 generation and that the other sequenced allele in the T3 plants was caused by somatic mutations in the leaf used for gDNA extraction. This putative chimerism in the T3 generation plants corresponds to the presence of more than two sequence peaks in some of the sequencing histograms of the T3 parental plants (Supplementary Figure S3).
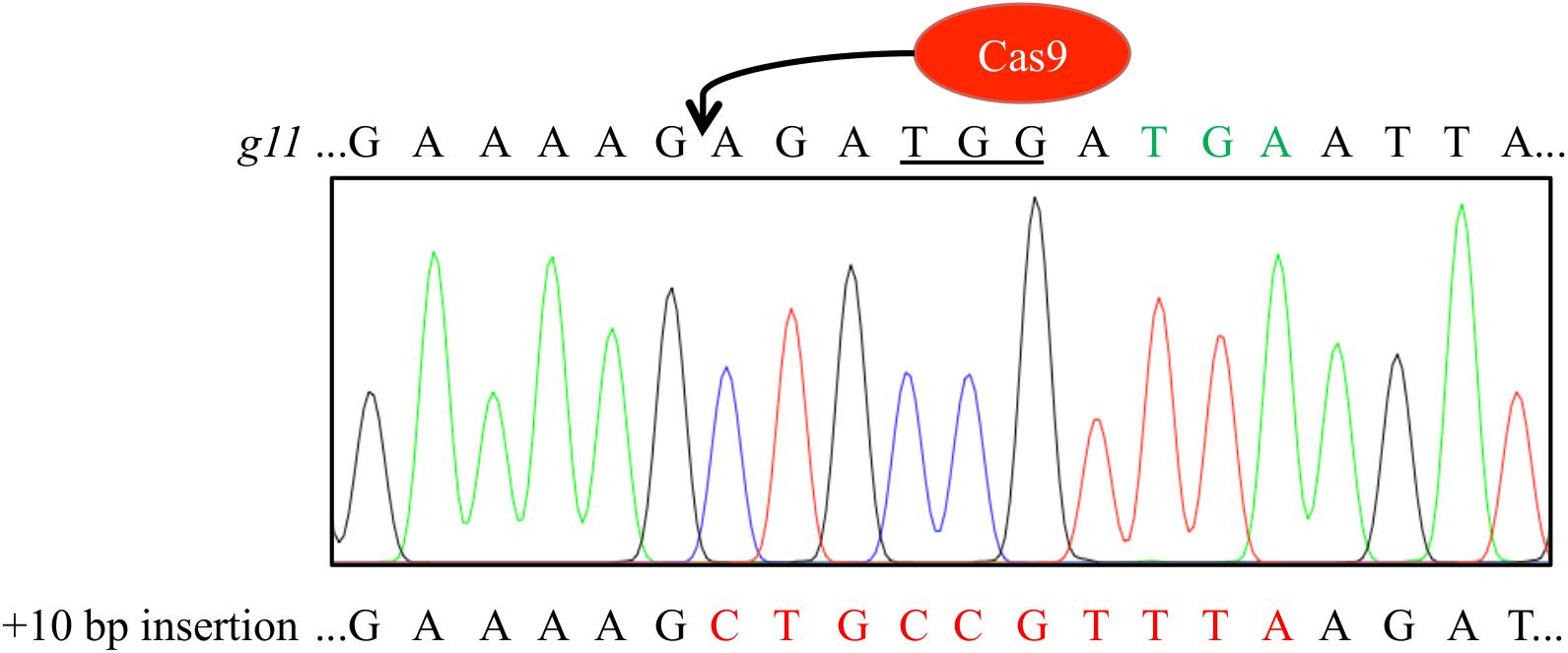
FIGURE 5. Sequencing histogram of T4 generation plant reveals homozygous integration of the 10 nucleotides thereby restoring the GL1 reading frame. Sequence of the dysfunctional gl1 gene is given on top of the sequencing histogram for comparison. Peak colors: Adenine = green, cytosine = blue, guanine = black, thymine = red; PAM sequence underlined, premature STOP codon marked in green letters.
In summary, we screened between 2,200 and 2,500 plants containing the gl1 repair constructs pIPGT-Nuc, pVIR-Nuc, and pVIR-Nick, respectively, over three generations. Using the viral replicon approach, we could not find non-glabrous plants. Using the IPGT approach, we were able to detect three stable GT events in the T3 generation with one functional GL1 allele.
Discussion
Trichome Formation Is a Marker for Mutagenic Efficiency
The Cas9 system has become a valuable and efficient tool for genome modifications in plants (Arora and Narula, 2017). However, while random mutagenesis in plants by error-prone DSB repair through the NHEJ-pathway has been successfully applied in many studies (Liu et al., 2017), introduction of targeted mutations and gene knock-ins by GT remains challenging.
To explore the efficiency of two systems that have been described to induce HR in plants, we used a Cas9-generated trichomeless Arabidopsis mutant (Hahn et al., 2017). This mutant contains a 10 bp deletion (Supplementary Figure S1) in the GL1 gene, which leads to a premature STOP codon. The trichome phenotype (Figure 3) allowed easy selection of transformed plants, which were likely to produce completely repaired offspring (Figure 4).
An important aspect of our marker system is that DSB repair has to occur via HR and not via NHEJ to induce trichome formation. Small indels of 1–2 nucleotides generated by error-prone NHEJ might restore the open reading frame (ORF) of the gene, but at least three amino acid residues are still missing in the MYB-binding domain of the GL1 protein, which are critical for protein function (Hauser et al., 2001; Dai et al., 2016). As NHEJ mediated repair of DSB rarely results in insertions of more than one nucleotide (Fauser et al., 2014), it is highly unlikely that NHEJ mediated repair can restore trichome formation. Consequently, in a large-scale screening of glabrous and non-glabrous T4 plants (Supplementary Figure S4), we detected a clear correlation of trichome formation and repair via HR, while NHEJ mutations did not result in non-glabrous plants even though the ORF of the gene was partly restored in some cases. Previously used markers, such as β-glucuronidase (GUS) or fluorescent proteins need first to be introduced into the plant and GT events can only be detected by staining or fluorescence microscopy. In contrast, our endogenous marker gene has two advantages: First, it allows to follow the mutagenesis progress by eye and second, trichome absence does not have pleiotropic effects on plant development under greenhouse conditions (Oppenheimer et al., 1991).
Activation of the Homology Template by Viral Replicons Did Not Result in Gene Targeting Events
Baltes et al. (2014) developed a GT approach in tobacco that employs geminivirus-based replicons to active the homology template (Figure 2). In a first approach in Arabidopsis, they aimed at inserting a short DNA sequence into an endogenous gene by GT using a homology template, which was activated by replication in recombinant bipartite cabbage leaf curl virus genomes. While GT events could be detected on somatic level by PCR, the authors did not report inheritance of the GT events to progeny plants (Baltes et al., 2014). In further experiments, several groups used deconstructed virus replicons of BeYDV (or other viruses) depleted of the coat and movement proteins. These can be stably transformed into plants and have an increased vector cargo capacity, allowing the use of larger homology templates. This approach allowed efficient GT in various crop plants, including tobacco, tomato, potato, rice, and wheat (Baltes et al., 2014; Čermák et al., 2015; Butler et al., 2016; Gil-Humanes et al., 2017; Wang et al., 2017).
While the initial experiments with the full virus demonstrated that GT using viral replicons can be achieved in Arabidopsis, no further studies reported the use of deconstructed replicons in Arabidopsis. We were also unable to detect GT events in form of trichome regeneration in 2,334 analyzed plants from three independent lines transformed with pVIR-Nuc except a single trichome (Table 1 and Figure 3). One possible explanation could be that the sgRNA that we used did not allow efficient DSB induction in the target locus. Since we generated successful GT events with the IPGT approach, using the same sgRNA, which requires efficient Cas9 cutting for homology template activation, we do not consider this as likely. As we detected high frequencies of NHEJ mutations next to GT events while sequencing T4 generation plants (Supplementary Figure S4), we conclude that our sgRNA is highly active. It could be possible that replicon formation is not efficient in Arabidopsis in contrast to other plant species such as tobacco, maize, and rice, where geminiviral replicons could be detected in high copy numbers ranging from several hundreds to 30000 copies per cell (Timmermans et al., 1992; Baltes et al., 2014; Wang et al., 2017). However, BeYDV is known to infect Arabidopsis (Liu et al., 1997). Thus, replicon formation should be possible. Consequently, we detected replicon formation on DNA level in T1 plants (Supplementary Figure S2). We consider a replication stop after an initial round of endoreplication as unlikely even though total copy numbers of the replicons were not assessed in this study. Another option could be an Arabidopsis-specific discrimination of the repair machinery for circular homology templates as these do not occur naturally in the plant nucleus.
Differences between plant species with handling of foreign DNA molecules have already been observed in Arabidopsis and tobacco (Orel and Puchta, 2003). It has been suggested that the stimulatory effect of viral replicons on GT is not only caused by the amount of homology template but also by the impact of RepA on the plant cell cycle. RepA stimulates entry of cells into the S-phase by interaction with plant proteins that control cell cycle so that the virus can use the necessary factors for replicon replication that the cell produces for DNA synthesis (Baltes et al., 2014). Cells in S-Phase show an increased HR efficiency in eukaryotes (Saleh-Gohari and Helleday, 2004; Mathiasen and Lisby, 2014). Possibly, this effect is less pronounced in Arabidopsis than in other plants.
In contrast to most of the studies using viral replicons, we did not include the SSN in the replicating unit. Baltes et al. (2014) reported comparable GT efficiencies if the ZFN was part of the replicating unit or not, and similar results were found in a Cas9-based approach in tomato (Čermák et al., 2015). However, it might be possible that a high amount of SSN is more critical in Arabidopsis.
Donor architecture might also account for variable efficiencies in GT. We used homology arms with a length of app. 800 bp, which is in the range of the most successful GT studies so far (Fauser et al., 2012; Baltes et al., 2014; Schiml et al., 2014; Čermák et al., 2015; Butler et al., 2016; Gil-Humanes et al., 2017; Wang et al., 2017). Additionally, we used the viral components described in the original report of Baltes et al. (2014) and just integrated our homology template. Even though we used a different vector backbone (Fauser et al., 2014), the replicons should therefore be comparable to the ones described in successful GT studies by Baltes et al. (2014) and Čermák et al. (2015).
While we could not detect considerable trichome formation in our experiments, it has to be taken into account that GT events might occur within cells, which are not determined to develop into trichomes. That is, using trichome formation as readout for mutagenic efficiencies, we actually underestimate the amount of successful GT events on the somatic cell level. PCR-based methods, as used for GT detection in Arabidopsis using cabbage leaf curl virus genomes (Baltes et al., 2014) will therefore allow detection of more GT events. In other experiments of our group with a different target gene (unpublished data), we actually could detect GT events using PCR-based approaches on somatic level at low frequencies but we were also unable to regenerate inheritable events. Taken together, these data indicate that viral replicons allow GT only at low frequencies in Arabidopsis, which contrasts with the considerable success in many crop species. Further experiments are needed to unravel the reasons for these differences so that this promising technique might also be applicable in this important model organism.
Cas9 Nickase Is Not Improving HR Events in Combination With Viral Replicons
We tested whether Cas9 nickase can induce GT at higher efficiencies than Cas9 nuclease as proposed by Fauser et al. (2014). We only applied the Cas9 nickase in combination with the viral replicon approach, since IPGT needs a nuclease to release the HR template. We did not detect GT events in form of trichome formation on any plant (Table 1). However, when we combined the viral replicon approach with a Cas9 nuclease, we also detected only a single putative GT event in ∼2,300 plants screened (Table 1 and Figure 3). Therefore, it is likely that the viral replicon approach does not trigger GT in Arabidopsis, independent from its Cas9 variant.
However, besides the initial report of Fauser et al. (2014), reports on GT using nickases in plants are scarce. For their GT experiments, Fauser et al. (2014) used a reporter construct (Orel et al., 2003) containing a disrupted GUS ORF as well as a homologous sequence, which can function as homology template for repair of the GUS ORF upon induction of a DSB by Cas9. Up to four times more HR events were detected compared to experiments with Cas9 nuclease. Importantly, only somatic mutations were quantified and information on the regeneration of stably mutated plants was not provided. It has been observed earlier, that GT efficiencies increase if target locus and donor construct are located on the same chromosome (Fauser et al., 2012). In the GUS experiments described above, target locus and homology template are directly adjacent, which possibly explains the successful HR events. In our case, the homology template integrated randomly into the genome and even after replicon formation, the replicons still had to “find” the nick site to be available for HR. Supporting this hypothesis, other studies detected lower GT efficiency when using nickases compared to nucleases (Ran et al., 2013; Čermák et al., 2017).
In a recent study, Cas9 nickase was used in combination with viral replicons to repair a dysfunctional GUS gene in tobacco. Using two sgRNAs to create adjacent nicks, a twofold increase in GT was observed (Čermák et al., 2017). However, paired nicks on different DNA strands in close proximity are considered to induce a DSB with single strand overhangs (Schiml et al., 2014). Thus, it is questionable if nick HR repair pathways were indeed responsible for the successful GT in that study. In human cells, DNA nick repair via HR occurs eight times more frequently on the transcribed (non-coding) DNA strand, probably due to stimulation by the transcription machinery (Davis and Maizels, 2014). The Cas9 nickase used in our study only contains a functional HNH-cleavage domain so that the nick is created on the non-coding strand. However, whether the strand preference in plants is comparable to that in human cells remains to be elucidated and might provide a possibility to enhance HR frequencies.
In general, HR repair mechanisms on DNA nicks in plants are not well studied. In humans, two competing HR mechanisms are proposed. The canonical HR pathway resembles the synthesis-dependent strand annealing repair pathway for DSB, while the alternative one preferentially employs single stranded oligonucleotides or nicked dsDNA homology templates for repair (Davis and Maizels, 2014). The alternative HR pathway shares features with another HR pathway, the single strand annealing pathway, which is induced by micro-homologies at the break ends. As HR via the single strand annealing pathway seems to be more efficient in plants than the synthesis-dependent strand annealing repair pathway (Orel et al., 2003; Fauser et al., 2014), it might be interesting to include nickase target site in the repair template to induce target locus repair via the alternative HR nick repair pathway. However, the detailed mechanisms underlying DNA nick repair in plants via HR needs to be elucidated to verify if the same mechanisms as in human cells apply. As the Cas9 nickase has already been shown to induce gene knock-ins in human embryonic stem cells using dsDNA as template (Rong et al., 2014), it remains an interesting option for future HR experiments in planta.
The In Planta Gene Targeting Approach Allows Regeneration of Stable Gene Targeting Events in Arabidopsis
The IPGT approach, which excises the homology template from the T-DNA backbone by a SSN (Figure 2), has been used successfully in Arabidopsis. By the application of the I-SceI meganuclease a GUS marker gene was restored (Fauser et al., 2012), and by the application of the Cas9 system a resistance cassette was integrated into the ADH locus (Schiml et al., 2014) and a GFP gene introduced into the TFL1 locus (Zhao et al., 2016). In contrast to these previous studies, we did not exploit an artificial marker gene but restored the gene function of the endogenous GL1 gene, using trichome formation as direct read-out for GT events.
We detected spots of trichomes ranging from single trichomes to fully covered leaves in T2 generation plants (Figure 3 and Table 1). Other studies reported similar chimeric GT spots using GUS staining (Fauser et al., 2012). The T2 plant, which showed the highest level of chimerism (Figure 3) produced offspring plants with WT-like trichome patterning. We therefore conclude, that the trichome phenotype can be used to select for plants, in which GT efficiencies are enhanced and which are candidate plants for production of stably mutated progeny. This enhancement can have several reasons. The location of the T-DNA, which contains HR template and SSN gene, in the plant genome likely has influence on GT and close proximity of repair template and DSB locus seems favorable (Fauser et al., 2012). Similarly, we found differences in the frequency of chimeric T2 plants depending on the parental T1 line (Table 1). It is therefore highly recommended to start with as many independent transformation events as possible, in contrast to screening a lot of progeny plants from few primary transformants (Schiml et al., 2017).
T2 plants might be homozygous or heterozygous for the T-DNA insertion. It has been shown that the heterozygosity state of the HR template influences recombination frequencies (Puchta et al., 1995; Orel et al., 2003). Indeed, since all three plants, which completely restored trichome formation in the T3 generation (Figure 4 and Supplementary Figure S3), contained the Cas9-T-DNA cassette and also produced only T-DNA containing offspring, it is likely, that the parental T2 plant contained already two copies of the repair template. Crop improvement usually requires the creation of marker-free plants. Thus, more screening effort might be needed to detect T-DNA free GT plants.
It has been reported that one-sided GT events can occur during repair (Schiml et al., 2014), in which one side of the DSB is repaired by HR and the other strand is repaired using the stably integrated T-DNA as template. This can lead to integration of large parts of the homology template, which finally results in a NHEJ-mediated ligation of the repaired strand and the loose end of the DSB. To detect such events, we had added single bp exchanges in both homology arms. Our sequencing results of our repaired GL1 alleles did not contain these bp exchanges, which indicates that we achieved true GT events.
In previous studies, the frequencies of GT events using IPGT in Arabidopsis ranged from 0.14 to 0.83% (Fauser et al., 2012; Schiml et al., 2014; Zhao et al., 2016) which corresponds well to our GT frequency of 0.12%. The single bp exchanges in the homology arms, which allowed us to detect true GT events, might explain the slightly lower efficiencies in our experimental approach. Additionally, efficiencies in Cas9 experiments are difficult to compare due to use of different sgRNAs (Peng et al., 2016).
Further Options to Enhance GT
In this report, we identified three stable GT event out of ∼2,500 Arabidopsis plants screened across three generations using an IPGT approach. In principle, a GT event at such frequency could be detected exclusively by PCR and without a phenotype based screening. However, we achieved such a high frequency because we were able to select parental lines prone to GT by phenotype. Therefore, it might still be desirable to increase GT efficiencies if no visual marker is used for GT detection. In general, it is advisable to test sgRNA efficiencies in protoplast systems before using them in stable plant transformations as efficient induction of DSB is a prerequisite for successful GT (Puchta et al., 1993).
Higher GT efficiencies of ∼5.5% have been achieved in Arabidopsis protoplasts using single stranded oligonucleotides as repair template (Sauer et al., 2016) to exchange specific nucleotides in a fluorescent protein to change its emission spectrum. However, single stranded oligonucleotides are not suitable for knock-in of larger DNA fragments or whole genes due to size limitations and are not transformed via Agrobacteria. For targeted exchange of a single bp, the use of base changer Cas9 provides an attractive alternative to HR-mediated repair processes. In this approach, an inactive Cas9 is fused to cytidine deaminase, which converts cytosines to uracil without DNA cutting (Komor et al., 2016; Zong et al., 2017). Recently, an adenine base editor Cas9 has also been described (Gaudelli et al., 2017). While mutation frequencies of up to 50% can be achieved using base changer Cas9, this approach cannot be applied for gene knock-ins.
In general, protoplast transformation seems to yield higher mutation frequencies compared to Agrobacterium-mediated transformation (Li et al., 2013; Sauer et al., 2016), since more DNA donor molecules are integrated in the plant cells. However, regeneration of Arabidopsis plants from protoplasts is challenging and is therefore not an attractive alternative to floral dipping. Focusing research on improved regeneration protocols is therefore desirable. Several groups tried to enhance HR efficiencies by disrupting either the NHEJ pathway (Maruyama et al., 2015; Zhu et al., 2015) or introducing HR stimulating enzymes from other organisms (Reiss et al., 2000; Shaked et al., 2005; Even-Faitelson et al., 2011). These approaches can destabilize the genome as HR frequencies are enhanced between all repetitive sequences in the genome (Puchta and Fauser, 2013).
In the IPGT approach, the homology template is excised from the genome. If this happens at an early stage of plant development, the homology template might not be passed on during cell division. This could reduce the efficiency of this approach. Using germline-specific promoters (Wang et al., 2015; Mao et al., 2016) for the SSN might increase chances of simultaneous cleavage of target locus and release of the homology template specifically in the cells, where GT needs to occur to generate stable offspring.
A similar problem is target locus inactivation through error-prone NHEJ repair. Besides the before mentioned nickase approach, the Cas9 variant CpfI (CRISPR from Prevotella and Francisella) might provide an attractive alternative. CpfI generates DSB more distal to the PAM than Cas9 (Zetsche et al., 2015), thus making the target locus accessible to a second round of cleavage even after NHEJ mediated repair. An interesting approach would be to combine the viral replicon approach with the IPGT approach. Therefore, the homology template would be flanked by SSN cutting site and the viral components. By this, large amounts of linear dsDNA molecules would be generated that can be used as repair templates. Since more target sites are present in this approach, we suggest including the gene for the SSN and the sgRNA within the replicon unit, so that sufficient amounts of all genome editing components are available.
Conclusion
In this report, we applied the Cas9 system to induce GT events in Arabidopsis. The homology template needed to be activated by an IPGT approach. We were able to stably introduce a short DNA sequence into an endogenous gene and the inserted sequence was transmitted through the germline into the next generation. The high GT efficiency allows to use GT approaches also in marker-free approaches in which GT events need to be detected by PCR. Thus, we conclude that Cas9 mediated GT in combination with IPGT is a powerful tool that has high potential to become routinely applied in plant research in the future. Trichome formation as marker for editing efficiency proved to be a reliable system to compare different GT approaches. We suggest that the GL1 gene can be used for optimization of Cas9-based mutagenesis approaches in future experiments.
Author Contributions
FH, OM, ME, and AW designed the study, interpreted the data, and wrote the manuscript. FH carried out all laboratory experiments.
Funding
This work was funded by the Cluster of Excellence on Plant Sciences (CEPLAS, EXC 1028) and the HHU Center for Synthetic Life Sciences (CSL).
Conflict of Interest Statement
The authors declare that the research was conducted in the absence of any commercial or financial relationships that could be construed as a potential conflict of interest.
Acknowledgments
We thank Prof. Dr. Holger Puchta and Felix Wolters for providing us with the vectors pDE-Cas9, pDE-Cas9-D10A, and pEN-C1.1, and for the valuable feedback. We thank Prof. Dr. Peter Westhoff and Dr. Shizue Matsubara for the helpful discussions. We thank Steffen Köhler for photography support. We also thank the gardeners for plant care and the student helper team for their support.
Supplementary Material
The Supplementary Material for this article can be found online at: https://www.frontiersin.org/articles/10.3389/fpls.2018.00424/full#supplementary-material
References
Arora, L., and Narula, A. (2017). Gene editing and crop improvement using CRISPR-Cas9 system. Front. Plant Sci. 8:1932. doi: 10.3389/fpls.2017.01932
Ayar, A., Wehrkamp-Richter, S., Laffaire, J.-B., Le Goff, S., Levy, J., Chaignon, S., et al. (2013). Gene targeting in maize by somatic ectopic recombination. Plant Biotechnol. J. 11, 305–314. doi: 10.1111/pbi.12014
Baltes, N. J., Gil-Humanes, J., Cermak, T., Atkins, P. A., and Voytas, D. F. (2014). DNA replicons for plant genome engineering. Plant Cell 26, 151–163. doi: 10.1105/tpc.113.119792
Bortesi, L., and Fischer, R. (2015). The CRISPR/Cas9 system for plant genome editing and beyond. Biotechnol. Adv. 33, 41–52. doi: 10.1016/j.biotechadv.2014.12.006
Butler, N. M., Baltes, N. J., Voytas, D. F., and Douches, D. S. (2016). Geminivirus-Mediated genome editing in potato (Solanum tuberosum L.) Using sequence-specific nucleases. Front. Plant Sci. 7:1045. doi: 10.3389/fpls.2016.01045
Čermák, T., Baltes, N. J., Čegan, R., Zhang, Y., and Voytas, D. F. (2015). High-frequency, precise modification of the tomato genome. Genome Biol. 16:232. doi: 10.1186/s13059-015-0796-9
Čermák, T., Curtin, S. J., Gil-Humanes, J., Čegan, R., Kono, T. J. Y., Konečná, E., et al. (2017). A multipurpose toolkit to enable advanced genome engineering in plants. Plant Cell 29, 1196–1217. doi: 10.1105/tpc.16.00922
Chen, Q., He, J., Phoolcharoen, W., and Mason, H. S. (2011). Geminiviral vectors based on bean yellow dwarf virus for production of vaccine antigens and monoclonal antibodies in plants. Hum. Vaccin. 7, 331–338. doi: 10.4161/hv.7.3.14262
Clough, S. J., and Bent, A. F. (1998). Floral dip: a simplified method for Agrobacterium-mediated transformation of Arabidopsis thaliana. Plant J. 16, 735–743. doi: 10.1046/j.1365-313x.1998.00343.x
Dai, X., Zhou, L., Zhang, W., Cai, L., Guo, H., Tian, H., et al. (2016). A single amino acid substitution in the R3 domain of GLABRA1 leads to inhibition of trichome formation in Arabidopsis without affecting its interaction with GLABRA3. Plant Cell Environ. 39, 897–907. doi: 10.1111/pce.12695
Davis, L., and Maizels, N. (2014). Homology-directed repair of DNA nicks via pathways distinct from canonical double-strand break repair. Proc. Natl. Acad. Sci. U.S.A. 111, E924–E932. doi: 10.1073/pnas.1400236111
Doudna, J. A., and Charpentier, E. (2014). Genome editing. The new frontier of genome engineering with CRISPR-Cas9. Science 346:1258096. doi: 10.1126/science.1258096
Dueva, R., and Iliakis, G. (2013). Alternative pathways of non-homologous end joining (NHEJ) in genomic instability and cancer. Trans. Cancer Res. 2, 163–177.
Even-Faitelson, L., Samach, A., Melamed-Bessudo, C., Avivi-Ragolsky, N., and Levy, A. A. (2011). Localized egg-cell expression of effector proteins for targeted modification of the Arabidopsis genome. Plant J. 68, 929–937. doi: 10.1111/j.1365-313X.2011.04741.x
Fauser, F., Roth, N., Pacher, M., Ilg, G., Sánchez-Fernández, R., Biesgen, C., et al. (2012). In planta gene targeting. Proc. Natl. Acad. Sci. U.S.A. 109, 7535–7540. doi: 10.1073/pnas.1202191109
Fauser, F., Schiml, S., and Puchta, H. (2014). Both CRISPR/Cas-based nucleases and nickases can be used efficiently for genome engineering in Arabidopsis thaliana. Plant J. Cell Mol. Biol. 79, 348–359. doi: 10.1111/tpj.12554
Gaudelli, N. M., Komor, A. C., Rees, H. A., Packer, M. S., Badran, A. H., Bryson, D. I., et al. (2017). Programmable base editing of A∙T to G∙C in genomic DNA without DNA cleavage. Nature 551, 464–471. doi: 10.1038/nature24644
Gil-Humanes, J., Wang, Y., Liang, Z., Shan, Q., Ozuna, C. V., Sánchez-León, S., et al. (2017). High-efficiency gene targeting in hexaploid wheat using DNA replicons and CRISPR/Cas9. Plant J. 89, 1251–1262. doi: 10.1111/tpj.13446
Gorbunova, V., and Levy, A. A. (1997). Non-homologous DNA end joining in plant cells is associated with deletions and filler DNA insertions. Nucleic Acids Res. 25, 4650–4657. doi: 10.1093/nar/25.22.4650
Hahn, F., Mantegazza, O., Greiner, A., Hegemann, P., Eisenhut, M., and Weber, A. P. M. (2017). An efficient visual screen for CRISPR/Cas9 activity in Arabidopsis thaliana. Front. Plant Sci. 8:39. doi: 10.3389/fpls.2017.00039
Hauser, M. T., Harr, B., and Schlötterer, C. (2001). Trichome distribution in Arabidopsis thaliana and its close relative Arabidopsis lyrata: molecular analysis of the candidate gene GLABROUS1. Mol. Biol. Evol. 18, 1754–1763. doi: 10.1093/oxfordjournals.molbev.a003963
Jinek, M., Chylinski, K., Fonfara, I., Hauer, M., Doudna, J. A., and Charpentier, E. (2012). A programmable dual-RNA-guided DNA endonuclease in adaptive bacterial immunity. Science 337, 816–821. doi: 10.1126/science.1225829
Komor, A. C., Kim, Y. B., Packer, M. S., Zuris, J. A., and Liu, D. R. (2016). Programmable editing of a target base in genomic DNA without double-stranded DNA cleavage. Nature 533, 420–424. doi: 10.1038/nature17946
Koornneef, M., Dellaert, L. W., and van der Veen, J. H. (1982). EMS- and radiation-induced mutation frequencies at individual loci in Arabidopsis thaliana (L.) Heynh. Mutat. Res. 93, 109–123. doi: 10.1016/0027-5107(82)90129-4
Kumar, S., Worden, A., Novak, S., Lee, R., and Petolino, J. F. (2016). A trait stacking system via intra-genomic homologous recombination. Planta 244, 1157–1166. doi: 10.1007/s00425-016-2595-2
Lee, K. Y., Lund, P., Lowe, K., and Dunsmuir, P. (1990). Homologous recombination in plant cells after Agrobacterium-mediated transformation. Plant Cell 2, 415–425. doi: 10.1105/tpc.2.5.415
Li, J.-F., Aach, J., Norville, J. E., McCormack, M., Zhang, D., Bush, J., et al. (2013). Multiplex and homologous recombination-mediated plant genome editing via guide RNA/Cas9. Nat. Biotechnol. 31, 688–691. doi: 10.1038/nbt.2654
Li, Z., Liu, Z.-B., Xing, A., Moon, B. P., Koellhoffer, J. P., Huang, L., et al. (2015). Cas9-Guide RNA directed genome editing in soybean. Plant Physiol. 169, 960–970. doi: 10.1104/pp.15.00783
Liu, L., van Tonder, T., Pietersen, G., Davies, J. W., and Stanley, J. (1997). Molecular characterization of a subgroup I geminivirus from a legume in South Africa. J. Gen. Virol. 78(Pt 8), 2113–2117. doi: 10.1099/0022-1317-78-8-2113
Liu, X., Wu, S., Xu, J., Sui, C., and Wei, J. (2017). Application of CRISPR/Cas9 in plant biology. Acta Pharm. Sin. B 7, 292–302. doi: 10.1016/j.apsb.2017.01.002
Mao, Y., Zhang, Z., Feng, Z., Wei, P., Zhang, H., Botella, J. R., et al. (2016). Development of germ-line-specific CRISPR-Cas9 systems to improve the production of heritable gene modifications in Arabidopsis. Plant Biotechnol. J. 14, 519–532. doi: 10.1111/pbi.12468
Maruyama, T., Dougan, S. K., Truttmann, M. C., Bilate, A. M., Ingram, J. R., and Ploegh, H. L. (2015). Increasing the efficiency of precise genome editing with CRISPR-Cas9 by inhibition of nonhomologous end joining. Nat. Biotechnol. 33, 538–542. doi: 10.1038/nbt.3190
Mathiasen, D. P., and Lisby, M. (2014). Cell cycle regulation of homologous recombination in Saccharomyces cerevisiae. FEMS Microbiol. Rev. 38, 172–184. doi: 10.1111/1574-6976.12066
Miao, Z. H., and Lam, E. (1995). Targeted disruption of the TGA3 locus in Arabidopsis thaliana. Plant J. Cell Mol. Biol. 7, 359–365. doi: 10.1046/j.1365-313X.1995.7020359.x
Offringa, R., de Groot, M. J., Haagsman, H. J., Does, M. P., van den Elzen, P. J., and Hooykaas, P. J. (1990). Extrachromosomal homologous recombination and gene targeting in plant cells after Agrobacterium mediated transformation. EMBO J. 9, 3077–3084.
Oppenheimer, D. G., Herman, P. L., Sivakumaran, S., Esch, J., and Marks, M. D. (1991). A myb gene required for leaf trichome differentiation in Arabidopsis is expressed in stipules. Cell 67, 483–493. doi: 10.1016/0092-8674(91)90523-2
Orel, N., Kyryk, A., and Puchta, H. (2003). Different pathways of homologous recombination are used for the repair of double-strand breaks within tandemly arranged sequences in the plant genome. Plant J. 35, 604–612. doi: 10.1046/j.1365-313X.2003.01832.x
Orel, N., and Puchta, H. (2003). Differences in the processing of DNA ends in Arabidopsis thaliana and tobacco: possible implications for genome evolution. Plant Mol. Biol. 51, 523–531. doi: 10.1023/A:1022324205661
Paszkowski, J., Baur, M., Bogucki, A., and Potrykus, I. (1988). Gene targeting in plants. EMBO J. 7, 4021–4026.
Pattanaik, S., Patra, B., Singh, S. K., and Yuan, L. (2014). An overview of the gene regulatory network controlling trichome development in the model plant, Arabidopsis. Front. Plant Sci. 5:259. doi: 10.3389/fpls.2014.00259
Peng, R., Lin, G., and Li, J. (2016). Potential pitfalls of CRISPR/Cas9-mediated genome editing. FEBS J. 283, 1218–1231. doi: 10.1111/febs.13586
Puchta, H. (1998). Repair of genomic double-strand breaks in somatic plant cells by one-sided invasion of homologous sequences. Plant J. 13, 331–339. doi: 10.1046/j.1365-313X.1998.00035.x
Puchta, H. (2002). Gene replacement by homologous recombination in plants. Plant Mol. Biol. 48, 173–182. doi: 10.1023/A:1013761821763
Puchta, H. (2005). The repair of double-strand breaks in plants: mechanisms and consequences for genome evolution. J. Exp. Bot. 56, 1–14. doi: 10.1093/jxb/eri025
Puchta, H., Dujon, B., and Hohn, B. (1993). Homologous recombination in plant cells is enhanced by in vivo induction of double strand breaks into DNA by a site-specific endonuclease. Nucleic Acids Res. 21, 5034–5040. doi: 10.1093/nar/21.22.5034
Puchta, H., Dujon, B., and Hohn, B. (1996). Two different but related mechanisms are used in plants for the repair of genomic double-strand breaks by homologous recombination. Proc. Natl. Acad. Sci. U.S.A. 93, 5055–5060. doi: 10.1073/pnas.93.10.5055
Puchta, H., and Fauser, F. (2013). Gene targeting in plants: 25 years later. Int. J. Dev. Biol. 57, 629–637. doi: 10.1387/ijdb.130194hp
Puchta, H., Swoboda, P., Gal, S., Blot, M., and Hohn, B. (1995). Somatic intrachromosomal homologous recombination events in populations of plant siblings. Plant Mol. Biol. 28, 281–292. doi: 10.1007/BF00020247
Ran, F. A., Hsu, P. D., Lin, C.-Y., Gootenberg, J. S., Konermann, S., Trevino, A. E., et al. (2013). Double nicking by RNA-Guided CRISPR Cas9 for enhanced genome editing specificity. Cell 154, 1380–1389. doi: 10.1016/j.cell.2013.08.021
Reiss, B., Schubert, I., Köpchen, K., Wendeler, E., Schell, J., and Puchta, H. (2000). RecA stimulates sister chromatid exchange and the fidelity of double-strand break repair, but not gene targeting, in plants transformed by Agrobacterium. Proc. Natl. Acad. Sci. U.S.A. 97, 3358–3363. doi: 10.1073/pnas.97.7.3358
Risseeuw, E., Offringa, R., Franke-van Dijk, M. E., and Hooykaas, P. J. (1995). Targeted recombination in plants using Agrobacterium coincides with additional rearrangements at the target locus. Plant J. Cell Mol. Biol. 7, 109–119. doi: 10.1046/j.1365-313X.1995.07010109.x
Rong, Z., Zhu, S., Xu, Y., and Fu, X. (2014). Homologous recombination in human embryonic stem cells using CRISPR/Cas9 nickase and a long DNA donor template. Protein Cell 5, 258–260. doi: 10.1007/s13238-014-0032-5
Saleh-Gohari, N., and Helleday, T. (2004). Conservative homologous recombination preferentially repairs DNA double-strand breaks in the S phase of the cell cycle in human cells. Nucleic Acids Res. 32, 3683–3688. doi: 10.1093/nar/gkh703
Sargent, R. G., Brenneman, M. A., and Wilson, J. H. (1997). Repair of site-specific double-strand breaks in a mammalian chromosome by homologous and illegitimate recombination. Mol. Cell. Biol. 17, 267–277. doi: 10.1128/MCB.17.1.267
Sauer, N. J., Narváez-Vásquez, J., Mozoruk, J., Miller, R. B., Warburg, Z. J., Woodward, M. J., et al. (2016). Oligonucleotide-Mediated genome editing provides precision and function to engineered nucleases and antibiotics in plants. Plant Physiol. 170, 1917–1928. doi: 10.1104/pp.15.01696
Schiml, S., Fauser, F., and Puchta, H. (2014). The CRISPR/Cas system can be used as nuclease for in planta gene targeting and as paired nickases for directed mutagenesis in Arabidopsis resulting in heritable progeny. Plant J. Cell Mol. Biol. 80, 1139–1150. doi: 10.1111/tpj.12704
Schiml, S., Fauser, F., and Puchta, H. (2017). CRISPR/Cas-Mediated in planta gene targeting. Methods Mol. Biol. 1610, 3–11. doi: 10.1007/978-1-4939-7003-2_1
Shaked, H., Melamed-Bessudo, C., and Levy, A. A. (2005). High-frequency gene targeting in Arabidopsis plants expressing the yeast RAD54 gene. Proc. Natl. Acad. Sci. U.S.A. 102, 12265–12269. doi: 10.1073/pnas.0502601102
Steinert, J., Schiml, S., and Puchta, H. (2016). Homology-based double-strand break-induced genome engineering in plants. Plant Cell Rep. 35, 1429–1438. doi: 10.1007/s00299-016-1981-3
Sun, Y., Zhang, X., Wu, C., He, Y., Ma, Y., Hou, H., et al. (2016). Engineering herbicide-resistant rice plants through CRISPR/Cas9-Mediated homologous recombination of acetolactate synthase. Mol. Plant 9, 628–631. doi: 10.1016/j.molp.2016.01.001
Timmermans, M. C., Das, O. P., and Messing, J. (1992). Trans replication and high copy numbers of wheat dwarf virus vectors in maize cells. Nucleic Acids Res. 20, 4047–4054. doi: 10.1093/nar/20.15.4047
Voytas, D. F. (2013). Plant genome engineering with sequence-specific nucleases. Annu. Rev. Plant Biol. 64, 327–350. doi: 10.1146/annurev-arplant-042811-105552
Wang, M., Lu, Y., Botella, J. R., Mao, Y., Hua, K., and Zhu, J.-K. (2017). Gene targeting by homology-directed repair in rice using a geminivirus-based CRISPR/Cas9 system. Mol. Plant 10, 1007–1010. doi: 10.1016/j.molp.2017.03.002
Wang, Z.-P., Xing, H.-L., Dong, L., Zhang, H.-Y., Han, C.-Y., Wang, X.-C., et al. (2015). Egg cell-specific promoter-controlled CRISPR/Cas9 efficiently generates homozygous mutants for multiple target genes in Arabidopsis in a single generation. Genome Biol. 16:144. doi: 10.1186/s13059-015-0715-0
Weigel, D., and Glazebrook, J. (2009). Quick miniprep for plant DNA isolation. Cold Spring Harb. Protoc. 2009:pdb.prot5179. doi: 10.1101/pdb.prot5179
Zetsche, B., Gootenberg, J. S., Abudayyeh, O. O., Slaymaker, I. M., Makarova, K. S., Essletzbichler, P., et al. (2015). Cpf1 is a single RNA-guided endonuclease of a Class 2 CRISPR-Cas system. Cell 163, 759–771. doi: 10.1016/j.cell.2015.09.038
Zhang, K., Raboanatahiry, N., Zhu, B., and Li, M. (2017). Progress in genome editing technology and its application in plants. Front. Plant Sci. 8:177. doi: 10.3389/fpls.2017.00177
Zhao, Y., Zhang, C., Liu, W., Gao, W., Liu, C., Song, G., et al. (2016). An alternative strategy for targeted gene replacement in plants using a dual-sgRNA/Cas9 design. Sci. Rep. 6:23890. doi: 10.1038/srep23890
Zhu, L., Mon, H., Xu, J., Lee, J. M., and Kusakabe, T. (2015). CRISPR/Cas9-mediated knockout of factors in non-homologous end joining pathway enhances gene targeting in silkworm cells. Sci. Rep. 5:18103. doi: 10.1038/srep18103
Keywords: CRISPR/Cas9, homologous recombination, Glabrous1, trichomes, gene editing, marker, in planta gene targeting, viral replicons
Citation: Hahn F, Eisenhut M, Mantegazza O and Weber APM (2018) Homology-Directed Repair of a Defective Glabrous Gene in Arabidopsis With Cas9-Based Gene Targeting. Front. Plant Sci. 9:424. doi: 10.3389/fpls.2018.00424
Received: 05 January 2018; Accepted: 16 March 2018;
Published: 05 April 2018.
Edited by:
Joachim Hermann Schiemann, Julius Kühn-Institut, GermanyReviewed by:
Holger Puchta, Karlsruher Institut für Technologie, GermanyAnne Bagg Britt, University of California, Davis, United States
Copyright © 2018 Hahn, Eisenhut, Mantegazza and Weber. This is an open-access article distributed under the terms of the Creative Commons Attribution License (CC BY). The use, distribution or reproduction in other forums is permitted, provided the original author(s) and the copyright owner are credited and that the original publication in this journal is cited, in accordance with accepted academic practice. No use, distribution or reproduction is permitted which does not comply with these terms.
*Correspondence: Andreas P. M. Weber, QW5kcmVhcy5XZWJlckB1bmktZHVlc3NlbGRvcmYuZGU=