- 1Proteomics Unit, PPGBq, Department of Biochemistry, Institute of Chemistry, Federal University of Rio de Janeiro, Rio de Janeiro, Brazil
- 2Laboratory of Proteomics, LADETEC, Institute of Chemistry, Federal University of Rio de Janeiro, Rio de Janeiro, Brazil
- 3Department of Agricultural Sciences, Federal University of Ceará, Fortaleza, Brazil
- 4Department of Biochemistry and Molecular Biology, Federal University of Ceará, Fortaleza, Brazil
Label-free quantitative proteome analysis of extrafloral (EFN) and floral nectar (FN) from castor (Ricinus communis) plants resulted in the identification of 72 and 37 proteins, respectively. Thirty proteins were differentially accumulated between EFN and FN, and 24 of these were more abundant in the EFN. In addition to proteins involved in maintaining the nectar pathogen free such as chitinases and glucan 1,3-beta-glucosidase, both proteomes share an array of peptidases, lipases, carbohydrases, and nucleases. A total of 39 of the identified proteins, comprising different classes of hydrolases, were found to have biochemical matching partners in the exudates of at least five genera of carnivorous plants, indicating the EFN and FN possess a potential to digest biological material from microbial, animal or plant origin equivalent to the exudates of carnivorous plants.
Introduction
Nectar is an energy rich substance secreted by glands situated at the base of flowers (floral nectar, FN) or in other parts such as leaves, stems, rachis, etc. (extrafloral nectar, EFN) (Shah et al., 2016). While FN attracts pollinating insects, EFN attracts aggressive ants and other mutualists, which in turn provide antiherbivore protection (Marazzi et al., 2013). Although these functional aspects are widely recognized (Roy et al., 2017), the dynamic of the relation FN/pollinators and EFN/mutualists is poorly known, especially the biochemical properties of the nectar which play a role in the attraction of particular pollinator/mutualists. Additionally, little is also known about the biochemical machinery involved in the secretion of nectar (Heil, 2015), and even less on the proteins responsible for maintaining these carbohydrate rich energy sources free of pathogens (Park and Thornburg, 2009; Heil, 2015; Roy et al., 2017). Up to now, only a limited number of studies have presented data on the proteomes of EFN and FN (Orona-Tamayo et al., 2013; Seo et al., 2013; Zhou et al., 2016). These studies demonstrated the worth of establishing the complete proteomes of EFN and FN to acquire a better understanding of the preference of certain mutualists for a particular type of EFN or FN and paved the way for establishing that proteins in nectars have roles which go beyond helping in keeping the nectar pathogen free.
The notion that the proteome of EFN is larger than FN is well established (Coulter et al., 2012) but it has not yet been tested directly. Likewise, it is still not known whether nectars from different sources possess a common set of proteins with the general role of keeping it pathogen free and a variable number of proteins conferring to a certain EFN or FN properties underlying its acceptance/rejection by mutualist animals. Last of all, the possibility that EFN and FN are involved in aspects of plant biology other than pollination and defense have not been investigated so far.
In order to address these questions, we have performed a label-free quantitative proteome analysis of EFN and FN from the castor plant (Ricinus communis) using nectar collected from plants grown under similar temporal and environmental conditions. Our analysis provides evidence for the presence in EFN and FN of a wide array of hydrolases (peptidases, carbohydrases, lipases, and nucleases) and a number of proteins related to the dismantling of the cell wall of plants and fungi. Additionally, we show that a sizable fraction of the proteins from EFN and FN have counterparts in the proteomes of the exudates of carnivorous plants.
Materials and Methods
Acquisition of Floral (FN) and Extrafloral (EFN) Nectar
Plants were grown under irrigation, in the experimental field of the Agronomy School, Federal University of Ceará, Fortaleza, Brazil. Nectar collection was performed daily from 6 to 8 a.m. (1–3 h after sunrise), by the use of a handmade glass syringe, totalizing four and three biological samples for the FN and EFN, respectively. The material collected was immediately centrifuged (10,000 g), and sterile-filtered and kept at −20°C until used.
Protein Precipitation and Trypsin Digestion
Collected FN and EFN were submitted to protein precipitation using cold acetone with 10% TCA as described (Vasconcelos et al., 2005). Precipitated proteins from both FN and EFN were solubilized in 7 M urea, 2 M thiourea. An aliquot was used to determine protein concentration by the Qubit Protein Assay Kit (Qubit® 2.0 Fluorometer, Thermo Scientific) according to the manufacturer's instructions. For protein digestion, 50 μg of proteins of each sample was reduced with dithiothreitol at a final concentration of 10 mM for 1 h at 30°C, followed by iodoacetamide alkylation at 40 mM final concentration for 30 min at room temperature in the dark. Samples were diluted with 50 mM ammonium bicarbonate to 1 M urea concentration and after trypsin addition (1:50, w/w, Sequencing Grade Modified Trypsin, V5111, Promega), solutions were incubated at 35°C for 18 h. Tryptic hydrolysis was stopped with TFA at 0.1% final concentration. After digestion peptides were concentrated and desalted by custom-made chromatographic Poros 50 R2 (PerSeptive Biosystems) reverse phase tip-columns and dried on vacuum concentrator (Thermo Scientific) (Gobom et al., 1999).
nLC-MS Analysis
Peptides resuspended in 0.1% formic acid were quantified by the Qubit Protein Assay Kit. MS analysis was performed in triplicates for each biological replicate from FN and EFN samples in a nano-LC EASY-II coupled to an LTQ-Orbitrap Velos mass spectrometer (Thermo Scientific). Two μg of peptides were loaded in a precolumn (2 cm length, 100 μm I.D., packed in-house with ReproSil-Pur C18-AQ 5 μm resin–Dr. Maisch GmbH HPLC) and fractionated in a New Objective PicoFrit® Column (25 cm length, 75 μm I.D., packed in-house with ReproSil-Pur C18-AQ 3 μm resin–Dr. Maisch GmbH HPLC). Peptides were eluted using a gradient from 95% phase A (0.1% formic acid, 5% acetonitrile) to 40% phase B (0.1% formic acid, 95% acetonitrile) for 107 min, 40–95% phase B for 5 min and 95% B for 8 min (total of 120 min at a flow rate of 200 nL/min). After each run, the column was washed with phase B and re-equilibrated with phase A. m/z spectra were acquired in a positive mode applying data-dependent automatic MS and MS/MS acquisition. MS scans (m/z 350–2,000) in the Orbitrap mass analyzer at resolution 30,000 (at m/z 400), 1 × 106 AGC and 500 ms maximum ion injection time, were followed by HCD MS/MS of the 10 most intense multiply charged ions in the Orbitrap at 10,000 signal threshold, resolution 7,500 (at m/z 400), 50,000 AGC, 300 ms maximum ion injection time, m/z 2.5 isolation width, 10 ms activation time at 30 normalized collision energy and dynamic exclusion enabled for 30 s with a repeat count of 1.
Database Search
Raw data were inspected in Xcalibur v.2.1 (Thermo Scientific). Database searches were performed using Proteome Discoverer 2.1 (Thermo Scientific) using SequestTM algorithm against Ricinus communis database downloaded from Uniprot database March 2017. The searches were performed with the following parameters: MS accuracy 10 ppm, MS/MS accuracy 0.1 Da, trypsin digestion with two missed cleavage allowed, fixed carbamidomethyl modification of cysteine and variable modification of oxidized methionine and acetyl at protein N-terminus. Protein groups and, numbers of peptides were estimated using Proteome Discoverer using false discovery rates around 1% at protein and peptide level and peptide rank. Three technical replicates were obtained for each FN and EFN samples, constituted by four and three biological replicates, respectively. Proteins were considered identified when present in at least two technical replicates for each biological replicate and in at least two biological replicates for each nectar sample. Proteins were filtered by FDR less than 1% and the presence of at least one unique peptide.
Data Analysis
Quantification was estimated using the workflow node Precursor Ions Area Detector in Proteome Discoverer. The peak area average of the most abundant distinct peptides of each protein was used for its relative quantification. Proteins with peak area averages present in at least two technical and two biological replicates were used to generate the list of proteins quantified. Normalization was executed using the total peptide amount where the total sum of the abundance values over all peptides identified within a file is used to correct the abundance in all files. Afterward, the values for the FN and EFN runs were merged and the total median was determined. A ratio of each protein between FN and EFN samples was measured and a t-test was performed to evaluate significant differences.
Proteins identified with the database description unclear or as “putative uncharacterized protein” were submitted to manual Blastp in Uniprot (http://www.uniprot.org/blast/) and NCBI (https://blast.ncbi.nlm.nih.gov) websites. Proteins with high identity were selected for the identification of uncharacterized proteins. The subcellular localization was predicted by TargetP (http://www.cbs.dtu.dk/services/TargetP/) (Emanuelsson et al., 2000) and the Top Hit Domains present in the identified proteins was evaluated by PFAM Batch sequence search (https://pfam.xfam.org/search).
Results
Label-free quantitative proteomics was employed to characterize the proteins present in the extrafloral (EFN) and floral nectars (FN) of castor plants (Ricinus communis). The proteomes of EFN and FN are populated by 72 and 37 proteins, respectively (Table 1, Table S1). For FN, 19, 11 and seven proteins were present in two, three, and four biological replicates, respectively. In the EFN, 62 and 10 proteins appeared in two and three biological replicates, respectively. From these, 30 are shared by both nectar types while 7 and 42 are restricted to FN and EFN respectively. As assessed by the TargetP software, 70% of the identified proteins have an N-terminal signal peptide for the secretory pathway (Table S1). Among the proteins unique to the EFN, 20 of them have biochemical counterparts in the FN (Table 1); however, the EFN proteome has a greater complexity in terms of diversity of kinds of enzymatic activities. Beta-fructofuranosidase (B9R9R9) an enzyme known to balance sucrose levels in the extrafloral nectar of several species is among the proteins unique to the EFN.
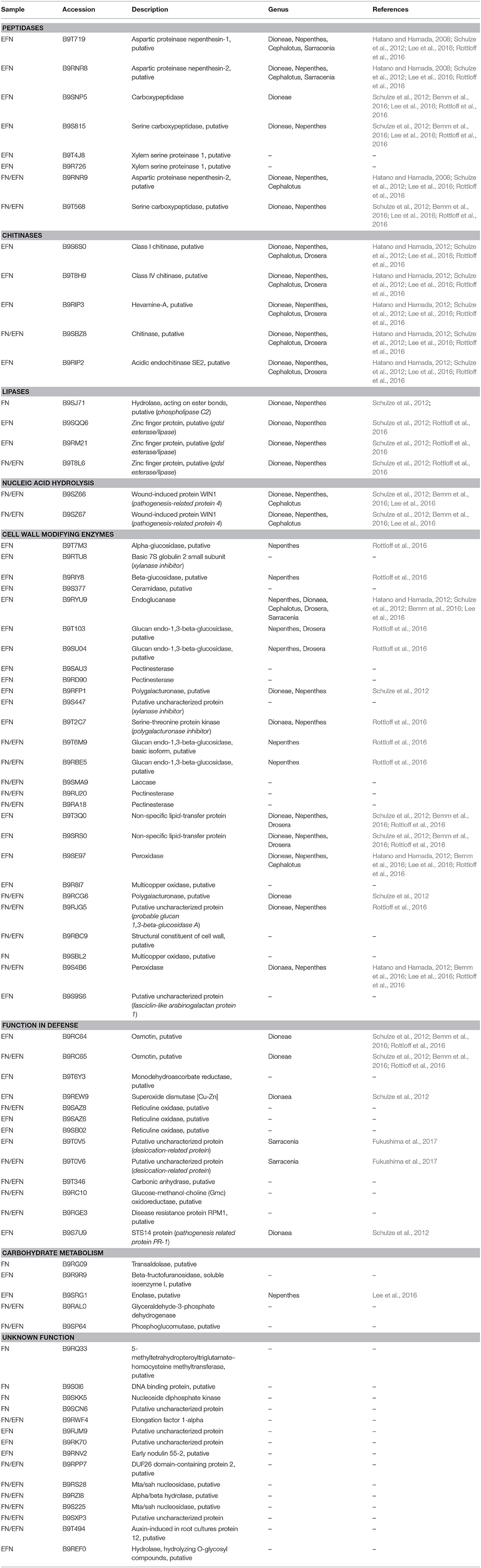
Table 1. Functional classes of proteins identified in EFN and FN proteomes from castor plants (Ricinus communis), and the genera of carnivorous plants in which counterpart proteins were identified.
Most of the proteins identified in this study are known to possess defined biochemical activity and/or physiological function in plants. Apart from 15 proteins, the remaining 64 can be tentatively sorted into the seven functional classes as shown in Table 1. Although a sizeable fraction of these proteins was previously identified in EFN and/or FN from other species, enzymes related to the dismantling of the cell wall (four pectinesterases, two polygalacturonases and one polygalacturonase inhibitor), protein hydrolysis (two xylem serine proteinases and one carboxypeptidases) have not been previously identified in any type of nectar.
The limited availability of the complete proteome of nectar from different species precludes a more precise appraisal regarding the distribution of these seven classes of proteins into EFN and FN of other plant taxa. However, as seen in Table 1, 39 out of the 79 proteins listed have identical predicted biochemical activities (biochemical matching partner) in the exudates of five genera of carnivorous plants. Apart from the proteins involved in defense functions, most of the other enzymes display hydrolytic activity against proteins, chitin, carbohydrates, lipids and nucleic acids as well as the capability of hydrolyzing/modifying components of the cell wall of plants or fungi. The presence of these enzymes provides evidence that the EFN and FN possess the enzymatic machinery to promptly digest biological material of microbial, plant or animal origin which happens to land into the floral or extrafloral nectaries.
Floral and extrafloral nectars are thought to possess a set of proteins that constitute the Carter-Thornburg nectar redox cycle, whose concerted action protect the nectar from infection (Liu and Thornburg, 2012). As Table 2 shows, only the EFN has the complete set of proteins of the Carter-Thornburg nectar redox cycle, while in FN only one enzyme (B9SAZ8) from this cycle could be identified, thus probably indicating that FN has alternative modes to avoid microbial infection. Both EFN and FN share a carbonic anhydrase (Table 1), that may act to avoid abrupt changes in the nectar pH, thus stabilizing the different enzymatic activities (see Table 2) in the nectar.
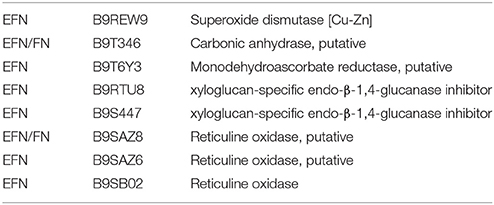
Table 2. The Carter-Thornburg redox cycle enzymes identified in the FN and EFN of castor plants (Ricinus communis).
Thirty proteins were differentially accumulated between EFN and FN (Table S1), and from these, 23 were distributed among all the seven functional classes shown in Table 1, while seven were classified as proteins of unknown function. Of the differentially expressed proteins, a total of 24 were more abundant in the EFN. A desiccation-related protein (B9T0V6) displayed the highest rate of differential expression, followed by a carbonic anhydrase B9T346 and a glucan 1,3-beta-glucosidase (B9RJG5), with a fold change of 17.4, 14.4, and 10.8 respectively. As discussed below, the functional significance of the differential expression of these proteins may bear relation to the persistent nature of the extrafloral nectary as compared to the floral nectary.
Discussion
We present here a direct comparison between the proteomes of EFN and FN from the same species, collected under similar temporal and environmental conditions. It confirms the greater complexity of the EFN, both in number of proteins species and in terms of biochemical capability, which probably underlies functional differences between the two nectar types.
The mechanisms employed to create an environment hostile to microbial infestation is a moot point in nectar biology (González-Teuber et al., 2009; Park and Thornburg, 2009; Heil, 2015; Roy et al., 2017). The task of creating an environment antagonistic to microbial infestation through the production of hydrogen peroxide seems to be one of the chosen strategies in EFN, as indicated by the presence in its proteome of a full set of proteins from the Carter-Thornburg redox-cycle (Table 2; Carter and Thornburg, 2004). The absence of these proteins in FN shows that rather than to rely on the steady production of hydrogen peroxide, the FN counts with a wide array of hydrolases, which may act in concert to ward off microbial growth. It should also be noted that in EFN the array of hydrolases is wider than in FN, indicating that EFN may rely on at least two different strategies to avoid microbial infection.
One of the most conspicuous evidence for the functional distinction between EFN and FN is the absence in the FN of a beta-fructofuranosidase. This enzyme is known to adjust the carbohydrate composition of the extrafloral nectar to exclude non-mutualistic ants (Heil et al., 2005; González-Teuber et al., 2009). The quantitative analysis we performed (Table S1), provides further support for the biochemical and functional differences between EFN and FN. Most of the differentially expressed proteins were more abundant in the EFN. A desiccation-related protein (B9T0V6), a carbonic anhydrase (B9T346) and a glucan 1, 3-beta-glucosidase (B9RJG5) were the most abundantly expressed. The desiccation-related proteins are involved in promoting the tolerance of plants to desiccation, although an alternative role as defense proteins against microorganisms has been proposed (Zha et al., 2013). Its differential accumulation in the EFN may be causally related to the long period of time in which the extrafloral nectary is metabolically active. The nectar produced at a given period if not consumed is either evaporated or reabsorbed, leading to the periodical increase in the osmotic pressure of the surface of the extrafloral nectary so that the presence of this desiccation related protein would counterbalance the detrimental biological effect of a high osmotic pressure. Carbonic anhydrase is a metalloenzyme that catalyzes the interconversion of CO2 and HCO and it is suggested to have a role in the stabilization of nectar pH (Park and Thornburg, 2009), thus propitiating the maintenance of the biochemical and functional properties of the nectar proteins; buffering nectar to a physiological pH would be essential if the enzymes in the nectar are to remain active. Finally, glucan 1, 3-beta-glucosidase (B9RJG5) has asserted roles in plant development and hold a well-characterized activity against phytopathogenic fungi (Balasubramanian et al., 2012). Again, the long-lasting nature of the extrafloral nectary as compared to the floral nectary provides a reason for the differential abundance of this protein in the EFN.
The wide array of peptidases, nucleases, lipases, cell wall modifying enzymes and chitinases found in the proteomes of EFN and FN, raises qualms about the contention that action of nectar proteins is limited to prevent microbial growth in the nectar. Apart from few proteins, notably the pectinesterases and polygalacturonases, most of the proteins identified in the proteomes of EFN and FN were previously identified in varied biochemical analysis of nectars from the castor plant and from other sources (see for example: Harper et al., 2010; Orona-Tamayo et al., 2013; Seo et al., 2013; Millán-Cañongo et al., 2014; Zha et al., 2016; Zhou et al., 2016). However, as these studies were generally focused in the identification of proteins that could have a role in maintaining the nectar a pathogen-free environment, the significance of proteins other than the classical pathogen-related proteins, was not reckoned worth of further inquiry. Our proteome analysis support to the idea that one of the roles of the nectar proteins is to prevent microbial growth, keep the nectar pH at the physiological level and provide a pH-balanced meal for visitors (Park and Thornburg, 2009). Although some of the identified proteins are known to be involved in defense reaction, most of the others cannot possibly be involved either in pathogen control or pH maintenance and therefore the adaptive role of these proteins is a question that warrants investigation.
The widely held notion that nectar represents phloem sap, does not find support in the data we present here. The proteomes of FN and EFN have not much in common with the proteomes of phloem, both in terms of number and diversity of functions of the proteins (for reviews see Carella et al., 2016; Rodríguez-Celma et al., 2016). In this context, it is relevant to point out that studies dealing with the transcriptome (Doering-Saad et al., 2006) and proteome (Barnes et al., 2004) of the phloem sap of R. communis indicated a much higher number and diversity of proteins than that we found in our proteome analysis of FN and EFN of R. communis.
As shown in Table 1, the proteomes of EFN and FN share a number of peptidases, lipases, nucleases, carbohydrases, and chitinases with the exudates of carnivorous plants. These hydrolytic enzymes act to give to the exudates the capability of digesting any prey that happens to be trapped, thus making available to the host plant sources of nitrogen, phosphorous, carbon, etc. (Ellison and Gotelli, 2009; Fukushima et al., 2017; Thorogood et al., 2017). Also shared by EFN, FN and the exudates are the proteins whose activity creates a pathogen-free environment, notably glucanases and chitinases. The identification of pectinesterases and polygalacturonases both in EFN and FN, point out a heightened potential for digesting complex carbohydrates of plant origin, including the major constituents of the cell wall. It thus appears to be likely that any biological material landing in the floral or extrafloral nectaries are liable to be digested, resulting in the production of nitrogen and carbon sources, which may be absorbed by the nectary gland and distributed throughout the plant to provide additional nutrition. This hypothesis begs for a careful experimental testing.
It is usually claimed that carnivory has evolved independently at least six times in five angiosperm orders and seems to be restricted to 0.2% of plant species (Ellison and Gotelli, 2009). However, following the report of a hitherto unknown type of herbivory in underground leaves from three Philcoxia species (Pereira et al., 2012), the authors suggested that carnivory may not be a rare trait and that the number of carnivorous plants is underestimated, thus giving support to a notion expressed years before by Chase et al. (2009), which famously claimed that “we are surrounded by murderous plants.” Therefore, whether carnivory is a pervasive trait continues to be a contentious issue, but the common features shown here between the proteomes of EFN and FN of castor plants and the proteome of exudates from carnivorous plants, adds a new twist to this debate: as a result of nectar secretion, extrafloral and floral nectaries are competent to digest biological material from animal or plant origin which land on its surface. Considering that these glands are widespread in the angiosperms and that these proteome features may be shared many other nectars, one is compelled to propose that we are indeed surrounded by “murderous plants.” Whether the hydrolytic capabilities of EFN and FN has any adaptive value and whether the carbon and nitrogen sources generated are absorbed and systemically distributed throughout the plants, are issues entreating cautious experimentation.
Mass spectrometry raw data files are available at: PRIDE Archive (https://www.ebi.ac.uk/pride/archive/) project accession PXD009104.
Author Contributions
FN and FC conceived and designed research. FN, AF, and FT conducted sample preparation and proteomics experiments. FN, AF, GD, and FC analyzed data. FN, AF, GD, and FC wrote the manuscript. All authors read and approved the manuscript.
Funding
The authors want to acknowledge the funds received from the following institutions: Brasilian National Research Council (CNPq), Brazilian Ministry of Education (CAPES), and de Janeiro State Foundation for Science Support (FAPERJ).
Conflict of Interest Statement
The authors declare that the research was conducted in the absence of any commercial or financial relationships that could be construed as a potential conflict of interest.
Acknowledgments
FC thanks CNPq for grant 302240/2016-0; AF thanks CAPES for her Ph.D. scholarship; FN for grant E-26/202.801/2015 from the Rio de Janeiro State Foundation for Science Support (FAPERJ); and GD for CNPq grant 306316/2015-3.
Supplementary Material
The Supplementary Material for this article can be found online at: https://www.frontiersin.org/articles/10.3389/fpls.2018.00549/full#supplementary-material
References
Balasubramanian, V., Vashisht, D., Cletus, J., and Sakthivel, N. (2012). Plant β-1,3-glucanases: their biological functions and transgenic expression against phytopathogenic fungi. Biotechnol. Lett. 34, 1983–1990. doi: 10.1007/s10529-012-1012-6
Barnes, A., Bale, J., Constantinidou, C., Ashton, P., Jones, A., and Pritchard, J. (2004). Determining protein identity from sieve element sap in Ricinus communis L. by quadrupole time of flight (Q-TOF) mass spectrometry. J. Exp. Bot. 55, 1473–1481. doi: 10.1093/jxb/erh161
Bemm, F., Becker, D., Larisch, C., Kreuzer, I., Escalante-Perez, M., Schulze, W. X., et al. (2016). Venus flytrap carnivorous lifestyle builds on herbivore defense strategies. Genome Res. 26, 812–825. doi: 10.1101/gr.202200.115
Carella, P., Wilson, D. C., Kempthorne, C. J., and Cameron, R. K. (2016). Vascular sap proteomics: providing insight into long-distance signaling during stress. Front. Plant Sci. 7:651. doi: 10.3389/fpls.2016.00651
Carter, C., and Thornburg, R. W. (2004). Is the nectar redox cycle a floral defense against microbial attack? Trends Plant Sci. 9, 320–324. doi: 10.1016/j.tplants.2004.05.008
Chase, M. W., Christenhusz, M. J. M., Sanders, D., and Fay, M. F. (2009). Murderous plants: victorian Gothic, Darwin and modern insights into vegetable carnivory. Bot. J. Linn. Soc. 161, 329–356. doi: 10.1111/j.1095-8339.2009.01014.x
Coulter, A., Poulis, B. A. D., and von Aderkas, P. (2012). Pollination drops as dynamic apoplastic secretions. Flora Morphol. Distrib. Funct. Ecol. Plants 207, 482–490. doi: 10.1016/j.flora.2012.06.004
Doering-Saad, C., Newbury, H. J., Couldridge, C. E., Bale, J. S., and Pritchard, J. (2006). A phloem-enriched cDNA library from Ricinus: insights into phloem function. J. Exp. Bot. 57, 3183–3193. doi: 10.1093/jxb/erl082
Ellison, A. M., and Gotelli, N. J. (2009). Energetics and the evolution of carnivorous plants - Darwin's “most wonderful plants in the world.” J. Exp. Bot. 60, 19–42. doi: 10.1093/jxb/ern179
Emanuelsson, O., Nielsen, H., Brunak, S., and von Heijne, G. (2000). Predicting subcellular localization of proteins based on their N-terminal amino acid sequence. J. Mol. Biol. 300, 1005–1016. doi: 10.1006/jmbi.2000.3903
Fukushima, K., Fang, X., Alvarez-Ponce, D., Cai, H., Carretero-Paulet, L., Chen, C., et al. (2017). Genome of the pitcher plant Cephalotus reveals genetic changes associated with carnivory. Nat. Ecol. Evol. 1, 1–9. doi: 10.1038/s41559-016-0059
Gobom, J., Nordhoff, E., Mirgorodskaya, E., Ekman, R., and Roepstorff, P. (1999). Sample purification and preparation technique based on nano-scale reversed-phase columns for the sensitive analysis of complex peptide mixtures by matrix-assisted laser desorption/ionization mass spectrometry. J Mass Spectrom. 34, 105–116. doi: 10.1002/(SICI)1096-9888(199902)34:2<105::AID-JMS768>3.0.CO;2-4
González-Teuber, M., Eilmus, S., Muck, A., Svatos, A., and Heil, M. (2009). Pathogenesis-related proteins protect extrafloral nectar from microbial infestation. Plant J. 58, 464–473. doi: 10.1111/j.1365-313X.2009.03790.x
Harper, A. D., Stalnaker, S. H., Wells, L., Darvill, A., Thornburg, R., and York, W. S. (2010). Interaction of nectarin 4 with a fungal protein triggers a microbial surveillance and defense mechanism in nectar. Phytochemistry 71, 1963–1969. doi: 10.1016/j.phytochem.2010.09.009
Hatano, N., and Hamada, T. (2012). Proteomic analysis of secreted protein induced by a component of prey in pitcher fluid of the carnivorous plant Nepenthes alata. J. Proteomics 75, 4844–4852. doi: 10.1016/j.jprot.2012.05.048
Hatano, N., and Hamada, T. (2008). Proteome analysis of pitcher fluid of the carnivorous plant Nepenthes alata. J. Proteome Res. 7, 809–816. doi: 10.1021/pr700566d
Heil, M. (2015). Extrafloral nectar at the plant-insect interface: a spotlight on chemical ecology, phenotypic plasticity, and food webs. Annu. Rev. Entomol. 60, 213–232. doi: 10.1146/annurev-ento-010814-020753
Heil, M., Rattke, J., and Boland, W. (2005). Postsecretory hydrolysis of nectar sucrose and specialization in ant/plant mutualism. Science 308, 560–563. doi: 10.1126/science.1107536
Lee, L., Zhang, Y., Ozar, B., Sensen, C. W., and Schriemer, D. C. (2016). Carnivorous nutrition in pitcher plants (Nepenthes spp.) via an unusual complement of endogenous enzymes. J. Proteome Res. 15, 3108–3117. doi: 10.1021/acs.jproteome.6b00224
Liu, G., and Thornburg, R. W. (2012). Knockdown of MYB305 disrupts nectary starch metabolism and floral nectar production. Plant J. 70, 377–388. doi: 10.1111/j.1365-313X.2011.04875.x
Marazzi, B., Bronstein, J. L., and Koptur, S. (2013). The diversity, ecology and evolution of extrafloral nectaries: Current perspectives and future challenges. Ann. Bot. 111, 1243–1250. doi: 10.1093/aob/mct109
Millán-Cañongo, C., Orona-Tamayo, D., and Heil, M. (2014). Phloem sugar flux and jasmonic acid-responsive cell wall invertase control extrafloral nectar secretion in Ricinus communis. J. Chem. Ecol. 40, 760–769. doi: 10.1007/s10886-014-0476-3
Orona-Tamayo, D., Wielsch, N., Escalante-Pérez, M., Svatos, A., Molina-Torres, J., Muck, A., et al. (2013). Short-term proteomic dynamics reveal metabolic factory for active extrafloral nectar secretion by Acacia cornigera ant-plants. Plant J. 73, 546–554. doi: 10.1111/tpj.12052
Park, S., and Thornburg, R. W. (2009). Biochemistry of nectar proteins. J. Plant Biol. 52, 27–34. doi: 10.1007/s12374-008-9007-5
Pereira, C. G., Almenara, D. P., Winter, C. E., Fritsch, P. W., Lambers, H., and Oliveira, R. S. (2012). Underground leaves of Philcoxia trap and digest nematodes. Proc. Natl. Acad. Sci. U.S.A. 109, 1154–1158. doi: 10.1073/pnas.1114199109
Rodríguez-Celma, J., Ceballos-Laita, L., Grusak, M. A., Abadía, J., and López-Millán, A. F. (2016). Plant fluid proteomics: delving into the xylem sap, phloem sap and apoplastic fluid proteomes. Biochim. Biophys. Acta. 1864, 991–1002. doi: 10.1016/j.bbapap.2016.03.014
Rottloff, S., Miguel, S., Biteau, F., Nisse, E., Hammann, P., Kuhn, L., et al. (2016). Proteome analysis of digestive fluids in Nepenthes pitchers. Ann. Bot. 117, 479–495. doi: 10.1093/aob/mcw001
Roy, R., Schmitt, A. J., Thomas, J. B., and Carter, C. J. (2017). Review: nectar biology: from molecules to ecosystems. Plant Sci. 262, 148–164. doi: 10.1016/j.plantsci.2017.04.012
Schulze, W. X., Sanggaard, K. W., Kreuzer, I., Knudsen, A. D., Bemm, F., Thøgersen, I. B., et al. (2012). The protein composition of the digestive fluid from the venus flytrap sheds light on prey digestion mechanisms. Mol. Cell. Proteomics 11, 1306–1319. doi: 10.1074/mcp.M112.021006
Seo, P. J., Wielsch, N., Kessler, D., Svatos, A., Park, C. M., Baldwin, I. T., et al. (2013). Natural variation in floral nectar proteins of two Nicotiana attenuata accessions. BMC Plant Biol. 13:101. doi: 10.1186/1471-2229-13-101
Shah, M., Teixeira, F. M., Soares, E. L., Soares, A. A., Carvalho, P. C., Domont, G. B., et al. (2016). Time-course proteome analysis of developing extrafloral nectaries of Ricinus communis. Proteomics 16, 629–633. doi: 10.1002/pmic.201500292
Thorogood, C. J., Bauer, U., and Hiscock, S. J. (2017). Convergent and divergent evolution in carnivorous pitcher plant traps. New Phytol. 217, 1035–1041. doi: 10.1111/nph.14879
Vasconcelos, É. A. R., Nogueira, F. C. S., Abreu, E. F. M., et al. (2005). Protein extraction from cowpea tissues for 2-D gel electrophoresis and MS analysis. Chroma. 62, 447–450. doi: 10.1365/s10337-005-0637-1
Zha, H. G., Liu, T., Zhou, J. J., and Sun, H. (2013). MS-desi, a desiccation-related protein in the floral nectar of the evergreen velvet bean (Mucuna sempervirens Hemsl): molecular identification and characterization. Planta 238, 77–89. doi: 10.1007/s00425-013-1876-2
Zha, H. G., Milne, R. I., Zhou, H. X., Chen, X. Y., and Sun, H. (2016). Identification and cloning of class II and III chitinases from alkaline floral nectar of Rhododendron irroratum, Ericaceae. Planta 244, 805–818. doi: 10.1007/s00425-016-2546-y
Keywords: floral nectar, extrafloral nectar, carnivorous plants, Ricinus communis, proteomics
Citation: Nogueira FCS, Farias ARB, Teixeira FM, Domont GB and Campos FAP (2018) Common Features Between the Proteomes of Floral and Extrafloral Nectar From the Castor Plant (Ricinus Communis) and the Proteomes of Exudates From Carnivorous Plants. Front. Plant Sci. 9:549. doi: 10.3389/fpls.2018.00549
Received: 05 February 2018; Accepted: 09 April 2018;
Published: 27 April 2018.
Edited by:
Robert W. Thornburg, Iowa State University, United StatesReviewed by:
Elisabeth Jamet, Université Toulouse III - Paul Sabatier, FranceR. Glen Uhrig, University of Alberta, Canada
Copyright © 2018 Nogueira, Farias, Teixeira, Domont and Campos. This is an open-access article distributed under the terms of the Creative Commons Attribution License (CC BY). The use, distribution or reproduction in other forums is permitted, provided the original author(s) and the copyright owner are credited and that the original publication in this journal is cited, in accordance with accepted academic practice. No use, distribution or reproduction is permitted which does not comply with these terms.
*Correspondence: Gilberto B. Domont, Z2lsYmVydG9AaXEudWZyai5icg==
Francisco A. P. Campos, YmlvcGxhbnRAdWZjLmJy